- 1Key Laboratory of Advanced Micro/Nano Electronic Devices and Smart Systems of Zhejiang, College of Information Science and Electronic Engineering, Zhejiang University, Hangzhou, China
- 2Frontier Center of Brain Science and Brain-machine Integration, Cancer Center, Zhejiang University, Hangzhou, China
- 3Department of Orthopedic Surgery, 2nd Affiliated Hospital, School of Medicine, Zhejiang University, Hangzhou, Zhejiang, China
- 4Key Laboratory of Biomedical Engineering of Education Ministry, Qiushi Academy for Advanced Studies, Zhejiang University, Hangzhou, China
In the medical field, it is important to monitor and evaluate the blood supply status of organs and tissues during the clinical surgery. However, this largely depends on the surgeon’s experience and naked eye, which is easy to misjudge due to the interference of blood stains or other factors. A portable and flexible photoplethysmographic (PPG) detection probe is developed in this paper. And a new evaluation methodology of blood supply status is proposed based on this probe. Three typical indicators based on PPG is proposed to comprehensively evaluate the blood supply status, which are the blood oxygen saturation and its pulsation, differential characteristics of different lights, and time-frequency energy spectral characteristic. The probe and its evaluation methodology are verified using the brain of rats as a model.
1 Introduction
In clinical surgery, it is necessary to collect and monitor the blood supply status of organs and tissues. Depend on the blood supply status of organs and tissues, the judgement could be given about its status of normal, abnormal or irreversible inactivation, then the appropriate further surgery can be performed. For instance, the judgment about the blood supply status of parathyroid gland (PG) decides surgery for removing the diseased PG during parathyroidectomy. It is an essential skill for thyroid surgeons to decide to remove or preserve mung bean-sized parathyroid glands through evaluating blood supply status of the thyroid capsule. However, it largely depends on the surgeon’s experience due to bloodstains, environmental disturbances and other interferential factors, so the visual observation of the decision is subjective and has a large error. Thus, the rapid and accurate identification of the blood supply status of organs and tissues during the operation has great clinical value. Near-infrared (NIR) [1–3] photodetectors have been used in more and more medical and health fields. The near-infrared autofluorescence (NIRAF) has been used as a non-invasive, real-time, and automatic live detection method for PG. Under the excitation and irradiation of 785 nm laser, it was found that the fluorescence intensity of the PG in all patients was always greater than the fluorescence intensity of the thyroid and all other tissues of the neck, especially the fluorescence intensity of the PG was 2–11 times higher than that of the thyroid tissue [4]. A study in 2019 further found that NIRAF could accurately distinguish between healthy PG and diseased PG, thereby minimizing hypocalcaemia after thyroidectomy [5]. Another application of organ or tissue blood supply status detection is that for comatose patients, the monitoring of brain activity is required to judge whether the patient is alive or dead [6]. Cerebral blood flow [7, 8] or neural activity [9, 10] can often be used as an auxiliary test to diagnose brain death. Tests used to assess neural activity include EEG and evoked potentials. The main limitations of tests in assessing neural activity are interference from artifacts and the effects of metabolic changes and medications. Cerebral angiography, which is used to assess cerebral blood flow, is recognized as the gold standard method for diagnosing brain death, but its disadvantage is that it requires the patients to leave the intensive care unit and use contrast agents [11]. And Doppler (TCD) ultrasound examination is another method to evaluate blood flow without invasive surgery [7, 8]. However, above methods is not suitable for surgery due to its complex operation and large instrument size. A portable detector to carry out real-time monitoring of blood supply status during surgery is strongly desired by surgeons. In some minimally invasive surgeries, they need a flexible and small tube-like probe to insert into body and approach the organ or tissues to detect the blood supply status in situ.
The photoplethysmographic (PPG) and image PPG (iPPG) technology has been used to measure the changes of blood volume [12–14]. The recent publications show the possible application of iPPG for intraoperative monitoring of tissue perfusion during neurosurgery and abdominal surgery [15, 16]. However, in order to capture the changes in blood perfusion, the imaging sensor of the iPPG system has higher requirements on the frame rate, and at the same time, due to its non-contact, it is more affected by movement and ambient light. In addition, the change of PPG of micro organ capillary is too weak to measure it with iPPG [17]. Compared with non-contact iPPG, the contact-based PPG system has better optical accuracy, and the sensitivity of the blood volume change received by the photodiode is higher than that obtained after the secondary conversion of the iPPG imaging sensor. And PPG could be more simple, flexible and integrated.
