- 1Science Department, Texas A&M University at Qatar, Doha, Qatar
- 2QMC, Livonia, MI, United States
- 3Qatar Computing Research Institute, Hamad Bin Khalifa University, Doha, Qatar
- 4Siemens Medical Solutions, Malvern, PA, United States
- 5Department of Radiology and Radiological Sciences, Medical University of South Carolina, Charleston, SC, United States
- 6Radiography and Diagnostic Imaging, University College Dublin, Dublin, Ireland
In light of widely expanding personalized medicine applications and their impact on clinical outcomes, it is naturally befitting to explore all the dimensional aspects of personalized radionuclide therapy (RNT). Adoption of absorbed radiation dose into clinical practice in the field of RNT has been hampered by difficulties such as evidence of dose-effect correlation, technical requirements in quantitative imaging of the radiopharmaceutical, heterogeneity of methods between not only centers, but also across software, hardware and radionuclides used. Additionally, standardized agreed upon definition of outcome measures is being debated whether it be solely related to toxicity, quality of life, survival or other measures. Many clinical RNT activity administrations are still based on empirical/fixed activities, or scaled based on parameters such as body surface area. Although still challenging, a tremendous amount of progress has been made to facilitate routine clinical dosimetry with discussions regarding standardization, harmonization and automated processing techniques. This has also been aided by the development and FDA approval of several companion diagnostics allowing within the theranostic paradigm not only a crude qualitative predictive biomarker but also an objective dosimetry based predictive therapeutic biomarker. This work aims to review the literature of [177Lu]Lu-PSMA RNT, focusing on clinical trials and studies, with the goal to summarize the range of dosimetry techniques and the range of doses calculated to organs and tissues of interest from these techniques. A dosimetry method for [177Lu]Lu-PSMA RNT should be reliable, reproducible and encompassing the knowledge gained from all clinical trials evaluating it. Its translation into clinical routine practice can be achieved with the confirmation that dose calculation represents good clinical efficacy and low treatment-related toxicity. Finally, some future perspectives on the future of [177Lu]Lu-PSMA RNT are made, especially in the rapidly emerging field of artificial intelligence (AI), where deep learning may be able to play a large role in the simplification of dosimetry calculations to aid in their clinical adoption.
Introduction
Prostate cancer (PC) is currently the most prevalent malignancy and the second most frequent cause of cancer mortality in adult men worldwide [1]. It is expected that the incidence and mortality will increase by approximately 57 and 64%, respectively, from the years 2020–2040 [2]. Latest statistics, provided by the National Institutes of Health (NIH), predict approximately 268,490 new diagnoses and 34,500 deaths in the United States in 2022 [3].
As the disease progresses, systemic chemotherapeutic options for metastatic castration-resistant PC (mCRPC) patients involve docetaxel and cabazitaxel as first and second line agents, respectively. Patients failing chemotherapy, eligibility (i.e. due to contraindications) and whose cancers become advanced or aggressive may be managed with prostatectomy, radiation therapy or androgen deprivation therapy. 10–20% of PC patients, experience disease progression into CRPC within 5 years of follow up, following surgical or medical castration (i.e., androgen deprivation) [4]. According to the Prostate Cancer Working Group 2 criteria, CRPC is defined as PC with any progression occurring in the presence of castrate-level testosterone levels, and may present itself as a continuous rise in prostate-specific antigen (PSA) serum levels, or progression in known sites of disease or with new metastases [5]. 90% of CRPC patients develop bone metastases causing significant morbidity, such as pain, pathologic fractures and bone marrow failure [6].
Early radioligand imaging for PC relied mainly on monoclonal antibodies. The murine (mAb) 7E11 and the humanized mAb hJ591 developed in the 90s showed some drawbacks such as long circulation half-life, low signal to noise ratio and poor target tissue uptake [7]. The 7E11 was developed as a theranostic agent, with [111In]In-7E11 (ProstaScint) as the diagnostic radiopharmaceutical (itself with poor positive predictive value), to potentially be used with SPECT imaging and [90Y]Y-7E11 as its therapeutic counterpart. However, when [90Y]Y-7E11 showed high myelotoxic effects and the hJ591 showed overall poor sensitivity as a SPECT imaging agent, both stopped in development [8, 9]. At the same time, radiolabelled J591 mAb, as [89Zr]Zr-hJ591, was investigated for PET/CT imaging with its therapeutic counterpart being [177Lu]Lu-hJ591 [9, 10].
More recently in the clinical space, the FDA has in 2020 and 2021 approved two diagnostic imaging agents [68Ga]Ga-PSMA-11 [11], and [18F]F-DCFPyL [Pylarify] [12]. Both agents work by imaging the prostate-specific-membrane-antigen (PSMA) protein, which is often found in large amounts on prostate cancer cells and is associated with biologic aggressiveness. Upon the development of small molecule PSMA peptide inhibitors, studies have shown them to be a superior alternative to antibody-based imaging agents [13]. The key parameters in mind when these agents were under development, was to ensure high PSMA affinity and rapid blood clearance and excretion [7].
Multi-center prospective trials have demonstrated the sensitivity of these PET radiotracers for identifying small sites of metastatic disease which has a significant impact on the selection of treatment options. In the context of theranostics (i.e. imaging radioisotope surrogates for therapeutic radioisotopes) these two agents offer a potential paradigm to deliver predictive therapeutic dosimetry. This is of great importance especially in lieu of the recent 2022 FDA approval of [177Lu]Lu-PSMA as a therapeutic option in mCRPC patients.
177Lu is a beta emitter with t1/2 = 6.7 days, Eβ−(av) = 134 keV, Eβ−(max) = 496 keV, Dav = 0.6 mm, Dmax = 1.6 mm and emits several accompanying photons of 208 keV (11%) and 113 keV (6.4%), which can be used for pre and post-treatment diagnostic evaluation and dosimetry purposes via imaging with a gamma camera [177Lu]Lu-PSMA-617 is currently only used on the basis of individual therapeutic trials in some European countries [14], in addition to its anticipated use in the United States as it becomes readily available [15]. As with all types of radiation therapy, the goal of [177Lu]Lu-PSMA radionuclide therapy (RNT) is to achieve the highest therapeutic efficacy by delivering maximum absorbed dose into the tumor lesions while sparing healthy tissues. In order to achieve a desired radiation absorbed dose (minimum dose for maximum efficacy) and to estimate the absorbed dose after administration of the radiopharmaceutical, accurate dosimetry is needed pre- and post-treatment [16]. Dosimetry has played a critical role in the development of [177Lu]Lu-PSMA RNT, yet due to the difficulty of adopting dosimetry in routine clinical practice, it was not integrated into large, randomized studies initially [17]. Although dosimetry helped initially estimate the absorbed doses to healthy tissues and tumor lesions, it was not until wider utilization in phase 1 and 2 clinical trials that better knowledge of toxicities was gained [17]. The recently published European Association of Nuclear Medicine (EANM) procedure guidelines for [177Lu]Lu-PSMA RNT are good practice standards to follow in line with national and international legal or regulatory provisions [18]. The aim of these guidelines is to identify the appropriate candidates for therapy, provide a protocol consensus to aid when performing the treatment, summarize potential toxicities, safety considerations and efficacy data, and describe the value of dosimetry in the optimization of therapy and its necessity to be carried out when the treatment to be given differs from the approved protocol [19]. These guidelines are similar to those published on 90Y microspheres [20] [131I]I-MIBG [21] [131I]I-NaI [22], and [177Lu]Lu-DOTATATE [23], summarizing the views of the EANM/Medical Internal Radiation Dose (MIRD) Committees for therapy optimization. Additional guidance has also been provided by EANM RNT dosimetry committee recommendations [24].
The purpose of this review on 177Lu-PSMA RNT dosimetry is to give a brief overview on imaging-based dosimetry methods, and primarily to provide a summary on the 177Lu-PSMA clinical trials that have been carried out or are ongoing, and the results of dosimetry from these trials. In this context, we present current efforts and highlight future perspectives on this matter.
Imaging-Based Dosimetry
To ensure that the radiation doses to organs at risk (OAR), (i.e. kidneys, salivary and lacrimal glands) in 177Lu-PSMA RNT is minimized, accurate determination of the activity of 177Lu within a defined volume is required and, hence, imaging-based dosimetry can fulfil this purpose [23]. The collection of fully quantitative data is an important step in accurate dosimetry calculations, as issues may arise with efficacy or adverse side effects with inaccurate calibrations for quantitative dosimetry [25].
Imaging-based dosimetry involves the patients being imaged at different time points, which allows for the rate of activity accumulation and depletion in each organ to be determined post-administration. To increase the accuracy of the dose estimates, the number of imaging time points acquired can be increased, however this is to be balanced against many logistical issues such as the patients’ willingness to be scanned over many days, scanner availability and cost with potential insurance/chargeback issues. Studies have been performed into the optimal timepoints for imaging in PSMA therapy, with a recent simulation study demonstrating that four timepoint imaging showed similar results as with three timepoints, and that a total imaging time of 96 h was feasible [26].
