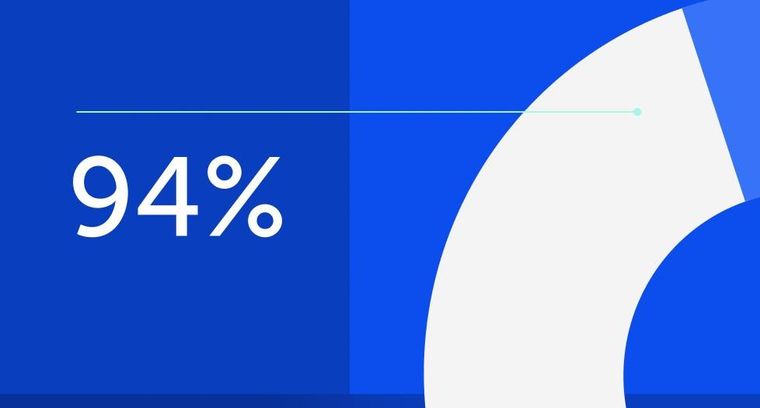
94% of researchers rate our articles as excellent or good
Learn more about the work of our research integrity team to safeguard the quality of each article we publish.
Find out more
ORIGINAL RESEARCH article
Front. Phys., 29 August 2022
Sec. Interdisciplinary Physics
Volume 10 - 2022 | https://doi.org/10.3389/fphy.2022.961671
Heat transport over an upper paraboloid horizontal surface of revolution (UPHSR) has attracted much interest of the researchers and aero dynamists. The flow of fluid around the pointed surface of rocket, bullets, bonnet, and fighting aircrafts exhibits flows in which engineering parameters like shear stresses and Nusselt number significantly contributed. Therefore, the study of fluid comprising heat generation/absorption, Lorentz forces, and chemical reaction over a radiative UPHSR is conducted. The basic constitutive relations are transformed into dimensionless version via similarity variables and tackled numerically. The temperature
The flow characteristics over an upper paraboloid horizontal surface of revolution (UPHSR) are significant and have attracted much interest from researchers and scientists. Flows of such a nature are seen across the tops of vehicles, the top face of bullets, and in aerodynamic phenomena. In this light, flow behavior over an UPHSR is a potential topic of interest in the field of fluid dynamics.
Keeping in mind the concept of the flow over an upper horizontal paraboloid surface of revolution, the researchers have focused on heat transport and fluid motion characteristics under various flow situations. Recently, Khan et al. [1] explored the behavior of Carreau liquid over a UPHSR. The model was introduced for bioconvection fluid by considering the influences of chemical reaction. The dimensionless comprising a new parametrization for Carreau fluid was treated by implementing Keller box techniques and decorated the results against the flow parameters. The reduction in the velocity of the bioconvection fluid against the stronger magnetic field was explored in the study. Moreover, they concluded that the stronger Prandtl effects reduced the fluid temperature and thermophoresis, and Brownian effects led to an increment in the bioconvection fluid temperature.
In 2020, Santoshi et al. [2] presented the analysis of non-Newtonian fluid over a paraboloid of revolution. They adopted similarity transformations for the nondimensionalization of the model and then found impacts for various flow quantities, such as the Prandtl number, the Casson parameter, and the Weissenberg number for the flow properties. They indicated that the fluid motion drops by increasing the strength of the imposed magnetic field. Furthermore, augmentation to fluid temperature was reported against the Casson parameter. The influences of thermal radiations on the flow behavior are significant from an industrial and engineering point of view. In this regard, Makinde et al. [3] modeled a flow problem by comprising the thermal radiations effects in constitutive relations and attained a dimensionless version of the model via similarity transformation. They elaborated the results for the fluid temperature and motion and discussed them comprehensively.
In recent decades, researchers and scientists focused on exploring heat transport mechanisms in nanofluids [4]. Nanofluids are newly engineered colloidal suspensions of a host liquid and metallic nanomaterials. These have attracted attention among scientists and engineers due to their superior thermal performance compared to conventional liquids. The Lorentz forces significantly alters the characteristics of the nanofluids. Therefore, Animasaun et al. [5] conducted thermal transport in the nanofluids by considering the impacts of Lorentz forces [6] over an UPHSR. The colloidal mixture is made up of 29 nm CuO nanomaterials, and water was taken as the host liquid. It was assumed that the metallic nanoparticles were uniformly diluted in the host liquid. Significant alterations in the nanofluid velocity in the vicinity of UPHSR and augmentation in the thermal field were examined against a higher volumetric fraction.
