- 1Department of Chemistry, National Institute of Technology Durgapur, Durgapur, India
- 2Department of Chemistry and Biochemistry, San Diego State University, San Diego, CA, United States
Recent studies postulate that the presence of polycyclic aromatic hydrocarbons (PAHs) in the interstellar medium (ISM) could have been formed through resonance-stabilized arylcarbene intermediates. However, identifying most of these reactive intermediates is very challenging experimentally due to their metastability and other experimental constrains. Thus, computational studies that cover the thermodynamic versus kinetic stability of various possible structures would be beneficial for successfully identifying new molecules either in the laboratory and/or in the ISM. In this paper, more than four hundred C11H8 carbene isomers have been theoretically investigated employing density functional theory (DFT). Hybrid density functionals B3LYP and ωB97XD with 6-311 + G (d,p) basis set have been used for singlet electronic states, whereas, triplet spin states were optimized at the same level using an unrestricted Hartree-Fock wavefunction. Although the skeletal structures of C11H8 can be categorized into monocyclic, bicyclic, tricyclic, tetracyclic and acyclic isomers, bicyclic carbenes have shown better stability due to the presence of resonance stabilized azulenyl/naphthyl rings. In this category, some isomers (1-, 2-, 5- and 6-azulenylcarbenes and 1- and 2-naphthylcarbenes) have also been detected recently in the laboratory and simple aromatic carbenes such as cyclopropenylidene and its homologues are detected in the ISM. Thus, we have systematically investigated the energetic and spectroscopic properties of resonance stabilized 5-, 6-, 7- and 8-membered ring containing bicyclic isomers of C11H8 and the fingerprint regions of the infrared spectrum for each class of these bicyclic compounds.
Introduction
Carbonaceous organic molecules especially polycyclic aromatic hydrocarbons (PAHs) are ubiquitously the key components in the astrochemical evolution of the interstellar medium (ISM) [1–4]. Understanding the chemical evolution of the Universe through the formation of the PAHs and their abundance in the astrochemical environment are one of the important aspects of astrochemistry [5, 6]. The detection and isolation of the building blocks of PAHs in the laboratory by creating “space-like” environment is experimentally a daunting task. However, the knowledge of the thermodynamic and spectroscopic properties of possible reactive intermediates and building blocks through accurate quantum mechanical calculations will be very helpful to understand the formation of PAHs and their identification in ISM [7–13].
Laboratory experiments with small organic molecules with neutral/radical reactive intermediates [14–16] have been studied by several research groups using advanced techniques including selected ion flow tubes (SIFT) and Ion Cyclotron Resonance (ICR) mass spectrometry, ion trapping using external electric and magnetic fields etc [17–20]. These experiments lead to the understanding of possible growth mechanism of the PAHs and soot formation in ISM. The cyclic and linear molecules of C5Hn configuration have been found to be the possible routes of benzene formation [21–23]. For example, isoprene (C5H8) and the methyl-substituted 1,3-butadiene can form phenyl radical with very low activation energy [24]. Earlier, in 2001, Zhang et al. had proposed the formation of bicyclic PAHs from phenyl radicals reacting with cis-3-penten-1-yne [25]. Later, formation of naphthalene had been identified as a result of bimolecular recombination of para-tolyl and phenyl radicals using crossed molecular beam experiments and higher order PAHs like anthracene and phenanthrene had been prepared in low temperature environments [26–28].
Very recently, a large number of cyano-derivatives of cyclic organic molecules have been discovered in the dark molecular cloud of TMC-1 using precise rotational spectroscopic measurement methods [29–32]. Apart from this, the recent discovery of two of the carbene molecules of C5H2, ethynylcyclopropenylidene and pentatetraenylidene [33–36] have opened up the possibility of detecting more organic molecules in the dark, low-pressure and low-temperature regions in space. Recent theoretical investigations on C5H2 isomers suggest both acyclic and cyclic carbenes and diradicals as potential target molecules for laboratory spectroscopy [37, 38]. It is expected that reactive intermediates, e.g., free radicals and carbene molecules might have played an important role in the formation of such molecules [39]. Carbene molecules such as cyclopropenylidene (c-C3H2) [40], propadienylidene (C3H2) [35], butatrienylidene (C4H2) [41], hexapentaenylidene (C6H2) [42] etc. had been detected as early as late 20th century in the ISM. Other studies have suggested that the isomeric conversion of several radical PAHs can undergo via linear and cyclic carbene formation at the high temperature environment [43, 44].
PAHs are considered to be responsible for the unidentified infrared emission bands (UIRs) associated with a wide range of interstellar species such as free radicals, ions, carbenes, and neutral molecules from near-UV to the near IR region [45–47]. These UIRs generally show characteristic spectral signatures in the 3–14 μm wavelength range and the variation of the observed IR spectra can be correlated with the structural type of PAHs and population of the hydrocarbons from difference sources [48]. Rastogi et al. also successfully modeled the PAHs into different groups based on their ring sizes, number of carbon atoms present and found significant spectral changes associated with the structural changes of the PAHs [49]. Nevertheless, it is not very clear if these bands originate from any particular species, or a group of structurally similar molecules that are responsible for such emission bands. Therefore, we intended to identify molecules, which are structurally similar and studied their thermodynamic and spectroscopic properties for future identification either in laboratory or in the ISM.
In this study, carbene and allene isomers of molecular formula C11H8 have been theoretically investigated using Density Functional Theory (DFT) calculations. Although several low-lying neutral isomers of C11H8 have been investigated recently [50], such an account for reactive carbene intermediates are still missing in the literature. Closely related to C11H8 isomers, Maier and co-workers have detected C11H9+ and C11H9• isomers in neon matrices [51]. 2-naphthylmethylium and benzotropylium cations were detected and their electronic absorption spectra were recorded. After photobleaching of matrices containing C11H9+, the corresponding neutral molecules were also characterized. Using ultraviolet population transfer spectroscopy, Zwier and co-workers have studied the excited state dynamics of C10H8 and probed the E/Z isomerization of 1-phenyl-1-buten-3-yne [52]. Moreover, the electronic spectroscopy of the indene-motif PAHs has been studied by Maier and co-workers [53].
As shown in Figure 1, various types of skeletal structures (monocyclo, bicyclo, tricyclo, tetracyclo, and linear) are possible, but, considering the importance of naphthyl, azulenyl, and indenyl group for the formation of PAHs in the ISM, we have focused on nearly four hundred bicyclic carbene and allene isomers of C11H8. Most of these molecular structures have been identified through chemical intuition and/or by using a Python code for Aggregation and Reaction (PyAR), which is based on a tabu-search algorithm, developed and maintained by Anoop and co-workers [54].
Theoretical Methodology
A bottom-up approach is adopted in the current implementation of the PyAR software and thus the constituent atoms will be added sequentially in building isomers of the molecular formula CnHm, where n = 1,2,3,…11 and m = 1,2,3,… 8 until the desired molecular formula (C11H8) is achieved. A tabu-based search algorithm is used to generate several trial geometries of C11H8 hydrocarbons with various combinations of C and H atoms. After screening the initial structures, geometry optimization and frequency calculations for all the carbene and allene isomers have been carried out using DFT at the B3LYP/6-311 + G (d,p) and ωB97XD/6-311 + G (d,p) levels of theory [55–62]. For singlet electronic states, restricted Hartree-Fock (HF) wavefunction have been used as reference in the DFT calculations, whereas, unrestricted HF (UHF) wavefunction at the UB3LYP/6-311 + G (d,p) and UωB97XD/6-311 + G (d,p) levels has been used for open-shell triplet electronic state calculations. Although one can use restricted open-shell HF (ROHF) as a reference to avoid spin-contamination, we have observed that in certain cases ROHF based calculations don’t converge. Therefore, we have used UHF wavefunction throughout. We note that the spin-contamination is not too high for the triplet state, and most-often the value we got for <S2> is closer to the ideal value (2.00).
