- UNAM—Institute of Materials Science and Nanotechnology, Bilkent University, Ankara, Türkiye
Semiconductors-core optical fibers have gathered attention for light guidance in the infrared spectrum. Cladded with glasses, fibers can be the ideal medium to transfer the favorable bulk properties of semiconductors into the micro/nano scaled one-dimensional form. The integration of these fibers with optical circuits, lasers and photonic crystals offers a wide variety of applications. In this perspective, the role of semiconductors in the future of optical fibers and their integration with photonic crystal structures are analyzed. The past and present efforts of semiconductor-core fibers are briefly reviewed, and the potential future application areas benefited by semiconductors as fiber materials are discussed.
1 Introduction
Semiconductors dramatically altered the progress of technology in the 1940s through the successful demonstration of the first transistor. The roles in electronic circuits, solar cells, light emitting diodes (LED) and lasers are some of the key applications that have provided the undisputable importance of semiconductors in today’s world. On the other hand, the bulk properties of the semiconductors also show promising applications in optics and photonics for the infrared (IR) spectrum. The usage of semiconductors as the core material of optical fibers was introduced within the first decade of the new millennia [1]. Semiconductors such as Si, Ge, SiGe, ZnSe, and SeTe have demonstrated light guidance in the near-IR and mid-IR regions, and many others have been proposed as fiber materials [2]. The high nonlinearity of semiconductors, that is, several orders of magnitude higher than the sonly ilica-based glasses, can demonstrate various optical phenomena as a core medium in optical fibers [2,3].
Photonic crystal fibers (PCFs) are 2-dimensionally microstructured optical fibers that can manipulate light via the periodic arrays of air holes and glass struts [4]. Controlling the light by tailoring a microstructured cladding around a core was a breakthrough for optical fibers, and research activities have accelerated in this newly born fiber group. Seminal works such as photonic bandgap guidance [5], endlessly single-mode operation [6] and later large mode area single-mode fiber [7] were enabled with PCFs. Following the discovery of PCFs, Hollow-core photonic crystal fibers (HC-PCF, or HCFs shortly in this work), a subgroup of PCFs, were introduced to guide the light through a low-index medium, i.e., hollow-core [8]. The discovery of air guidance with HCFs has led to the demonstrations of strong light-matter interaction, high damage threshold and reduced latency [9]. A high figure of merit, ratio of effective interaction length to the modal area, is a critical advantage of HCFs for the demonstration of nonlinear phenomena. Moreover, light guidance through the HCFs differentiates from the solid-core fiber that operates by the total internal reflection showing the uniqueness of HCFs for the fiber community. The capability of ultralow-loss guidance through the air core, that has lower theoretical limits than glasses, have been shown by surpassing the fundamental Rayleigh limit in the visible light region [10]. This achievement can potentially lead the way to help outperform the solid-core silica optical fibers “telecom fiber” with HCFs in the near-IR region where the majority of data around the world is transmitted [11].
In this perspective, the role of semiconductors in the future of optical fibers and the opportunities in the intersection of PCF/HCF and semiconductors are discussed. First, the historical progress of semiconductors as fiber materials and their present status are briefly reviewed including types of fibers, materials and fabrication techniques. Later, a discussion on the possible future directions of semiconductor-core fibers is presented by emphasizing the integration of semiconductors with PCFs/HCFs.
2 Past and present of the semiconductor-core fibers
The initial works on the light guidance properties of semiconductors were performed via on-chip waveguides and circuit components for integrated circuit applications [12]. Over time, waveguides made out of a variety of semiconductors such as silicon, germanium and III-nitrides have been proposed for near-IR and mid-IR regions [13]. The transition from waveguide to fiber was realized by the introduction of two novel fabrication methods, namely, high-pressure chemical vapor deposition (HP-CVD) technique and the molten core (MC) method [1,14]. A semiconductor-core glass cladded fiber can be formed either by chemical deposition of semiconductors in micron-sized capillaries (in the case of HP-CVD) or directing molten semiconductors through viscous glasses as in the standard fiber drawing (in the case of MC method). Among the two fabrication methods, the MC method is more favorable due to the wide range of material selection and capability of long-length and low-loss fiber fabrication in a shorter time period. Also, different forms of materials such as rod, powder and pellet can be used to draw fibers by the MC method. In a semiconductor-core fiber, light is guided via the total internal reflection by forming refractive index differences between the core and cladding regions. So far, semiconductors such as Si [14], Ge [15], SiGe [16], ZnSe [17], SeTe [18] and Se [19] were fabricated and demonstrated IR light guidance. Although several other promising semiconductors such as InSb, GaSb, Te, Se, InSe, GeSe, BiTe, and GaAs were proposed as the core material of fibers, to date, the successful demonstration of optical transmission has not been achieved yet [2,20].