This paper develop a portable and flexible micro-PPG detection probe for surgeon to monitor and evaluate the blood supply status of organs and tissues during the clinical surgery. A new evaluation methodology of blood supply status is proposed through verification using the brain of rats as a model. The acquisition of PPG signal was extracted and the organ activity was charactered with the sensitive eigenvalue from PPG. Three typical indicators based on PPG is proposed to comprehensively evaluate the blood supply status, which are the Blood oxygen saturation and its pulsation, differential characteristics of different lights, and time-frequency energy spectral characteristic. The micro-probe has been verified using the brain of rats as a model. This technology hopefully provides a powerful technical means for in-situ detection of micro-organ’s blood supply status in clinical operations.
2 Materials and methods
2.1 Design of portable flexible probe
The working principle of the PPG probe for identifying the blood supply status of organs is described as follows in brief: when a light beam of a certain wavelength illuminates surface of the tissue, the contraction and expansion of blood vessels affect the transmission or reflection of lights every time the pulse beats. When the lights pass through skin and then are reflected back to the photosensitive sensor, the lights will be attenuated to a certain degree. The absorption of lights by muscles, bones, veins and other connecting tissues is similar, but that by arteries is different. Because of the pulsation of blood in the arteries, the absorption of lights will change repeatedly according to the pulsation of the blood. In the cardiovascular system, capillaries are the sites of material exchange with tissues and organs. Since the movement of red blood cells in the capillary microcirculation is a heterogeneous fluid movement, red blood cells pass through the capillaries one by one or one after another, but when the red blood cells exchange substances in the capillary microcirculation and the microcirculation boundary, the interaction of the environment and the interaction between red blood cells, prone to accumulation and aggregation, etc., will affect the blood flow of capillaries. Under normal circumstances, the blood volume is relatively stable due to the non-pulsating nature of the rigid structure of capillaries. However, when organs or tissues are damaged, red blood cells may accumulate and aggregate, and capillary microcirculation is blocked, resulting in corresponding changes in blood volume and changes in blood flow parameters reflected by PPG. Also, the growing transmural pressure of the arteries during the systole compresses the connective tissues of the dermis in a local place, which results in the increasing density of capillaries and changes in blood volume [18–20]. Therefore, PPG can be used to evaluate the blood supply status.
The flexible structure of the probe mainly includes two parts: the first part is the flexible adhesive at the front end of the probe acquisition, mainly using polydimethylsiloxane (PDMS), which is a transparent flexible silicone, non-toxic insulating material with good light transmittance and good biocompatibility, which will not affect the accurate collection of biological probes. The second part is the flexible circuit board structure, which is convenient for the application in the detection of minimally invasive surgery in the body in the future, and can be flexibly bent and moved. Figure 1A is the schematic drawing of the proposed PPG probe in this work.
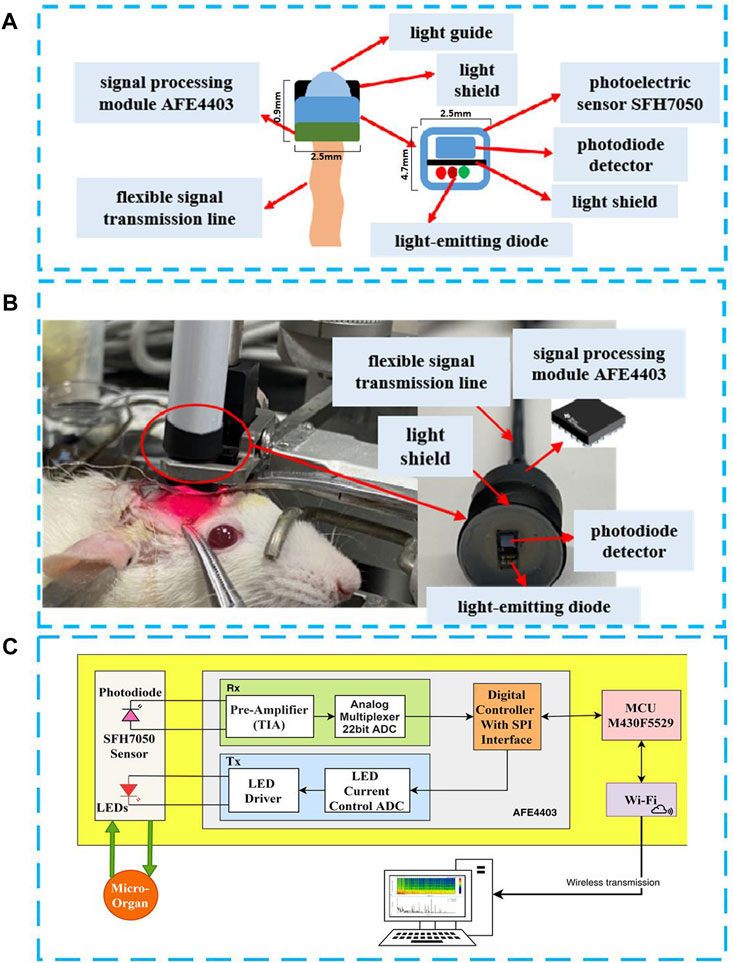
FIGURE 1. Portable and flexible PPG detection probe in this work. (A) Probe structure diagram; (B) Rat brain vivo experiment for verification; (C) Probe circuit system diagram system.