The infrared (IR) spectral frequencies and intensities have been captured from the frequency calculations on the optimized geometry. All calculations have been performed using the Gaussian 09 program [63].
Results and Discussions
Depending upon the skeletal structures, we have sub-divided the resonance stabilized bicylic isomers into six different groups (Figure 1). For example, 8-membered and 5-membered fused ring containing carbenes are represented as (8R-5R)-group. Similarly, other isomers are categorized as (7R-6R), (7R-5R), (6R-6R), (6R-5R), and (5R-5R) groups depending on the size of the rings. In the present study, we have identified 5 isomers in the 7R-6R, 12 in 6R-6R, 5 in 8R-5R, 26 in 7R-5R, 149 in 6R-5R and 220 isomers in 5R-5R groups, respectively. We have not considered the carbenes with less than five-membered ring because of higher relative energies due to lack of resonance stabilization or conjugation. The zero-point vibrational energy (ZPVE)-corrected relative energies of all isomers are scaled with respect to 1-azulenylcarbene, which is experimentally known [64]. On the basis of these relative energies, we found that the allene-like isomers of the (7R-6R) group are most stable, whereas, the isomers of the (5R-5R) group are high-lying isomers.
The current study reveals that several carbene and allene isomers remain elusive in the literature, some of which are more stable than the experimentally identified carbenes. The ZPVE-corrected relative energy, point group symmetry, and permanent dipole moments of each group have been listed along with their structures in Figures 2–7 for few low-lying carbene or allene isomers. Molecular structures of rest of the isomers are listed in the Electronic Supporting Information (ESI). Experimentally detected isomers are represented with an asterisk symbol. We note that, some of the carbene isomers corresponding to the allene-like structures turned out to be local maxima on the C11H8 PES. These low-lying transition states or intermediate saddle point structures are shown separately in Figure 8, along with their relative energies. The detailed ZPVE-corrected relative energies, Gibbs free energies and singlet−triplet energy gaps of all the low-lying isomers are shown in Tables 1–4 at the B3LYP/6-311+G (d,p) and ωB97XD/6-311+G (d,p) levels of theory. Energy details for all other isomers, along with useful spectroscopic parameters, such as, rotational constants, inertial axis dipole moments, and centrifugal distortion constants are listed in the ESI.
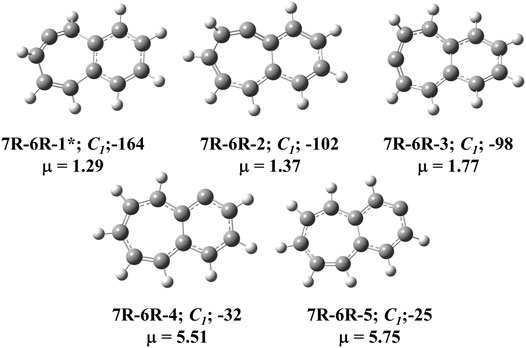
FIGURE 2. All low-lying C11H8 carbenes/cyclic allenes isomers with seven-membered and six-membered fused ring system i.e., (7R-6R) group. ZPVE-corrected relative energies (in kJ mol−1), absolute dipole moment values (in Debye) are calculated at the B3LYP/6-311 + G (d,p) level of theory. Experimentally detected isomers are marked with an asterisk symbol.
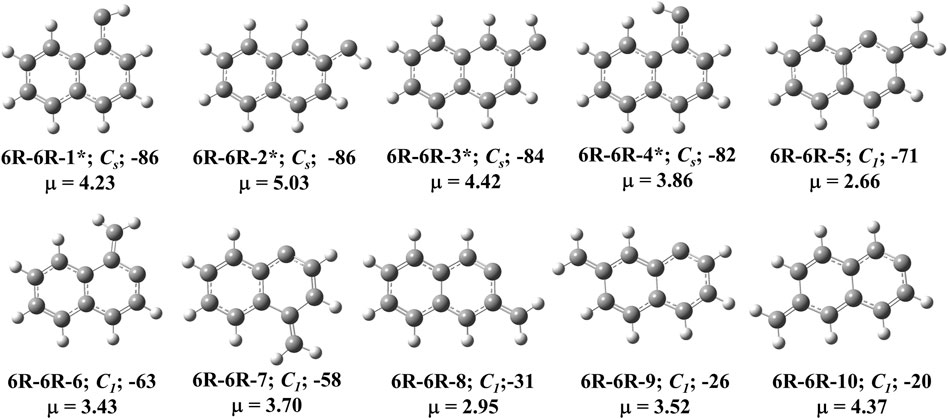
FIGURE 3. First ten low-lying C11H8 carbenes/cyclic allenes isomers of (6R-6R) group. ZPVE-corrected relative energies (in kJ mol−1), absolute dipole moment values (in Debye) are calculated at the B3LYP/6-311 + G (d,p) level of theory. Experimentally detected isomers are marked with an asterisk symbol.
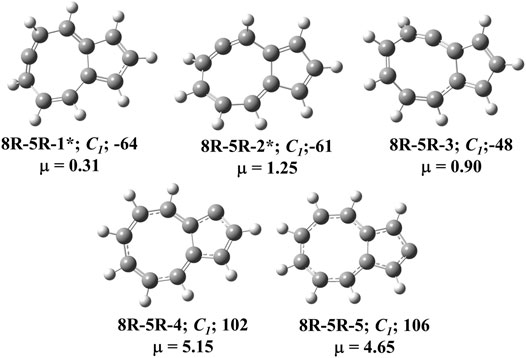
FIGURE 4. All low-lying C11H8 carbenes/cyclic allenes isomers of (8R-5R) group. ZPVE-corrected relative energies (in kJ mol−1), absolute dipole moment values (in Debye) are calculated at the B3LYP/6-311 + G (d,p) level of theory. Experimentally detected isomers are marked with an asterisk symbol.
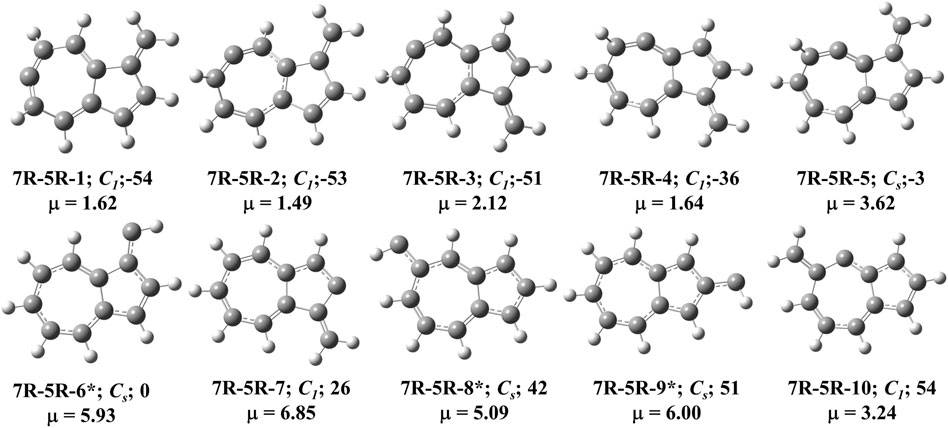
FIGURE 5. First ten low-lying C11H8 carbenes/cyclic allenes isomers of (7R-5R) group. ZPVE-corrected relative energies (in kJ mol−1), absolute dipole moment values (in Debye) are calculated at the B3LYP/6-311 + G (d,p) level of theory. Experimentally detected isomers are marked with an asterisk symbol.