Since most of the studied semiconductors as core materials for fibers are transparent in near-IR and mid-IR regions, optical characterizations have been focused on the IR spectrum to analyze the optical performance of the fabricated fibers. Light guidance in a broad spectrum spanning from 1 to 10.6 µm was investigated through semiconductor-core fibers [21,22]. Although theoretically and numerically semiconductor-core fibers have low optical losses, the lowest measured losses are in the order of 0.1–1 dB/cm. This could be attributed to the fabrication-related defects such as cracks, ellipticity and nonuniform core-cladding interfaces and impurities mostly diffused from the cladding [23,24]. The mismatch between the coefficient of thermal expansion (CTE) of core and cladding materials is the leading cause of the formation of defects. Furthermore, the polycrystalline structure of the semiconductor-core fibers that mostly occur after the fabrication contributes to additional losses due to the compositional fluctuations through the grain boundaries, especially in the multi-material fibers. To minimize the effect of fabrication or structure-related defects, several processes have been proposed. Laser recrystallization of the fiber core facilitates the annihilation of the grain boundaries by the single crystal formation [16,25,26]. Thermal annealing of the fibers to further improve the morphology by redistributing the elements within alloy cores has improved the optical performance of fibers [27]. Another strategy involves the introduction of an alkali oxide interface layer formed between the core and cladding to lower the thermal strain during the fiber drawing [28].
Over the last two decades, the rapid advancement in PCFs, and more specifically in the HCF subgroup, have also created the possibility for the integration of semiconductors in PCFs. The spectacular optical performances of PCFs could enable further improvement or demonstration of new optical phenomena with the semiconductor-core fibers. The air holes of HCFs made out of silica-based glasses can be a suitable host for semiconductors. So far, the integration of semiconductors and PCFs has been demonstrated as Si, Ge and SiGe filling of cladding tubes [29], full-infiltration of the core and cladding with Si [30] and coating of the inner layer of a hollow-core with Si [31]. Further exploration of PCFs to demonstrate low-loss light guidance and novel applications with semiconductors will open new avenues of applications of optical fibers.
3 Future of the semiconductor-core fibers
It has been less than 20 years since light guidance through semiconductor-core fibers has been demonstrated. In the intervening period, these fibers have been the subject of extensive investigation not only for their optical properties but also their thermoelectric, optoelectronic and photovoltaic properties. In the near future, we may expect to see further applications of semiconductor-core fibers [32]. Figure 1 shows the currently developed fibers including the solid-core and semiconductor-infilled fibers. Moreover, several potential design proposals for future demonstration are also provided. Although the optical losses of semiconductor-core fibers are comparable with the planar semiconductor waveguides, their losses are still relatively high to further expand the applications. Recrystallization of the core, graded-index profiling and tapering are some of the techniques to further improve the optical performance of the fibers. On the other hand, they may be good candidates for several applications that are possible even with the present optical performances. The following topics are some of the opportunities for the future of semiconductor-core fibers.
