- 1Department of Mechanical Engineering, Orthopaedic Bioengineering Research Laboratory, Colorado State University, Fort Collins, CO, United States
- 2Department of Clinical Sciences, Colorado State University, Fort Collins, CO, United States
- 3School of Biomedical Engineering, Colorado State University, Fort Collins, CO, United States
- 4Saddle Rock Institute, Aurora, CO, United States
The temporomandibular joint (TMJ) is a bilateral ginglymoarthroidal joint containing a fibrocartilaginous disc which distributes compressive stress and reduces friction on the articulating surfaces of the joint. Initially, conservative treatments can address disorders of the TMJ, but surgical procedures such as discectomy may be employed if dysfunction persists. Unfortunately, discectomy increases friction and alters the mechanical behavior of the TMJ when the disc is not replaced. An ideal replacement for the TMJ disc would restore healthy function of the joint and prevent further degeneration. Rigorous evaluation of materials is necessary to ensure the safety and efficacy of novel implants. Poly(vinyl) alcohol (PVA) hydrogels have been suggested as a good candidate for artificial cartilage replacement in other applications and are promising for replacing the TMJ disc due to their biocompatibility, low friction, and biomimetic levels of hydration. The objective of this study was to mechanically characterize hydrogel formulations of 15, 20, and 25% PVA and to compare them with the ovine TMJ disc to investigate the appropriateness of PVA hydrogels for TMJ disc replacement in a translational model. Experimental evaluations were conducted in indentation, unconfined compression, friction, and uniaxial tension. There were no statistically significant differences in the coefficient of friction between the PVA hydrogels and ovine TMJ disc. No statistically significant differences were found between at least one PVA hydrogel group and the TMJ disc for the indentation properties or the unconfined compression properties at low stretch magnitudes. The 20% PVA and 25% PVA hydrogels exhibited significantly higher failure stretch as compared to the ovine TMJ disc, and they were not statistically different in ultimate tensile strength from the TMJ disc in the mediolateral direction. However, the ovine TMJ disc tensile elastic moduli were 630 times larger in the anteroposterior direction and 53 times larger in the mediolateral direction than the stiffest PVA hydrogel. Ultimately, the 25% PVA hydrogel was the best candidate for TMJ disc replacement, demonstrating the most similarity to the ovine TMJ disc compressive properties; however, tensile reinforcement of the hydrogels would be necessary to approach the tensile modulus of the native TMJ disc.
Introduction
The temporomandibular joint (TMJ) disc is a biconcave, fibrocartilaginous disc positioned between the mandibular condyle and glenoid fossa [1]. The TMJ disc separates an upper and lower joint capsule and acts to distribute shear and compressive forces throughout the range of jaw movements [2]. Musculoskeletal conditions of the TMJ complex are classified by a wide range of symptoms including functional limitations and orofacial pain, which may be myogenous or arthrogenous [3–5]. Pain in the TMJ is prevalent, effecting approximately 10% of the population over the age of 18 [6]. Conservative, non-invasive methods to reduce pain are implemented initially, but in cases where joint degradation continues to progress, a surgical procedure may be required [7]. TMJ disc excision is a common surgical technique and can be effective in reducing pain [8]. However, TMJ disc excision does not address the underlying causes of joint degradation and inherently increases friction on the condylar and fossa surfaces [9], which may lead to crepitation and morphological changes [10, 11]. To address this problem, numerous interpositional graft materials have been investigated with the objective of restoring joint homeostasis after disc removal. Artificial materials such as silastic and Proplast-Teflon were introduced and subsequently removed from the market after reports of foreign body giant cell reactions and material fragmentation [12]. Autogenous muscle and skin-fat grafts have also been implemented with some positive results, but these procedures have not been shown to consistently outperform discectomy [13]. There has also been a recent push for tissue engineering approaches to TMJ disc replacement; the goal of these treatments is to alleviate pain, improve function, and to slow or reverse degenerative changes in the TMJ [14–16]. Unfortunately, low vascularity of native TMJ tissues and insufficient construct mechanical strength complicate the application of current tissue engineering solutions [17]. Developing a robust alloplastic replacement strategy could provide a reliable solution for patients requiring TMJ disc excision while circumventing the biological barriers to clinical implementation of tissue engineering solutions, and may also prolong patient function with a native joint delaying or preventing the need for total alloplastic joint replacement.
Polyvinyl alcohol (PVA) hydrogels have been identified as a promising candidate for musculoskeletal soft tissue replacement. These hydrogels can be formulated to be mechanically similar to a range of cartilaginous tissues, exhibit low friction, and have biomimetic water content [18–21]. PVA hydrogels and associated wear particles exhibit excellent biocompatibility and hemocompatibility [22], and have been shown to exhibit a lower tissue response than ultra-high molecular weight polyethylene [23]. Among other applications, PVA hydrogels have been investigated as a replacement for intervertebral discs [24], meniscus [25], vasculature [26], and other load bearing joint replacements [27–29]. However, to our knowledge, PVA hydrogels have only recently been investigated for replacement of the TMJ disc [30].
The goal of this study was to directly compare the mechanical behavior of PVA hydrogels with known weight concentrations to freshly harvested ovine TMJ discs in order to determine the appropriateness of PVA hydrogels for TMJ disc replacement. Ovine tissue was utilized to determine the suitability of the materials for use in preclinical studies. PVA hydrogels with polymer weight concentrations of 15, 20, and 25% were evaluated in indentation, unconfined compression, friction, and uniaxial tension experiments. Ovine TMJ discs were also experimentally tested in unconfined compression and friction. PVA hydrogel results in indentation and uniaxial tension were compared to previously published ovine TMJ disc results following identical testing protocols [31].
Materials and methods
PVA hydrogel synthesis
PVA (molecular weight 89,000-98,000, 99+% hydrolyzed, Sigma-Aldrich, St. Louis, MO) was mixed with deionized (DI) water in a sealed beaker and heated to 80°C for at least two hours while stirred. PVA hydrogels were synthesized with 15, 20%, and 25% initial polymer weight concentrations at mixing. Sheets of PVA hydrogel were molded between two glass microscope slides with a thickness of 2 mm for friction testing specimens and 1 mm for compressive, indentation, and tensile testing specimens. All groups were subjected to 6 freeze-thaw cycles (12 h at −18°C, 3 h at 20°C). After cycling, all gels were removed from their molds and submerged in phosphate buffered saline (PBS) for at least 24 h prior to testing. Molded PVA sheets were cut to the appropriate geometries (Figure 1) immediately before testing.