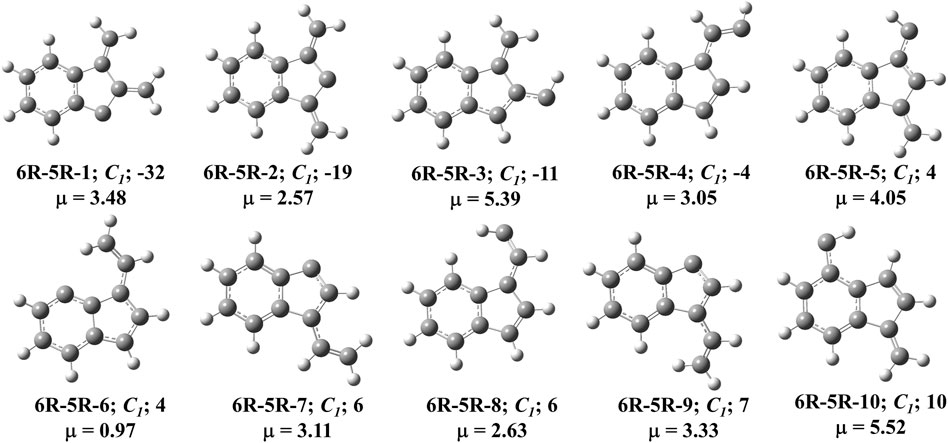
FIGURE 6. First ten low-lying C11H8 carbenes/cyclic allenes isomers of (6R-5R) group. ZPVE-corrected relative energies (in kJ mol−1), absolute dipole moment values (in Debye) are calculated at the B3LYP/6-311 + G (d,p) level of theory. Experimentally detected isomers are marked with an asterisk symbol.
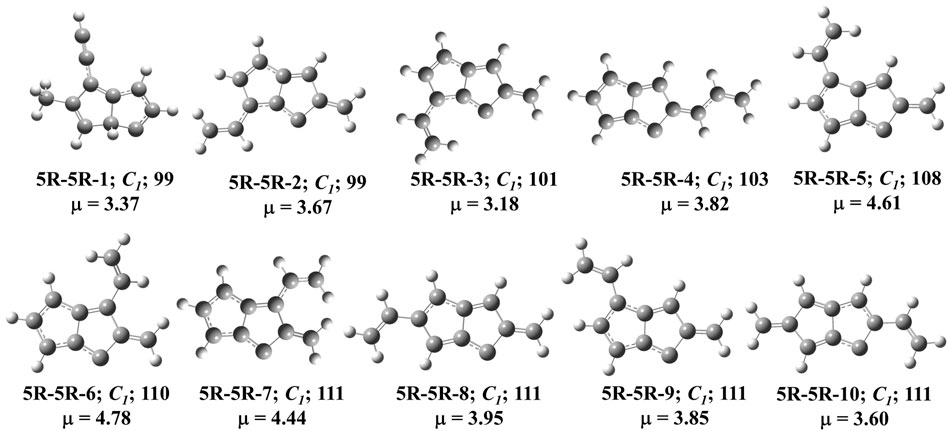
FIGURE 7. First ten low-lying C11H8 carbenes/cyclic allenes isomers of (5R-5R) group. ZPVE-corrected relative energies (in kJ mol−1), absolute dipole moment values (in Debye) are calculated at the B3LYP/6-311 + G (d,p) level of theory. Experimentally detected isomers are marked with an asterisk symbol.
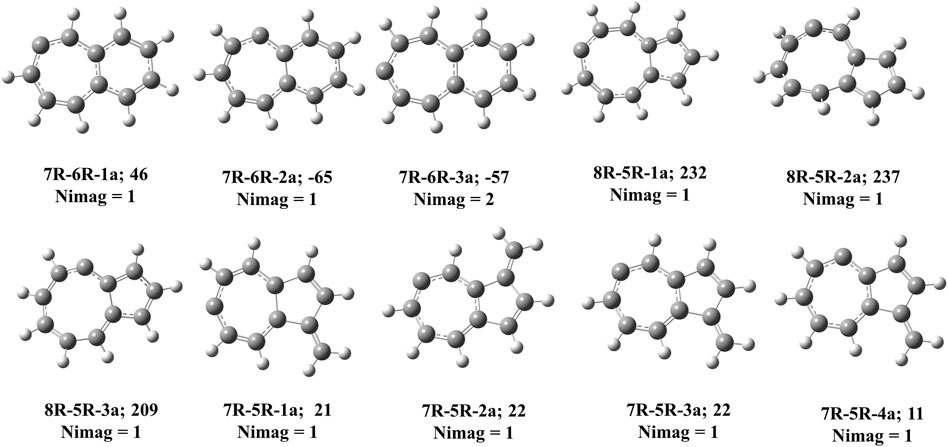
FIGURE 8. Carbene counter parts of various cyclic allene isomers at local maxima in C11H8 potential energy surface. ZPVE-corrected relative energies (in kJ mol−1), absolute dipole moment values (in Debye) are calculated at the B3LYP/6-311 + G (d,p) level of theory.
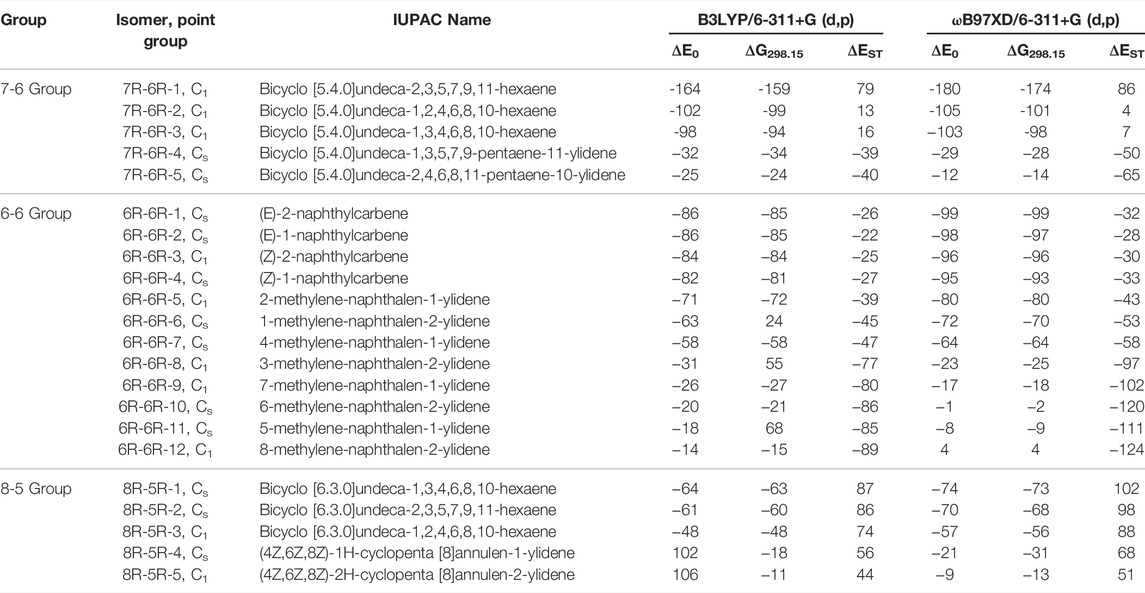
TABLE 1. ZPVE-Corrected Relative Energies (ΔE0; in kJ mol−1), Gibbs Free Energies (ΔG298.15; in kJ mol−1), and Singlet-Triplet Energy Gaps (ΔEST; in kJ mol−1) of low-lying C11H8 carbene/cyclic allene isomers calculated at Different Levels.