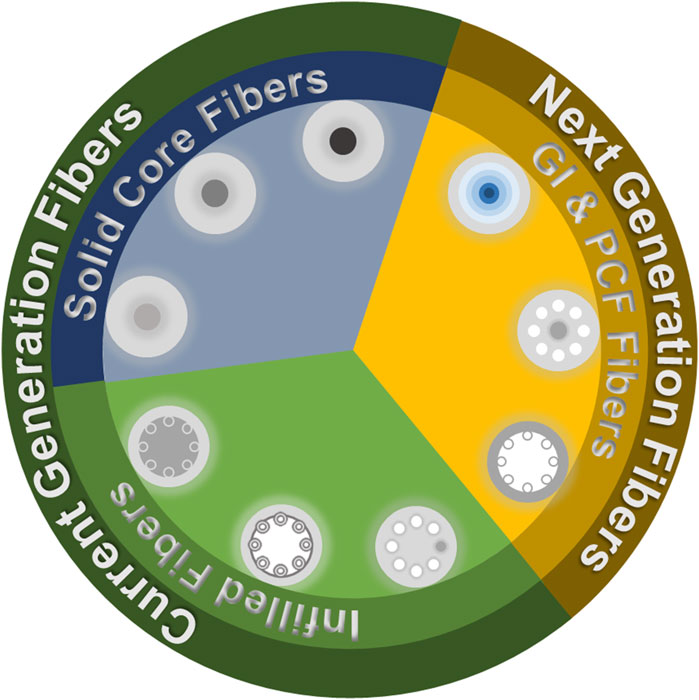
FIGURE 1. The present and future of semiconductor-core fibers. Solid-core semiconductors (single components, alloys and compounds) and semiconductor infilled fibers are demonstrated with various materials and designs. The next-generation fibers may include the graded-index (GI) structure and semiconductor-PCF integration.
3.1 Mid-IR guidance
Silica-based glasses have high material absorption beyond the near-IR region, and alternative materials such as chalcogenides, fluorides and telluride glasses have been proposed for IR light guidance [33]. On the other hand, the favorable bulk mechanical and optical properties of semiconductors have attracted attention for IR guidance. The wide transmission band of semiconductors allows guidance in the spectral region that covers most of the near-IR and mid-IR. Further improvement for the semiconductor-core fibers that can lower the losses to the range of 0.1–1 dB/m can position these fibers for practical consideration. A recent study has shown that losses under 0.2 dB/cm are possible with a 3.3 µm diameter Si-core in the near-IR region [34]. Furthermore, forming a graded-index (GI) structure to confine the light in the inner part of the core to decrease the interaction of the guided light with the highly absorbing cladding could be another solution to reduce the losses and expand the transmission bandwidth [27]. The radial compositional difference was observed with an alloy semiconductor-core, but further studies are needed for a robust GI optical fiber [35].
Another strategy to lower the losses is to develop a cladding glass that fits better optically and mechanically with the semiconductor cores. So far, the demonstrated semiconductor-core fibers have glass claddings that absorb IR light, resulting in elevated losses due to the interaction of the evanescent field of the guided light with the absorbing cladding medium. IR transparent glasses such as chalcogenides or fluorides may lower the losses as the cladding of the semiconductor-cores. However, the working temperature of these glasses is generally lower than the melting point of semiconductors preventing the combination. Several studies were focused to develop specialized cladding glasses to match the coefficient of thermal expansion and melting/working temperature of core/clad materials [36–38]. However, these studies generally lack optical characterization to demonstrate the performance of the fibers. A mechanically robust and at the same time IR transparent cladding glass would substantially improve the optical performance of semiconductor-core fibers.
3.2 Semiconductor integrated photonic crystal fibers
As mentioned in the previous section, several works have demonstrated the integration of semiconductors in PCFs. Figure 1 illustrates two additional ways to integrate semiconductors with PCFs. The first design proposed a microstructured cladding around a semiconductor to further decrease the losses by 1) tailoring the average refractive index in the cladding and 2) forming a photonic bandgap structure. The combination of air holes that are transparent to the IR radiation and glass struts forms a lower average refractive index and improves the IR transparency of the cladding. However, a thorough study is necessary to find the optimal features of air hole and glass strut to primarily form the bandgap. Secondly, the fabrication of all-semiconductor PCFs may expand the usage of semiconductor fibers since the losses will alter radically in an IR transparent medium. The fabrication of this ideal structure with the aforementioned fabrication techniques is challenging for any PCF design. Stating that, one way to fabricate all-semiconductor PCF can be using a highly precise additive manufacturing technique specialized for semiconductors [39]. A recent study has demonstrated the successful fabrication of PCFs with micron-sized features by utilizing a polymer-based additive manufacturing technique [40]. A similar additive manufacturing method capable of large-scale fabrication of semiconductor fibers will help the realization of the proposed design, and thus will have substantial effects on the semiconductor fibers.