Another significant analysis regarding the heat transport in the nanofluids over an UPHSR was conducted by Animasaun et al. [7] in 2019. They presented an analysis in the presence of Lorentz forces ([8]) and reported the fascinating flow behavior and thermal transport in the nanofluid against multiple flow quantities. The heat transfer and fluid motion for gyrotactic microorganisms in the presence of thermoelectric phenomena was reported in [9]. The authors examined the maximum fluid motion against Peclet and buoyancy parameters. To enhance the novelty of the study, they also incorporated the thermal radiation effects in the governing model and explored significant results for thermal performance in the flow of gyrotactic microorganisms.
The flow over a UPHSR exhibits a boundary layer flow and practiced in daily life and in engineering sides as well. The boundary layer is a thin layer of fluid in which the effects of viscosity are dominant. Such flows occur naturally around the wings of fish, flow of air around birds, and other occasions species. Therefore, the applications of such flows comprised in solar collectors, aeronautical engineering, and in aerodynamics. In 2017, Reddy et al. [10] introduced a computational model over an UPHSR by taking convective flow condition and Lorentz forces. To intensify the heat transport mechanism in the fluid, they used ferro magnetic nanomaterials and gained a colloidal model. They examined a better heat transfer rate in ferrofluid in comparison with simple fluid. Moreover, the results for skin friction coefficient and the local thermal performance rate were elaborated in the form of numeric values.
The study of micropolar fluid over UPHSR in the presence of chemical reaction was carried out by Koriko and Animasaun [11] in 2017. They revealed that the temperature-dependent viscosity leads to an increment in the vertical fluid motion near the free stream, but the microrotation parameter declines near UPHSR. Furthermore, thermal radiation [12] had a significant influence on fluid thermal performance and walls shear stresses. The viscous dissipation effects greatly influence and alter the fluid characteristics over a finite or semi-infinite regions. In 2017, Ajayi et al. [13] detected the results for Casson dissipative fluid over a thermally radiative UPHSR. They reduced the constitutive relations for the momentum and energy into a dimensionless flow model, and then for mathematical analysis of the model, they utilized an RK scheme and portrayed the results in opposition to the flow parameters. They explored the significant effects of St on the wall shear stresses. Furthermore, significant investigation of the fluid characteristics over different geometries and flow conditions were reported in [14, 15] and in the literature cited therein.
The investigation of thermal performance in variety of nanofluids under bunch of physical conditions representing the fluid flow behavior at the boundaries with certain sources (magnetic field, thermal radiations [16], viscous dissipation, heat generation/absorption etc.) and activation energy is examined in [17–21]. The authors pointed that thermal radiations and activation energy are better sources to improve internal heat ability of the nanofluids particularly is Sutterby nanofluid. The exploration of fluid behavior in porous media is very significant and is of huge importance for underground reservoirs. In this context, a study is reported by Turkyilmazoglu [22] and engaged the study with novel aspects of solar heat, MHD, and internal thermal sources. The role of hybrid nanomaterials (Cu-Al2O3) for thermal enhancement in water under the impact of MHD, radiation source, and surface slip are discussed by Wahid et al. [23]. A recent analysis of heat transport was conducted by taking nonvariable magnetic field along a horizontal direction in [24].
Consider two-dimensional, steady, and laminar flow of chemically reacting and electrically conducting Newtonian fluid past a thermally stratified upper horizontal surface of paraboloid of revolution place in Cartesian coordinate system. The coordinate axes are taken in such a way that
The equations that govern the flow of chemically reacting and electrically conducting fluid over a paraboloid surface of revolution are the following [3, 13, 15]:
The law of conservation of mass is given as in Eq. 1. The momentum and energy equations in the presence of magnetic field, internal heat source, and resistive heating are given in Eqs 2, 3, respectively. Furthermore, Eq. 4 shows the mass transfer of the fluid. The dimensional physical quantities ingrained in Eqs 1–4 are fluid density
For our flow model, feasible set of boundary conditions for fluid motion, temperature, and concentration at the surface of paraboloid and away from the surface of paraboloid are listed in Eqs 5, 6. Physically, these conditions convey the message that velocity and concentration at
Furthermore, supporting self-similar variables are defined in the following way:
In Eq. 7,
The dimensional form of the flow model reduced into the following set of nonlinear differential equations by means of Eqs 7–9.