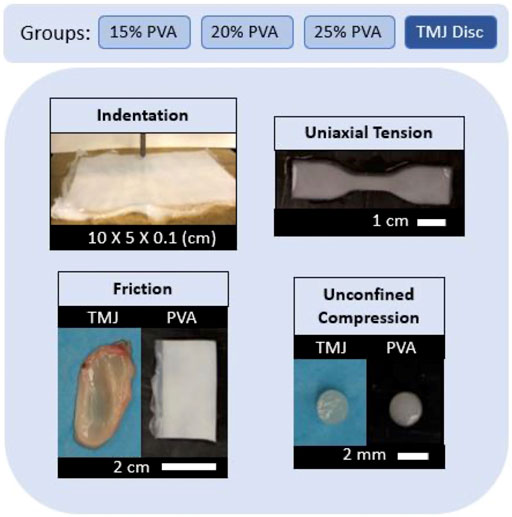
FIGURE 1. Summary of experimental groups and mechanical evaluations with representative sample images.
Temporomandibular joint discs
Fresh TMJ discs were harvested from skeletally mature female Columbian Rambouillet sheep euthanized for unrelated studies (Colorado State University Institutional Animal Care and Use Committee protocol KR104). A total of eight left and eight right TMJ discs were excised and designated for unconfined compression or friction testing.
Percent polymer fraction
Excess PVA hydrogel cuttings from each concentration were stored in PBS. To calculate percent polymer fraction (%PF), PVA hydrogel samples from each group were removed from PBS, blotted to remove excess surface fluid, and weighed. These samples were then placed in a vacuum chamber for 7 days, which was sufficient time for the sample weight to reach equilibrium. The %PF for each sample was calculated according to Eq. 1, where Ww is the wet weight and WD is the dehydrated weight.
The %PF calculated for the 15% PVA, 20% PVA, and 25% PVA hydrogel groups were 17.3, 23.8, and 26.1%, respectively.
Indentation
Indentation stress-relaxation testing was performed on hydrogel samples using a servo-hydraulic material testing machine (Model 370.02, MTS Systems Corporation, Eden Prairie, MN) with a calibrated 8.9 N capacity load cell (LSB200, Futek, Irvine, CA). Twenty indentations were performed for each PVA hydrogel concentration across two molded PVA hydrogel sheets. A stainless-steel indenter tip with a radius of 0.40 mm was used for all indentation. A preload of 0.5 mN was used to identify the surface of the sample, followed by a 30 s hold period before indentation. All samples were indented to a depth of 0.2 mm at a rate of 0.2 mm/s and held for a relaxation period of 100 s before tip retraction, following the protocol for previous ovine TMJ disc testing [31]. Force and displacement data were collected throughout the test. The elastic moduli (E) were estimated following Hertzian contact mechanics [32],
where, υ = 0.44 is the Poisson’s ratio of the sample [33], υi = 0.29 is the Poisson’s ratio of the indenter tip, Ei = 210 GPa is the Young’s modulus of the indenter tip, F is the measured force, and d is the indenter tip displacement. The instantaneous modulus was calculated using a least-squares fit of Eq. 2 to the loading portion of the force-displacement data. Force-displacement data from the end of the 100s hold period was used to calculate the equilibrium modulus using Eq. 2. Any indentations for which the root mean squared error percentage for the least squares fit was greater than 5% were discarded. This eliminated indents where the surface of the sample was incorrectly identified, or where there were sudden compliance changes uncharacteristic of elastic materials [34].
Unconfined compression
Unconfined compression tests were performed on cylindrical samples of the ovine TMJ discs and PVA hydrogels for direct comparison. Ten (n = 5 right, n = 5 left) ovine TMJ discs and eight samples from each PVA hydrogel concentration were designated for use in unconfined compression experiments. A cylindrical sample was removed from the central portion of each TMJ disc and from PVA hydrogels using a 2 mm diameter biopsy punch. TMJ disc and PVA hydrogel samples were mounted in a hydraulic testing system between two parallel compressive platens (Model 858, MTS, Eden Prairie, MN) with a 220 N load cell (661.09 B-21, MTS, Eden Prairie, MN). Each sample was loaded to a minimum of 60% strain at a strain rate of 0.005 s−1, and paired force-displacement data were collected. Stress was calculated as the axial force divided by the sample cross sectional area, and stretch was calculated as the displacement of the upper platen divided by the initial height of the sample. Incompressibility was assumed due to the high water content of the TMJ discs and PVA hydrogels [35]. The experimental stress-stretch data between λ = 1.00 and λ = 0.56 were fitted to a 2-term Yeoh model:
where ψ is the strain energy density, C1 and C2 are material constants, and I1 is the first invariant of the right Cauchy-Green deformation tensor,
Here, λ1, λ2, and λ3, are the principal stretches. For unconfined compression, λ1 = λ, λ2 = λ3 = λ−1/2, and σ22 = σ33 = 0. Therefore, the axial Cauchy stress (σ11) can be related to the axial stretch (λ) according to the following equation:
The values of C1 and C2 were determined using a least-squares fit of Eq. 5 to the stress-stretch data. In addition, the tangent moduli at stretch values from λ = 1.00 to λ = 0.96 and λ = 0.60 to λ = 0.54 were calculated using a localized linear regression. Stretch ranges were chosen to represent an initial tangent modulus (λ = 1.00 to λ = 0.96), and tangent modulus at a physiologically relevant maximum strain based on finite element modeling of the human TMJ while clenching (λ = 0.60 to λ = 0.54) [36].
Friction
The coefficient of friction (COF) of PVA hydrogels and TMJ discs were tested against a bearing surface of ovine TMJ fossa cartilage. Six (n = 3 right, n = 3 left) ovine TMJ discs were designated for friction testing. Eight PVA hydrogel samples from each group were cut to an approximate size of 40 mm by 20 mm. A cylindrical osteochondral plug with radius 3.2 mm was extracted from an ovine TMJ fossa and kept hydrated with PBS. The same osteochondral specimen was used for all tests on all materials in order to keep the testing conditions consistent and comparable. This plug was mounted in a torsional hydraulic testing machine with an offset of 90 mm from the center of rotation (Figure 2). A mass was applied above the cylindrical plug to induce a uniform pressure of 100 kPa throughout the test [37].