The photoelectric volume sensor SFH7050 contains three light-emitting diodes (LED) with wavelengths of 532 nm for the green light, 660 nm for the red light and 940 nm for the infrared light, respectively. The PPG probe has a lampshade-like structure as shown in Figure 1B at the front end, and the overall structure of the probe is fixed by a flexible circuit board, which can flexibly adapt to different surgical environments. The head of the PPG probe has lampshade-like structure, similar size with the detected organ or tissue, which can effectively prevent interferences from the ambient light, so as to improve the accuracy and reliability of the detection. In order to make the photoelectric sensor attached closely and comfortable with the organs, a transparent flexible PDMS film was coated inside the light shield. This structure can effectively prevent the interferences of tissue fluid during the detection of organ activity in the body without affecting the light path. The PDMS film was obtained by coating it on a silicon substrate with a thickness of 100 um by spinning coat PDMS solution at 3,000 rpm for 30 s. Prebaked it at 150°C for 5 min on hotplate, and hard baked at 250°C for 1 h on hotplate. In the end, PDMS film was peeled off the substrate and adhered on the light shield manually. In order to dynamically observe the PPG signal from the organ (rat brain was used for this work) for a long time, we added a casing for fixing. This tubular structure is also suitable to be used as a hand-held or standalone medical device during operation in an open surgical environment. Figure 1C is a photo of the developed flexible PPG probe used in the experiment, and Figure 1C is the functional block diagram of the PPG probe system. The PPG probe system is mainly consisted of a photoelectric sensor SFH7050, a large-area photodetector, an analog front-end AFE4403, which is composed of a low-noise receiver channel with an integrated analog-to-digital converter for PPG signal acquisition, and an ultra-low power microcontroller MSP430 for on-board signal processing. The processed signal is then sent to a PC with WiFi for displaying the results in real-time. The flexible PPG probe is powered by a 4.2 V, 240 mAh lithium battery. A fully charged battery can power the probe for about 16 h.
The mirco-probe mentioned in this paper is mainly used to determine the blood supply status of small organs/tissues, so as to assist surgeons in rapid intervention. For example, in the removal of parathyroid glands, for the judgment of necrotic parathyroid glands, these tiny organs/tissues are often small in size, usually about 6 mm long and 3–4 mm wide, and 1–2 mm anteroposteriorly [21], the size of the rat brain window in this experiment is only 7*6 mm. The size of the PPG sensor in this article is only 4.7 mm × 2.5 mm x 0.9 mm. Therefore, on the one hand, it can be adapted to small organs/tissues, and at the same time, the data collected at a single point is also sufficient for judging the blood supply status.
2.2 Experiments
A health Sprague-Dawley (SD) rat with a weight of 287 g was used for the proof of concept experiment. It was anesthetized with propofol (10 mg/ml solution, 0.012 ml/g) via intraperitoneal injection (IP) and set in the stereotaxic frame. A cranial window (size: 5 × 5 mm2) was produced into the skull of the rat by drilling, so that the PPG detection probe can be inserted into skull and attached Sulcus gyrus of brain. PPG signal recording was initiated once the cranial window was open, and it was followed with the excessive pentobarbital sodium (150 mg/kg) injection through IP for euthanasia, and observe the response of the brain activity by using the PPG detector.
It should be pointed out that, before euthanasia, the rat was also utilized for other studies to avoid the use of additional animals in our study. All surgical and experimental procedures followed the Guide for The Care and Use of Laboratory Animals (China Ministry of Health) and were approved by the Animal Care Committee of Zhejiang University, China. It should be noted that when SD is injected intraperitoneally or intravenously with sodium pentobarbital ≥100 mg/kg, it will experience euthanasia [22]. Therefore, during the death of SD, the blood circulation in the brain will gradually stop, and the brain gradually loses the arterial blood supply.
2.3 Characteristic indicators and evaluation methodology
In this paper, we provide three characteristic indicators to comprehensively evaluate the blood supply status of organs and tissues organs, which are related with organs and tissues vascular insufficiency or irreversible inactivation. These three indicators are the blood oxygen saturation and its pulsation, differential characteristics of PPG signals of different lights, and time-frequency energy spectral characteristics of PPG, which are explained as follows.
2.3.1 Blood oxygen saturation and pulsation
The blood oxygen saturation [23] represents the ratio of the volume of oxyhemoglobin (HbO2) bound to oxygen to the volume of all bound hemoglobin (Hb) in the arterial blood, i.e., the amount of oxygen in the blood, usually expressed as a percentage. Lambert Beer’s law is the basic law of the spectrophotometry [24], which describes the relationship between the strength of the absorption under a certain wavelength light of a substance, the concentration of the light-absorbing substance, and the thickness of the liquid layer. Derived from Lambert Beer’s law, the blood oxygen saturation is expressed as follows:
Here, A and B are constants, and can be obtained through experiments.