There are two main imaging-based dosimetry methods commonly used: dosimetry performed with 2D planar scintigraphy and 3D imaging using single-photon emission computed tomography (SPECT) or positron emission tomography (PET), or a combination of both methods using dual modality with CT [27]. The hybrid 2D and 3D SPECT/CT methods allows for the gathering of half-life information from planar images as well as 3D image derived local uptake distribution. The 2D planar data-based absorbed dose estimates approach has long been the method of choice for its ease of use and thorough documentation [28]. In this case the well-recognized MIRD dosimetry schema has been implemented through a range of commercial and freely available software solutions such as OLINDA (https://www.hermesmedicalsolutions.com/organdosimetry/) and OpenDose (https://www.opendose.org/). The shift from using planar imaging alone to tomographic imaging, or a combination of both, has provided an increase in the accuracy of activity quantification for dosimetry [29]. As an example, a comparison of 2D planar-based, 3D SPECT/CT-based, and hybrid dosimetry that combines 2D and 3D data demonstrated high agreement between the absorbed doses of normal organs using 3D and hybrid dosimetry for normal organs (median up to 4.0%) [30]. However, substantial differences (median up to 10.9%) were reported when 2D and 3D dosimetry approaches were compared. Hybrid dosimetry was found to have high accuracy when estimating the absorbed dose, relative to 3D dosimetry for all organs of interest. Despite the potential accuracy of 3D dosimetry, it is important to note however, that evaluation of SPECT/CT reconstruction techniques are vital in ensuring accurate dosimetry quantification. Recent work demonstrated pitfalls using different reconstruction schemes of a kidney cortex/medulla phantom, noting that large differences from the true organ distribution can be obtained, and demonstrated a potential remedy by using a partial volume correction technique [31].
When quantitative SPECT is fully utilized, attenuation and scatter correction are required, but also corrections for dead time and distant-dependent detector blurring, which degrade image quality. The wide range of correction techniques found for SPECT makes the optimization process challenging. Also, with small organs such as the salivary/lacrimal glands, and inherently due to a lower spatial resolution, partial volume effects may be challenging and recovery coefficient corrections may be needed. 177Lu isotope specific guidelines for quantitative 177Lu SPECT (not specific for prostate PSMA) imaging are available in the MIRD pamphlet [16]. Examples of application of this quantitative 177Lu SPECT methodology in a physical phantom using a 20% energy window for photopeaks of 113 keV, 208 keV and a combination of both demonstrated a quantification error up to 40% for the 113 keV energy window only, <3.2% for the 208 keV window only and 14% error for a combination of windows [23].
Although not relevant for 177Lu imaging, Bremsstrahlung (continuous X-ray spectrum) imaging for certain theranostic isotopes that emit no or low-yield gamma rays (i.e. 90Y and 89Sr) may be employed, however the quantification of Bremsstrahlung imaging remains challenging [32].
Methods of Dosimetry Calculation
To date, there are several dosimetry techniques available, with the MIRD schema (developed by the MIRD Committee of the Society of Nuclear Medicine and Molecular Imaging (SNMMI)) being the most incorporated technique for radiolabelled PSMA [33,34,35]. The schema was developed as a means to estimate the average absorbed radiation doses of radiopharmaceuticals to patients’ organs, tissues, voxels and cellular compartments [36,37], via the computation of time-activity curves, which then allow for the calculation of the cumulative radioactivity in a volume of interest [38,39]. It can be implemented using “S-values” (absorbed dose rate per unit activity) that are determined using Monte Carlo (MC) simulations for different isotopes [40,41,42]. Limitations of S-value calculations are based on assumptions such as homogenous distribution of radioactivity within organs and standardized organ masses [43,44]. Due to its relative simplicity, quick algorithms that require 2D imaging, and the use of average organ characteristics, S-value dosimetry has been used in routine clinical settings and represents a minimum standard of dosimetry computation [35,45,46,47,48]. Additionally, in the past S-values used in dosimetric analysis have been used as a reference for new dosimetry methodologies [49,50,51].
Further accuracy in dosimetry calculations can be achieved using direct MC simulations of radiation transport. MC simulations involve input parameters that initiate an iterative statistical process, resulting in voxel-level absorbed dose calculations being carried out through estimating interactions of particles [43]. They can account for inhomogeneous radioactivity distribution and secondary particles, and allow for simulation in patient-specific organ and lesion geometries [44,45]. However, due to its computational and operational resources and expertise required to implement a simulation, MC simulation is used more so in research settings and can be considered a de facto reference standard due to the high level of accuracy that can be achieved.
In analogy with the MIRD formalism, MIRD pamphlet no.17 (1999) [39], provides voxel-based dosimetry using voxel S-values (VSV). Using MC simulations, VSV are calculated for specific isotopes and voxel dimensions [52,53]. Consequently a kernel matrix is used to estimate the mean absorbed dose for each voxel, whereby each voxel is a uniform source and each nearby voxel is a target ([54], [39]). This, as a result, generates a voxel-by-voxel dose map [56]. Although different MC codes may provide variances in dose estimates within a few percent, this is not a large enough margin to be considered relevant in a clinical setting [51],[44], [58]. The VSV method is particularly advantageous for its ability to handle inhomogeneous radioactivity distributions [54]. Figure 1 demonstrates a comparison of VSV, direct MC technique and a deep learning technique for dose rate calculation on images of the same patient.
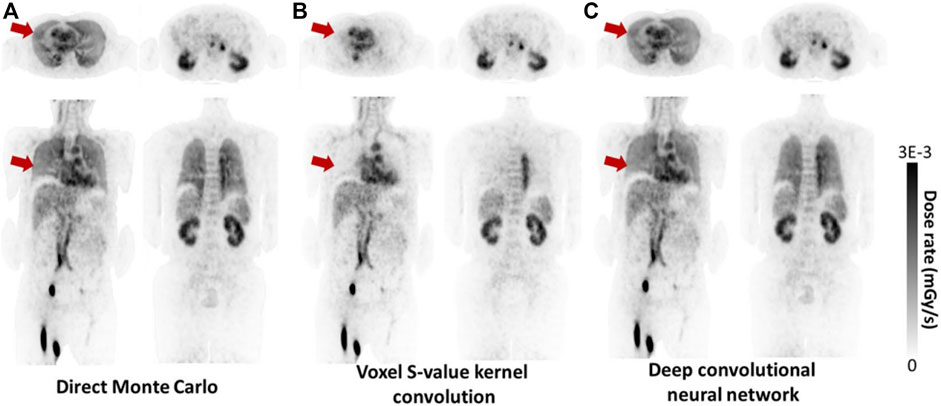
FIGURE 1. Example of dose rate maps produced by direct MC simulation (considered ground truth), voxel S-value convolution and a deep convolutional neural network (CNN). The CNN approach generates dose rate maps similar to MC, and better agreement in certain areas such as lungs compared to VSV, as highlighted by the red arrows (With copyright permission - [146]).
The local energy deposition method is yet another technique that is applied in dosimetry calculations, proposed to simplify the application of VSV for beta emitters. In this method, the assumption is that all of the energy is absorbed in the voxel of origin. It is primarily used for alpha and beta particles or auger electrons and not gamma emitters or secondary photons because of their longer range in tissue [59].
Various studies have demonstrated the use of simplified dosimetry calculation methodologies for potential clinical adaptation. Recent work reported on two simplified [177Lu]Lu-PSMA-617 dosimetry schemes for organs at risk, whereby one method simplified image acquisition and the other, dose calculation [60]. The simplified schemes were found to be feasible and showed potential for simplification in future works, and were both consistent with their reference method for cumulative absorbed dose. Mix et al. attempted to simplify dosimetry by using intratherapeutically acquired SPECT/CT scans to determine the kidney dose per cycle, noting that extrapolation of individual data from dosimetry of the first treatment cycle was highly predictive of the cumulative kidney dose at the end of treatment [61]. Other work similarly reported on the ability to approximate the [177Lu]Lu-DOTATATE absorbed doses to abdominal organs and lesions from a single time point measurement of the abdominal activity distribution [62]. The study found that a single measurement by SPECT/CT 4 days after the administration can be used to estimate the doses absorbed efficiently. A recent simulation study also looked at optimal sampling schedules for renal and tumor dosimetry for [177Lu]Lu-PSMA RNT. The study demonstrated that a timepoint at 192 h was necessary, and improvements in accuracy and precision could be achieved with careful selection of timepoints [63].
Clinical Trials
Since the beginning of 2021, the results of two large, randomized, controlled clinical trials, the TheraP [64] and VISION [65] trials, have been published showing promising clinical outcomes. Consequently, the international phase 3 VISION trial (NCT03511664) led to the Food and Drug Administration (FDA) granting breakthrough designation to [177Lu]Lu-PSMA RNT, eventually leading to its final approval in March 2022 [66]. Results reported on the overall survival (OS) and radiographic progression-free survival (rPFS) of [177Lu]Lu-PSMA RNT plus protocol-permitted standard of care (SOC) in 831 prostate cancer patients, with a sub-dosimetry study aimed at estimating the absorbed dose in organs at risk. For the dosimetry study, a separate cohort of 29 patients from four centers received 7.4 GBq of [177Lu]Lu-PSMA-617 per cycle in addition to SOC every 6 weeks for a maximum of 6 cycles. Whole body scintigraphy scans and SPECT/CT scans after the first administration of the RNT, as well as blood and urine samples were collected. The radiation absorbed doses per unit activity were found to be the highest in the lacrimal glands (mean 2.1 Gy/GBq) and the salivary glands (mean 0.63 Gy/GBq) [67].
The TheraP multicenter, randomized phase 2 trial (NCT03392428) reported on prostate-specific antigen (PSA) response of 201 patients receiving 6–8.5 GBq of [177Lu]Lu-PSMA-617 every 6 weeks for up to 6 cycles. Similarly, large clinical trials are underway such as UpFrontPSMA [68], PSMAfore [69], PSMAddition [70], and ENZA-p [71] that have not specifically included imaging-based dosimetric related outcomes. They have instead focused attention on endpoints such as efficacy, safety and toxicity, which may have been improved by looking at dosimetry implications. There are currently four phase 1 and 2 clinical trials underway with radiation dosimetry of [177Lu]Lu-PSMA in humans as an endpoint, as summarized in Table 1. A further study (NCT03042468) demonstrated that in a phase 1 dose-escalation cohort patients received 7.4–22 GBq and in phase 2 the patients received a 22.2 GBq dose, a maximum tolerable dose of 22.2 GBq of [177Lu]Lu-PSMA-617 is safe for a fractionated cycle [72].
