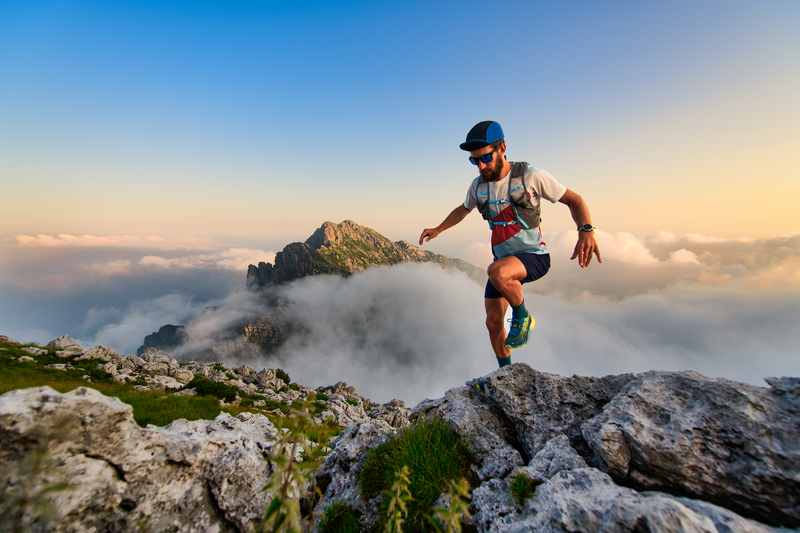
95% of researchers rate our articles as excellent or good
Learn more about the work of our research integrity team to safeguard the quality of each article we publish.
Find out more
ORIGINAL RESEARCH article
Front. Phys. , 07 September 2022
Sec. Atomic and Molecular Physics
Volume 10 - 2022 | https://doi.org/10.3389/fphy.2022.971059
This article is part of the Research Topic Quantum Precision Measurement and Cold Atom Physics View all 17 articles
The Cold Atom Physics Rack (CAPR) of Chinese space station will be launched at the end of 2022. The important goal of CAPR is to achieve BEC at 100 pk. In order to obtain ultracold atoms in microgravity of space station, we propose a two-stage cooling scheme using all-optical trap with different waist beams. The cold atom cloud obtained by this scheme is composed of condensate and thermal atoms around condensate. The design of our two-stage cooling scheme will effectively reduce the temperature of the thermal atom cloud and the effective temperature generated by the interaction energy of the condensate. The atomic temperature of 5 nk is obtained from the ground test experiment, and the corresponding temperature under the microgravity condition of the space station is theoretically predicted to be less than 100 pk. Taking the advantages of ultracold temperature and long-time detection, many scientific experiments will be arranged. In this paper, the ground test experiments based on ground principle prototype and pre-prototype for CAPR are also introduced.
Ultra-low temperature has always been the tireless pursuit of scientists in the field of atomic and molecular physics since the beginning of the last century. The lower the atomic temperature, the more impetus and representative significance for the verification of many physical theories [1–3]. The sensitivity and accuracy of atomic interferometers, gravimeters, and gyroscopes can also achieve an order of magnitude leap in cooler degenerate gases [4–7]. In 1908, Kamerlingh Onnes produced liquid Helium, of which the temperature is below 4.2K [8]. In 1911, he found superconductivity in mercury at 4.1K [9]. The development of laser cooling overwrote the record to micro Kelvin [10, 11]. The success of Bose-Einstein Condensation (BEC) paves the way for lowering temperatures in nano-Kelvin [12–14], and adiabatic release of degenerate gases pushes the limit to sub-nanometer Kelvin [15]. Further atomic deep cooling is limited by gravity. Continuously reducing the depth of the potential well can theoretically obtain lower temperature trapped atoms, but the shallower potential well will not be able to resist the effect of the atom’s own gravity, causing the atoms to leak out of the well, resulting in a substantial loss of the number of atoms and a decrease in the collision rate between atoms. Thus the evaporative cooling process tends to be ineffective.