3.3 Nonlinear optics
The nonlinearity of semiconductors, known to be several orders of magnitude higher than silica glasses, is expected to play a major role in the future of optical fibers. Recent efforts have shown the potential of these fibers for nonlinear optical applications. For instance, supercontinuum generation with silica solid-core PCFs generally requires several meters of fibers to generate a wide spectrum. On the other hand, only a couple of centimeters long semiconductor-core fiber is enough to show a similar spectral band. To date, detuning oscillations [41], supercontinuum generation [42], stimulated Raman amplification [43] and frequency comb generation [44] are some of the nonlinear phenomena demonstrated with semiconductor-core fibers. Further improvement in the design of these fibers will help the exploration of other nonlinear effects.
3.4 Integration of fibers in photonic circuit
Simultaneous usage of semiconductor-core fibers with silica optical fibers or on-circuit semiconductor waveguides may benefit telecommunication applications. Tip-to-tip direct coupling from the semiconductor-core fibers to silica optical fibers by tapering both of the fibers has already been demonstrated [45]. Although numerically calculated coupling loss is under 1 dB, the experimentally demonstrated value is around 4 dB that hinders the usage of the semiconductor-core fibers. Further optimization of the fabrication may help to improve the coupling efficiency. On the other hand, a flexible and long-length (therefore low-loss) fiber with matching optical parameters with waveguides in the integrated circuits could be advantageous to connect multiple circuits without any intermediate structure. On-circuit free-space or contact coupling between waveguides with either the same or different materials has been demonstrated [46,47]. This could be achieved with the semiconductor-core fibers that have better matching optical properties with on-chip structures. However, engineering the fiber/waveguide to lower the reflection loss, reduce large mode mismatch and improve the coupling efficiency is vital for successful integration.
3.5 Other applications
Semiconductors are generally opaque in the ultraviolet (UV) and visible regions, but they can be used to guide light in the THz frequency region [48]. On the other hand, the superior bulk properties of the semiconductors are not limited only with the optical demonstrations [32]. It is worth mentioning that, an emerging field for the semiconductor-core fibers to be considered is energy harvesting [49–52]. This field can be utilized for wearable electronic applications.
In conclusion, semiconductors have had an enormous impact on the recent history of human society through numerous critical applications. The utilization of semiconductors as a light-guiding medium for optical fibers has carried the demonstration of them for alternative purposes. The exploitation of favorable bulk properties of semiconductors in PCFs is expected to play a significant role in the future of optical fibers.
Data availability statement
The raw data supporting the conclusion of this article will be made available by the authors, without undue reservation.
Author contributions
The manuscript was prepared solely by MO.
Acknowledgments
I would like to thank M. M. Hasan for the realization of Figure 1.
Conflict of interest
The authors declare that the research was conducted in the absence of any commercial or financial relationships that could be construed as a potential conflict of interest.
Publisher’s note
All claims expressed in this article are solely those of the authors and do not necessarily represent those of their affiliated organizations, or those of the publisher, the editors and the reviewers. Any product that may be evaluated in this article, or claim that may be made by its manufacturer, is not guaranteed or endorsed by the publisher.