Also under investigation is a trial of 43 patients (NCT03454750), who were administered 3.7–5.5 GBq of [177Lu]Lu-PSMA-617, four times at 8-weeks intervals [73]. Dosimetry was carried out using the MIRD formalism (OLINDA/EXM software) in 30.2% of the patients (n = 13), low grade hematological (with the exception of two patients showing G3), salivary gland and renal toxicity was reported, concluding that [177Lu]Lu-PSMA-617 is safe and salivary gland uptake may be reduced by co-administration of polyglutamate tablets.
Tumor Lesion Dosimetry
Recent work has shown high tumor doses for skeletal, lymph node, and liver metastases [74], and in some (prospective) studies for lung metastases as well [75] in patients administered with [177Lu]Lu-PSMA-617. Radiation dosimetry demonstrated high tumor absorbed doses, as shown in Table 2, and low exposure to OAR. Similarly, with [177Lu]Lu-PSMA-I&T, two studies reported on the absorbed doses to tumor lesions [88,89]. The studies found the highest absorbed doses in bone, lymph node, liver and lung tumor lesions [177Lu]Lu-PSMA-I&T was noted to achieve high tumor to background ratios of the mean absorbed doses [90,91].
Normal Organ and Tissue Dosimetry
Various studies have reported on absorbed doses to normal organs and tissues [74,75,76,77,89,92], as is shown in Table 3. Many retrospective and prospective studies, concluded that the organs with the highest absorbed doses were the kidneys and salivary glands [81,85,87,93,94]. Additional studies have also reported that the maximum cumulative doses to the kidneys and salivary glands were the highest [78,85,94] and the lacrimal glands possibly representing the dose-limiting organs [95]; they concluded that the doses did not exceed the commonly applied dose constraints for the kidney (23 Gy [96]) and salivary glands (45 Gy [93]) [97,98]. When the kidney doses were above the International Commission on Radiological Commission (ICRP) critical dose limits however, they were observed to not cause clinical complications due to nephrotoxicity [99].
Predictive Dosimetry
Many works have reported on pretherapeutic [68Ga]Ga-PSMA-617 PET possibly serving to indicate the dosimetry of [177Lu]Lu-PSMA-617. One such study by Wang et al. [74] reported on a high correlation between the standard uptake value (SUV) of tumor lesions in [68Ga]Ga-PSMA-617 PET and those in [177Lu]Lu-PSMA-617. Maffey-Steffan et al. [76] studied the 68Ga/177Lu-theranostic concept in PSMA-targeting of mCRPC patients. The study concluded that while whole body scintigraphy allows for accurate follow up of patients treated with [177Lu]Lu-PSMA-617 [68Ga]Ga-PSMA-11 PET/CT is recommended to be performed for both patient selection and final response assessment.
The use of [68Ga]Ga-PSMA-11 PET/CT for an absorbed estimation of [177Lu]Lu-PSMA RNT has not yet been evaluated extensively in literature. However, a recent study compared predicted absorbed doses to actual delivered doses; it did so through the use of [177Lu]Lu-PSMA SPECT imaging data to determine population tissue effective half-times and estimate dosimetry using a single time point pretherapeutic [68Ga]Ga-PSMA PET [102]. The PET/SPECT absorbed dose ratios were 1.01 ± 0.21 for the kidneys, 1.10 ± 0.15 for the liver, 1.20 ± 0.34 for the submandibular glands, and 1.11 ± 0.29 for the parotid glands. As for the lesion kinetics, a larger range was reported with a PET/SPECT absorbed dose ratio of 1.3 ± 0.7 (range: 0.4–2.7), possibly due to the difficulty in calculating the SPECT absorbed dose for small structures. This raises the increased probability of larger uncertainties for the calculations associated with these small volumes [103,104]. Predictive dosimetry has also been shown to be improved by obtaining continuous tracer distribution data 1 h post [68Ga]Ga-PSMA administration using PET imaging [105], however would likely suffer from complex acquisition logistics.
Discussion & Future Perspectives
Seeing the results of both retrospective and prospective studies, the dosimetry of [177Lu]Lu-PSMA RNT played a pivotal role in its FDA approval and hence its use in clinical practice. We project that the current ongoing clinical trials will bring more light to the dosimetry of [177Lu]Lu-PSMA, in hopes of optimizing its use so its clinical feasibility will be expanded. There are various studies that have investigated “cocktail therapy” (a combination of drugs used to treat a certain condition) [106], while others explored [177Lu]Lu-PSMA RNT post-exhaustion of standard treatment options. However, there are studies yet to evaluate the optimal sequencing of treatment of options.
Recently, alpha-based RNT has proven to be a promising therapeutic option in mCRPC patients with tumors refractory to beta RNT [107]. Clinical studies with alpha emitters such as 213Bi have suggested that contrary to beta-emitters RNT, patients do not develop resistance to alpha emitter therapy [108]. By lessening the localized dose pattern with higher energy beta electrons to achieve uniformity [109], or chelating PSMA molecules with radioisotopes, such as alpha emitters, capable of causing greater DNA damage through high LET emission [110], radiation dose delivery may be optimized. While 223RaCl is the first and only alpha emitting radioligand currently FDA and European Medicines Agency (EMA) approved for mCRPC with bone metastases with no known extra-skeletal metastases [111,112,113], radionuclides such as 149Tb, 211At, 213Bi, 212Pb/212Bi, 227Th, and 225Ac are being evaluated for their use in PSMA RNT preclinical and clinical studies [114,115,116,117,118]. The dosimetry of the latter three radionuclides for RNT in prostate cancer patients is being investigated with both PSMA and other chelators [119,120,121,122,123,124,125,126,127].
While encouraging results are being documented, some studies have reported on irreversible toxicities associated with alpha emitting PSMA agents. The shorter tissue range of alpha radiation has shown beneficial in targeting tumor cells that have penetrated bone marrow with a relatively lower toxicity when compared to beta emitters [110]. However, a high rate of irreversible xerostomia was reported as the dose-limiting toxicity upon exceeding 100 kBq/kg per cycle, thus leading to the use of 225Ac-PSMA therapy only for salvage therapy [107,110]. Similarly, irreversible grade 2 + neutropenia was observed in the use of 50kBq/kg of 223Ra in prostate cancer patients [128].
Artificial Intelligence in RNT
The field of diagnostic imaging has seen a huge increase in the use of AI in almost all aspects of the clinical patient pathway. For Nuclear Imaging, this has led to AI developments in patient positioning, attenuation and scatter correction [129], low-count image reconstruction techniques [130,131], event positioning in monolithic crystals [132] and various postprocessing applications. In external beam radiotherapy, AI has been investigated for radiation dose calculations such as optimization of treatment plan quality/uniformity with a reduction of planning time [133], prediction of human operator behavior in the treatment planning process of prostate IMRT [134] and automated organ at risk and lesion segmentation [135,136]. Although technically very similar, lesion segmentation has proven to be more challenging when compared to organ segmentation, due to the possible variability in the location, size and shape of lesions leading to unusual pathophysiology that may be unseen by AI training algorithms. Clinical tools from major vendors are available for automated/semi-automated segmentations with varying degrees of success in oncological cases. To improve efficiency and standardization, U-Net architecture using AI-based algortihms has shown promise [137,138,139]. A full review on AI developments in EBRT treatment planning and segmentation is reported by Wang et al [140].
Similarly, explorations are also underway to incorporate AI into RNT dosimetry, primarily where MC simulations are used as a reference standard. Recent work has used a deep neural network (DNN) to extend single S-value kernels to specific S-value kernels corresponding to patient-specific anatomy to construct 3D dose maps of [18F]FDG distribution [141]. Using training data of density maps (via CT images) and reference voxel wise S-values (generated using Monte Carlo simulations), whole-body dose maps can be constructed like the standard voxel-based MIRD scheme. The growth in the importance of mandatory regulation for each test that will be put to routine use in clinical practice, with the latest regulatory papers undergoing certification to prove reproducibility is expected [142,143]. Perhaps more studies are needed to address the challenges of how these AI ML models will join clinical practice to predict PC using histopathological or imaging methods for diagnosis [144].
Similar work aimed at incorporating dosimetry calculations into clinical practice with less computational effort used a U-net architecture with full MC simulation as a reference to predict individual absorbed dose distributions with inputs of CT (density maps) and dose maps (estimated using MIRD calculations, whole organ S-values and time activity data) [145]. This work examined the predictions of 26 177Lu-PSMA datasets (4 timepoint imaging), and their resulting method outperformed the standard MIRD DVK dose calculation method in terms of dose deviation. Similar work using data also trained from MC ground truth to predict a 3D dose rate map evaluated their method on 10 datasets of [68Ga]Ga-NOTA-RGD [146]. Their CNN-based dose rate map agreed with the ground truth with voxel dose rate errors of 2.54 ± 2.09%. Although their work demonstrates a proof of principle of learning dose estimates, and the ability to significantly reduce dosimetry calculation time while retaining accuracy of MC simulations, the cohort of these 2 studies are small. Similarly, many AI methods are limited by small cohort studies. Therefore, the collaborations between various clinical centers will be necessary for more generalizable models and accurate training data.
Such advances in AI dosimetry methods in diagnostic imaging, histopathology, and genomics offers the possibility for the delivery of personalized, precision medicine. Current work performed is remains difficult to standardize in terms of quantification, dosimetry calculation technique and analysis but offers good insight into the true potential of dosimetry in predicting toxicity and outcomes. The use of AI is promising and has the potential to truly deliver predictive dosimetry.
Author Contributions
All authors, RA, MD, OB and JD, contributed to the drafting of the manuscript and have approved the final manuscript.
Conflict of Interest
JD is an employee of Siemens Medical Solutions.
The remaining authors declare that the research was conducted in the absence of any commercial or financial relationships that could be construed as a potential conflict of interest.