Here, it is important to mention that the lower value of
This implies that suitable auxiliary conditions obtain at the surface for feasible scale at
Furthermore, to restrict the domain
For our flow problem, nondimensional boundary conditions are set in the following manner:
The non-dimensional physical quantities appeared in Eqs 16–18 are as follows:
Quantities of physical and practical interest, such as skin friction coefficient, local heat transfer, and mass transfer gradients in nondimensional form, are as follows:
Here,
For our flow model, it is very difficult to perform analytical computations, or such solutions may not even exist. Thus, for the flow model, we adopted numerical technique called the Runge-Kutta scheme [25–27]. First, we transformed under consideration flow model in a system of first-order initial value problem, and then computations are carried out by means of Mathematica 10.0. To obtain the first-order initial value problem, we made the following substitutions:
The system given in Eqs 16-18 can be written in the following manner:
With the help of the substitution given in Eq. 22, we arrive with the following system of first-order initial value problem:
The set of initial conditions is as follows:
Here,
This section explores the influence of various dimensionless physical quantities appearing in the flow in terms of the velocity, temperature, and concentration of the fluid. These quantities play significant role in the flow regimes. In this study, these parameters are magnetic number, Prandtl number, Eckert number, radiation parameter, chemical reaction parameter, Schmidt number, and Biot number. For this purpose, Figures 2–8 are portrayed. Three-dimensional scenario of the flow regimes is also provided. Furthermore, in this section numerical computations for skin friction coefficient, local rate of heat, and mass transfer are carried out for varying physical quantities.
Figures 2A,B illustrate the impact of the velocity index parameter
The effects of the Eckert number
The next set of figures highlight the variations in fluid temperature
The influence of thermal radiation and the magnetic parameter
The influence of chemical reaction parameter
The variations in skin friction coefficient, local rate of heat transfer (local Nusselt number), and local rate of mass transfer (Sherwood number) are described in Table 2 due to variations in pertinent flow parameters. It is noted that for increasing velocity index parameter
The current investigation is validated through numerical comparison with previously described data in the literature. This was carried out using certain assumptions for the model, and a fine comparison between both the current and reported results for
A novel study regarding the heat and mass transfer over an UPHSR subject to magnetic field, radiation effects, chemical reaction, and internal heat source is reported. The results for the flow field demonstrated and indicated that:
• The velocity index parameter opposes the fluid motion inside the flow field.
• Thermal field
• The more chemically reacting fluid and increasing Schmidt number,
• The skin friction coefficient shows absolutely increasing behavior for
• The local heat and mass transfer rate intensify by enhancing convection from the surface and Schmidt effects.
The raw data supporting the conclusion of this article will be made available by the authors, without undue reservation.
All authors listed have made a substantial, direct, and intellectual contribution to the work and approved it for publication.
The authors would like to thank the Deanship of Scientific Research at Umm Al-Qura University for supporting this work by Grant Code: (22UQU4310392DSR18).
The authors declare that the research was conducted in the absence of any commercial or financial relationships that could be construed as a potential conflict of interest.
All claims expressed in this article are solely those of the authors and do not necessarily represent those of their affiliated organizations, or those of the publisher, the editors, and the reviewers. Any product that may be evaluated in this article, or claim that may be made by its manufacturer, is not guaranteed or endorsed by the publisher.
1. Khan M, Hussain A, Malik MY, Salahuddin T. Biconvection Flow of Carreau Fluid over an Upper Paraboloid Surface: A Computational Study. Results Phys (2017) 7:4050–7. doi:10.1016/j.rinp.2017.10.023
2. Santoshi PN, Reddy GVR, Padma P. Flow Features of Non-newtonian Fluid through a Paraboloid of Revolution. Int J Appl Comput Math (2020) 6. doi:10.1007/s40819-020-00828-z
3. Makinde OD, Sandeep N, Ajayi TM, Animasaun IL. Numerical Exploration of Heat Transfer and Lorentz Force Effects on the Flow of MHD Casson Fluid over an Upper Horizontal Surface of a Thermally Stratified Melting Surface of a Paraboloid of Revolution. Int J Nonlinear Sci Numer Simulation (2018) 19:93–106. doi:10.1515/ijnsns-2016-0087
4. Adnan Ahmed N, Mohyud-Din ST, Hamadneh NN, Khan I, Andualem . The Dynamics of H2O Suspended by Multiple Shaped Cu Nanoadditives in Rotating System. J Nanomater (2021) 2021:1–11. doi:10.1155/2021/7299143
5. Animasaun IL, Mahanthesh B, Jagun AO, Bankole TD, Sivaraj R, Shah NA, et al. Significance of Lorentz Force and Thermoelectric on the Flow of 29 Nm CuO-Water Nanofluid on an Upper Horizontal Surface of a Paraboloid of Revolution. J Heat Transfer (2019) 141(2). doi:10.1115/1.4041971
6. Khan W, Khan U, Adnan Ullah B, Khan I, Alqahtani AM, et al. The Effects of Magneto-Radiative Parameters on the Heat Transfer Mechanism in H2O Composed by Cu-Al2O3 Hybrid Nanomaterial: Numerical Investigation. Math Probl Eng (2022) 2022:1–10. doi:10.1155/2022/3098781
7. Animasaun IL, Mahanthesh B, Jagun AO, Bankole TD, Sivaraj R, Shah NA, et al. Significance of Lorentz Force and Thermoelectric on the Flow of 29 Nm CuO-Water Nanofluid on an Upper Horizontal Surface of a Paraboloid of Revolution. J Heat Transfer-transactions Asme (2019) 141. doi:10.1115/1.4041971
8. Abbasi A, Ahmed N, Mohyud-Din ST. Flow of Magneto-Nanofluid over a Thermally Stratified Bi-directional Stretching Sheet in the Presence of Ohmic Heating: A Numerical Study of Particle Shapes. Eng Computations (2017) 34(8):499–2513.