References
1. Sazio PJA, Amezcua-Correa A, Finlayson CE, Hayes JR, Scheidemantel TJ, Baril NF, et al. Microstructured optical fibers as high-pressure microfluidic reactors. Science (2006) 311:1583–6. doi:10.1126/science.1124281
2. Ordu M, Basu SN. Recent progress in germanium-core optical fibers for mid-infrared optics. Infrared Phys Technol (2020) 111:103507. doi:10.1016/j.infrared.2020.103507
3. Shen L, Ren H, Huang M, Wu D, Peacock AC. A review of nonlinear applications in silicon optical fibers from telecom wavelengths into the mid-infrared spectral region. Opt Commun (2020) 463:125437. doi:10.1016/j.optcom.2020.125437
5. Knight JC, Birks TA, Russell PSJ, Atkin DM. All-silica single-mode optical fiber with photonic crystal cladding. Opt Lett (1996) 21:1547–9. doi:10.1364/OL.21.001547
6. Birks TA, Knight JC, Russell PSJ. Endlessly single-mode photonic crystal fiber. Opt Lett (1997) 22:961–3. doi:10.1364/OL.22.000961
7. Knight JC, Birks TA, Cregan RF, Russell PSJ, de Sandro JP. Large mode area photonic crystal fibre. Electron Lett (1998) 34:1347–8. doi:10.1049/el:19980965
8. Cregan RF, Mangan BJ, Knight JC, Birks TA, Russell PSJ, Roberts PJ, et al. Single-mode photonic band gap guidance of light in air. Science (1999) 285:1537–9. doi:10.1126/science.285.5433.1537
9. Debord B, Amrani F, Vincetti L, Gérôme F, Benabid F. Hollow-core fiber technology: The rising of “gas photonics”. Fibers (2019) 7:16. doi:10.3390/fib7020016
10. Gao S, Wang Y, Ding W, Hong Y, Wang P. Conquering the Rayleigh scattering limit of silica glass fiber at visible wavelengths with a hollow-core fiber approach. Laser Photon Rev (2020) 14:1900241. doi:10.1002/lpor.201900241
11. Jasion GT, Sakr H, Hayes JR, Sandoghchi SR, Hooper L, Fokoua EN, et al. 0.174 dB/km hollow core double nested antiresonant nodeless fiber (DNANF). In: Proceeding of the 2022 Optical Fiber Communications Conference and Exhibition (OFC); March 2022; San Diego, CA, USA. IEEE (2022). p. 1–3.
12. Soref R. The past, present, and future of silicon photonics. IEEE J Sel Top Quan Electron. (2006) 12:1678–87. doi:10.1109/JSTQE.2006.883151
13. Soref R. Mid-infrared photonics in silicon and germanium. Nat Photon (2010) 4:495–7. doi:10.1038/nphoton.2010.171
14. Ballato J, Hawkins T, Foy P, Stolen R, Kokuoz B, Ellison M, et al. Silicon optical fiber. Opt Express (2008) 16:18675–83. doi:10.1364/OE.16.018675
15. Ballato J, Hawkins T, Foy P, Morris S, Hon NK, Jalali B, et al. Silica-clad crystalline germanium core optical fibers. Opt Lett (2011) 36:687–8. doi:10.1364/OL.36.000687
16. Coucheron DA, Fokine M, Patil N, Breiby DW, Buset OT, Healy N, et al. Laser recrystallization and inscription of compositional microstructures in crystalline SiGe-core fibres. Nat Commun (2016) 7:13265. doi:10.1038/ncomms13265
17. Sparks JR, He R, Healy N, Krishnamurthi M, Peacock AC, Sazio PJA, et al. Zinc selenide optical fibers. Adv Mater (2011) 23:1647–51. doi:10.1002/adma.201003214
18. Tang G, Qian Q, Wen X, Chen X, Liu W, Sun M, et al. Reactive molten core fabrication of glass-clad Se0.8Te0.2 semiconductor core optical fibers. Opt Express (2015) 23:23624–33. doi:10.1364/OE.23.023624
19. Peng S, Tang G, Huang K, Qian Q, Chen D, Zhang Q, et al. Crystalline selenium core optical fibers with low optical loss. Opt Mater Express (2017) 7:1804–12. doi:10.1364/OME.7.001804
20. Zaengle T, Gibson UJ, Hawkins TW, McMillen C, Ghimire B, Rao AM, et al. A novel route to fibers with incongruent and volatile crystalline semiconductor cores: GaAs. ACS Photon (2022) 9:1058–64. doi:10.1021/acsphotonics.2c00008