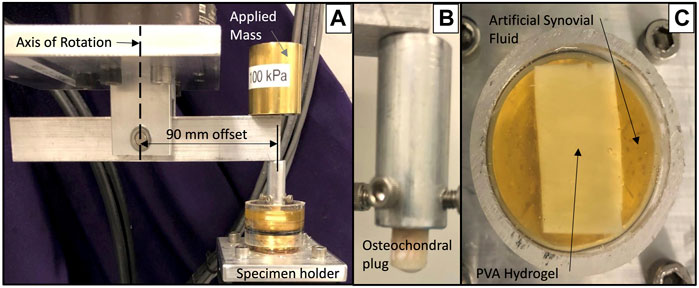
FIGURE 2. Friction testing setup showing (A) offset loading apparatus, (B) mounted osteochondral plug and (C) PVA hydrogel sample immersed in artificial synovial fluid.
All samples were fixed to a brass substrate via cyanoacrylate adhesive and immersed in an artificial synovial fluid consisting of 30 g/L newborn calf serum diluted with PBS, 2 g/L sodium azide, 3 g/L EDTA, and 3 g/L hyaluronic acid [38]. The cylindrical cartilage plug was drawn back and forth across the surface five times each at rates of 0.4 mm/s, 4.0 mm/s, and 40 mm/s [39, 40]. The induced normal and transverse forces were measured using an axial and torsional load cell (Model 661.19H-03, MTS, Eden Prairie, MN). The COF was calculated as the absolute value of the transverse force, divided by the normal force. The average COF was calculated over the central 90% of the total stroke length for the five cycles at each speed.
Tensile testing
PVA hydrogel tensile specimens were tested following the protocol previously described for ovine TMJ disc testing [31]. PVA hydrogel specimens were cut to a dog-bone shape with width 3.48 ± 0.14 mm and thickness 1.44 ± 0.10 mm (mean ± standard deviation). The initial distance between grips was measured after a preload of 0.05 N was applied. Graphite powder was applied to the top surface of each sample to enhance the texture for measuring strain via digital image correlation. Each sample was preconditioned for 10 cycles to 8% strain at a displacement at a rate of 8% strain/second. Once preconditioned, samples were loaded to failure at a rate of 0.01 s−1. The initial grip-to-grip distance was used to define the strain rate used for each test. A MATLAB-based digital image correlation algorithm was used to calculate the average stretch of a central region of interest of each sample up to a stretch of λ = 1.4. The stress-stretch data were used to determine a tensile elastic modulus using a linear fit of the data between λ = 1.1 to λ = 1.2, matching the range used for TMJ disc samples. The failure stretch for each sample was calculated by measuring fiducial markers using ImageJ (Version 1.52s, National Institute of Health, Bethesda, MD) [41]. PVA ultimate strength was defined as the maximum stress observed for each sample, and PVA sample toughness was calculated as the peak first Piola-Kirchhoff stress multiplied by the failure stretch, divided by two due to the highly linear stress-stretch behavior.
Statistical analysis
A one-way ANOVA with post hoc Tukey test was used to compare the PVA hydrogel and TMJ disc tensile moduli, failure stretch, ultimate tensile strength, and toughness. A logarithmic transformation was applied to all of the tensile data in order to satisfy the assumptions of normality and equal variance. For the remaining parameters, data collected from the TMJ discs were non-normal and exhibited higher variation than the PVA hydrogels. Thus, PVA and TMJ disc results were compared using a Kruskal–Wallis test, with post hoc Wilcoxon comparisons. Any comparisons with p < 0.05 were reported as significant, and all p-values are provided. Previously published indentation data collected regionally on the ovine TMJ disc [31] were grouped for comparison with the PVA hydrogels. Separate statistical comparisons were made of the instantaneous indentation moduli, equilibrium indentation moduli, the average coefficients of friction for each speed, as well as the tangent moduli and fitting coefficients for unconfined compression.
Results
Indentation
The instantaneous and equilibrium moduli of the PVA hydrogels increased with increasing polymer concentration. The equilibrium moduli of all PVA hydrogels and pooled TMJ disc data were smaller than their respective instantaneous moduli (Figure 3). The ratio of average equilibrium moduli to average instantaneous moduli was 0.19, 0.55, 0.75, and 0.82 for the TMJ disc, 15% PVA, 20% PVA, and 25% PVA, respectively. No statistical difference was found between the TMJ disc moduli and the 15% PVA hydrogel moduli, or between the instantaneous moduli of the TMJ disc and 20% PVA. There was a statistically significant difference between the 25% PVA and the TMJ disc for both the instantaneous and equilibrium moduli. However, the instantaneous moduli of all PVA hydrogel concentrations were within the range of the highly variable TMJ disc moduli (Figure 3).
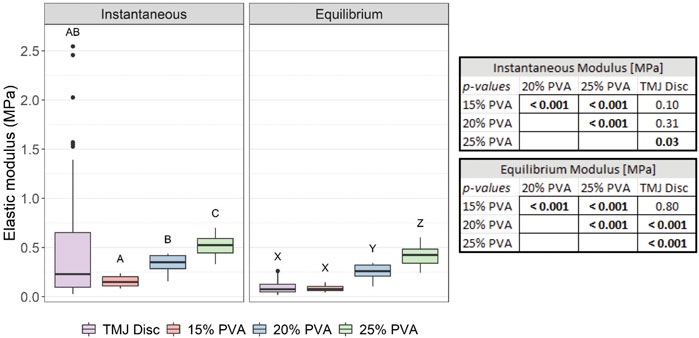
FIGURE 3. Box-and-whisker plots of the calculated instantaneous and equilibrium elastic moduli from indentation of the PVA hydrogels, and the pooled TMJ data from Labus et al [29]. Groups that do not share a letter were significantly different. All p-values for post hoc Wilcoxon rank-sum comparisons are provided in the associated table (right).
Unconfined compression
The stress-stretch behavior appeared similar between the PVA hydrogels and the TMJ disc samples under small deformations, however, the TMJ disc data exhibited a greater degree of nonlinearity under larger deformations (Figure 4). For small deformations (stretch values approaching 1.00), the TMJ disc tangent modulus was not statistically different from the 20% PVA and 25% PVA. However, for larger compressive stretches, the TMJ disc tangent modulus was significantly larger than all PVA hydrogels (Figure 5). The mean tangent moduli and fitted C1 parameter increased with increasing PVA concentration (Figure 5). The average percent root mean squared error (%RMSE) for the 2-term Yeoh model fits of the unconfined compression data was 0.60% for the PVA hydrogels and 3.5% for the TMJ disc. The values of C1 and C2 for the TMJ disc were significantly higher than those of the PVA hydrogels (p-values provided in Figure 5).