Publisher’s Note
All claims expressed in this article are solely those of the authors and do not necessarily represent those of their affiliated organizations, or those of the publisher, the editors and the reviewers. Any product that may be evaluated in this article, or claim that may be made by its manufacturer, is not guaranteed or endorsed by the publisher.
References
1. von Eyben FE, Roviello G, Kiljunen T, Uprimny C, Virgolini I, Kairemo K, et al. Third-line Treatment and 177Lu-PSMA Radioligand Therapy of Metastatic Castration-Resistant Prostate Cancer: a Systematic Review. Eur J Nucl Med Mol Imaging (2018) 45(3):496–508. doi:10.1007/s00259-017-3895-x
2.World Health Organization (WHO). GLOBOCAN (2020). Available at: https://gco.iarc.fr/tomorrow/en/dataviz/bubbles?mode=population&cancers=39&populations=900&types=1 (Accessed December 6, 2021).
3.Cancer of the prostate - cancer stat facts. (2022). Available at: https://seer.cancer.gov/statfacts/html/prost.html#content (Accessed April, 2022). SEER
4. Kirby M, Hirst C, Crawford ED. Characterising the Castration-Resistant Prostate Cancer Population: a Systematic Review. Int J Clin Pract (2011) 65(11):1180–92. doi:10.1111/j.1742-1241.2011.02799.x
5. Scher HI, Halabi S, Tannock I, Morris M, Sternberg CN, Carducci MA, et al. Design and End Points of Clinical Trials for Patients with Progressive Prostate Cancer and Castrate Levels of Testosterone: Recommendations of the Prostate Cancer Clinical Trials Working Group. Jco (2008) 26(7):1148–59. doi:10.1200/JCO.2007.12.4487
6. Hotte SJ, Saad F. Current Management of Castrate-Resistant Prostate Cancer. Curr Oncol (2010) 17(Suppl. 2suppl 2):72–9. doi:10.3747/co.v17i0.718
7. Bois F, Noirot C, Dietemann S, Mainta IC, Zilli T, Garibotto V, et al. [68Ga]Ga-PSMA-11 in Prostate Cancer: a Comprehensive Review. Am J Nucl Med Mol Imaging (2020) 10(6):349–74. Published 2020 Dec 15.
8. Deb N, Goris M, Trisler K, Fowler S, Saal J, Ning S, et al. Treatment of Hormone-Refractory Prostate Cancer with 90Y-CYT-356 Monoclonal Antibody. Clin Cancer Res (1996) 2(8):1289–97.
9. Pandit-Taskar N, O’Donoghue JA, Beylergil V, Lyashchenko S, Ruan S, Solomon SB, et al. 89Zr-huJ591 Immuno-PET Imaging in Patients with Advanced Metastatic Prostate Cancer. Eur J Nucl Med Mol Imaging (2014) 41(11):2093–105. doi:10.1007/s00259-014-2830-7
10. Tagawa ST, Milowsky MI, Morris M, Vallabhajosula S, Christos P, Akhtar NH, et al. Phase II Study of Lutetium-177-Labeled Anti-prostate-specific Membrane Antigen Monoclonal Antibody J591 for Metastatic Castration-Resistant Prostate Cancer. Clin Cancer Res (2013) 19(18):5182–91. doi:10.1158/1078-0432.CCR-13-0231
11. Carlucci G, Ippisch R, Slavik R, Mishoe A, Blecha J, Zhu S. 68Ga-PSMA-11 NDA Approval: A Novel and Successful Academic Partnership. J Nucl Med (2021) 62(2):149–55. doi:10.2967/jnumed.120.260455
12. Solnes LB, Werner RA, Jones KM, Sadaghiani MS, Bailey CR, Lapa C, et al. Theranostics: Leveraging Molecular Imaging and Therapy to Impact Patient Management and Secure the Future of Nuclear Medicine. J Nucl Med (2020) 61(3):311–8. doi:10.2967/jnumed.118.220665
13. Lütje S, Slavik R, Fendler W, Herrmann K, Eiber M. PSMA Ligands in Prostate Cancer - Probe Optimization and Theranostic Applications. Methods (2017) 130:42–50. doi:10.1016/j.ymeth.2017.06.026
14.European Medicines Agency - Ema.europa.eu. Available at:https://www. https://www.ema.europa.eu/en/documents/pip-decision/p/0127/2019-ema-decision-17-april-2019-granting-product-specific-waiver-177lu-psma-617-emea-002419-pip02-18_en.pdf. Published 2019. (Accessed December 10, 2021).
15.FDA grants Priority Review for Investigational Targeted Radioligand Therapy 177Lu-PSMA-617 for Patients with Metastatic Castration-Resistant Prostate Cancer (mCRPC). Novartis. Available at: https://www.novartis.com/news/fda-grants-priority-review-investigational-targeted-radioligand-therapy-177lu-psma-617-patients-metastatic-castration-resistant-prostate-cancer-mcrpc. (2021). (Accessed December 10, 2021).
16. Ljungberg M, Gleisner K. Hybrid Imaging for Patient-specific Dosimetry in Radionuclide Therapy. Diagnostics (2015) 5(3):296–317. doi:10.3390/diagnostics5030296
17. Jackson P, Hofman M, McIntosh L, Buteau JP, Ravi Kumar A. Radiation Dosimetry in 177Lu-PSMA-617 Therapy. Semin Nucl Med (2022) 52(2):243–54. doi:10.1053/j.semnuclmed.2021.11.003
18. Kratochwil C, Fendler WP, Eiber M, Baum R, Bozkurt MF, Czernin J, et al. EANM Procedure Guidelines for Radionuclide Therapy with 177Lu-Labelled PSMA-Ligands (177Lu-PSMA-RLT). Eur J Nucl Med Mol Imaging (2019) 46:2536–44. doi:10.1007/s00259-019-04485-3
19. Konijnenberg M, Herrmann K, Kobe C, Verburg F, Hindorf C, Hustinx R, et al. EANM Position Paper on Article 56 of the Council Directive 2013/59/Euratom (Basic Safety Standards) for Nuclear Medicine Therapy. Eur J Nucl Med Mol Imaging (2021) 48:67–72. doi:10.1007/s00259-020-05038-9
20. Chiesa C, Sjogreen-Gleisner K, Walrand S, Strigari L, Flux G, Gear J, et al. EANM Dosimetry Committee Series on Standard Operational Procedures: a Unified Methodology for 99mTc-MAA Pre- and 90Y Peri-Therapy Dosimetry in Liver Radioembolization with 90Y Microspheres. EJNMMI Phys (2021) 8(1):77. doi:10.1186/s40658-021-00394-3
21. Gear J, Chiesa C, Chiesa C, Lassmann M, Gabiña PM, Tran-Gia J, et al. EANM Dosimetry Committee Series on Standard Operational Procedures for Internal Dosimetry for 131I mIBG Treatment of Neuroendocrine Tumours. EJNMMI Phys (2020) 7:15. doi:10.1186/s40658-020-0282-7
22.European Association of Nuclear Medicine - Eanm.org. Available at: https://www.eanm.org/publications/guidelines/2013_published_DC_SOP_Benign_Thyroid_Diseases.pdf. Published 2013. (Accessed December 10, 2021).
23. Ljungberg M, Celler A, Konijnenberg MW, Eckerman KF, Dewaraja YK, Sjögreen-Gleisner K. MIRD Pamphlet No. 26: Joint EANM/MIRD Guidelines for Quantitative 177Lu SPECT Applied for Dosimetry of Radiopharmaceutical Therapy. J Nucl Med (2016) 57(1):151–62. doi:10.2967/jnumed.115.159012
24. Sjögreen Gleisner K, Chouin N, Gabina PM, Cicone F, Gnesin S, Stokke C, et al. EANM Dosimetry Committee Recommendations for Dosimetry of 177Lu-Labelled Somatostatin-Receptor- and PSMA-Targeting Ligands. Eur J Nucl Med Mol Imaging (2022) 49:1778–809. doi:10.1007/s00259-022-05727-7
25. D’Arienzo M, Cazzato M, Cozzella ML, Cox M, D’Andrea M, Fazio A, et al. Gamma Camera Calibration and Validation for Quantitative SPECT Imaging with 177Lu. Appl Radiat Isot (2016) 112:156–64. doi:10.1016/j.apradiso.2016.03.007
26. Rinscheid A, Kletting P, Eiber M, Beer AJ, Glatting G. Influence of Sampling Schedules on [177Lu]Lu-PSMA Dosimetry. EJNMMI Phys (2020) 7(1):41. doi:10.1186/s40658-020-00311-0
27. Rinscheid A, Kletting P, Eiber M, Beer AJ, Glatting G. Technical Note: Optimal Sampling Schedules for Kidney Dosimetry Based on the Hybrid Planar/SPECT Method in 177 Lu‐PSMA Therapy. Med Phys (2019) 46(12):5861–6. doi:10.1002/mp.13846
28. Cremonesi M, Ferrari M, Bodei L, Tosi G, Paganelli G. Dosimetry in Peptide Radionuclide Receptor Therapy: a Review. J Nucl Med (2006) 47(9):1467–75.
29. Sgouros G, Bolch WE, Chiti A, Dewaraja YK, Emfietzoglou D, Hobbs RF, et al. ICRU REPORT 96, Dosimetry-Guided Radiopharmaceutical Therapy. JIcru (2021) 21(1):1–212. doi:10.1177/14736691211060117
30. He B, Nikolopoulou A, Osborne J, Vallabhajosula S, Goldsmith S. Quantitative SPECT Imaging with Lu-177: A Physical Phantom Evaluation. J Nucl Med (2012) 53(Suppl. 1):2407.