As we know, due to the presence of blood supply, PPG will regularly fluctuate with the diastole and contraction of the pulse. After the PPG is converted to the frequency domain, in addition to the DC component absorbed by bones, skin and other tissues, there is also a fundamental frequency near the DC component, which reflects the size of the pulse rate. The pulse rate is also an important physiological parameter of the respiratory cycle [25], which can characterize the activity of the organ. This has been utilized to measure the pulsation as expressed by Eq. 3:
2.3.2 Differential characteristics of PPG of different lights
According to the absorption coefficient curves [26, 27],
2.3.3 Time-frequency energy spectral characteristics of PPG
Due to the existence of blood supply, the PPG will beat regularly with the relaxation and contraction of the heart. Therefore, the PPG will have components in its fundamental frequency and harmonic components, and its energy is higher at this time [28]. With the occurrence of organ death, the blood circulation of the organ gradually becomes weak and eventually stops, the PPG no longer presents regular beat function, and the energy gradually decreases when the fundamental frequency and harmonic components are small. Therefore, the instantaneous energy change of the PPG can be observed through the short-time Fourier transform, so as to judge activity of the organ in real-time. Eq. 5 represents the Fourier transform of a signal, and Eq. 6 represents the power spectral density of the signal.
2.3.4 Evaluation methodology of blood supply status
In a conclusion, we provide three characteristic indicators to comprehensively evaluate the blood supply status, which are the blood oxygen saturation and its pulsation, differential characteristics of PPG signals of different lights, and time-frequency energy spectral characteristics of PPG [29, 30]. These three indicators can show us the organs and tissues vascular insufficiency or irreversible inactivation according with the whole process of euthanasia of rats, there are actually three stages:
1 Normal blood supply status
Because
2 Abnormal blood supply status but is reversible
If
3 Blood supply stops and is irreversible
At this time, the reflected light intensity of infrared light and red light gradually tends to the constant DC component, indicating that
3 Results and discussion
3.1 The difference between the intensity of red and infrared light
The real-time change of PPG of the reflected red light and infrared light is illustrated in Figure 2A, respectively. For the initial period of the experiment, arterial blood still circulated in the rat brain. For the active period, the initial reflected intensity of the red-light (660 nm) was much stronger than that of the infrared light (940 nm), and the difference became smaller with time over a period of 100 s. Both the red light and infrared light PPG waveforms oscillated regularly as the rat’s brain is still active as normal. The intensities of the reflected red light and infrared light from the rat brain were reversed after a 100 s and both of them varied with time irregularly. The rat was near dead at this stage. After 750 s, the reflected infrared light intensity became much stronger than that of the red light, and the difference became larger with the progress of time thereafter. The rat was completely dead after 17 min. Two features can also be noticed: 1) The regular oscillation of the PPG signal observed at the initial stage became weaker after 100 s and disappeared completely after 150 s, indicating the brain activity became weaker; 2) The PPG base line increased with time. This is because of the gradual blood coverage of the brain with time, which leds to the increased DC components of the PPG signal. Figure 2B shows the change of difference between the intensity of reflected red light and infrared light obtained by the PPG probe. Diff is equal to Red PPG minus IR PPG in Figure 2A. It can be seen that the decrease in brain activity results in an overall decrease in the difference between the red light and infrared light reflected, which reveals the changes of brain blood supply status.
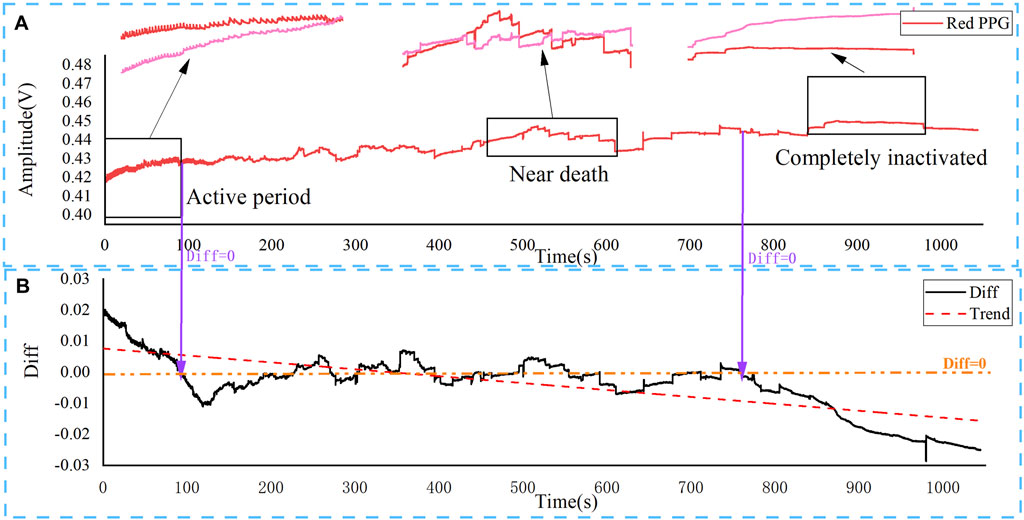
FIGURE 2. Changes of blood supply status in the brain of rats after overdose of anesthetic injection by PPG. The results show three typical periods, corresponding to different blood supply status. (A) Real-time change of red light (660 nm) and infrared light PPG (940 nm); (B) The difference (Diff) between red light and infrared light PPG.