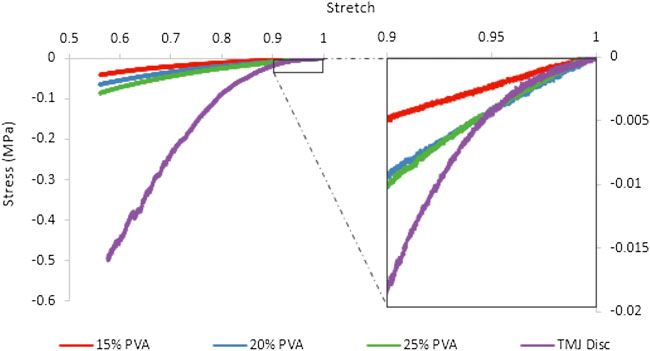
FIGURE 4. Representative stress-stretch data for the PVA hydrogels and TMJ discs tested in unconfined compression.
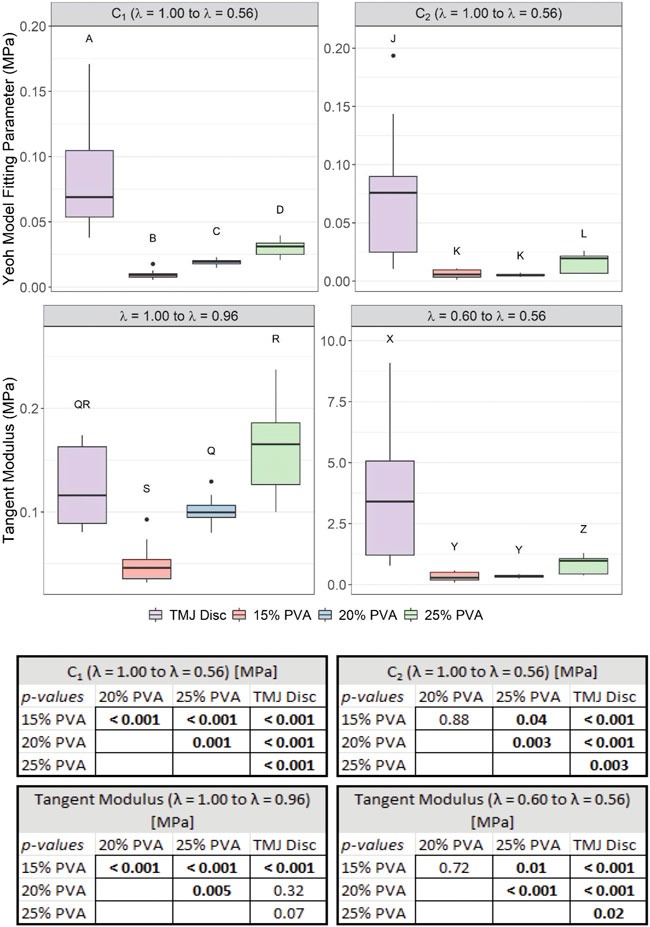
FIGURE 5. Box-and-whisker plots of the calculated 2-term Yeoh model fitting parameters (C1, C2) from A = 1.00 to A = 0.56, and the tangent moduli from A = 1.00 to A = 0.96 and from A = 0.60 to A = 0.56. Groups that do not share a letter were significantly different. All p-values for post hoc Wilcoxon rank-sum comparisons are provided in the associated table (bottom).
Friction
No statistically significant difference in COF was found between the TMJ disc and any of the PVA hydrogel groups at any of the testing speeds. There were no differences in COF between the three PVA hydrogel concentrations at the 0.4 mm/s speed, but the COF of the 15% PVA hydrogel was significantly lower than the 20 and 25% PVA hydrogels at 4 mm/s and 40 mm/s speeds. The COF for the TMJ disc was nearly identical across testing speeds (Figure 6).
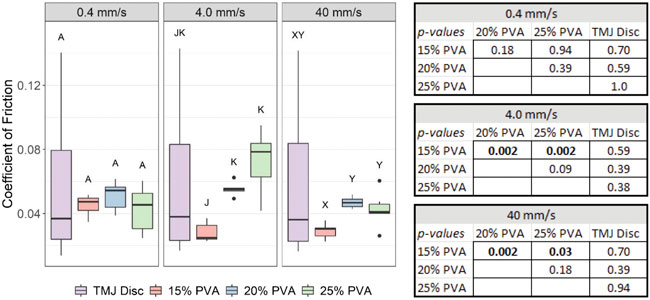
FIGURE 6. Box-and-whisker plots of the average COF at each speed from friction evaluation of the PVA hydrogels and ovine TMJ discs. Groups that do not share a letter were significantly different. All p–values from post hoc Wilcoxon rank - sum comparisons are provided in the associated table (right).
Tensile testing
The average elastic modulus, failure stretch, ultimate tensile strength, and toughness increased with increasing PVA concentration (Figure 7). The elastic modulus, ultimate tensile strength, and toughness were all greater in the anteroposterior (AP) direction (predominant fiber direction) than in the mediolateral (ML) direction (transverse to the fibers) for the TMJ disc. The mean elastic modulus of the TMJ disc tissue in the AP and ML directions was 630 and 53 times greater, respectively, than the mean elastic modulus of the 25% PVA hydrogel. The ultimate tensile strength of the TMJ disc in the ML direction was not significantly different from the 20% or 25% PVA hydrogels (Figure 7). In the AP direction, the TMJ disc ultimate strength was significantly greater than all PVA hydrogel materials. Failure stretch of the 20% PVA and 25% PVA was significantly greater than the TMJ disc regardless of direction. The toughness of the 20% PVA and 25% PVA was not statistically different from the AP TMJ disc.