31. Tran-Gia J, Salas-Ramirez M, Lassmann M. What You See Is Not what You Get: On the Accuracy of Voxel-Based Dosimetry in Molecular Radiotherapy. J Nucl Med (2020) 61(8):1178–86. doi:10.2967/jnumed.119.231480
32. Li T, Ao ECI, Lambert B, Brans B, Vandenberghe S, Mok GSP. Quantitative Imaging for Targeted Radionuclide Therapy Dosimetry - Technical Review. Theranostics (2017) 7(18):4551–65. doi:10.7150/thno.19782
33. Emmett L, Willowson K, Violet J, Shin J, Blanksby A, Lee J. Lutetium177PSMA Radionuclide Therapy for Men with Prostate Cancer: a Review of the Current Literature and Discussion of Practical Aspects of Therapy. J Med Radiat Sci (2017) 64(1):52–60. doi:10.1002/jmrs.227
34. Hindorf C, Glatting G, Chiesa C, Lindén O, Flux G, Dosimetry Committee EANM. EANM Dosimetry Committee Guidelines for Bone Marrow and Whole-Body Dosimetry. Eur J Nucl Med Mol Imaging (2010) 37(6):1238–50. doi:10.1007/s00259-010-1422-4
35. Siegel JA, Thomas SR, Stubbs JB, Stabin MG, Hays MT, Koral KF, et al. MIRD Pamphlet No. 16: Techniques for Quantitative Radiopharmaceutical Biodistribution Data Acquisition and Analysis for Use in Human Radiation Dose Estimates. J Nucl Med (1999) 40(2):37S–61S.
36. Stabin MG, Sparks RB, Crowe E. OLINDA/EXM: the Second-Generation Personal Computer Software for Internal Dose Assessment in Nuclear Medicine. J Nucl Med (2005) 46(6):1023–7.
38. Goddu S, Howell RW, Bouchet LG, Bolch WE, Rao DV. MIRD Cellular S Values. Reston, VA: Soc Nucl Med (1997).
39. Bolch WE, Bouchet LG, Robertson JS, Wessels BW, Siegel JA, Howell RW, et al. MIRD Pamphlet No. 17: the Dosimetry of Nonuniform Activity Distributions-Rradionuclide S Values at the Voxel Level. Medical Internal Radiation Dose Committee. J Nucl Med (1999) 40(1):11S–36S.
40. Watson EE, Stabin MG, Siegel JA. MIRD Formulation. Med Phys (1993) 20(2 Pt 2):511–4. doi:10.1118/1.597046
41. Snyder WS, Ford MR, Warner GG, Watson SB. MIRD Pamphlet No 11: S, Absorbed Dose Per Unit Cumulated Activity for Selected Radionuclides and Organs. Soc Nucl Med (1975).
42. Snyder WS, Ford MR, Warner GG. Revision of MIRD Pamphlet No. 5 Entitled “Estimates of Absorbed Fractions for Monoenergetic Photon Sources Uniformly Distributed in Various Organs of a Heterogeneous Phantom. Soc Nucl Med (1978).
43. Kost SD, Dewaraja YK, Abramson RG, Stabin MG. VIDA: a Voxel-Based Dosimetry Method for Targeted Radionuclide Therapy Using Geant4. Cancer Biother Radiopharm (2015) 30(1):16–26. doi:10.1089/cbr.2014.1713
44. Lanconelli N, Pacilio M, Meo SL, Botta F, Dia AD, Aroche LAT, et al. A Free Database of Radionuclide Voxel S Values for the Dosimetry of Nonuniform Activity Distributions. Phys Med Biol (2012) 57(2):517–33. doi:10.1088/0031-9155/57/2/517
45. Van Binnebeek S, Baete K, Vanbilloen B, Terwinghe C, Koole M, Mottaghy FM, et al. Individualized Dosimetry-Based Activity Reduction of 90Y-DOTATOC Prevents Severe and Rapid Kidney Function Deterioration from Peptide Receptor Radionuclide Therapy. Eur J Nucl Med Mol Imaging (2014) 41(6):1141–57. doi:10.1007/s00259-013-2670-x
46. Bergsma H, Konijnenberg MW, van der Zwan WA, Kam BLR, Teunissen JJM, Kooij PP, et al. Nephrotoxicity after PRRT with 177Lu-DOTA-Octreotate. Eur J Nucl Med Mol Imaging (2016) 43(10):1802–11. doi:10.1007/s00259-016-3382-9
47. Guerriero F, Ferrari ME, Botta F, Fioroni F, Grassi E, Versari A, et al. Kidney Dosimetry in177Lu and90Y Peptide Receptor Radionuclide Therapy: Influence of Image Timing, Time-Activity Integration Method, and Risk Factors. Biomed Res Int (2013) 2013:1–12. doi:10.1155/2013/935351
48. Zaknun JJ, Bodei L, Mueller-Brand J, Pavel ME, Baum RP, Hörsch D, et al. The Joint IAEA, EANM, and SNMMI Practical Guidance on Peptide Receptor Radionuclide Therapy (PRRNT) in Neuroendocrine Tumours. Eur J Nucl Med Mol Imaging (20132013) 4140(35):584800–816. M [corrected to O’Dorisio, T M]]. doi:10.1007/s00259-012-2330-6
49. Grassi E, Fioroni F, Ferri V, Mezzenga E, Sarti MA, Paulus T, et al. Quantitative Comparison between the Commercial Software STRATOS by Philips and a Homemade Software for Voxel-Dosimetry in Radiopeptide Therapy. Physica Med (2015) 31(1):72–9. doi:10.1016/j.ejmp.2014.10.002
50. Jackson PA, Beauregard J-M, Hofman MS, Kron T, Hogg A, Hicks RJ. An Automated Voxelized Dosimetry Tool for Radionuclide Therapy Based on Serial Quantitative SPECT/CT Imaging. Med Phys (2013) 40(11):112503. doi:10.118/1.482431810.1118/1.4824318
51. Saeedzadeh E, Sarkar S, Abbaspour Tehrani-Fard A, Ay MR, Khosravi HR, Loudos G. 3D Calculation of Absorbed Dose for 131I-Targeted Radiotherapy: a Monte Carlo Study. Radiat Prot Dosimetry (2012) 150(3):298–305. doi:10.1093/rpd/ncr411
52. Dieudonné A, Hobbs RF, Bolch WE, Sgouros G, Gardin I. Fine-resolution Voxel S Values for Constructing Absorbed Dose Distributions at Variable Voxel Size. J Nucl Med (2010) 51(10):1600–7. doi:10.2967/jnumed.110.077149
53. Loudos G, Tsougos I, Boukis S, Karakatsanis N, Georgoulias P, Theodorou K, et al. A Radionuclide Dosimetry Toolkit Based on Material-specific Monte Carlo Dose Kernels. Nucl Med Commun (2009) 30(7):504–12. doi:10.1097/MNM.0b013e3283299a11
54. Dieudonné A, Hobbs RF, Lebtahi R, Maurel F, Baechler S, Wahl RL, et al. Study of the Impact of Tissue Density Heterogeneities on 3-dimensional Abdominal Dosimetry: Comparison between Dose Kernel Convolution and Direct Monte Carlo Methods. J Nucl Med (2013) 54(2):236–43. doi:10.2967/jnumed.112.105825
56. Pasciak AS, Bourgeois AC, McKinney JM, Chang TT, Osborne DR, Acuff SN, et al. Radioembolization and the Dynamic Role of 90Y PET/CT. Front Oncol (2014) 4:38. doi:10.3389/fonc.2014.00038
58. Grimes J, Celler A. Comparison of Internal Dose Estimates Obtained Using Organ-Level, Voxel S Value, and Monte Carlo Techniques. Med Phys (2014) 41(9):092501. doi:10.1118/1.4892606
59. Huizing DMV, de Wit-van der Veen BJ, Verheij M, Stokkel MPM. Dosimetry Methods and Clinical Applications in Peptide Receptor Radionuclide Therapy for Neuroendocrine Tumours: a Literature Review. EJNMMI Res (2018) 8(1):89. doi:10.1186/s13550-018-0443-z
60. Kurth J, Heuschkel M, Tonn A, Schildt A, Hakenberg OW, Krause BJ, et al. Streamlined Schemes for Dosimetry of 177Lu-Labeled PSMA Targeting Radioligands in Therapy of Prostate Cancer. Cancers. 2021;13(15):3884. Published 2021. doi:10.3390/cancers13153884
61. Mix M, Renaud T, Kind F, Nemer U, Yousetzadeh-Nowsha E, Moalosi TCG, et al. Kidney Doses in 177Lu-Based Radioligand Therapy in Prostate Cancer: Is Dose Estimation Based on Reduced Dosimetry Measurements Feasible? J Nucl Med (2022) 63(2):253–8. doi:10.2967/jnumed.121.262245
62. Hänscheid H, Lapa C, Buck AK, Lassmann M, Werner RA. Dose Mapping after Endoradiotherapy with 177Lu-DOTATATE/DOTATOC by a Single Measurement after 4 Days. J Nucl Med (2018) 59(1):75–81. doi:10.2967/jnumed.117.193706
63. Rinscheid A, Kletting P, Eiber M, Beer AJ, Glatting G. Influence of Sampling Schedules on [177Lu]Lu-PSMA Dosimetry. EJNMMI Phys. 2020;7(1):41. Published 2020. doi:10.1186/s40658-020-00311-0
64. Hofman MS, Emmett L, Sandhu S, Iravani A, Joshua AM, Goh JC, et al. [177Lu]Lu-PSMA-617 versus Cabazitaxel in Patients with Metastatic Castration-Resistant Prostate Cancer (TheraP): a Randomised, Open-Label, Phase 2 Trial. Lancet (2021) 397(10276):797–804. doi:10.1016/S0140-6736(21)00237-3
65. Sartor O, de Bono J, Chi KN, Fizazi K, Herrmann K, Rahbar K, et al. Lutetium-177-PSMA-617 for Metastatic Castration-Resistant Prostate Cancer. N Engl J Med (2021) 385(12):1091–103. doi:10.1056/NEJMoa2107322
66. Sternberg A. FDA Approves 177Lu-PSMA-617 for Pretreated PSMA+ Metastatic Castration-Resistant Prostate Cancer. [online] Cancer NetworkAvailable at: https://www.cancernetwork.com/view/fda-approves-177lu-psma-617-for-pretreated-psma-metastatic-castration-resistant-prostate-cancer (Accessed April, 2022).