Thanks to microgravity, degenerate atomic gases can be cooled to lower temperature than ever before. Germany, France, the United States and China have all carried out cold atom experiments under microgravity conditions. In the QUANTUS project in Germany, Rasel’s research team used an atomic chip to realize BEC with atomic kinetic energy of 9 nk in the Bremen drop tower experiment in 2010 [16]. In 2013, they used delta-kick cooling (DKC) method to reduce the atomic temperature to about 1 nk and conducted interference experiments [17]. And in the German MAIUS-1 mission in 2018, they achieved a rubidium BEC of about 1 nk for a longer experimental time under the 6-min microgravity conditions created by the sounding rocket [18]. In France, Bouyer’s research team created a microgravity environment by flying the parabolic plane in 2011, using a velocity-selective Raman light pulse carrying two counter-propagating laser fields and cooled the atoms to a temperature of 300 nK in the longitudinal velocity distribution and obtained an improvement in the sensitivity of the interferometer at 0 g [19]. The JPL team of NASA in the United States installed the Cold Atom Laboratory (CAL) experimental module on the International Space Station (ISS) in 2018, using the atom chip to conduct the deep cooling experiment of degenerate gas of rubidium and potassium [20–23]. One of the aim of the CAL is to break the limit of atomic temperature on the ground and realize ultra-low temperature of pK or even fK. Limited by the experimental system and mechanical vibration disturbances in the space station, the current cold atom temperature is on the order of 10 nK. China is scheduled to launch the space station experimental module by the end of 2022, which will be equipped with a Cold Atom Physics Rack (CAPR). The atomic deep cooling adopts the two-stage cooling (TSC) scheme proposed by Peking University [24–27]. In the first stage, atoms undergo the runaway evaporation cooling process in an optical trap formed by two crossed laser beams with narrow beam diameter and high power. In the second stage, low temperatures atoms are loaded into the other optical trap formed by two crossed laser beams with wide waist and weak power. The TSC scheme has been validated to effectively reduce the thermal atomic cloud temperature. The atomic temperature of 5 nk is obtained from the ground verification test, and it is theoretically predicted that the corresponding atomic temperature under the microgravity condition of the space station is less than 100 pk. The project for CAPR includes three stages: ground principle prototype, pre-prototype, formal prototype. We have achieved ground principle prototype, pre-prototype, and completed the test experiments based on pre-prototype.
This article firstly emphasizes the necessity of conducting ultra-cold atomic physics experiments in the microgravity environment. And then introduces the structure, modules, key technologies of ground principle prototype and pre-prototype, as well as ground test experiments for the CAPR on Chinese Space Station. The atomic deep cooling scheme of TSC is introduced through physical principles, theoretical simulation results, ground verification experiments, and the reduction of thermodynamic temperature. Finally, it is concluded that the space station ultra-cold atomic experiment using the two-stage cooling scheme is of great significance to the advancement of atomic and molecular physics research under the conditions of ultra-low temperature and long-term detection.
Currently, the main factor limiting further temperature reductions is the acceleration of earth’s gravity. For DKC cooling and pulsed optical lattice momentum filter, the atoms cannot be trapped by the external potential field during the cooling process, otherwise the external potential field will heat the atomic gas. But the existence of gravitational acceleration will make the atoms leave off the optical trap in the direction of gravity. Therefore, the cooling of the atomic gas by these cooling technologies on the ground only reduces the momentum width of the atomic gas in some dimensions, but does not reduce the average kinetic energy of the atomic gas. Hence, the realization of microgravity conditions is crucial for the reduction of atomic temperature [28], and the long-term gravity-free gradient environment of the space station is very beneficial to the implementation of precise physical experiments.