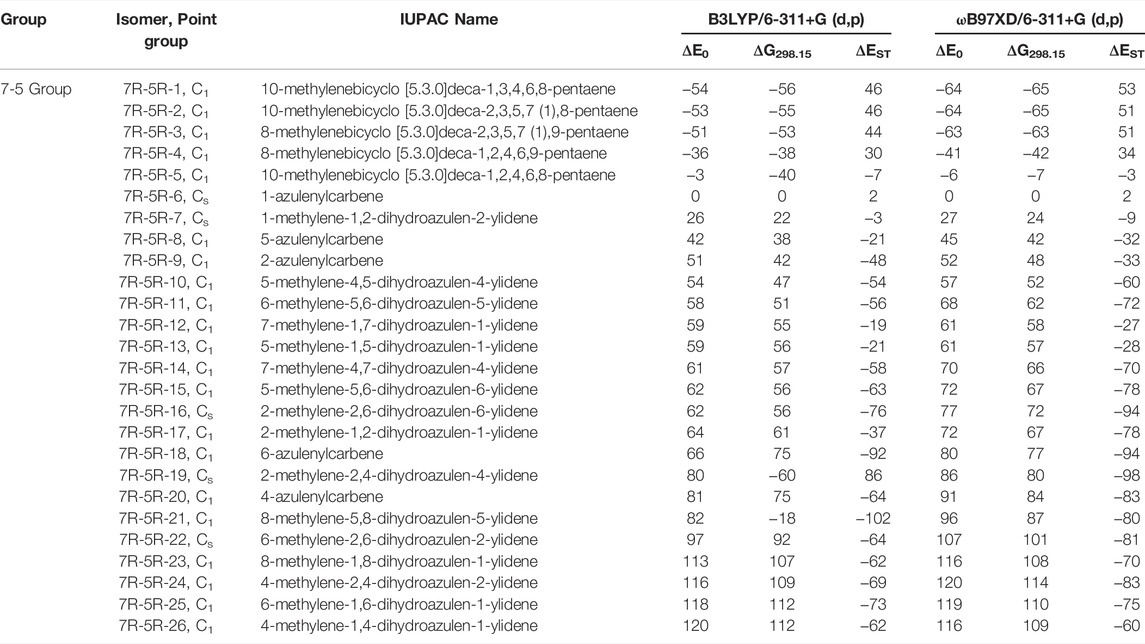
TABLE 2. Same as Table 1, low-lying C11H8 carbene isomer of (7R-5R) group calculated at different levels.
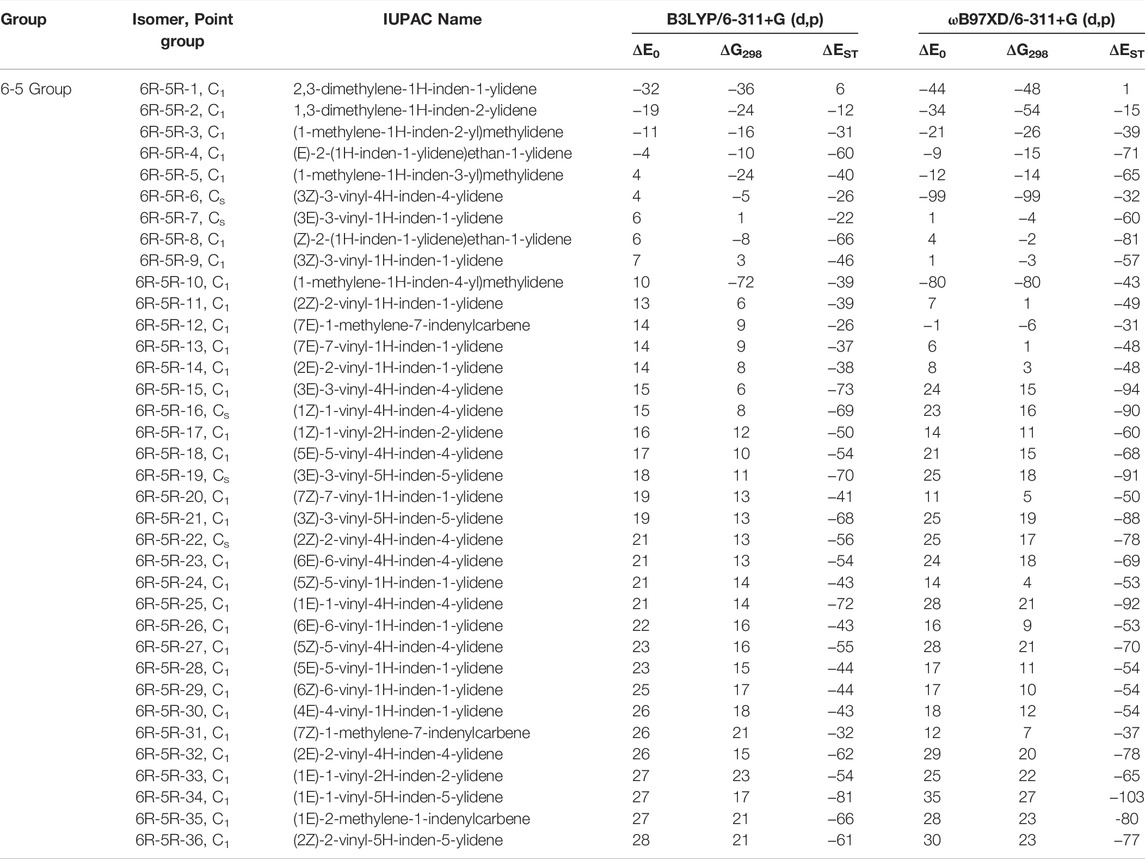
TABLE 3. Same as Table 1, low-lying C11H8 isomer of (6R-5R) Group within ∼30 kJ mol−1 energy range calculated at different levels.
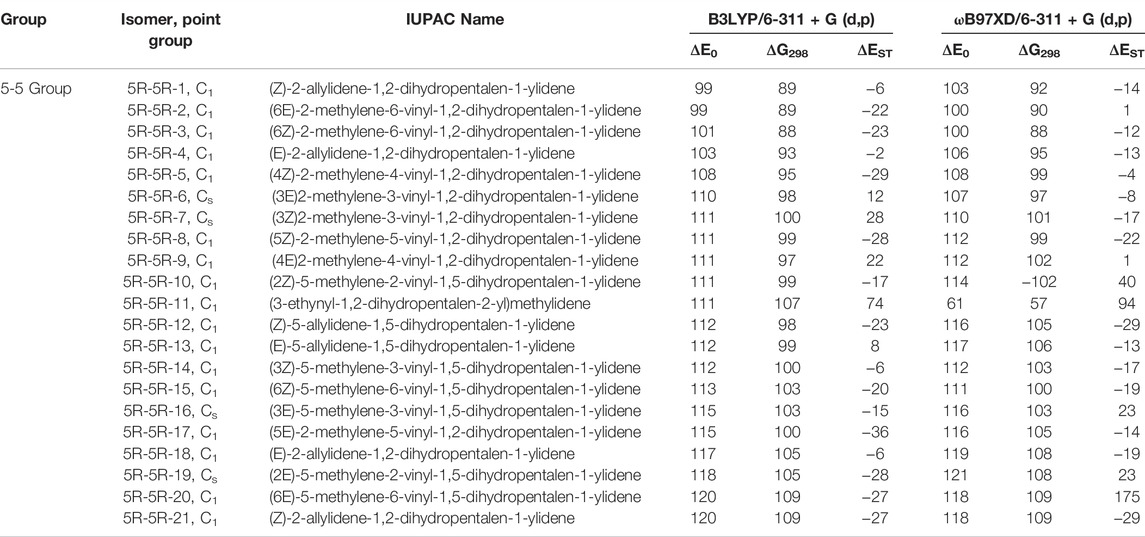
TABLE 4. Same as Table 1, low-lying C11H8 isomer of (5R-5R) group with in ∼120 kJ mol−1 energy range calculated at different levels.
The detailed energetics of each skeletal group have been discussed in the following:
(7R-6R) Group
The C11H8 carbene and allene like structures having one 7-membered and one 6-membered fused ring systems are listed in this group. As shown in Figure 2, total five minimum energy isomers are possible in this group and two transition states (7R-6R-1a and 7R-6R-2a) and one second order saddle point (7R-6R-3a) (Figure 8). Relative energies are shown in Table 1.