21. Mehta P, Krishnamurthi M, Healy N, Baril NF, Sparks JR, Sazio PJA, et al. Mid-infrared transmission properties of amorphous germanium optical fibers. Appl Phys Lett (2010) 97:071117. doi:10.1063/1.3481413
22. Ordu M, Guo J, Tai B, Hong MK, Erramilli S, Ramachandran S, et al. Mid-infrared transmission through germanium-core borosilicate glass-clad semiconductor fibers. Opt Mater Express (2017) 7:3107–15. doi:10.1364/OME.7.003107
23. Ballato J, Hawkins T, Foy P, Yazgan-Kokuoz B, McMillen C, Burka L, et al. Advancements in semiconductor core optical fiber. Opt Fiber Technol (2010) 16:399–408. doi:10.1016/j.yofte.2010.08.006
24. Syed MW, Akosman AE, Ordu M. Numerical investigation of confinement losses in semiconductor-core optical fibers. In: Proceeding of the 2021 IEEE Research and Applications of Photonics in Defense Conference (RAPID); August 2021; Miramar Beach, FL, USA. IEEE (2021). p. 1–2. doi:10.1109/RAPID51799.2021.9521383
25. Ji X, Page RL, Chaudhuri S, Liu W, Yu S-Y, Mohney SE, et al. Single-crystal germanium core optoelectronic fibers. Adv Opt Mater (2017) 5:1600592. doi:10.1002/adom.201600592
26. Ji X, Lei S, Yu S-Y, Cheng HY, Liu W, Poilvert N, et al. Single-crystal silicon optical fiber by direct laser crystallization. ACS Photon (2017) 4:85–92. doi:10.1021/acsphotonics.6b00584
27. Ordu M, Guo J, Akosman AE, Erramilli S, Ramachandran S, Basu SN. Effect of thermal annealing on mid-infrared transmission in semiconductor alloy-core glass-cladded fibers. Adv Fiber Mater (2020) 2:178–84. doi:10.1007/s42765-020-00030-2
28. Nordstrand EF, Dibbs AN, Eraker AJ, Gibson UJ. Alkaline oxide interface modifiers for silicon fiber production. Opt Mater Express (2013) 3:651–7. doi:10.1364/OME.3.000651
29. Tyagi HK, Schmidt MA, Sempere LP, Russell PSJ. Optical properties of photonic crystal fiber with integral micron-sized Ge wire. Opt Express (2008) 16:17227–36. doi:10.1364/OE.16.017227
30. Healy N, Sparks JR, Petrovich MN, Sazio PJA, Badding JV, Peacock AC. Large mode area silicon microstructured fiber with robust dual mode guidance. Opt Express (2009) 17:18076–82. doi:10.1364/OE.17.018076
31. Belardi W, Lucia FD, Poletti F, Sazio PJ. Composite material hollow antiresonant fibers. Opt Lett (2017) 42:2535–8. doi:10.1364/OL.42.002535
32. Gibson UJ, Wei L, Ballato J. Semiconductor core fibres: Materials science in a bottle. Nat Commun (2021) 12:3990. doi:10.1038/s41467-021-24135-3
33. Tao G, Ebendorff-Heidepriem H, Stolyarov AM, Danto S, Badding JV, Fink Y, et al. Infrared fibers. Adv Opt Photon (2015) 7:379–458. doi:10.1364/AOP.7.000379
34. Kudinova M, Bouwmans G, Vanvincq O, Habert R, Plus S, Bernard R, et al. Two-step manufacturing of hundreds of meter-long silicon micrometer-size core optical fibers with less than 0.2 dB/cm background losses. APL Photon (2021) 6:026101. doi:10.1063/5.0028195
35. Wu W, Balci M, Song S, Liu C, Fokine M, Laurell F, et al. CO2 laser annealed SiGe core optical fibers with radial Ge concentration gradients. Opt Mater Express (2020) 10:926–36. doi:10.1364/OME.390482
36. Morris S, Hawkins T, Foy P, Ballato J, Martin SW, Rice R. Cladding glass development for semiconductor core optical fibers. Int J Appl Glass Sci (2012) 3:144–53. doi:10.1111/j.2041-1294.2012.00085.x
37. Dmitrieva I, Lopez-Iscoa P, Milanese D, Petit L. Ternary borosilicates as potential cladding glasses for semiconductor core optical fibers. Int J Appl Glass Sci (2019) 10:151–6. doi:10.1111/ijag.12874
38. Mathewson C, Urbina I, Badding JV, Gopalan V, Mauro JC. Aluminosilicate glasses for zinc selenide tunable fiber laser cladding. J Am Ceram Soc (2021) 104:691–6. doi:10.1111/jace.17471