67. Herrmann K, Rahbar K, Eiber M, Krause BJ, Lassmann M, Jentzen W, et al. Dosimetry of 177Lu-PSMA-617 for the Treatment of Metastatic Castration-Resistant Prostate Cancer: Results from the VISION Trial Sub-study. Jco (2022) 40(6_Suppl. l):97. doi:10.1200/jco.2022.40.6_suppl.097
68. Dhiantravan N, Emmett L, Joshua AM, Pattison DA, Francis RJ, Williams S, et al. UpFrontPSMA: a Randomized Phase 2 Study of Sequential 177 Lu‐PSMA‐617 and Docetaxel vs Docetaxel in Metastatic Hormone‐naïve Prostate Cancer (Clinical Trial Protocol). BJU Int (2021) 128(3):331–42. doi:10.1111/bju.15384
69. Morris MJ, Sartor O, Chi KN, de Bono JS, Shore ND, Crosby M, et al. 648TiP PSMAfore: A Phase III Study to Compare 177Lu-PSMA-617 Treatment with a Change in Androgen Receptor Pathway Inhibitor in Taxane-Naïve Patients with mCRPC. Ann Oncol (2021) 32:S675–S676. doi:10.1016/j.annonc.2021.08.1161
70. Tagawa ST, Sartor O, Saad F, Sakharova O, de Bono J, Feng F, et al. 647TiP PSMAddition: A Phase III Trial to Compare Treatment with 177Lu-PSMA-617 Plus Standard of Care (SOC) versus SOC Alone in Patients with Metastatic Hormone-Sensitive Prostate Cancer. Ann Oncol (2021) 32:S673–S675. doi:10.1016/j.annonc.2021.08.1160
71. Emmett L, Subramaniam S, Zhang AY, Martin AJ, Yip S, Crumbaker M, et al. ENZA-p: A Randomized Phase II Trial Using PSMA as a Therapeutic Agent and Prognostic Indicator in Men with Metastatic Castration-Resistant Prostate Cancer Treated with Enzalutamide (ANZUP 1901). Jco (2021) 39(6_Suppl. l):TPS177. doi:10.1200/jco.2021.39.6_suppl.tps177
72. Tagawa ST, Osborne JR, Hackett A, Niaz MJ, Cooley V, Christos P, et al. Preliminary Results of a Phase I/II Dose-Escalation Study of Fractionated Dose 177Lu-PSMA-617 for Progressive Metastatic Castration Resistant Prostate Cancer (mCRPC). Ann Oncol (2019) 30:v329–v330. doi:10.1093/annonc/mdz248.006
73. Paganelli G, Sarnelli A, Severi S, Sansovini M, Belli ML, Monti M, et al. Dosimetry and Safety of 177Lu PSMA-617 along with Polyglutamate Parotid Gland Protector: Preliminary Results in Metastatic Castration-Resistant Prostate Cancer Patients. Eur J Nucl Med Mol Imaging (2020) 47(13):3008–17. doi:10.1007/s00259-020-04856-1
74. Wang J, Zang J, Wang H, Liu Q, Li F, Lin Y, et al. Pretherapeutic 68Ga-PSMA-617 PET May Indicate the Dosimetry of 177Lu-PSMA-617 and 177Lu-EB-PSMA-617 in Main Organs and Tumor Lesions. Clin Nucl Med (2019) 44(6):431–8. doi:10.1097/RLU.0000000000002575
75. Kamaldeep WG, Wanage G, Sahu SK, Maletha P, Adnan A, Suman S, et al. Examining Absorbed Doses of Indigenously Developed 177Lu-PSMA-617 in Metastatic Castration-Resistant Prostate Cancer Patients at Baseline and during Course of Peptide Receptor Radioligand Therapy. Cancer Biother Radiopharm (2021) 36(3):292–304. doi:10.1089/cbr.2020.3640
76. Maffey-Steffan J, Scarpa L, Svirydenka A, Nilica B, Mair C, Buxbaum S, et al. The 68Ga/177Lu-theragnostic Concept in PSMA-Targeting of Metastatic Castration-Resistant Prostate Cancer: Impact of post-therapeutic Whole-Body Scintigraphy in the Follow-Up. Eur J Nucl Med Mol Imaging (2020) 47(3):695–712. doi:10.1007/s00259-019-04583-2
77. Scarpa L, Buxbaum S, Kendler D, Fink K, Bektic J, Gruber L, et al. The 68Ga/177Lu Theragnostic Concept in PSMA Targeting of Castration-Resistant Prostate Cancer: Correlation of SUVmax Values and Absorbed Dose Estimates. Eur J Nucl Med Mol Imaging (2017) 44(5):788–800. doi:10.1007/s00259-016-3609-9
78. Violet J, Jackson P, Ferdinandus J, Sandhu S, Akhurst T, Iravani A, et al. Dosimetry of 177Lu-PSMA-617 in Metastatic Castration-Resistant Prostate Cancer: Correlations between Pretherapeutic Imaging and Whole-Body Tumor Dosimetry with Treatment Outcomes. J Nucl Med (2019) 60(4):517–23. doi:10.2967/jnumed.118.219352
79. Zhang J, Kulkarni HR, Singh A, Schuchardt C, Niepsch K, Langbein T, et al. 177Lu-PSMA-617 Radioligand Therapy in Metastatic Castration-Resistant Prostate Cancer Patients with a Single Functioning Kidney. J Nucl Med (2019) 60(11):1579–86. doi:10.2967/jnumed.118.223149
80. Delker A, Fendler WP, Kratochwil C, Brunegraf A, Gosewisch A, Gildehaus FJ, et al. Dosimetry for 177Lu-DKFZ-PSMA-617: a New Radiopharmaceutical for the Treatment of Metastatic Prostate Cancer. Eur J Nucl Med Mol Imaging (2016) 43(1):42–51. doi:10.1007/s00259-015-3174-7
81. Kratochwil C, Giesel FL, Stefanova M, Benešová M, Bronzel M, Afshar-Oromieh A, et al. PSMA-targeted Radionuclide Therapy of Metastatic Castration-Resistant Prostate Cancer with 177Lu-Labeled PSMA-617. J Nucl Med (2016) 57(8):1170–6. doi:10.2967/jnumed.115.171397
82. Sarnelli A, Belli M, Di Iorio V, Mezzenga E, Celli M, Severi S, et al. Dosimetry of 177Lu-PSMA-617 after Mannitol Infusion and Glutamate Tablet Administration: Preliminary Results of EUDRACT/RSO 2016-002732-32 IRST Protocol. Molecules (2019) 24(3):621. doi:10.3390/molecules24030621
83. Peters SMB, Privé BM, de Bakker M, de Lange F, Jentzen W, Eek A, et al. Intra-therapeutic Dosimetry of [177Lu]Lu-PSMA-617 in Low-Volume Hormone-Sensitive Metastatic Prostate Cancer Patients and Correlation with Treatment Outcome. Eur J Nucl Med Mol Imaging (2022) 49(2):460–9. doi:10.1007/s00259-021-05471-4
84. Völter F, Mittlmeier L, Gosewisch A, Brosch-Lenz J, Gildehaus FJ, Zacherl MJ, et al. Correlation of an Index-Lesion-Based SPECT Dosimetry Method with Mean Tumor Dose and Clinical Outcome after 177Lu-PSMA-617 Radioligand Therapy. Diagnostics (2021) 11(3):428. doi:10.3390/diagnostics11030428
85. Yadav MP, Ballal S, Tripathi M, Damle NA, Sahoo RK, Seth A, et al. Post-therapeutic Dosimetry of 177Lu-DKFZ-PSMA-617 in the Treatment of Patients with Metastatic Castration-Resistant Prostate Cancer. Nucl Med Commun (2017) 38(1):91–8. doi:10.1097/MNM.0000000000000606
86. Fendler WP, Reinhardt S, Ilhan H, Delker A, Böning G, Gildehaus FJ, et al. Preliminary Experience with Dosimetry, Response and Patient Reported Outcome after 177Lu-PSMA-617 Therapy for Metastatic Castration-Resistant Prostate Cancer. Oncotarget (2017) 8(2):3581–90. doi:10.18632/oncotarget.12240
87. Rosar F, Schön N, Bohnenberger H, Bartholomä M, Stemler T, Maus S, et al. Comparison of Different Methods for post-therapeutic Dosimetry in [177Lu]Lu-PSMA-617 Radioligand Therapy. EJNMMI Phys (2021) 8(1):40. doi:10.1186/s40658-021-00385-4
88. Barna S, Haug AR, Hartenbach M, Rasul S, Grubmüller B, Kramer G, et al. Dose Calculations and Dose-Effect Relationships in 177Lu-PSMA I&T Radionuclide Therapy for Metastatic Castration-Resistant Prostate Cancer. Clin Nucl Med (2020) 45(9):661–7. doi:10.1097/RLU.0000000000003157
89. Okamoto S, Thieme A, Allmann J, D’Alessandria C, Maurer T, Retz M, et al. Radiation Dosimetry for 177Lu-PSMA I&T in Metastatic Castration-Resistant Prostate Cancer: Absorbed Dose in Normal Organs and Tumor Lesions. J Nucl Med (2017) 58(3):445–50. doi:10.2967/jnumed.116.178483
90. Herrmann K, Bluemel C, Weineisen M, Schottelius M, Wester H-J, Czernin J, et al. Biodistribution and Radiation Dosimetry for a Probe Targeting Prostate-specific Membrane Antigen for Imaging and Therapy. J Nucl Med (2015) 56(6):855–61. doi:10.2967/jnumed.115.156133