Chinese space station has been launched in 2021, and the science module II is due to launch in late 2022 with the Cold Atom Physics Rack. The goal of CAPR is to achieve the quantum degenerate gas of picokelvin ultra-low temperature and conduct a series of scientific experiments for quantum simulations and precise verification of physical laws in microgravity conditions on the space station. The CAPR is based on the constraints of external mechanical, electrical, thermal, information, measurement and control resources. Due to the particularity of space station and rocket carrying, CAPR must be small in volume (1.5 cubic meters), light in weight (500 kg) and low in power consumption (1,000 W). There are five units in the miniaturized and highly integrated CAPR system: 1) Physics module; 2) Laser and optics module; 3) Electronics module; 4) Remote control module; 5) Rack support module. As shown in Figure 1.
FIGURE 1. The structure of Cold Atom Physics Rack (CAPR) on the Chinese space station. There are five units in the miniaturized and highly integrated CAPR system: (1) Physics module; (2) Laser and optics module; (3) Electronics module; (4) Remote control module; (5) Rack support module.
Our ground-based verification system for CAPR is a Rb quantum gas system for physical experiments in space microgravity. In order to achieve the goal of miniaturization and low power consumption in the space station, there are several key techniques in the process of preparing Bose-Einstein condensation. High frequency laser phase lock technology is used to produce a tunable frequency-stabilized laser to cool atoms. The tuning range is ±12 GHz (dynamic range 500 MHz). Optical phase lock loop (OPLL) avoids the larger volume and high power consumption of the traditional AOM complex optical path. The complex programmable logic device (CPLD) is used to replace the LabVIEW board card (not available in space) to realize the control of system time sequence. It is just a 10 cm × 10 cm circuit board programmed through a computer, which realize the switch control of shutter driver, AOM driver, magnetic coil driver, laser power variation curve of optical dipole trap, camera trigger and so on. And high current magnetic trap control technology enables a high-current coil to have a current of 500 A and a magnetic field of 600 Gs.
Ground test experiments was performed first from the ground principle prototype for CAPR (see Figure 2), which demonstrated that the principle of two-stage cooling is correct and some experimental techniques are available. The pre-prototype for CAPR (see Figure 3) adopts an all-optical scheme, which is almost identical to the launched one in the future. It needs to use the specified components available in aerospace. The following verification experiments were implemented on the pre-prototype. It tested that the techniques and approaches for two-stage cooling and the subsequent experiments are available for the CAPR in microgravity.
FIGURE 2. Ground principle prototype for CAPR. The purpose of the ground principle prototype is to verify that some technical solutions adopted on CAPR are available.
FIGURE 3. Pre-prototype for CAPR (2D-MOT and 3D-MOT parts). The size is almost same of the formal one. All laser transmissions on the pre-prototype use optical fiber, which is different from the space optical path transmission on the ground-based prototype. Various tests are also carried out on the pre-prototype to make reference and backup for the real launched one.
Compared with the Cold Atom Laboratory of the US International Space Station, which uses the atom chip and magnetic trap plus microwave cooling, the CAPR on Chinese space station adopts all-optical trap two-stage cooling scheme. Theoretical and ground-based experiments show that our scheme can obtain lower temperature. In addition, the CAPR system is developed to support the research of space ultracold atom physics and carry out quantum simulation experiments. Four fundamental physics experiments based on quantum gas will be implemented on Chinese space station in first 3 years: 1) Quantum Magnetism [29]; [30]; [31]; 2) Exotic material [32]; [33]; 3) Acoustic black hole [34]; 4) Efimov effect [35].
When directly applying evaporation cooling method [36–38] to break through to a lower temperature (pico-Kelvin), a major difficulty is that when the groups of atoms are cooled to a temperature of the order of nK, its internal collision rate per unit time will be greatly reduced. Such a low collision rate is not sufficient to maintain an effective evaporative cooling process. And the longer the cooling process continues, the more atomic number loss is caused by inelastic collisions. Therefore, how to introduce a more efficient mechanism so that the cooling can continue when the atomic gases enter the temperature of the order of nK is a key question. In order to ensure that the temperature of pK magnitude can be reached within an acceptable time, our experimental group proposed the two-stage cross beam cooling method in 2013 [24], and applied it to the ultra-cold atomic physics experiments on Chinese space station launched at the end of 2022.