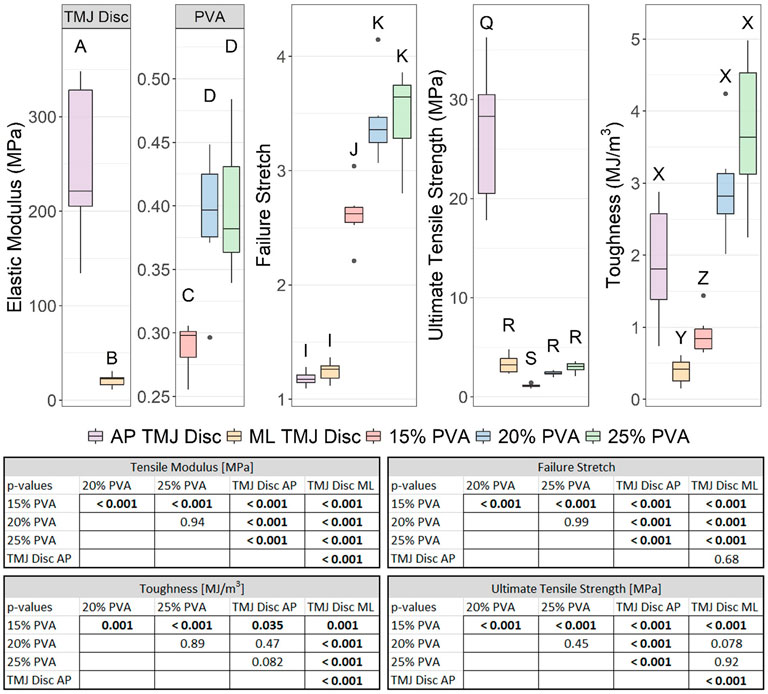
FIGURE 7. Box-and-whisker plots of the elastic modulus, failure stretch, ultimate tensile strength, and failure stretch from the tensile evaluation of the PVA hydrogels and ovine TMJ discs [29]. Groups that do not share a letter were significantly different. All p–values for post hoc Tukey’s HSD tests are provided in the associated table (bottom).
Discussion
These experiments provide a broad analysis of the mechanical behavior of PVA hydrogels with a direct comparison to the ovine TMJ disc. Overall, trends in these data show increasing elastic moduli measured for indentation, tension, and unconfined compression with increasing %PF. The PVA hydrogels exhibited similar properties to the TMJ disc in indentation, friction, unconfined compression at small deformations, and tensile strength. However, the TMJ disc exhibited a greater tensile elastic modulus and compressive elastic modulus at large deformations compared to the PVA hydrogels.
The instantaneous and equilibrium moduli of the 15% PVA group were not statistically different from the TMJ disc, demonstrating that the 15% PVA most closely matches the behavior of the TMJ discs in indentation. However, the ratio of instantaneous to equilibrium modulus for the PVA hydrogels was 2.9 (15% PVA) to 4.3 (25% PVA) times greater than the ratio for the TMJ disc tissue (0.19). This emphasizes the high degree of relaxation seen in TMJ disc tissue in comparison to the PVA hydrogels. Ultimately, none of the PVA hydrogels matched the degree of relaxation of the TMJ disc, but all groups fell within the range of observed instantaneous moduli of the TMJ disc. Activities such as chewing or talking induce larger dynamic stresses on the TMJ disc as compared to more static, distributed forces induced by clenching [42]. The dynamic mechanical behavior is primarily governed by the instantaneous properties compared to the equilibrium properties of the tissues. Therefore, the instantaneous modulus may be a more critical consideration in a TMJ disc replacement material than the equilibrium modulus.
The unconfined compression results reveal a disproportionate increase in tangent modulus with increasing stretch magnitude for the TMJ disc compared to the PVA hydrogels. At lower levels of stretch, the tangent modulus of the TMJ disc was not significantly different from the 20 and 25% PVA hydrogel. However, at higher (λ = 0.60 to λ = 0.56) compressive stretch magnitudes, the tangent modulus of the TMJ disc was significantly higher than the PVA hydrogels. These findings are explained by the highly non-linear behavior of the TMJ disc tissue which has been documented across species [43]. In addition, the tangent moduli for unconfined compression in the TMJ disc at higher values of stretch also exceeded the indentation moduli. This behavior is likely due to the significant collagen fiber component in the TMJ disc, which can contribute to the stiffness to a greater degree in macro-scale unconfined compression compared to micro-scale indentation [44]. Thus, we have demonstrated that the PVA hydrogels do not fully mimic the non-linear behavior of the TMJ disc. These results suggest that transversely-aligned tensile reinforcement may be required to increase the compressive stiffness of PVA hydrogel materials at high strains in order to mimic the compressive behavior of the TMJ disc.
Other research groups have demonstrated similar COF values for PVA-based hydrogels under similar loading conditions [19]. However, hydration, applied pressure, translation speed, hydrogel concentration, hydrogel molecular weight, and contacting material all influence the apparent friction coefficient [45, 46]. These factors underscore our motivation for comparative experiments to allow for direct assessment between the PVA hydrogels and the ovine TMJ disc. Our experimental conditions approximate the in-vivo environment by including ovine TMJ cartilage as the contacting material and lubrication with artificial synovial fluid. At moderate (4 mm/s) and high (40 mm/s) speeds, the 15% PVA hydrogel exhibited significantly lower friction than the 20 and 25% PVA hydrogels, suggesting that lower polymer concentrations may exhibit better frictional properties at higher speeds. However, this trend may not be consistent for different applied loads. Experiments on PVA/polyvinylpyrrolidone hydrogels have shown a decrease in COF with increasing polymer concentration at lower applied loads, with a reversal in trend at higher applied loads [47]. However, the friction experiments in the current study showed no statistical difference in COF between the ovine TMJ disc and PVA hydrogels regardless of translation speed or %PF.
The most disparate material property between the PVA hydrogels and TMJ disc was the tensile modulus. Previous tensile characterization of unmodified PVA hydrogels with the same molecular weight and degree of hydrolysis report tensile moduli of 0.23 ± 0.02 MPa [48] and 7.6 MPa [49]. This broad range can be attributed to differences in synthesis methods and PVA concentration. In our evaluation, the tensile modulus of the 25% PVA group (0.40 MPa) was 53–630 times smaller than that of the ovine TMJ disc. These results suggest that mimicking the tensile stiffness of the ovine TMJ disc would require reinforcement of the PVA hydrogels. Significant research efforts towards reinforcing PVA hydrogel networks have been investigated [21]. Introduction of additional polymers into the PVA hydrogel networks has shown increased tensile moduli of approximately 200 MPa [50], and one group measured a tensile modulus of 258.1 ± 40.1 MPa for a PVA hydrogel reinforced with melt-blown ultra-high molecular weight polyethylene and polypropylene fibers [48].