9. Sivaraj R, Animasaun IL, Olabiyi AS, Saleem S, Sandeep N. Gyrotactic Microorganisms and Thermoelectric Effects on the Dynamics of 29 Nm CuO-Water Nanofluid over an Upper Horizontal Surface of Paraboloid of Revolution. Mmms (2018) 14:695–721. doi:10.1108/MMMS-10-2017-0116
10. Ramana Reddy JV, Sugunamma V, Sandeep N. Enhanced Heat Transfer in the Flow of Dissipative Non-newtonian Casson Fluid Flow over a Convectively Heated Upper Surface of a Paraboloid of Revolution. J Mol Liquids (2017) 229:380–8. doi:10.1016/j.molliq.2016.12.100
11. Koriko LIOK, Animasaun IL. New Similarity Solution of Micropolar Fluid Flow Problem over an UHSPR in the Presence of Quartic Kind of Autocatalytic Chemical Reaction. Front Heat Mass Transfer (2017) 8(26). doi:10.5098/hmt.8.26
12. Ahmed N, Adnan Khan U, Khan I, Murtaza R, Hussain I, et al. A Novel Investigation and Hidden Effects of MHD and Thermal Radiations in Viscous Dissipative Nanofluid Flow Models. Front Phys (2020) 8. doi:10.3389/fphy.2020.00075
13. Ajayi TM, Omowaye AJ, Animasaun IL. Viscous Dissipation Effects on the Motion of Casson Fluid over an Upper Horizontal Thermally Stratified Melting Surface of a Paraboloid of Revolution: Boundary Layer Analysis. J Appl Maths (2017) 2017:1–13. doi:10.1155/2017/1697135
14. Kumaran G, Sandeep N, Animasaun IL. Computational Modeling of Magnetohydrodynamic Non-newtonian Fluid Flow Past a Paraboloid of Revolution. Alexandria Eng J (2018) 57:1859–65. doi:10.1016/j.aej.2017.03.019
15. Abegunrin OA, Animasaun IL, Sandeep N. Insight into the Boundary Layer Flow of Non-newtonian Eyring-Powell Fluid Due to Catalytic Surface Reaction on an Upper Horizontal Surface of a Paraboloid of Revolution. Alexandria Eng J (2018) 57:2051–60. doi:10.1016/j.aej.2017.05.018
16. Adnan Ashraf W, Junaid Anjum H, Mousa IM, Mehrez S. Energy Transformation and Entropy Investigation in the Nanofluid Composed by γ-Nanomaterial over a Permeable Convective Surface with Solar Thermal Radiation: A Numerical Analysis. Front Energ Res. (2022) 10. doi:10.3389/fenrg.2022.888389
17. Azam M. Effects of Cattaneo-Christov Heat Flux and Nonlinear thermal Radiation on MHD Maxwell Nanofluid with Arrhenius Activation Energy. Case Stud Therm Eng (2022) 34:102048. doi:10.1016/j.csite.2022.102048
18. Azam M, Abbas Z. Recent Progress in Arrhenius Activation Energy for Radiative Heat Transport of Cross Nanofluid over a Melting Wedge. Propulsion Power Res (2021) 10(4):383–95. doi:10.1016/j.jppr.2021.11.004
19. Azam M, Mabood F, Khan M. Bioconvection and Activation Energy Dynamisms on Radiative Sutterby Melting Nanomaterial with Gyrotactic Microorganism. Case Stud Therm Eng (2022) 30:101749. doi:10.1016/j.csite.2021.101749
20. Azam M. Bioconvection and Nonlinear thermal Extrusion in Development Ofchemically Reactive Sutterby Nano-Material Due to Gyrotactic Microorganisms. Int Commun Heat Mass Transfer (2022) 130:105820. doi:10.1016/j.icheatmasstransfer.2021.105820
21. Azam M, Xu T, Mabood F, Khan M. Non-linear Radiative Bioconvection Flow of Cross Nano-Material with Gyrotatic Microorganisms and Activation Energy. Int Commun Heat Mass Transfer (2021) 127:105530. doi:10.1016/j.icheatmasstransfer.2021.105530
22. Turkyilmazoglu M. MHD Natural Convection in Saturated Porous media with Heat Generation/absorption and thermal Radiation: Closed-form Solutions. Arch Mech (2019) 71(1):49–64.