Here, we deduce the feasibility of the two-stage cooling scheme from physical principles. Without regard to gravity, the potential of the crossed optical dipole trap can be expressed as
Where
Where
Taking the x-direction as an example, the potential energy at the center of the simple harmonic trap can be written as
Where m is the mass of the atom,
Similarly, the harmonic trap frequencies in the y and z directions are
The critical temperature of atoms is directly related to the potential trap frequency, which can be obtained from the following equation
Where
The relationship between the ground state atomic ratio and temperature is
From Eq. 6, we can get that the atomic temperature is proportional to the root square of the laser power and inversely proportional to the square of the beam waist size. The reduction of ODT laser power
The experimental process of the two-stage cooling scheme is shown in Figure 4. First, the atoms of micro-Kelvin temperature are loaded into the tightly confined optical dipole trap formed by two thin-waisted crossed beams. Atoms undergo the runaway evaporation cooling process with decreasing laser intensity. In the first stage the atoms will be cooled to tens of nanokelvin temperature in about 5 s. Next, overlap the loose optical dipole trap consisting of a pair of thick-waisted intersecting beams. With the continuous weakening of the laser intensity of the thin-waisted optical trap and the continuous increase of the intensity of the thick-waisted optical trap, the atoms undergo adiabatic transfer in these two optical traps. Afterwards, continuously reducing the intensity of the thick-waisted beams, the atoms are diffusively cooled in the loose trap to the picokelvin temperature. A deeply cooled quantum degenerate gas is thus formed.
FIGURE 4. Schematic diagram of the experimental process of the two-stage cooling (TSC) scheme. (A) Atoms (red dots) trapped in the tightly confined optical dipole trap formed by two thin-waisted crossed beams for evaporative cooling. (B) Adiabatic transfer of atoms from the thin-waisted optical trap (blue beams) to the thick-waisted optical trap (yellow beams). (C) Atoms are diffusively cooled in the loose optical trap while decreasing the intensity of the thick-waisted beams. After decompression cooling process, the temperature of atoms below 1e-10K is expected to be achieved.
In order to provide reference and verification for the space station experiment, we did a series of tests on the ground. Figure 5 shows the illustrations of experimental parameter settings for the TSC scheme in the ground verification experiments towards Chinese space station.
FIGURE 5. Experimental parameter settings for the two-stage cooling scheme in the ground verification experiments towards Chinese space station. Illustrations (A,B) show the variation of laser power with time for the thin-waisted ODT and the thick-waisted ODT, respectively, during the implementation of the TSC scheme. (C) The gradient of the lifting magnetic field (compensating for gravitational effects) changes as the experiment progresses.
In the experiment, we used a pair of 1,064 nm far-detuned lasers with the waist radius of 30
Where
FIGURE 6. (A) First stage cooling with tight crossed beam trap. The temperature of BEC reached to 74 nK; (B) Second stage cooling with loose crossed beam trap. The temperature of BEC reached to 5 nK. Corresponding 100 pK in the microgravity.
FIGURE 7. The schematic diagram of the numerical simulation results of TSC scheme. It is theoretically confirmed that the atomic temperature can be reduced to 100 pK when the TSC process is proceeded around 7.3 s in microgravity on the space station [39]. The insert shows the image of BEC after deep cooling in the ground verification experiments. The equivalent temperature of BEC is T = 5 pK and the number of 87Rb atoms is n =
Our group has previously performed multiple numerical simulations using the direct simulation Monte Carlo (DSMC) method [25, 39]. The simulation results theoretically confirm that the atomic temperature can be reduced to 100 pK when the TSC process is carried out for about 7.3 s in the microgravity on the space station [39]. This includes 5 s of evaporative cooling in the first stage and about 2.3 s of adiabatic diffusion cooling in the second stage. Simultaneously, the numerical simulation results show that if the CAPR on the Chinese space station are isolated from vibration, the temperature of quantum gas can be below 10 pK around 15 s [39]. The schematic diagram of simulation results of TSC scheme is shown in Figure 7.