The PVA hydrogels demonstrated excellent toughness, failure stretch, and ultimate tensile strength properties. The 20% PVA and 25% PVA exhibited significantly higher failure stretch compared to the TMJ disc, and these polymers matched the ultimate tensile strength of the TMJ disc in the ML direction. Also, the toughness of the 20% PVA and 25% PVA was not statistically different from the AP TMJ disc. These results, paired with low coefficients of friction, suggest that PVA hydrogels represent a promising candidate for TMJ disc replacement.
Ovine TMJ disc tissue was chosen for comparison in order to assess potential implant materials in the context of preclinical large animal studies. Pigs, goats, and sheep have all been identified as good preclinical options, each with advantages and disadvantages [51, 52]. Although sheep have different diets and joint kinematics compared to humans, the overall size, anatomy, and surgical access in sheep is similar to that of humans. This, paired with their relatively low cost make sheep a suitable choice for studies focused on surgical procedure and implant development [53].
Our study was limited in scope, focusing on one PVA molecular weight and degree of hydrolysis. The mechanical behavior of PVA hydrogels is highly tunable, influenced by a multitude of synthesis conditions and polymer characteristics [54]. PVA hydrogel properties may also be optimized through other strategies, including reinforcement from additional polymers [55, 56] or directional freezing [49]. However, this study does provide a baseline for comparison between one PVA hydrogel formulation and the ovine TMJ disc.
Conclusion
Of the concentrations tested, we suggest that the 25% PVA group (%PF 26.05%) was the most promising candidate for TMJ disc replacement. The 25% PVA hydrogel was most similar to the TMJ discs in compressive modulus and ultimate tensile strength, and outperformed the TMJ discs in failure stretch. The indentation results demonstrated better agreement between the 15% PVA and the TMJ disc, but the instantaneous modulus of the 25% PVA hydrogel group was within the range of the TMJ disc. Additionally, the COF of 25% PVA was not significantly different from the ovine TMJ disc at any speed.
The purpose of these experiments was to provide a broad mechanical comparison between PVA hydrogels and the ovine TMJ disc to inform further development of a mechanically biomimetic PVA hydrogel implant. The 25% PVA hydrogel was most similar to the ovine TMJ disc in unconfined compression and tension. In addition, the PVA hydrogels showed promise for TMJ disc replacement due to their biomimetic frictional values and potential for further tunability. However, the disparity between the tensile modulus and high-stretch compressive modulus of the 25% PVA hydrogel and ovine TMJ disc suggests a need for tensile reinforcement of the PVA hydrogel structure. Overall, these experiments support further development of PVA hydrogels for TMJ disc replacement in an ovine preclinical model.
Data availability statement
The raw data supporting the conclusion of this article will be made available by the authors, without undue reservation.
Ethics statement
The animal study was reviewed and approved by Colorado State University Institutional Animal Care and Use Committee protocol KR104.
Author contributions
Conceptualization (JK, CP, JR, RD, and KL), data collection (JK), data analysis (JK), interpretation of results (JK, CP, JR, RD, and KL). All authors reviewed literature, participated in drafting, and critically revising the manuscript.
Conflict of interest
The authors declare that the research was conducted in the absence of any commercial or financial relationships that could be construed as a potential conflict of interest.
Publisher’s note
All claims expressed in this article are solely those of the authors and do not necessarily represent those of their affiliated organizations, or those of the publisher, the editors and the reviewers. Any product that may be evaluated in this article, or claim that may be made by its manufacturer, is not guaranteed or endorsed by the publisher.
References
1. Alomar X, Medrano J, Cabratosa J, Clavero J, Lorente M, Serra I, et al. Anatomy of the temporomandibular joint. Semin Ultrasound CT MRI (2007) 28(3):170–83. doi:10.1053/j.sult.2007.02.002
2. Nickel JC, Iwasaki LR, Gonzalez YM, Gallo LM, Yao H. Mechanobehavior and ontogenesis of the temporomandibular joint. J Dent Res (2018) 97(11):1185–92. doi:10.1177/0022034518786469
3. Molinari F, Manicone PF, Raffaelli L, Raffaelli R, Pirronti T, Bonomo L. Temporomandibular joint soft-tissue pathology, I: Disc abnormalities. Semin Ultrasound CT MRI (2007) 28(3):192–204. doi:10.1053/j.sult.2007.02.004
4. Laskin DM. Temporomandibular disorders: A term whose time has passed. J Oral Maxill Surg (2020) 78(4):496–7. doi:10.1016/j.joms.2019.11.038
5. Bielajew BJ, Donahue RP, Espinosa MG, Arzi B, Wang D, Hatcher DC, et al. Knee orthopedics as a template for the temporomandibular joint. Cel Rep Med (2021) 2(5):100241. doi:10.1016/j.xcrm.2021.100241
6. LeResche L. Epidemiology of temporomandibular disorders: Implications for the investigation of etiologic factors. Crit Rev Oral Biol Med (1997) 8(3):291–305. doi:10.1177/10454411970080030401
7. Dimitroulis G. Management of temporomandibular joint disorders: A surgeon's perspective. Aust Dent J (2018) 63(1):S79–S90. doi:10.1111/adj.12593
8. Eriksson L, Westesson PL. Discectomy as an effective treatment for painful temporomandibular joint internal derangement: A 5-year clinical and radiographic follow-up. J Oral Maxillofac Surg (2001) 59:750. doi:10.1053/joms.2001.24288