Bicyclo [5.4.0]undeca-2,3,5,7,9,11-hexaene (7R-6R-1) has been identified by West et al. in 1986 [65] and spectroscopically characterized by McMahon’s group in the year of 2000 through matrix isolation method [66]. According to our theoretical calculation at B3LYP/6-311 + G (d,p) level of theory, this allene-like molecule (7R-6R-1) is the lowest energy isomer on the C11H8 carbenes potential energy surface (PES). It is ∼164 kJ mol−1 lower in energy than the experimentally identified 1-azulenylcarbene. In 1- and 2-naphthylcarbene rearrangement, 7R-6R-1 has been considered as the potential key intermediate [67]. Isomers 7R-6R-2 (bicyclo [5.4.0]undeca-1,2,4,6,8,10-hexaene) and 7R-6R-3 (bicyclo [5.4.0]undeca-1,3,4,6,8,10-hexaene) are elusive in the laboratory till date. Both of them are lower in energy than 1-azulenylcarbene. 7R-6R-1a (benzo [7]annulen-6-ylidene, υi = 1,461.75i cm−1), 7R-6R-2a (benzo [7]annulen-5-ylidene, υi = 217.77i cm−1), and 7R-6R-3a (benzo [7]annulen-7-ylidene, υi = 134.77i cm−1, 119.63i cm−1) are the carbene counterparts of the stable allenes and they lie as the local maxima on the C11H8 PES. Bicyclo [5.4.0]undeca-1,3,5,7,9-pentaene-11-ylidene (7R-6R-4) and bicyclo [5.4.0]undeca-2,4,6,8,11-pentaene-10-ylidene (7R-6R-5) are also lower in energy than 1-azulenylcarbene by 32 and 25 kJ mol−1, respectively.
(6R-6R) Group
This group of carbenes have naphthalene moiety and 12 resonance stabilized naphthalene ring-containing isomers are possible for C11H8. Ten low-energy carbenes in this group have been shown in Figure 3 and their relative energies have been shown in Table 1. The most stable isomer in this group is (E)-1-naphthylcarbene (6R-6R-1), which is 86 kJ mol−1 lower in energy than 1-azulnylcarbene. (6R-6R-1), (E)-2-naphthylcarbene (6R-6R-2), (Z)-2-naphthylcarbene (6R-6R-3) and (Z)-1-naphthylcarbene (6R-6R-4) are the anti- and syn-conformer of 1- and 2-naphthylcarbenes, respectively. Though the singlet-triplet energy gap (ΔEST) of 1- and 2-naphthylcarbenes is relatively low (∼25 kJ mol−1), the triplet isomer state is more stable than the singlet isomer. Many years ago, in 1965, Trozzolo and co-workers have identified 2-naphthylcarbene (isomer 6R-6R-1 and 6R-6R-3) in its triplet ground electronic state via ESR spectroscopy [68]. Based on our chemical intuition, by varying the position of methylene group and carbene carbon, we have identified total eight methylene substituted naphthalene ring containing C11H8 carbene isomers. 2-methylene-naphthalen-1-ylidene (6R-6R-5), 1-methylene-naphthalen-2-ylidene (6R-6R-6), 4-methylene-naphthalen-1-ylidene (6R-6R-7), 3-methylene-naphthalen-2-ylidene (6R-6R-8), 7-methylene-naphthalen-1-ylidene (6R-6R-9), 6-methylene-naphthalen-2-ylidene (6R-6R-10), 5-methylene-naphthalen-1-ylidene (6R-6R-11), and 8-methylene-naphthalen-2-ylidene (6R-6R-12) are 71, 63, 58, 31, 26, 20, 18, and 14 kJ mol−1 lower in energy than 1-azulenylcarbene, respectively. The hydrocarbon derivatives of 6R-6R-5, 6R-6R-6, and 6R-6R-7 have been detected experimentally [69, 70]. According to our knowledge, parent carbene isomers 6R-6R-8, 6R-6R-9, 6R-6R-10, 6R-6R-11, and 6R-6R-12 are absent in the laboratory till date.
(8R-5R) Group
Total eight isomers are possible containing one eight-membered and one five-membered ring including carbene counter part of the low-lying cyclic allenes (Figures 4, 9). Out of these eight isomers, bicyclo [6.3.0]undeca-1,3,4,6,8,10-hexaene (8R-5R-1), bicyclo [6.3.0]undeca-2,3,5,7,9,11-hexaene (8R-5R-2), and bicyclo [6.3.0]undeca-1,2,4,6,8,10-hexaene (8R-5R-3) are 64, 61 and 48 kJ mol−1 lower in energy than 1-azulenylcarbene, respectively. Ring expansion reaction of 5-azulenylcarbene gives 8R-5R-1 and 8R-5R-2. In 2016, these two cyclic allenes were identified for the first time by Sander and co-workers in 2016 [71]. Bicyclo [6.3.0]undeca-1,2,4,6,8,10-hexaene (8R-5R-3) has not been unidentified in the laboratory till date. The carbene counterparts of these cyclic allenes (shown in Figure 8) are not minima on the C11H8 PES. 8R-5R-1a [(4Z,7Z,9Z)-cyclopenta [8]annulen-6-ylidene, υi = 377.36i cm−1], 8R-5R-2a [(3aZ,6Z,8Z)-cyclopenta [8]annulen-5-ylidene, υi = 507.32i cm−1], and 8R-5R-3a [(5Z,7Z,9Z)-cyclopenta [8]annulen-4-ylidene, υi = 292.69i cm−1] have one imaginary frequency each, therefore, they represent local maxima or transition states on the C11H8 PES. 8R-5R-4 [(4Z,6Z,8Z)-1H-cyclopenta [8]annulen-1-ylidene] and 8R-5R-5 [(4Z,6Z,8Z)-2H-cyclopenta [8]annulen-2-ylidene] are 102 and 106 kJ mol−1 higher in energy than 1-azulenylcarbene, respectively.
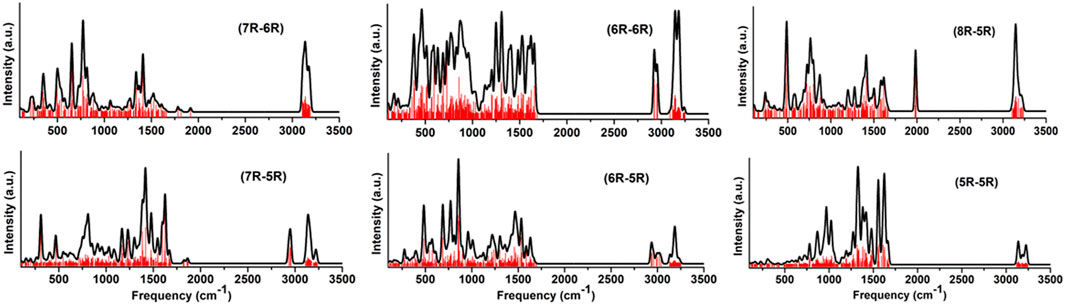
FIGURE 9. Convoluted vibrational spectra of low-lying carbene/cyclic allene isomers of various groups.