3.2 Variation of SPO2 and pulserate
Figure 3A illustrates PPG of the red-light reflection as a function of time for different periods in details (The data of Figure 3 were extracted from the first 90 s data in Figure 2A for calculation, Figure 2A only shows the 30 s data). As it can be seen from Figure 3A, the pulsation intensity of the PPG decreased with the increase of time, and the according pulserate decreased gradually. According to Eq. 2, the change of blood oxygen saturation in the rat brain can be calculated for the 90 s period before the experiment with the result shown in Figure 3B.
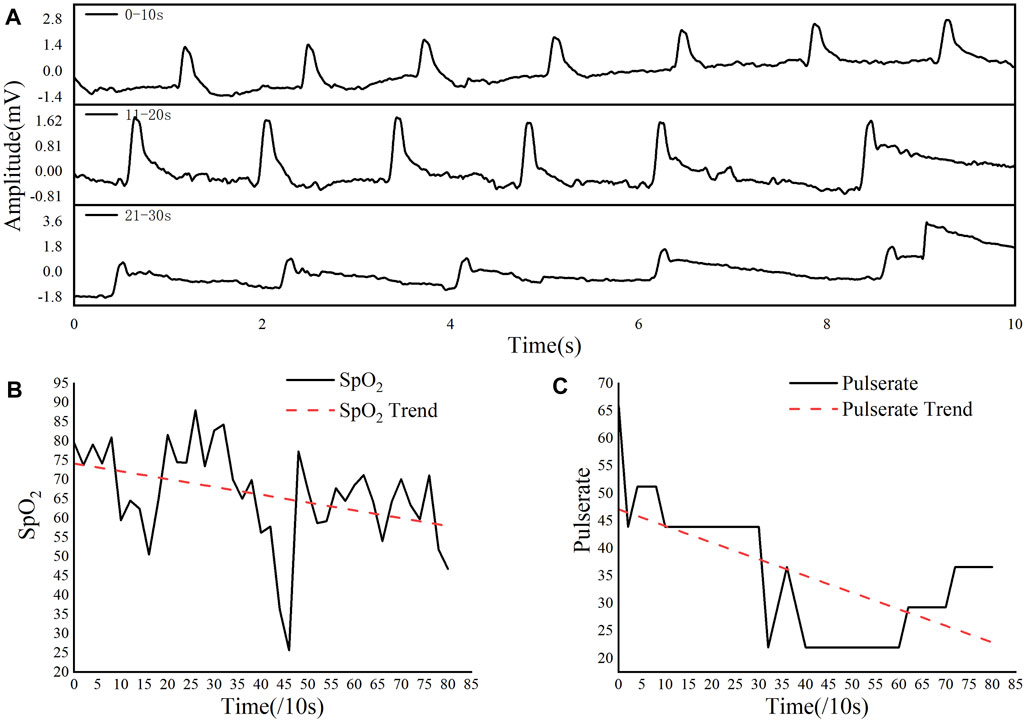
FIGURE 3. Red light PPG of the rat brain in the first 90 s. (A) The intensity of the red light reflected every 10 s. (B) The change of blood oxygen saturation gradually decrease. (C) Pulsation gradually decrease. Variation and analysis of Time-frequency energy spectrum characteristics.
As it is clear that during this period, the blood oxygen saturation fluctuated greatly, but overall it showed a downward trend with the increase of time. The pulserate of the PPG of the rat was calculated by using Eq. 3 for the 90 s before the experiment. As shown in Figure 3C, the pulsation showed a gradual decline trend during the inactivation process.
Further time-frequency analysis was conducted on PPG signals to obtain more information of the brain activity, which can be intuitive to grasp the changes in the light energy absorbed by the brain of the rats after injection of pentobarbital sodium.