9. Tanaka E, Dalla-Bona DA, Iwabe T, Kawai N, Yamano E, van Eijden T, et al. The effect of removal of the disc on the friction in the temporomandibular joint. J Oral Maxillofac Surg (2006) 64:1221–4. doi:10.1016/j.joms.2006.04.017
10. McKenna SJ. Discectomy for the treatment of internal derangements of the temporomandibular joint. J Oral Maxillofac Surg (2001) 59(9):1051–6. doi:10.1053/joms.2001.26682
11. Hagandora CK, Almarza AJ. TMJ disc removal: Comparison between pre-clinical studies and clinical findings. J Dent Res (2012) 91(8):745–52. doi:10.1177/0022034512453324
12. De Meurechy N, Mommaerts MY. Alloplastic temporomandibular joint replacement systems: A systematic review of their history. Int J Oral Maxillofac Surg (2018) 47(6):743–54. doi:10.1016/j.ijom.2018.01.014
13. Dimitroulis G. A critical review of interpositional grafts following temporomandibular joint discectomy with an overview of the dermis-fat graft. Int J Oral Maxillofac Surg (2010) 40(6):561–8. doi:10.1016/j.ijom.2010.11.020
14. Allen KD, Athanasiou KA, Tissue engineering of the TMJ disc: A review, Tissue Eng (2006) 12:1183. doi:10.1089/ten.2006.12.1183
15. Aryaei A, Vapniarsky N, Hu JC, Athanasiou KA. Recent tissue engineering advances for the treatment of temporomandibular joint disorders. Curr Osteoporos Rep (2016) 14:269–79. doi:10.1007/s11914-016-0327-y
16. Vapniarsky N, Huwe LW, Arzi B, Houghton MK, Wong ME, Wilson JW, et al. Tissue engineering toward temporomandibular joint disc regeneration. Sci Transl Med (2018) 10:eaaq1802. doi:10.1126/scitranslmed.aaq1802
17. Donahue RP, Hu JC, Athanasiou KA. Remaining hurdles for tissue-engineering the temporomandibular joint disc. Trends Mol Med (2019) 25(3):241–56. doi:10.1016/j.molmed.2018.12.007
18. Borges C, Colaço R, Paula Serro A. Poly(vinyl alcohol)-based hydrogels for joint prosthesis. Ann Med (2019) 51:105. sup1. doi:10.1080/07853890.2018.1562711
19. Sardinha VM, Lima LL, Belangero WD, Zavaglia CA, Bavaresco VP, Gomes JR. Tribological characterization of polyvinyl alcohol hydrogel as substitute of articular cartilage. Wear (2013) 301(1):218–25. doi:10.1016/j.wear.2012.11.054
20. Paradossi G, Cavalieri F, Chiessi E, Spagnoli C, Cowman MK. Poly(vinyl alcohol) as versatile biomaterial for potential biomedical applications. J Mater Sci Mater Med (2003) 14(8):687–91. doi:10.1023/A:1024907615244
21. Kumar A, Han SS. PVA-based hydrogels for tissue engineering: A review. Int J Polymeric Mater Polymeric Biomater (2017) 66(4):159–82. doi:10.1080/00914037.2016.1190930
22. Noguchi T, Yamamuro T, Oka M, Kumar P, Kotoura Y, Hyonyt SH, et al. Poly(vinyl alcohol) hydrogel as an artificial articular cartilage: Evaluation of biocompatibility. J Appl Biomater (1991) 2(2):101–7. doi:10.1002/jab.770020205
23. Alexandre N, Ribeiro J, Gartner A, Pereira T, Amorim I, Fragoso J, et al. Biocompatibility and hemocompatibility of polyvinyl alcohol hydrogel used for vascular grafting--in vitro and in vivo studies. J Biomed Mater Res A (2014) 102(12):4262–75. doi:10.1002/jbm.a.35098
24. Wang BH, Campbell G. Formulations of polyvinyl alcohol cryogel that mimic the biomechanical properties of soft tissues in the natural lumbar intervertebral disc. Spine (Phila Pa 1976) (2009) 34:2745. doi:10.1097/BRS.0b013e3181b4abf5
25. Kobayashi M, Chang YS, Oka M. A two year in vivo study of polyvinyl alcohol-hydrogel (PVA-H) artificial meniscus. Biomaterials (2005) 26(16):3243–8. doi:10.1016/j.biomaterials.2004.08.028
26. Alexandre N, Amorim I, Caseiro AR, Pereira T, Alvites R, Rema A, et al. Long term performance evaluation of small-diameter vascular grafts based on polyvinyl alcohol hydrogel and dextran and MSCs-based therapies using the ovine pre-clinical animal model. Int J Pharm X (2017) 523(2):515–30. doi:10.1016/j.ijpharm.2017.02.043
27. Suciu AN, Iwatsubo T, Matsuda M, Nishino T. A study upon durability of the artificial knee joint with PVA hydrogel cartilage. JSME Int J Ser C (2004) 47(1):199–208. doi:10.1299/jsmec.47.199
28. Kobayashi M, Hyu HS. Development and evaluation of polyvinyl alcohol-hydrogels as an artificial atrticular cartilage for orthopedic implants. Materials (Basel) (2010) 3(4):2753–71. (in eng). doi:10.3390/ma3042753
29. Baker MI, Walsh Sp Fau - Schwartz Z, Fau - Boyan BDSZ, Boyan BD. A review of polyvinyl alcohol and its uses in cartilage and orthopedic applications. J Biomed Mater Res B Appl Biomater (2006) 100:1451. doi:10.1002/jbm.b.32694
30. Jiang N, Yang Y, Zhang L, Jiang Y, Wang M, Zhu S. 3D-Printed polycaprolactone reinforced hydrogel as an artificial TMJ disc. J Dent Res (2021) 100:839. doi:10.1177/00220345211000629
31. Labus KM, Kuiper JP, Rawlinson J, Puttlitz CM. Mechanical characterization and viscoelastic model of the ovine temporomandibular joint Disc in indentation, uniaxial tension, and biaxial tension. J Mech Behav Biomed Mater (2021) 116:104300. doi:10.1016/j.jmbbm.2020.104300
32. Wu C-E, Lin K-H, Juang J-Y. Hertzian load–displacement relation holds for spherical indentation on soft elastic solids undergoing large deformations. Tribology Int (2016) 97:71–6. doi:10.1016/j.triboint.2015.12.034
33. Lee J-H, Lee SS, Chang JD, Thompson MS, Kang DJ, Park S, et al. A novel method for the accurate evaluation of Poisson’s ratio of soft polymer materials. Scientific World J (2013) 2013:1–7. doi:10.1155/2013/930798
34. Oyen ML, Cook RF. A practical guide for analysis of nanoindentation data. Mech Behav Biomed Mater (2009) 2:396. doi:10.1016/j.jmbbm.2008.10.002