Mankind is making continuous efforts in the pursuit of low temperature. From the preparation of liquid helium to cold atomic gases, the level of low temperature continued to improve from K (liquid helium) to
The original contributions presented in the study are included in the article/Supplementary Material, further inquiries can be directed to the corresponding author.
HL, JY, XY, BW, YX, LL, AL, MH, SJ, WX, BW, DC, TL, XH, LL, XZ, WC, and XC participated in the construction of prototypes. XC, WX, and WC directed the research. HL and BW performed the experiments and measured experimental data. LL, AL, MH, and SJ assisted the experiments. JY and XY contributed to the analysis of data. YX contributed to the numerical simulation. HL wrote the manuscript. XC revised the manuscript.
This work is supported by the National Natural Science Foundation of China (Grants Nos. 11920101004, 11334001, 61727819, 61475007) and the National Key Research and Development Program of China (Grant Nos. 2021YFA1400900, 2021YFA0718300).
Thanks to all the project staff for the construction and promotion of the Cold Atomic Physics Rack on the Chinese Space Station. Thanks to Tian Luan and Bo Fan for their previous simulation studies on the two-stage cooling scheme.
The authors declare that the research was conducted in the absence of any commercial or financial relationships that could be construed as a potential conflict of interest.
All claims expressed in this article are solely those of the authors and do not necessarily represent those of their affiliated organizations, or those of the publisher, the editors and the reviewers. Any product that may be evaluated in this article, or claim that may be made by its manufacturer, is not guaranteed or endorsed by the publisher.
1. Bergmann P, Sabbata V. Advances in the interplay between quantum and gravity physics, 103-33. Boston: Kluwer (2002). p. 317–39.
2. Bloch I, Dalibard J, Nascimbène S. Quantum simulations with ultracold quantum gases. Nat Phys (2012) 8(4):267–76. doi:10.1038/nphys2259
3. Rosi G, Sorrentino F, Cacciapuoti L, Prevedelli M, Tino GM. Precision measurement of the Newtonian gravitational constant using cold atoms. Nature (2014) 510:518–21. doi:10.1038/nature13433
4. Bouyer P, Kasevich MA. Heisenberg-limited spectroscopy with degenerate Bose-Einstein gases. Phys Rev A (Coll Park) (1997) 56(2):R1083–6. doi:10.1103/physreva.56.r1083
5. Dowling JP. Correlated input-port, matter-wave interferometer: Quantum-noise limits to the atom-laser gyroscope. Phys Rev A (Coll Park) (1998) 57(6):4736–46. doi:10.1103/physreva.57.4736
6. Gupta S, Dieckmann K, Hadzibabic Z, Pritchard DE. Contrast interferometry using bose-einstein condensates to measureh/mandαand. Phys Rev Lett (2002) 89(14):140401. doi:10.1103/physrevlett.89.140401
7. Eckert K, Hyllus P, Bruß D, Poulsen UV, Lewenstein M, Jentsch C, et al. Differential atom interferometry beyond the standard quantum limit. Phys Rev A (Coll Park) (2006) 73(1):013814. doi:10.1103/physreva.73.013814