(7R-5R) Group
This type of C11H8 carbene/cyclic allene isomers contains fused seven and five membered moiety along with one substituent. Total twenty-six minima have been identified including carbene and allene like structures, out of which, 10 low-lying structures are shown in Figure 5, and energies of all the isomers are listed in Table 2. 10-methylenebicyclo [5.3.0]deca-1,3,4,6,8-pentaene (7R-5R-1), 10-methylenebicyclo [5.3.0]deca-2,3,5,7 (1),8-pentaene (7R-5R-2), 8-methylenebicyclo [5.3.0]deca-2,3,5,7 (1),9-pentaene (7R-5R-3) and 8-methylenebicyclo [5.3.0]deca-1,2,4,6,9-pentaene (7R-5R-4) are cyclic allene like structures. They are 54, 53, 51, and 36 kJ mol−1 lower in energy than 1-azulenylcarbene (7R-5R-6), respectively. All of them are elusive in the literature till date. The carbene counterparts of these allenes are not minima on the C11H8 PES (Figure 8). 1-methylene-1,6-dihydroazulen-6-ylidene (7R-5R-1a, υi = 405.80i cm−1), 3-methylene-3,5-dihydroazulen-5-ylidene (7R-5R-2a, υi = 384.13i cm−1), 1-methylene-1,5-dihydroazulen-5-ylidene (7R-5R-3a, υi = 394.10i cm−1) and 1-methylene-1,4-dihydroazulen-4-ylidene (7R-5R-4a, υi = 362.01i cm−1) are 21, 22, 22, and 10 kJ mol−1 higher in energy than 7R-5R-6. Five azulenylcarbenes (1-, 2-, 4-, 5-, and 6-azulenylcarbene) are present in this group. Among them 1-azulenylcarbene (7R-5R-6), 2-azulenylcarbene (7R-5R-9), 5-azulenylcarbene (7R-5R-8), and 6-azulenylcarbene (7R-5R-18) have already been detected experimentally [71] except 4-azulenylcarbene (8R-5R-20). 8R-5R-6 had been isolated by Sander’s group in 2012, through low-temperature argon matrix in its singlet ground electronic state [64]. But the other regio-isomers of 8R-5R-6 have been trapped at their triplet ground electronic state [71]. In this group, 21 low-lying methylene substituted azulenyl ring containing carbene/cyclic allene like C11H8 isomers are also present. Out of these 21 isomers, 1-methyleneazulene-8-ylidene (7R-5R-5) is 3 kJ mol−1 lower in energy than experimentally identified isomer 7R-5R-6. Isomer 7R-5R-5 is still elusive in the literature. The other methylene substituted azulenyl ring containing carbene-derivatives are higher in energy than 7R-5R-6. All of them are missing in the laboratory till date.
(6R-5R) Group
One six and one five membered fused indenyl ring along with one or two substituent containing C11H8 carbene isomers are mainly present in this group. We have identified one hundred forty-nine isomers in this group. Only 10 lowest energy carbene molecules are shown in Figure 6, rest of them are listed in the ESI. Energies of lowest thirty-six isomers (up to ∼28 kJ mol−1) have been shown in Table 3, rest is listed in ESI. 2,3-dimethylene-1H-inden-1-ylidene (6R-5R-1) and 1,3-dimethylene-1H-inden-2-ylidene (6R-5R-2) are the bi-methylene substituted indene derivative having carbene carbon at first and second position of the indenyl ring respectively. 6R-5R-1 is the most stable C11H8 carbene isomer with having one indenyl ring and it is 32 kJ mol−1 lower in energy than 1-azulenylcarbene. (1-methylene-1H-inden-2-yl)methylidene (6R-5R-3) and (E)-2-(1H-inden-1-ylidene)ethan-1-ylidene (6R-5R-4) are 11 and 4 kJ mol−1 lower in energy than 1-azulenylcarbene, respectively. (1-methylene-1H-inden-3-yl)methylidene (6R-5R-5), (3Z)-3-vinyl-4H-inden-4-ylidene (6R-5R-6), (3E)-3-vinyl-1H-inden-1-ylidene (6R-5R-7), (Z)-2-(1H-inden-1-ylidene)ethan-1-ylidene (6R-5R-8), (3Z)-3-vinyl-1H-inden-1-ylidene (6R-5R-9) and (1-methylene-1H-inden-4-yl)methylidene (6R-5R-10) are within 10 kJ mol−1 higher in energy than 1-azulenylcarbene. To the best of our knowledge, most of the carbene isomers listed in this group are still elusive in the laboratory.
(5R-5R) Group
This group of molecules contain two five membered fused ring along with mono-, di- or tri-substituted fragments. We have identified total two hundred twenty-seven carbene isomers in the C11H8 elemental composition. All these isomers are relatively higher in energy (within +98 to +435 kJ mol−1) from 1-azulenylcarbene. Only 10 isomers in this group have been shown in Figure 7, rest of the isomers is tabulated in the ESI. Although the lowest energy isomer, (Z)-2-allylidene-1,2-dihydropentalen-1-ylidene (5R-5R-1) is 98 kJ mol−1 higher in energy than the reference, ∼20 isomers lie within ∼20 kJ mol−1 in energy with respect to 5R-5R-1 (Table 4). Most of the isomers in this group are unidentified in the laboratory till date.
Stability of Carbenes Based on Hückel’s Rule
In this work, we have studied a large number of bicyclo-C11H8 isomers, most of which are carbenes. Previous studies [72] suggest that carbenes can be considered as a carbon atom with doubly occupied orbital in the molecular plane and an empty orbital perpendicular to the molecular plane. Therefore, the π-electron system in carbene is equivalent to a carbocation without any charge and usual rules for stabilization based on aromaticity could be applied to carbenes also. The doubly occupied orbital for carbenes having two nonbonded electrons can be either spin-paired (singlet state) or in parallel-spin (triplet state), whereas, in case of allenes, the electrons are always in paired form, therefore more stable than its carbene counterpart. Out of the six skeletal structures, (7R-6R) and (6R-6R) groups show better stability due to the presence of aromatic benzylic carbenes. In (7R-6R) group, 7R-6R-1, 7R-6R-2, and 7R-6R-3 show better stability due to their puckered cyclic-allene geometry where the electrons are always spin-paired, therefore, the singlet state geometry is more stable compared to triplet one. Likewise, one can explain the energetics of (8R-5R) group and (7R-5R) group, where the eight or seven membered cyclic allene like structure gives the better stability than the carbene isomers. Antiaromatic (8π electron) structure of eight membered ring containing carbene isomers (8R-5R-4 and 8R-5R-5) in (8R-5R) group led to high-energy isomers. Aryl carbenes along with its planar geometrical structure gives maximum delocalization between the vacant p-orbital of the carbene carbon with the neighboring arene π-system. Therefore, in case of (6R-6R) group, the electronic delocalization explains the stability of 6R-6R-1 to 6R-6R-4 where the carbene carbon is attached with an aromatic naphthyl ring. Isomer 6R-6R-5 to 6R-6R-8 has intact delocalized benzene rings, which leads to better stabilization of these compounds, whereas, in 6R-6R-9 and 6R-6R-10, methylene group breaks the delocalization. In similar manner, one can explain the energetics of (6R-5R) group. 6R-5R-10 contains 8π electrons in the cyclic conjugated system; the antiaromatic behavior of this isomer leads to instability. Most of the isomers in (5R-5R) groups are less conjugated structure and none of them fully satisfy the Huckel’s (4n+2) π-electron rule of aromaticity/antiaromaticity which explains why the (5R-5R) carbenes are so thermodynamically high in energy.
Spectroscopic Properties
Based on the permanent dipole moments obtained at the B3LYP/6-311+G (d,p) level, it is quite clear that many of these molecules are suitable targets for rotational spectroscopy. For example, the dipole moments of 7R-6R-4, 7R-6R-5, 6R-6R-10, 7R-5R-7, and 8R-5R-3 are greater than 5 Debye but they still remain elusive in the laboratory. These polar molecules are very good targets and lack of laboratory rest frequencies of these highly polar molecules may only lead to further complications in successfully resolving confirmation of molecules in the ISM.