39. Saengchairat N, Tran T, Chua C-K. A review: Additive manufacturing for active electronic components. Virtual Phys Prototyp (2017) 12:31–46. doi:10.1080/17452759.2016.1253181
40. Bertoncini A, Liberale C, Liberale C. 3D printed waveguides based on photonic crystal fiber designs for complex fiber-end photonic devices. Optica (2020) 7:1487–94. doi:10.1364/OPTICA.397281
41. Ordu M, Guo J, Ng Pack G, Shah P, Ramachandran S, Hong MK, et al. Nonlinear optics in germanium mid-infrared fiber material: Detuning oscillations in femtosecond mid-infrared spectroscopy. AIP Adv (2017) 7:095125. doi:10.1063/1.5003027
42. Peacock AC, Campling J, Runge AFJ, Ren H, Shen L, Aktas O, et al. Wavelength conversion and supercontinuum generation in silicon optical fibers. IEEE J Sel Top Quan Electron. (2018) 24:1–9. doi:10.1109/JSTQE.2017.2762958
43. Huang M, Sun S, Wu D, Ren H, Shen L, Hawkins TW, et al. Continuous-wave Raman amplification in silicon core fibers pumped in the telecom band. APL Photon (2021) 6:096105. doi:10.1063/5.0060108
44. Sohanpal R, Ren H, Shen L, Deakin C, Heidt AM, Hawkins TW, et al. All-fibre heterogeneously-integrated frequency comb generation using silicon core fibre. Nat Commun (2022) 13:3992. doi:10.1038/s41467-022-31637-1
45. Ren H, Aktas O, Franz Y, Runge AFJ, Hawkins T, Ballato J, et al. Tapered silicon core fibers with nano-spikes for optical coupling via spliced silica fibers. Opt Express (2017) 25:24157–63. doi:10.1364/OE.25.024157
46. Akihama Y, Hane K. Single and multiple optical switches that use freestanding silicon nanowire waveguide couplers. Light Sci Appl (2012) 1:e16. doi:10.1038/lsa.2012.16
47. Thubthimthong B, Sasaki T, Hane K. Asymmetrically and vertically coupled hybrid Si/GaN microring resonators for on-chip optical interconnects. IEEE Photon J (2015) 7:1–11. doi:10.1109/JPHOT.2015.2464721
48. Sørgård T, Song S, Vullum PE, Vullum PE, Kores C, Mølster KM, et al. Broadband infrared and THz transmitting silicon core optical fiber. Opt Mater Express (2020) 10:2491–9. doi:10.1364/OME.403591
49. He R, Day TD, Krishnamurthi M, Sparks JR, Sazio PJA, Gopalan V, et al. Silicon p-i-n junction fibers. Adv Mater (2013) 25:1461–7. doi:10.1002/adma.201203879
50. Martinsen FA, Smeltzer BK, Ballato J, Hawkins T, Jones M, Gibson UJ. Light trapping in horizontally aligned silicon microwire solar cells. Opt Express (2015) 23:A1463–A1471. doi:10.1364/OE.23.0A1463
51. Zhang T, Li K, Zhang J, Chen M, Wang Z, Ma S, et al. High-performance, flexible, and ultralong crystalline thermoelectric fibers. Nano Energy (2017) 41:35–42. doi:10.1016/j.nanoen.2017.09.019
Keywords: semiconductors, optical fibers, photonic crystal fibers, infrared, nonlinear optics
Citation: Ordu M (2023) The role of semiconductors in the future of optical fibers. Front. Phys. 11:1141795. doi: 10.3389/fphy.2023.1141795
Received: 10 January 2023; Accepted: 13 July 2023;
Published: 21 July 2023.
Edited by:
Jitendra Bahadur Maurya, National Institute of Technology Patna, IndiaReviewed by:
John Ballato, Clemson University, United StatesCopyright © 2023 Ordu. This is an open-access article distributed under the terms of the Creative Commons Attribution License (CC BY). The use, distribution or reproduction in other forums is permitted, provided the original author(s) and the copyright owner(s) are credited and that the original publication in this journal is cited, in accordance with accepted academic practice. No use, distribution or reproduction is permitted which does not comply with these terms.
*Correspondence: Mustafa Ordu, b3JkdUB1bmFtLmJpbGtlbnQuZWR1LnRy