91. Baum R, Kulkarni H, Volkmer B, Bohuslavizki K, Schuchardt C, Klette I, et al. Theranostik des metastasierten Prostatakarzinoms mittels Lu-177 PSMA-Liganden in Kombination mit Ga-68 PSMA PET/CT. Nuklearmediziner (2015) 38:145–52. doi:10.1055/s-0035-1549991
92. Gosewisch A, Delker A, Tattenberg S, Ilhan H, Todica A, Brosch J, et al. Patient-specific Image-Based Bone Marrow Dosimetry in Lu-177-[DOTA0,Tyr3]-Octreotate and Lu-177-DKFZ-PSMA-617 Therapy: Investigation of a New Hybrid Image Approach. EJNMMI Res (2018) 8(1):76. doi:10.1186/s13550-018-0427-z
93. Kabasakal L, AbuQbeitah M, Aygün A, Yeyin N, Ocak M, Demirci E, et al. Pre-therapeutic Dosimetry of normal Organs and Tissues of 177Lu-PSMA-617 Prostate-specific Membrane Antigen (PSMA) Inhibitor in Patients with Castration-Resistant Prostate Cancer. Eur J Nucl Med Mol Imaging (2015) 42(13):1976–83. doi:10.1007/s00259-015-3125-3
94. Kabasakal L, Toklu T, Yeyin N, Demirci E, Abuqbeitah M, Ocak M, et al. Lu-177-PSMA-617 Prostate-specific Membrane Antigen Inhibitor Therapy in Patients with Castration-Resistant Prostate Cancer: Stability, Bio-Distribution and Dosimetry. Mirt (2017) 26(2):62–8. doi:10.4274/mirt.08760
95. Hohberg M, Eschner W, Schmidt M, Dietlein M, Kobe C, Fischer T, et al. Lacrimal Glands May Represent Organs at Risk for Radionuclide Therapy of Prostate Cancer with [177Lu]DKFZ-PSMA-617. Mol Imaging Biol (2016) 18(3):437–45. doi:10.1007/s11307-016-0942-0
96. Emami B, Lyman J, Brown A, Cola L, Goitein M, Munzenrider JE, et al. Tolerance of normal Tissue to Therapeutic Irradiation. Int J Radiat Oncology*Biology*Physics (1991) 21(1):109–22. doi:10.1016/0360-3016(91)90171-y
97. Gensheimer MF, Liao JJ, Garden AS, Laramore GE, Parvathaneni U. Submandibular Gland-Sparing Radiation Therapy for Locally Advanced Oropharyngeal Squamous Cell Carcinoma: Patterns of Failure and Xerostomia Outcomes. Radiat Oncol (2014) 9:255. doi:10.1186/s13014-014-0255-x
98. Hey J, Setz J, Gerlach R, Janich M, Hildebrandt G, Vordermark D, et al. Parotid Gland-Recovery after Radiotherapy in the Head and Neck Region - 36 Months Follow-Up of a Prospective Clinical Study. Radiat Oncol (2011) 6:125. doi:10.1186/1748-717X-6-125
99. Özkan A, Uçar B, Seymen H, Yildiz Yarar Y, Falay FO, Demirkol MO. Posttherapeutic Critical Organ Dosimetry of Extensive 177Lu-PSMA Inhibitor Therapy with Metastatic Castration-Resistant Prostate Cancer. Clin Nucl Med (2020) 45(4):288–91. doi:10.1097/RLU.0000000000002942
100. Gosewisch A, Ilhan H, Tattenberg S, Mairani A, Parodi K, Brosch J, et al. 3D Monte Carlo Bone Marrow Dosimetry for Lu-177-PSMA Therapy with Guidance of Non-invasive 3D Localization of Active Bone Marrow via Tc-99m-Anti-Granulocyte Antibody SPECT/CT. EJNMMI Res (2019) 9(1):76. doi:10.1186/s13550-019-0548-z
101. Privé BM, Peters SMB, Muselaers CHJ, van Oort IM, Janssen MJR, Sedelaar JPM, et al. Lutetium-177-PSMA-617 in Low-Volume Hormone-Sensitive Metastatic Prostate Cancer: A Prospective Pilot Study. Clin Cancer Res (2021) 27(13):3595–601. doi:10.1158/1078-0432.CCR-20-4298
102. Peters SMB, Hofferber R, Privé BM, de Bakker M, Gotthardt M, Janssen M, et al. [68Ga]Ga-PSMA-11 PET Imaging as a Predictor for Absorbed Doses in Organs at Risk and Small Lesions in [177Lu]Lu-PSMA-617 Treatment. Eur J Nucl Med Mol Imaging (2022) 49(4):1101–12. doi:10.1007/s00259-021-05538-2
103. Peters SMB, Prive BM, Gotthardt M, de Lange F, Nagarajah J, Konijnenberg MW, et al. Dosimetry of Lu-177-PSMA-617 in Low-Volume Hormone Sensitive Metastatic Prostate Cancer and Correlation with Treatment Outcome. Eur J Nucl Med Mol Imaging (2021).
104. Gear JI, Cox MG, Gustafsson J, Gleisner KS, Murray I, Glatting G, et al. EANM Practical Guidance on Uncertainty Analysis for Molecular Radiotherapy Absorbed Dose Calculations. Eur J Nucl Med Mol Imaging (2018) 45(13):2456–74. doi:10.1007/s00259-018-4136-7
105. Sachpekidis C, Kopka K, Eder M, Hadaschik BA, Freitag MT, Pan L, et al. 68Ga-PSMA-11 Dynamic PET/CT Imaging in Primary Prostate Cancer. Clin Nucl Med (2016) 41(11):e473–e479. doi:10.1097/RLU.0000000000001349
106. Miller DR, Ingersoll MA, Teply BA, Lin MF. Combination Treatment Options for Castration-Resistant Prostate Cancer. In: SRJ Bott, and KL Ng, editors. Prostate Cancer. Brisbane (AU): Exon PublicationsMay 27, 2021).
107. Belli ML, Sarnelli A, Mezzenga E, Cesarini F, Caroli P, Di Iorio V, et al. Targeted Alpha Therapy in mCRPC (Metastatic Castration-Resistant Prostate Cancer) Patients: Predictive Dosimetry and Toxicity Modeling of 225Ac-PSMA (Prostate-specific Membrane Antigen). Front Oncol (2020) 10:531660. doi:10.3389/fonc.2020.531660
108. Ahenkorah S, Cassells I, Deroose CM, Cardinaels T, Burgoyne AR, Bormans G, et al. Bismuth-213 for Targeted Radionuclide Therapy: From Atom to Bedside. Pharmaceutics (2021) 13(5):599. doi:10.3390/pharmaceutics13050599
109. Rathke H, Flechsig P, Mier W, Bronzel M, Mavriopoulou E, Hohenfellner M, et al. Dosimetry Estimate and Initial Clinical Experience with 90Y-PSMA-617. J Nucl Med (2019) 60(6):806–11. doi:10.2967/jnumed.118.218917
110. Kratochwil C, Bruchertseifer F, Rathke H, Bronzel M, Apostolidis C, Weichert W, et al. Targeted α-Therapy of Metastatic Castration-Resistant Prostate Cancer with 225Ac-PSMA-617: Dosimetry Estimate and Empiric Dose Finding. J Nucl Med (2017) 58(10):1624–31. doi:10.2967/jnumed.117.191395
111. Parker C, Heidenreich A, Nilsson S, Shore N. Current Approaches to Incorporation of Radium-223 in Clinical Practice. Prostate Cancer Prostatic Dis (2018) 21:37–47. doi:10.1038/s41391-017-0020-y
112. Dandapani SV, Wong J, Twardowski P. Review of Radium-223 and Metastatic Castration-Sensitive Prostate Cancer. Cancer Biother Radiopharm (2020) 35:490–6. doi:10.1089/cbr.2019.3493
113. Etchebehere E, Brito AE, Rezaee A, Langsteger W, Beheshti M. Therapy Assessment of Bone Metastatic Disease in the Era of 223radium. Eur J Nucl Med Mol Imaging (2017) 44:84–96. doi:10.1007/s00259-017-3734-0
114. Poty S, Francesconi LC, McDevitt MR, Morris MJ, Lewis JS. α-Emitters for Radiotherapy: From Basic Radiochemistry to Clinical Studies-Part 1. J Nucl Med (2018) 59(6):878–84. doi:10.2967/jnumed.116.186338
115. Garashchenko BL, Korsakova VA, Yakovlev RY. Radiopharmaceuticals Based on Alpha Emitters: Preparation, Properties, and Application. Phys Atom Nuclei (2018) 81:1515–25. doi:10.1134/S1063778818100071
116. Guerra Liberal FDC, O'Sullivan JM, McMahon SJ, Prise KM. Targeted Alpha Therapy: Current Clinical Applications. Cancer Biother Radiopharm (2020) 35:404–17. doi:10.1089/cbr.2020.3576
117. Ferrier MG, Radchenko V. An Appendix of Radionuclides Used in Targeted Alpha Therapy. J Med Imaging Radiat Sci (2019) 50:S58–S65. doi:10.1016/j.jmir.2019.06.051
118.National Nuclear Data Center. National Nuclear Data Center. Upton, NY: Brookhaven National Laboratory. Available online: https://www.nndc.bnl.gov/ (Accessed April, 2022).