A 0.01–50 Hz band-pass filtering and wavelet processing were first performed on the red light PPG signal, as to remove DC component (Such as power supply) and high-frequency interference (Such as changes in capillary density and venous blood volume, temperature changes, etc.). The result is shown in Figure 4A. It can be seen from the time domain that the PPG wave signal gradually becomes sparse during the euthanasia of rats injected with pentobarbital sodium. It indicates that the rat’s heart gradually stops beating during this process, resulting in insufficient blood supply to the cerebral arteries, unchangeable light absorption, and PPG waveform that gradually stops beating.
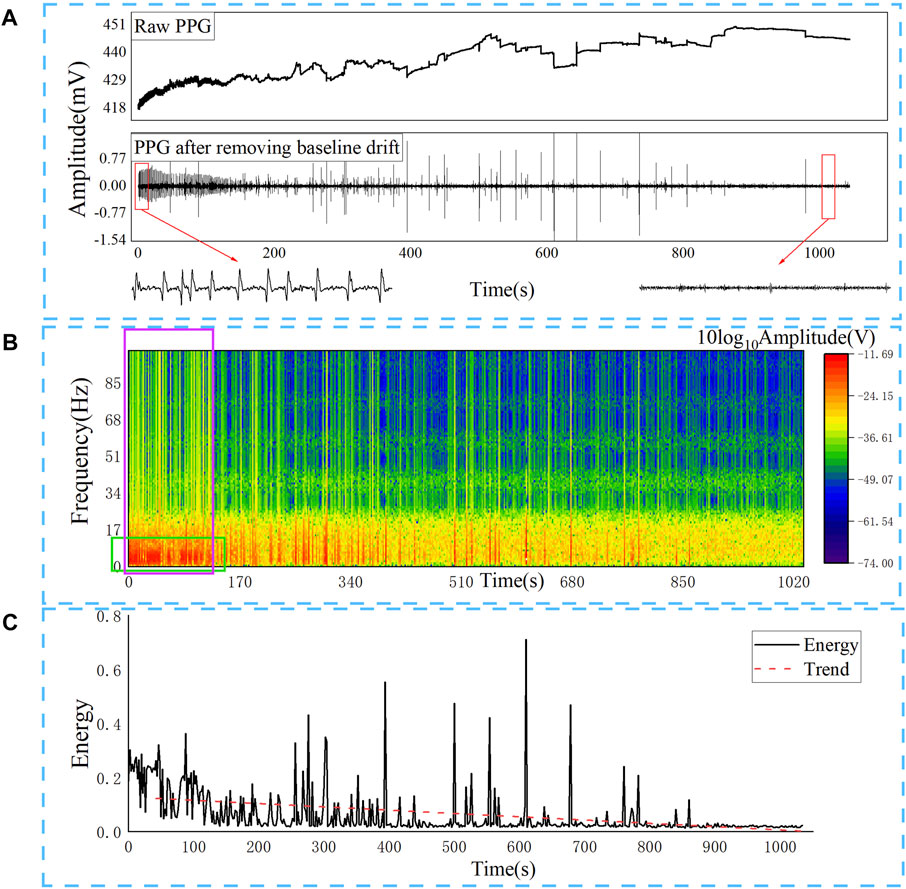
FIGURE 4. After removing the baseline drift of the original red light PPG, it can be seen that the pulsation gradually disappears, and low-frequency components in the frequency domain are gradually decreasing, and the total energy is continuously decreasing. (A) Red light PPG after band-pass filtering and wavelet processing; (B) Time-frequency change of red light PPG; (C) Energy change of red light PPG.
Then we converted to the frequency domain to analyse the energy changes of the PPG signal during the euthanasia of the rat. Use Eq. 5 to perform short-time Fourier transform on the recorded PPG waveform to observe the instantaneous energy change of the PPG wave as shown in Figure 4B, and the energy gradually decreases when the fundamental frequency and harmonic components are small. Figure 4C shows the sum of the energy of all frequency components of the PPG signal of the short-time Fourier transform, that is, the sum of all frequency energy at each moment expressed in Eq. 6. It can be seen that as the blood supply of the rat brain gradually decreases, the energy of the PPG gradually decreases. The energy change obtained according to the time-frequency change more intuitively reflects the overall decreasing trend of PPG energy in the process of brain inactivation in rats.
4 Conclusion
A portable and flexible PPG detection probe is developed in this paper. And a new evaluation methodology of blood supply status is proposed based on this probe. Three typical indicators are proposed to comprehensively evaluate the blood supply status, which are the blood oxygen saturation and its pulsation, differential characteristics of PPG signals of different lights, and time-frequency energy spectral characteristics of PPG signal. Among them, the time-frequency energy feature is the most important discriminant indicator, followed by the red light and the Infrared light. Differential features, the indicators with lower reference are blood oxygen saturation and pulsation frequency. The probe and its evaluation methodology are verified using the brain of rats as a model. In the future work, through a large number of experimental or clinical samples, machine learning can be used to build a model that can identify the three active states, which will further improve the efficiency of discrimination. In general, this technology provides a powerful technical means for in-situ detection of micro-organ’s blood supply status in clinical operations.