To understand the astronomical emission features, computational modeling is commonly done along with laboratory experiments. In this regard, an IR spectroscopic database for a large number of PAH molecules have been created by NASA Ames Research Center [73, 74]. Considering the importance of the bicyclic carbene and cyclic allene isomers of C11H8 in the ISM, we have simulated the key IR spectroscopic signatures of the low energy isomers for each skeletal group. The vibrational frequencies and their intensities for each low-lying isomers have been computed using harmonic approximation at B3LYP/6-311 + G (d,p) level of theory as implemented in the Gaussian 09 software [63] without introducing any scaling factor. Considering a very crude model, all the vibrational frequencies along with their intensities are combined for a particular skeletal group and convoluted using a Gaussian profile with a full width at half maximum (FWHM) of 25 cm−1. The convoluted vibrational spectrum for each group is shown in Figure 9. Although from quantum chemistry perspective, some high-energy molecules may have stronger emission features, such effects have not been incorporated in this model.
From the spectral features, it can be easily identified that the common stretching frequencies of C-H symmetric and asymmetric vibrations in ∼3,000–3,200 cm−1 range. All of the isomers are resonance stabilised, therefore the C=C starching frequency can also be identified at ∼1,500 cm−1 for every group. Apart from the common features, the groups containing allene like isomers, (7R-6R), (8R-5R) and (7R-5R) group in particular, show characteristic vibrational stretching frequency at ∼1,800–2,000 cm−1 range. The fingerprint regions below 1,000 cm−1 are almost similar for all groups and no characteristic features can be highlighted to identify the skeletal difference (different ring size) between the bicyclo groups.
Summary and Future Studies
In summary, more than 400 isomers of C11H8 have been computationally identified using chemical intuition and tabu-search algorithm implemented in PyAR. All the stationary points have been obtained at the B3LYP/6-311 + G (d,p) level of theory initially and calculations are also done at the ωB97XD/6-311 + G (d,p) level to see effect of empirical dispersion corrections. It is noted here that we did not find huge changes in structures or energetics whether the calculations are done with or without empirical dispersion corrections. ZPVE-corrected relative energies, dipole moments, and point group symmetry of all molecules are collected at the B3LYP/6-311++G (d,p) level of theory. High-level electron-correlation effects have not been incorporated through coupled-cluster methods considering a huge set of isomers. However, we had done such calculations for few selective low-lying isomers in the past. The dipole moments of 7R-6R-4, 7R-6R-5, 6R-6R-10, 7R-5R-7, and 8R-5R-3 are greater than 5 Debye but they still remain elusive in the laboratory. It is noted here that C11H8 isomers are important key reactive intermediates for the formation of PAHs. Therefore, further experimental investigations are warranted. To the best of our knowledge, so far none of the isomers of C11H8 are characterized by rotational spectroscopy though they are having a permanent dipole moment (μ ≠ 0).
Data Availability Statement
The original contributions presented in the study are included in the article/Supplementary Material, further inquiries can be directed to the corresponding authors.
Author Contributions
TR: Structure prediction, Theoretical calculations, Data analysis and interpretation. SS: Structure prediction, Theoretical calculations, Data analysis and interpretation. VT: Data analysis and interpretation, Critical revision of the article, Final approval of the version to be published. SG: Conception or design of the work, Data analysis and interpretation, Drafting the article, Final approval of the version to be published.
Conflict of Interest
The authors declare that the research was conducted in the absence of any commercial or financial relationships that could be construed as a potential conflict of interest.
Publisher’s Note
All claims expressed in this article are solely those of the authors and do not necessarily represent those of their affiliated organizations, or those of the publisher, the editors and the reviewers. Any product that may be evaluated in this article, or claim that may be made by its manufacturer, is not guaranteed or endorsed by the publisher.
Acknowledgments
SG thanks Govt. of India (GoI) for grant no DST/NSM/R&D_HPC_Applications/2021/21 for the Paramshakti HPC facilities at IIT Kharagpur and doctoral fellowship for SS Computational facilities in NITD supported by EMR/2017/002653 and support provided at SDSU (for VT) by DURIP grant W911NF-10-1-0157 from the U.S. Department of Defense and by NSF CRIF grant CHE-0947087 is gratefully acknowledged. TR thanks NITD for doctoral fellowship. We thank the reviewers for insightful comments to enrich this manuscript.
Supplementary Material
The Supplementary Material for this article can be found online at: https://www.frontiersin.org/articles/10.3389/fphy.2022.907466/full#supplementary-material
References
1. Kaiser RI, Parker DSN, Mebel AM. Reaction Dynamics in Astrochemistry: Low-Temperature Pathways to Polycyclic Aromatic Hydrocarbons in the Interstellar Medium. Annu Rev Phys Chem (2015) 66(1):43–67. doi:10.1146/annurev-physchem-040214-121502
2. Lemmens AK, Rap DB, Thunnissen JMM, Willemsen B, Rijs AM. Polycyclic Aromatic Hydrocarbon Formation Chemistry in a Plasma Jet Revealed by Ir-Uv Action Spectroscopy. Nat Commun (2020) 11(1):269. doi:10.1038/s41467-019-14092-3
3. Li A. Spitzer’s Perspective of Polycyclic Aromatic Hydrocarbons in Galaxies. Nat Astron (2020) 4(4):339–51. doi:10.1038/s41550-020-1051-1
4. Reddy SN, Mahapatra S. Theoretical Study on Molecules of Interstellar Interest. Ii. Radical Cation of Compact Polycyclic Aromatic Hydrocarbons. J Phys Chem B (2015) 119(34):11391–402. doi:10.1021/acs.jpcb.5b03614
5. Reizer E, Viskolcz B, Fiser B. Formation and Growth Mechanisms of Polycyclic Aromatic Hydrocarbons: A Mini-Review. Chemosphere (2022) 291:132793. doi:10.1016/j.chemosphere.2021.132793
6. Herbst E. Three Milieux for Interstellar Chemistry: Gas, Dust, and Ice. Phys Chem Chem Phys (2014) 16(8):3344–59. doi:10.1039/C3CP54065K
7. Schlemmer S, Giesen T, Mutschke H. Laboratory Astrochemistry: From Molecules through Nanoparticles to Grains. Weinheim: John Wiley & Sons (2014).
8. Woon DE, Herbst E. Quantum Chemical Predictions of the Properties of Known and Postulated Neutral Interstellar Molecules. Astrophys J Suppl Ser (2009) 185(2):273–88. doi:10.1088/0067-0049/185/2/273
9. Bauschlicher CW, Peeters E, Allamandola LJ. The Infrared Spectra of Very Large Irregular Polycyclic Aromatic Hydrocarbons (Pahs): Observational Probes of Astronomical Pah Geometry, Size, and Charge. Astrophys J (2009) 697(1):311–27. doi:10.1088/0004-637x/697/1/311
10. Fortenberry RC. Quantum Astrochemical Spectroscopy. Int J Quan Chem. (2017) 117(2):81–91. doi:10.1002/qua.25180
11. Puzzarini C. Astronomical Complex Organic Molecules: Quantum Chemistry Meets Rotational Spectroscopy. Int J Quan Chem. (2017) 117(2):129–38. doi:10.1002/qua.25284
12. Candian A, Mackie CJ. Anharmonic Interstellar Pah Molecules. Int J Quan Chem. (2017) 117(2):146–50. doi:10.1002/qua.25292
13. Peverati R, Bera PP, Lee TJ, Head-Gordon M. Insights into Hydrocarbon Chain and Aromatic Ring Formation in the Interstellar Medium: Computational Study of the Isomers of C4h3+, C6h3+ and C6h5+ and Their Formation Pathways. Astrophys J (2016) 830(2):128. doi:10.3847/0004-637x/830/2/128
14. Kaiser RI, Ochsenfeld C, Stranges D, Head-Gordon M, Lee YT. Combined Crossed Molecular Beams and Ab Initio Investigation of the Formation of Carbon-Bearing Molecules in the Interstellar Medium via Neutral–Neutral Reactions. Faraday Disc. (1998) 109:183–204. doi:10.1039/a800077h
15. Kaiser RI, Ochsenfeld C, Head-Gordon M, Lee YT, Suits AG. A Combined Experimental and Theoretical Study on the Formation of Interstellar C3h Isomers. Science (1996) 274(5292):1508–11. doi:10.1126/science.274.5292.1508
16. Heikkilä A, Johansson LE, Olofsson H. Molecular Abundance Variations in the Magellanic Clouds. Astron Astrophys (1999) 344:817–47.