35. Ortún-Terrazas J, Cegoñino J, Pérez del Palomar A. Biomechanical impact of the porous-fibrous tissue behaviour in the temporomandibular joint movements. An in silico approach. J Mech Behav Biomed Mater (2021) 120:104542. doi:10.1016/j.jmbbm.2021.104542
36. Beek M, Koolstra Jh Fau - van Ruijven LJ, van Ruijven Lj Fau - van Eijden TM, van Eijden TM. Three-dimensional finite element analysis of the human temporomandibular joint disc. J Biomech (2000) 33:307. doi:10.1016/s0021-9290(99)00168-2
37. Donzelli PS, Gallo Lm Fau - Spilker RL, Spilker Rl Fau - Palla S, Palla S. Biphasic finite element simulation of the TMJ disc from in vivo kinematic and geometric measurements. J Biomech (2004) 37:1787. doi:10.1016/j.jbiomech.2004.01.029
38. Bortel EL, Charbonnier B, Heuberger R. Development of a synthetic synovial fluid for tribological testing. Lubricants (2015) 3(4):664–86. doi:10.3390/lubricants3040664
39. Gallo LM, Nickel Jc Fau - Iwasaki LR, Iwasaki Lr Fau - Palla S, Palla S. Stress-field translation in the healthy human temporomandibular joint. J Dent Res (2000) 79:1740. doi:10.1177/00220345000790100201
40. Middendorf JM, Albahrani SA, Bonassar LJ. Stribeck curve analysis of temporomandibular joint condylar cartilage and disc. J Biomech Eng (2019) 141:12. doi:10.1115/1.4045283
41. Schneider CA, Rasband WS, Eliceiri KW. NIH image to ImageJ: 25 years of image analysis. Nat Methods (2012) 9(7):671–5. doi:10.1038/nmeth.2089
42. Barrientos E, Pelayo F, Tanaka E, Lamela-Rey MJ, Fernandez-Canteli A. Dynamic and stress relaxation properties of the whole porcine temporomandibular joint disc under compression. J Mech Behav Biomed Mater (2015) 57:109–15. doi:10.1016/j.jmbbm.2015.12.003
43. Lamela MJ, Prado Y, Fernández P, Fernández-Canteli A, Tanaka E. Non-linear viscoelastic model for behaviour characterization of temporomandibular joint discs. Exp Mech (2011) 51(8):1435–40. doi:10.1007/s11340-011-9465-4
44. Fazaeli S, Ghazanfari S, Everts V, Smit TH, Koolstra JH. The contribution of collagen fibers to the mechanical compressive properties of the temporomandibular joint disc. Osteoarthritis Cartilage (2016) 24(7):1292–301. doi:10.1016/j.joca.2016.01.138
45. Wu G, Wang C, Zhang W. The factors of speeds and loads on the tribological properties of PVA-H/HA composites. J Appl Polym Sci (2007) 106(6):3908–14. doi:10.1002/app.27031
46. Ma R, Xiong D, Miao F, Zhang J, Peng Y. Novel PVP/PVA hydrogels for articular cartilage replacement. Mater Sci Eng C (2009) 29(6):1979–83. doi:10.1016/j.msec.2009.03.010
47. Shi Y, Xiong D. Microstructure and friction properties of PVA/PVP hydrogels for articular cartilage repair as function of polymerization degree and polymer concentration. Wear (2013) 305(1):280–5. doi:10.1016/j.wear.2012.12.020
48. Holloway JL, Lowman AM, Palmese GR. Mechanical evaluation of poly(vinyl alcohol)-based fibrous composites as biomaterials for meniscal tissue replacement. Acta Biomater (2010) 6(12):4716–24. doi:10.1016/j.actbio.2010.06.025
49. Duan S, Wu S, Hua M, Wu D, Yan Y, Zhu X, et al. Tendon-inspired anti-freezing tough gels. iScience (2021) 24(9):102989. doi:10.1016/j.isci.2021.102989
50. Yang F, Zhao J, Koshut WJ, Watt J, Riboh JC, Gall K, et al. A synthetic hydrogel composite with the mechanical behavior and durability of cartilage. Adv Funct Mater (2020) 30(36):2003451. doi:10.1002/adfm.202003451
51. Almarza AJ, Brown BN, Arzi B, Angelo DF, Chung W, Badylak SF, et al. Preclinical animal models for temporomandibular joint tissue engineering. Tissue Eng B: Rev (2018) 24(3):171–8. doi:10.1089/ten.TEB.2017.0341
52. Kalpakci KN, Willard Vp Fau - Wong ME, Wong Me Fau - Athanasiou KA, Athanasiou KA. An interspecies comparison of the temporomandibular joint disc. J Dent Res (2011) 90:193. doi:10.1177/0022034510381501
53. Bosanquet AG, Goss AN, "The sheep as a model for temporomandibular joint surgery," Int J Oral Maxill Surg. 16. 600. 1987 doi:10.1016/S0901-5027(87)80113-3
54. Wu S, Hua M, Alsaid Y, Du Y, Ma Y, Zhao Y, et al. Poly(vinyl alcohol) hydrogels with broad-range tunable mechanical properties via the hofmeister effect. Adv Mater (2021) 33(11):2007829. doi:10.1002/adma.202007829
55. Tan BK, Ching YC, Poh SC, Abdullah LC, Gan SN. A review of natural fiber reinforced poly(vinyl alcohol) based composites: Application and opportunity. Polymers (2015) 7(11):2205–22. [Online]. Available: https://www.mdpi.com/2073-4360/7/11/1509. doi:10.3390/polym7111509
Keywords: temporomandibular joint disc, polyvinyl alcohol, ovine, biomechanics, alloplastic
Citation: Kuiper JP, Puttlitz CM, Rawlinson JE, Dobbs R and Labus KM (2022) A mechanical evaluation of polyvinyl alcohol hydrogels for temporomandibular joint disc replacement. Front. Phys. 10:928579. doi: 10.3389/fphy.2022.928579
Received: 25 April 2022; Accepted: 04 August 2022;
Published: 25 August 2022.
Edited by:
Jeff Scifert, Cook Medical Inc., United StatesReviewed by:
Boaz Arzi, University of California, Davis, United StatesAlessandro Tel, University of Udine, Italy
Copyright © 2022 Kuiper, Puttlitz, Rawlinson, Dobbs and Labus. This is an open-access article distributed under the terms of the Creative Commons Attribution License (CC BY). The use, distribution or reproduction in other forums is permitted, provided the original author(s) and the copyright owner(s) are credited and that the original publication in this journal is cited, in accordance with accepted academic practice. No use, distribution or reproduction is permitted which does not comply with these terms.
*Correspondence: Kevin M. Labus, a2V2aW4ubGFidXNAY29sb3N0YXRlLmVkdQ==