8. Onnes HK. The resistance of pure mercury at helium temperatures. Comm Phys Lab Univ Leiden (1911) 12.
9. Onnes HK. The superconductivity of mercury. Leiden, Netherlands: Comm. Phys. Lab. Univ. Leiden (1911). p. 122–4.
10. Chu S, Hollberg L, Bjorkholm JE, Cable A, Ashkin A. Three-dimensional viscous confinement and cooling of atoms by resonance radiation pressure. Phys Rev Lett (1985) 55:48–51. doi:10.1103/physrevlett.55.48
11. Dalibard J, Cohen-Tannoudji C. Laser cooling below the Doppler limit by polarization gradients: Simple theoretical models. J Opt Soc Am B (1989) 6(11):2023–45. doi:10.1364/josab.6.002023
12. Anderson MH, Ensher JR, Matthews MR, Wieman CE, Cornell EA. Observation of bose-einstein condensation in a dilute atomic vapor. Science (1995) 269(5221):198–201. doi:10.1126/science.269.5221.198
13. Davis KB, Mewes MO, Andrews MR, van Druten NJ, Durfee DS, Kurn DM, et al. Bose-einstein condensation in a gas of sodium atoms. Phys Rev Lett (1995) 75(22):3969–73. doi:10.1103/physrevlett.75.3969
14. Bradley CC, Sackett CA, Tollett JJ, Hulet RG. Evidence of bose-einstein condensation in an atomic gas with attractive interactions. Phys Rev Lett (1995) 75(9):1687–90. doi:10.1103/physrevlett.75.1687
15. Leanhardt AE, Pasquini TA, Saba M, Schirotzek A, Shin Y, Kielpinski D, et al. Cooling Bose-Einstein condensates below 500 picokelvin. Science (2003) 301(5639):1513–5. doi:10.1126/science.1088827
16. Van Zoest T, Gaaloul N, Singh Y, Ahlers H, Herr W, Seidel ST, et al. Bose-Einstein condensation in microgravity. Science (2010) 328(5985):1540–3. doi:10.1126/science.1189164
17. Müntinga H, Ahlers H, Krutzik M, Wenzlawski A, Arnold S, Becker D, et al. Interferometry with Bose-Einstein condensates in microgravity. Phys Rev Lett (2013) 110:093602. doi:10.1103/physrevlett.110.093602
18. Becker D, Lachmann MD, Seidel ST, Ahlers H, Dinkelaker AN, Grosse J, et al. Space-borne Bose-Einstein condensation for precision interferometry. Nature (2018) 562:391–5. doi:10.1038/s41586-018-0605-1
19. Geiger R, Ménoret V, Stern G, Zahzam N, Cheinet P, Battelier B, et al. Detecting inertial effects with airborne matter-wave interferometry. Nat Commun (2011) 2(1):474. doi:10.1038/ncomms1479
20. Sackett CA, Lam TC, Stickney JC, Burke JH. Extreme adiabatic expansion in micro-gravity: Modeling for the cold atomic laboratory. Microgravity Sci Technol (2018) 30, 155–63. doi:10.1007/s12217-017-9584-3
21. Gibney E. Universe’s coolest lab set to open up quantum world. Nature (2018) 557:151–2. doi:10.1038/d41586-018-05111-2
22. Elliott ER, Krutzik MC, Williams JR, Thompson RJ, Aveline DC. NASA’s cold atom lab (CAL): System development and ground test status. npj Microgravity (2018) 4(1):16. doi:10.1038/s41526-018-0049-9
23. Aveline DC, Williams JR, Elliott ER, Dutenhoffer C, Kellogg JR, Kohel JM, et al. Observation of bose–einstein condensates in an earth-orbiting research lab. Nature (2020) 582(7811):193–7. doi:10.1038/s41586-020-2346-1
24. Wang L, Zhang P, Chen X, Ma Z. Generating a picokelvin ultracold atomic ensemble in microgravity. J Phys B: Mol Opt Phys (2013) 46:195302. doi:10.1088/0953-4075/46/19/195302
25. Yao H, Luan T, Li C, Zhang Y, Ma Z, Chen X. Comparison of different techniques in optical trap for generating picokelvin 3D atom cloud in microgravity. Opt Commun (2016) 359:123–8. doi:10.1016/j.optcom.2015.09.065
26. Luan T, Li Y, Zhang X, Chen X. Realization of two-stage crossed beam cooling and the comparison with Delta-kick cooling in experiment. Rev Sci Instrum (2018) 89:123110. doi:10.1063/1.5046815
27. Chen X, Fan B. The emergence of picokelvin physics. Rep Prog Phys (2020) 83:076401. doi:10.1088/1361-6633/ab8ab6
28. Deppner C, Herr W, Cornelius M, Stromberger P, Sternke T, Grzeschik C, et al. Collective-mode enhanced matter-wave optics. Phys Rev Lett (2021) 127(10):100401. doi:10.1103/physrevlett.127.100401