Data availability statement
The raw data supporting the conclusion of this article will be made available by the authors, without undue reservation.
Ethics statement
The animal study was reviewed and approved by the Guidelines for the Care and Use of Laboratory Animals of Zhejiang University (No. ZJU20200129).
Author contributions
All authors have read and agreed to the published version of the manuscript. JX, JP, SD, GF, JL, YW, and RY designed and performed the research. FZ and LZ performed experiments. JX and JP wrote the manuscript.
Funding
This work was funded by National Key R&D Program of China (No. 2018YFA0701400) and Zhejiang Province Key R&D programs (Nos 2021C05004, 2021C03108, and 2021C03062).
Acknowledgments
All experimental protocols and live animals were approved by the IACUC of Zhejiang University (No. ZJU20200129).
Conflict of interest
The authors declare that the research was conducted in the absence of any commercial or financial relationships that could be construed as a potential conflict of interest.
Publisher’s note
All claims expressed in this article are solely those of the authors and do not necessarily represent those of their affiliated organizations, or those of the publisher, the editors and the reviewers. Any product that may be evaluated in this article, or claim that may be made by its manufacturer, is not guaranteed or endorsed by the publisher.
References
1. Xu H, Li J, Leung BHK, Poon CCY, Ong BS, Zhang Y, et al. A high-sensitivity near-infrared phototransistor based on an organic bulk heterojunction. Nanoscale (2013) 5(23):11850–5. doi:10.1039/C3NR03989G
2. Xue Z, Li X, Li Y, Jiang M, Ren G, Liu H, et al. A 980 Nm laser-activated upconverted persistent probe for nir-to-nir rechargeable in vivo bioimaging. Nanoscale (2017) 9(21):7276–83. doi:10.1039/C6NR09716B
3. Yang Y, Aw J, Xing B. Nanostructures for nir light-controlled therapies. Nanoscale (2017) 9(11):3698–718. doi:10.1039/C6NR09177F
4. Paras C, Keller M, White L, Phay J, Mahadevan-Jansen A. Near-infrared autofluorescence for the detection of parathyroid glands. J Biomed Opt (2011) 16(6):067012. doi:10.1117/1.3583571
5. Solórzano CC, Thomas G, Baregamian N, Mahadevan-Jansen A. Detecting the near infrared autofluorescence of the human parathyroid. Ann Surg (2020) 272(6):973–85. doi:10.1097/SLA.0000000000003700
6. Beecher HK. After the definition of irreversible coma. N Engl J Med (1969) 281(3):1070–1. doi:10.1056/NEJM196911062811909
7. Flowers WM, Patel BR. Persistence of cerebral blood flow after brain death. South Med J (2000) 93(4):364–70. doi:10.1097/00007611-200004000-00003
8. Pistoia F, Johnson DW, Darby JM, Horton JA, Applegate LJ, Yonas H. The role of xenon ct measurements of cerebral blood flow in the clinical determination of brain death. AJNR Am J Neuroradiol (1991) 12(1):97–103.
9. Vicenzini E, Pro S, Randi F, Pulitano P, Spadetta G, Rocco M, et al. Transcranial Doppler for brain death after decompressive craniectomy: Persistence of cerebral blood flow with flat eeg. Intensive Care Med (2010) 36(12):2163–4. doi:10.1007/s00134-010-2008-0
10. Welschehold S, Boor S, Reuland K, Thömke F, Kerz T, Reuland A, et al. Technical aids in the diagnosis of brain death. Dtsch Arztebl Int (2012) 109(39):624–30. doi:10.3238/arztebl.2012.0624
11. Kramer AH. Ancillary testing in brain death. Semin Neurol (2015) 35(2):125–38. doi:10.1055/s-0035-1547541
12. Pelaez EA, Villegas ER. Led power reduction trade-offs for ambulatory pulse oximetry. Annu Int Conf IEEE Eng Med Biol Soc (2007) 2007:2296–9. doi:10.1109/IEMBS.2007.4352784
13. Reisner A, Shaltis PA, McCombie D, Asada HH, Warner S, Warner MA. Utility of the photoplethysmogram in circulatory monitoring. Anesthesiology (2008) 108(5):950–8. doi:10.1097/ALN.0b013e31816c89e1
14. Ruiz-Rodríguez JC, Ruiz-Sanmartín A, Ribas V, Caballero J, García-Roche A, Riera J, et al. Innovative continuous non-invasive cuffless blood pressure monitoring based on photoplethysmography technology. Intensive Care Med (2013) 39(9):1618–25. doi:10.1007/s00134-013-2964-2