119. Bayer . Study to Evaluate the Safety, Tolerability,Pharmacokinetics, and Antitumor Activity of a Thorium-227 Labeled Antibody-Chelator Conjugate Alone and in Combination with Darolutamide, in Patients with Metastatic Castration Resistant Prostate Cancer - Full Text View - ClinicalTrials.Gov. Available at: https://clinicaltrials.gov/ct2/show/NCT03724747?term=227th&cond=Prostate+Cancer&draw=2&rank=1. (Accessed April 21, 2022).
120. Hurt JD. Safety and Tolerability of 212Pb-DOTAM-GRPR1 212Pb-DOTAM-GRPR1 in Adult Subjects with Recurrent or Metastatic GRPR-Expressing Tumors - Full Text View - ClinicalTrials.Gov. Available at: https://clinicaltrials.gov/ct2/show/NCT05283330?term=212Pb&cond=Prostate+Cancer&draw=2&rank=1. (Accessed April 21, 2022).
121. Tagawa S. 225Ac-J591 Plus 177Lu-PSMA-I&T for mCRPC - Full Text View - ClinicalTrials.Gov. Available at: https://clinicaltrials.gov/ct2/show/NCT04886986?term=225Ac&cond=Prostate+Cancer&draw=2&rank=1. (Accessed April 21, 2022).
122. Tagawa S. Phase I Trial of 225Ac-J591 in Patients with mCRPC - Full Text View - ClinicalTrials.Gov. Available at: https://clinicaltrials.gov/ct2/show/NCT03276572?term=225Ac&cond=Prostate+Cancer&draw=2&rank=2. (Accessed April 21, 2022).
123. Tagawa S. Fractionated and Multiple Dose 225Ac-J591 for Progressive mCRPC - Full Text View - ClinicalTrials.Gov. Available at: https://clinicaltrials.gov/ct2/show/NCT04506567?term=225Ac&cond=Prostate+Cancer&draw=2&rank=3. (Accessed April 21, 2022).
124. Tagawa S. Maximizing Responses to Anti-PD1 Immunotherapy with PSMA-Targeted Alpha Therapy in mCRPC – Full Text View – ClinicalTrials.Gov. Available at: https://clinicaltrials.gov/ct2/show/NCT04946370?term=225Ac&cond=Prostate+Cancer&draw=2&rank=4. (Accessed April 21, 2022).
125.Janssen Research & Development, LLC. A Study of JNJ-69086420, an Actinium-225-Labeled Antibody Targeting Human Kallikrein-2 (hK2) for Advanced Prostate Cancer – Full Text View – ClinicalTrials.Gov. Available at: https://clinicaltrials.gov/ct2/show/NCT04644770?term=225Ac&cond=Prostate+Cancer&draw=2&rank=8. (Accessed April 21, 2022).
126.Novartis Pharmaceuticals. Study of 225Ac-PSMA-617 in Men with PSMA-Positive Prostate Cancer – Full Text View – ClinicalTrials.Gov. Available at: https://clinicaltrials.gov/ct2/show/NCT04597411?term=225Ac&cond=Prostate+Cancer&draw=2&rank=9. (Accessed April 21, 2022).
127. Ezziddin S. Prospectiv3 Registry of Targeted RadionucLide TherapY in Patients with mCRPC (REALITY Study) – Full Text View – ClinicalTrials.Gov. Available at: https://clinicaltrials.gov/ct2/show/NCT04833517?term=225Ac&cond=Prostate+Cancer&draw=2&rank=10. (Accessed April 21, 2022).
128. Sartor O, Sharma D. Radium and Other Alpha Emitters in Prostate Cancer. Transl Androl Urol (2018) 7(3):436–44. doi:10.21037/tau.2018.02.07
129. Liu F, Jang H, Kijowski R, Zhao G, Bradshaw T, McMillan AB. A Deep Learning Approach for 18F-FDG PET Attenuation Correction. EJNMMI Phys (2018) 5(1):24. doi:10.1186/s40658-018-0225-8
130. Häggström I, Schmidtlein CR, Campanella G, Fuchs TJ. DeepPET: A Deep Encoder-Decoder Network for Directly Solving the PET Image Reconstruction Inverse Problem. Med Image Anal (2019) 54:253–62. doi:10.1016/j.media.2019.03.013
131. Reader AJ, Corda G, Mehranian A, Costa-Luis Cd., Ellis S, Schnabel JA. Deep Learning for PET Image Reconstruction. IEEE Trans Radiat Plasma Med Sci (2021) 5(1):1–25. doi:10.1109/TRPMS.2020.3014786
132. Hashimoto F, Ote K, Ota R, Hasegawa T. A Feasibility Study on 3D Interaction Position Estimation Using Deep Neural Network in Cherenkov-Based Detector: A Monte Carlo Simulation Study. Biomed Phys Eng Express (2019) 5(3):035001. doi:10.1088/2057-1976/ab098e
133. Nguyen D, Long T, Jia X, Lu W, Gu X, Iqbal Z, et al. A Feasibility Study for Predicting Optimal Radiation Therapy Dose Distributions of Prostate Cancer Patients from Patient Anatomy Using Deep Learning. Sci Rep (2019) 9:1076. doi:10.1038/s41598-018-37741-x
134. Shen C, Nguyen D, Chen L, Gonzalez Y, McBeth R, Qin N, et al. Operating a Treatment Planning System Using a Deep‐reinforcement Learning‐based Virtual Treatment Planner for Prostate Cancer Intensity‐modulated Radiation Therapy Treatment Planning. Med Phys (2020) 47(6):2329–36. doi:10.1002/mp.14114
135. Zeleznik R, Weiss J, Taron J, Guthier C, Bitterman DS, Hancox C, et al. Deep-learning System to Improve the Quality and Efficiency of Volumetric Heart Segmentation for Breast Cancer. Npj Digit Med (2021) 4:43. doi:10.1038/s41746-021-00416-5
136. Arabi H, Zaidi H. Applications of Artificial Intelligence and Deep Learning in Molecular Imaging and Radiotherapy. Eur J Hybrid Imaging (2020) 4(1). doi:10.1186/s41824-020-00086-8
137. Zhao W, Shen L, Han B, Yang Y, Cheng K, Toesca DAS, et al. Markerless Pancreatic Tumor Target Localization Enabled by Deep Learning. Int J Radiat Oncology*Biology*Physics (2019) 105(2):432–9. doi:10.1016/j.ijrobp.2019.05.071
138. Huang B, Chen Z, Wu P-M, Ye Y, Feng S-T, Wong C-YO, et al. Fully Automated Delineation of Gross Tumor Volume for Head and Neck Cancer on PET-CT Using Deep Learning: A Dual-Center Study. Contrast Media Mol Imaging (2018) 2018:1–12. Published. doi:10.1155/2018/8923028
139. Zhao X, Li L, Lu W, Tan S. Tumor Co-segmentation in PET/CT Using Multi-Modality Fully Convolutional Neural Network. Phys Med Biol. 2018;64(1):015011. Published 2018. doi:10.1088/1361-6560/aaf44b
140. Wang M, Zhang Q, Lam S, Cai J, Yang R. A Review on Application of Deep Learning Algorithms in External Beam Radiotherapy Automated Treatment Planning. Front Oncol (2020) 10:580919. doi:10.3389/fonc.2020.580919
141. Akhavanallaf A, Shiri I, Arabi H, Zaidi H. Whole-body Voxel-Based Internal Dosimetry Using Deep Learning. Eur J Nucl Med Mol Imaging (2021) 48(3):670–82. doi:10.1007/s00259-020-05013-4
142. Smith JA, Abhari RE, Hussain Z, Heneghan C, Collins GS, Carr AJ. Industry Ties and Evidence in Public Comments on the FDA Framework for Modifications to Artificial Intelligence/machine Learning-Based Medical Devices: a Cross Sectional Study. BMJ Open (2020) 10(10):e039969. doi:10.1136/bmjopen-2020-039969
143. Colling R, Pitman H, Oien K, Rajpoot N, Macklin P, Bachtiar V, et al. Artificial Intelligence in Digital Pathology: a Roadmap to Routine Use in Clinical Practice. J Pathol (2019) 249(2):143–50. doi:10.1002/path.5310
144. Acs B, Rantalainen M, Hartman J. Artificial Intelligence as the Next Step towards Precision Pathology. J Intern Med (2020) 288(1):62–81. doi:10.1111/joim.13030
145. Götz TI, Schmidkonz C, Chen S, Al-Baddai S, Kuwert T, Lang EW. A Deep Learning Approach to Radiation Dose Estimation. Phys Med Biol (2020) 65(3):035007. doi:10.1088/1361-6560/ab65dc
Keywords: dosimetry, 177 lu, PSMA, prostate, cancer
Citation: Alsadi R, Djekidel M, Bouhali O and Doherty JO (2022) Towards Routine Clinical Use of Dosimetry in [177Lu]Lu-PSMA Prostate Cancer Radionuclide Therapy: Current Efforts and Future Perspectives. Front. Phys. 10:940677. doi: 10.3389/fphy.2022.940677
Received: 10 May 2022; Accepted: 13 June 2022;
Published: 14 July 2022.
Edited by:
Stephane Chauvie, Azienda Sanitaria Ospedaliera S.Croce e Carle Cuneo, ItalyReviewed by:
Jens Kurth, Rostock University Medical Center Rostock, GermanyPrice Jackson, Peter MacCallum Cancer Centre, Australia
Copyright © 2022 Alsadi, Djekidel, Bouhali and Doherty. This is an open-access article distributed under the terms of the Creative Commons Attribution License (CC BY). The use, distribution or reproduction in other forums is permitted, provided the original author(s) and the copyright owner(s) are credited and that the original publication in this journal is cited, in accordance with accepted academic practice. No use, distribution or reproduction is permitted which does not comply with these terms.
*Correspondence: Jim O’ Doherty, amltLm9kb2hlcnR5QGdtYWlsLmNvbQ==