29. LeBlanc L. Physics.APS. Physics (2018) 11:131. Available at: https://physics.aps.org/articles/v11/131.
30. Stamper-Kurn D, Ueda M. Spinor Bose gases: Symmetries, magnetism, and quantum dynamics. Rev Mod Phys (2013) 85(3):1191–244. doi:10.1103/revmodphys.85.1191
31. Wang W, Zhu H, Wang L, Bai W, Chen X, Liu W. Precise measurements of quantum magnetism of cold atoms in ground and microgravity environment. Sci Sin -Phys Mech Astron (2021) 51:074207. doi:10.1360/sspma-2020-0475
32. Banerjee A, Bridges CA, Yan JQ, Aczel AA, Li L, Stone MB, et al. Proximate Kitaev quantum spin liquid behaviour in a honeycomb magnet. Nat Mater (2016) 15(7):733–40. doi:10.1038/nmat4604
33. Jin S, Zhang W, Guo X, Chen X, Zhou X, Li X. Evidence of potts-nematic superfluidity in a hexagonal sp2 optical lattice. Phys Rev Lett (2021) 126:035301. doi:10.1103/physrevlett.126.035301
34. Muñoz de Nova JR, Golubkov K, Kolobov VI, Steinhauer J. Observation of thermal Hawking radiation and its temperature in an analogue black hole. Nature (2019) 569(7758):688–91. doi:10.1038/s41586-019-1241-0
35. Helfrich K, Hammer H. Resonant atom-dimer relaxation in ultracold atoms. Europhys Lett (2009) 86:53003. doi:10.1209/0295-5075/86/53003
36. Ketterle W, Van Druten N. Evaporative cooling of trapped atoms. Adv Mol Opt Phys (1996) 37:181–236.
37. Barrett MD, Sauer JA, Chapman MS. All-optical formation of an atomic bose-einstein condensate. Phys Rev Lett (2001) 87(1):010404. doi:10.1103/physrevlett.87.010404
38. Condon G, Rabault M, Barrett B, Chichet L, Arguel R, Eneriz-Imaz H, et al. All-optical bose-einstein condensates in microgravity. Phys Rev Lett (2019) 123(24):240402. doi:10.1103/physrevlett.123.240402
Keywords: space station, cold atom physics rack, two-stage cooling, picokelvin, microgravity
Citation: Li H, Yu J, Yuan X, Wu B, Xie Y, Li L, Liang A, Huang M, Jin S, Xiong W, Wang B, Chen D, Li T, Hou X, Liu L, Zhou X, Chen W and Chen X (2022) Deep cooling scheme of quantum degenerate gas and ground experimental verification for chinese space station. Front. Phys. 10:971059. doi: 10.3389/fphy.2022.971059
Received: 16 June 2022; Accepted: 19 August 2022;
Published: 07 September 2022.
Edited by:
Andrea Bertoldi, ParisTech Institut d’Optique Graduate School, FranceReviewed by:
Nassim Zahzam, Office National d'Études et de Recherches Aérospatiales, FranceCopyright © 2022 Li, Yu, Yuan, Wu, Xie, Li, Liang, Huang, Jin, Xiong, Wang, Chen, Li, Hou, Liu, Zhou, Chen and Chen. This is an open-access article distributed under the terms of the Creative Commons Attribution License (CC BY). The use, distribution or reproduction in other forums is permitted, provided the original author(s) and the copyright owner(s) are credited and that the original publication in this journal is cited, in accordance with accepted academic practice. No use, distribution or reproduction is permitted which does not comply with these terms.
*Correspondence: Xuzong Chen, eHV6b25nY2hlbkBwa3UuZWR1LmNu
Disclaimer: All claims expressed in this article are solely those of the authors and do not necessarily represent those of their affiliated organizations, or those of the publisher, the editors and the reviewers. Any product that may be evaluated in this article or claim that may be made by its manufacturer is not guaranteed or endorsed by the publisher.
Research integrity at Frontiers
Learn more about the work of our research integrity team to safeguard the quality of each article we publish.