15. Kamshilin AA, Zaytsev VV, Lodygin AV, Kashchenko VA. Imaging photoplethysmography as an easy-to-use tool for monitoring changes in tissue blood perfusion during abdominal surgery. Sci Rep (2022) 12(1):1143. doi:10.1038/s41598-022-05080-7
16. Mamontov OV, Shcherbinin AV, Romashko RV, Kamshilin AA. Intraoperative imaging of cortical blood flow by camera-based photoplethysmography at green light. Appl Sci (2020) 10(18):6192. doi:10.3390/app10186192
17. Unakafov AM, Möller S, Kagan I, Gail A, Treue S, Wolf F. Using imaging photoplethysmography for heart rate estimation in non-human primates. PLoS One (2018) 13(1):e0202581. doi:10.1371/journal.pone.0202581
18. Glazier JB, Hughes JM, Maloney JE, West JB. Measurements of capillary dimensions and blood volume in rapidly frozen Lungs. J Appl Physiol (1969) 26(1):65–76. Epub 1969/01/01. doi:10.1152/jappl.1969.26.1.65
19. Kamshilin AA, Nippolainen E, Sidorov IS, Vasilev PV, Erofeev NP, Podolian NP, et al. A new Look at the essence of the imaging photoplethysmography. Sci Rep (2015) 5(1):10494. doi:10.1038/srep10494
20. Lipowsky HH. Microvascular rheology and hemodynamics. Microcirculation, 12(1994):5–15. doi:10.1080/10739680590894966
21. Driscoll P. Gray's anatomy, 39th edition. Emerg Med J (2006) 23(6):492. doi:10.1136/emj.2005.027847
22. Underwood W, Anthony R. Avma Guidelines for the euthanasia of animals: 2020 edition. Retrieved on March (2020) 2020(30):1–20.
23. Mamontov OV, Sokolov AY, Volynsky MA, Osipchuk AV, Zaytsev VV, Romashko RV, et al. Animal model of assessing cerebrovascular functional reserve by imaging photoplethysmography. Sci Rep (2020) 10(1):19008–10. doi:10.1038/s41598-020-75824-w
24. Beer B. Bestimmung der Absorption des rothen Lichts in farbigen Flüssigkeiten. Ann Phys Chem (1852) 162:78–88. doi:10.1002/andp.18521620505
25. Kim H, Kim J-Y, Im C-H. Fast and robust real-time estimation of respiratory rate from photoplethysmography. Sensors (2016) 16(9):1494. doi:10.3390/s16091494
26. Azmal GM, Al-Jumaily A, Al-Jaafreh A. Continuous measurement of oxygen saturation level using photoplethysmography signal. IEEE Int Conf Biomed Pharm Eng (2006) 504–7.
27. Kanders K, Grabovskis A, Marcinkevics Z, Aivars JI. Assessment of conduit artery vasomotion using photoplethysmography. SPIE Proc Biophotonics Riga (2013) 9032(1):121–9. doi:10.1117/12.2044705
28. Han C-H, Kim E, Im C-H. Development of a brain-computer interface toggle switch with low false-positive rate using respiration-modulated photoplethysmography. Sensors (2020) 20(2):348. doi:10.3390/s20020348
29. Sassaroli A, Pierro M, Bergethon PR, Fantini S. Low-frequency spontaneous oscillations of cerebral hemodynamics investigated with near-infrared spectroscopy: A review. IEEE J Select Top Quan Electron. (2012) 18(4):1478–92. doi:10.1109/jstqe.2012.2183581
Keywords: optoelectronic sensor, blood supply probe, photoplethysmographic, parathyroidectomy, biomedical photonic device, flexible applications
Citation: Xia J, Pan J, Yan R, Zhang F, Zhang L, Feng G, Luo J, Dong S and Wang Y (2022) Portable flexible probe for detecting blood supply status in clinical surgery. Front. Phys. 10:972916. doi: 10.3389/fphy.2022.972916
Received: 19 June 2022; Accepted: 29 August 2022;
Published: 20 September 2022.
Edited by:
Xunbin Wei, Peking University, ChinaReviewed by:
Xuan Weipeng, Hangzhou Dianzi University, ChinaRichard Yongqing Fu, Northumbria University, United Kingdom
Fanli Meng, Northeastern University, China
Copyright © 2022 Xia, Pan, Yan, Zhang, Zhang, Feng, Luo, Dong and Wang. This is an open-access article distributed under the terms of the Creative Commons Attribution License (CC BY). The use, distribution or reproduction in other forums is permitted, provided the original author(s) and the copyright owner(s) are credited and that the original publication in this journal is cited, in accordance with accepted academic practice. No use, distribution or reproduction is permitted which does not comply with these terms.
*Correspondence: Ruijian Yan, eWFucnVpamlhbkB6anUuZWR1LmNu