23. Wahid NS, Md Arifin N, Turkyilmazoglu M, Hafidzuddin MEH, Abd Rahmin NA. MHD Hybrid Cu-Al2O3/Water Nanofluid Flow with Thermal Radiation and Partial Slip Past a Permeable Stretching Surface: Analytical Solution. JNanoR (2020) 64:75–91. doi:10.4028/www.scientific.net/JNanoR.64.75
24. Turkyilmazoglu M. Flow and Heat over a Rotating Disk Subject to a Uniform Horizontal Magnetic Field. Z für Naturforschung A (2022). doi:10.1515/zna-2021-0350
25. Adnan Ashraf W, Khan I, Andualem M. Thermal Transport in Radiative Nanofluids by Considering the Influence of Convective Heat Condition. J Nanomater (2022) 2022:1–11. doi:10.1155/2022/1854381
26. Adnan Ashraf W, Khan I, Andualem M. Thermal Transport Investigation and Shear Drag at Solid-Liquid Interface of Modified Permeable Radiative-SRID Subject to Darcy-Forchheimer Fluid Flow Composed by γ-nanomaterial. Sci Rep (2022) 12. doi:10.1038/s41598-022-07045-2
27. Adnan Khan I, Shemseldin MA, Mousa AAA. Numerical Energy Storage Efficiency of MWCNTs-Propylene Glycol by Inducing Thermal Radiations and Combined Convection Effects in the Constitutive Model. Front Chem (2022) 10. doi:10.3389/fchem.2022.879276
28. Animasaun IL, Sandeep N. Buoyancy Induced Model for the Flow of 36 Nm Alumina-Water Nanofluid along Upper Horizontal Surface of a Paraboloid of Revolution with Variable thermal Conductivity and Viscosity. Powder Tech (2016) 301:858–67. doi:10.1016/j.powtec.2016.07.023
Keywords: heat transfer, resistive heating, MHD, paraboloid of revolution, heat generation/absorption
Citation: M. Alharbi KA, Adnan , Tag-Eldin E, F. Yassen M, Ahmed N and Khan U (2022) Numerical Hydromagnetic Thermal Mechanism in Chemically Reacting Fluid Over a Radiative Melting UPHSR With Resistive Heating. Front. Phys. 10:961671. doi: 10.3389/fphy.2022.961671
Received: 05 June 2022; Accepted: 20 June 2022;
Published: 29 August 2022.
Edited by:
Muhammad Mubashir Bhatti, Shandong University of Science and Technology, ChinaReviewed by:
Muhammad Azam, Beijing Institute of Technology, ChinaCopyright © 2022 M. Alharbi, Adnan, Tag-Eldin, F. Yassen, Ahmed and Khan. This is an open-access article distributed under the terms of the Creative Commons Attribution License (CC BY). The use, distribution or reproduction in other forums is permitted, provided the original author(s) and the copyright owner(s) are credited and that the original publication in this journal is cited, in accordance with accepted academic practice. No use, distribution or reproduction is permitted which does not comply with these terms.
*Correspondence: Adnan, YWRuYW5fYWJiYXNpODlAeWFob28uY29t
†ORCID: Khalid Abdulkhaliq M. Alharbi, https://orcid.org/0000-0002-7290-8398; Adnan, https://orcid.org/0000-0003-0071-4743
Disclaimer: All claims expressed in this article are solely those of the authors and do not necessarily represent those of their affiliated organizations, or those of the publisher, the editors and the reviewers. Any product that may be evaluated in this article or claim that may be made by its manufacturer is not guaranteed or endorsed by the publisher.
Research integrity at Frontiers
Learn more about the work of our research integrity team to safeguard the quality of each article we publish.