17. Böhme DK. Experimental Studies of Positive Ion Chemistry with Flow-Tube Mass Spectrometry: Birth, Evolution, and Achievements in the 20th Century. Int J Mass Spectrom (2000) 200(1-3):97–136. doi:10.1016/s1387-3806(00)00299-2
18. Gronert S. Quadrupole Ion Trap Studies of Fundamental Organic Reactions. Mass Spectrom Rev (2005) 24(1):100–20. doi:10.1002/mas.20008
19. Nibbering NMM. Four Decades of Joy in Mass Spectrometry. Mass Spectrom Rev (2006) 25(6):962–1017. doi:10.1002/mas.20099
20. Uggerud E. Physical Organic Chemistry of the Gas Phase. Reactivity Trends for Organic Cations. In: Modern Mass Spectrometry. Springer (2003). p. 3–36. doi:10.1007/3-540-36113-8_1
21. Mebel AM, Georgievskii Y, Jasper AW, Klippenstein SJ. Temperature- and Pressure-dependent Rate Coefficients for the HACA Pathways from Benzene to Naphthalene. Proc Combust Inst (2017) 36(1):919–26. doi:10.1016/j.proci.2016.07.013
22. Kislov VV, Sadovnikov AI, Mebel AM. Formation Mechanism of Polycyclic Aromatic Hydrocarbons beyond the Second Aromatic Ring. J Phys Chem A (2013) 117(23):4794–816. doi:10.1021/jp402481y
23. Violi A, D’Anna A, D’Alessio A. Modeling of Particulate Formation in Combustion and Pyrolysis. Chem Eng Sci (1999) 54(15-16):3433–42. doi:10.1016/s0009-2509(98)00460-6
24. Raj A, Al Rashidi MJ, Chung SH, Sarathy SM. Pah Growth Initiated by Propargyl Addition: Mechanism Development and Computational Kinetics. J Phys Chem A (2014) 118(16):2865–85. doi:10.1021/jp410704b
25. Wei M, Zhang T, Chen X, Yan F, Guo G, Zhang D. Formation of Bicyclic Polycyclic Aromatic Hydrocarbons (Pahs) from the Reaction of a Phenyl Radical with Cis-3-Penten-1-Yne. RSC Adv (2018) 8(24):13226–36. doi:10.1039/c8ra01449c
26. Parker DSN, Zhang F, Kim YS, Kaiser RI, Landera A, Kislov VV, et al. Low Temperature Formation of Naphthalene and its Role in the Synthesis of Pahs (Polycyclic Aromatic Hydrocarbons) in the Interstellar Medium. Proc Natl Acad Sci U.S.A (2012) 109(1):53–8. doi:10.1073/pnas.1113827108
27. Yang T, Muzangwa L, Kaiser RI, Jamal A, Morokuma K. A Combined Crossed Molecular Beam and Theoretical Investigation of the Reaction of the Meta-Tolyl Radical with Vinylacetylene – toward the Formation of Methylnaphthalenes. Phys Chem Chem Phys (2015) 17(33):21564–75. doi:10.1039/C5CP03285G
28. Kaiser RI, Dangi BB, Yang T, Parker DSN, Mebel AM. Reaction Dynamics of the 4-Methylphenyl Radical (P-Tolyl) with 1, 2-Butadiene (1-Methylallene): Are Methyl Groups Purely Spectators? J Phys Chem A (2014) 118(32):6181–90. doi:10.1021/jp505868q
29. Burkhardt AM, Long Kelvin Lee K, Bryan Changala P, Shingledecker CN, Cooke IR, Loomis RA, et al. Discovery of the Pure Polycyclic Aromatic Hydrocarbon Indene (C-C9h8) with Gotham Observations of Tmc-1. Astrophys J Lett (2021) 913(2):L18. doi:10.3847/2041-8213/abfd3a
30. McGuire BA, Loomis RA, Burkhardt AM, Lee KLK, Shingledecker CN, Charnley SB, et al. Detection of Two Interstellar Polycyclic Aromatic Hydrocarbons via Spectral Matched Filtering. Science (2021) 371(6535):1265–9. doi:10.1126/science.abb7535
31. Kelvin Lee KL, Changala PB, Loomis RA, Burkhardt AM, Xue C, Cordiner MA, et al. Interstellar Detection of 2-Cyanocyclopentadiene, C5h5cn, a Second Five-Membered Ring toward Tmc-1. Astrophys J Lett (2021) 910(1):L2. doi:10.3847/2041-8213/abe764
32. McGuire BA, Burkhardt AM, Kalenskii S, Shingledecker CN, Remijan AJ, Herbst E, et al. Detection of the Aromatic Molecule Benzonitrile (C-C6h5cn) in the Interstellar Medium. Science (2018) 359(6372):202–5. doi:10.1126/science.aao4890
33. Cabezas C, Tercero B, Agúndez M, Marcelino N, Pardo J, de Vicente P, et al. Cumulene Carbenes in Tmc-1: Astronomical Discovery of L-H2c5. Astron Astrophys (2021) 650:L9. doi:10.1051/0004-6361/202141274
34. McCarthy MC, Travers MJ, Kovács A, Chen W, Novick SE, Gottlieb CA, et al. Detection and Characterization of the Cumulene Carbenes H2c5 and H2c6. Science (1997) 275(5299):518–20. doi:10.1126/science.275.5299.518
35. Cernicharo J, Gottlieb CA, Guélin M, Killian TC, Paubert G, Thaddeus P, et al. Astronomical Detection of H2ccc. Astrophys J (1991) 368:L39–L41. doi:10.1086/185943
36. Cernicharo J, Agúndez M, Cabezas C, Tercero B, Marcelino N, Pardo JR, et al. Pure Hydrocarbon Cycles in Tmc-1: Discovery of Ethynyl Cyclopropenylidene, Cyclopentadiene and Indene. Astron Astrophys (2021) 649:649. doi:10.1051/0004-6361/202141156
37. Thimmakondu VS, Ulusoy I, Wilson AK, Karton A. Theoretical Studies of Two Key Low-Lying Carbenes of C5h2 Missing in the Laboratory. J Phys Chem A (2019) 123(30):6618–27. doi:10.1021/acs.jpca.9b06036
38. Karton A, Thimmakondu VS. From Molecules with a Planar Tetracoordinate Carbon to an Astronomically Known C5H2 Carbene. The J Phys Chem A (2022) 126(16):2561–8. doi:10.1021/acs.jpca.2c01261
39. Fortenberry RC. The Formation of Astromolecule Ethynyl Cyclopropenylidene (C-C3hcch) from C2h and C-C3h2. Astrophys J (2021) 921(2):132. doi:10.3847/1538-4357/ac222c
40. Thaddeus P, Vrtilek JM, Gottlieb CA. Laboratory and Astronomical Identification of Cyclopropenylidene, C3h2. Astrophys J (1985) 299:L63–L6. doi:10.1086/184581
41. Cernicharo J, Gottlieb CA, Guélin M, Killian TC, Thaddeus P, Vrtilek JM. Astronomical Detection of H2cccc. Astrophys J (1991) 368:L43–L5. doi:10.1086/185944
42. Langer WD, Velusamy T, Kuiper TBH, Peng R, McCarthy MC, Travers MJ, et al. First Astronomical Detection of the Cumulene Carbon Chain Molecule H2c6 in Tmc-1. Astrophys J (1997) 480(1):L63–L66. doi:10.1086/310622
43. Menon A, Leon G, Akroyd J, Kraft M. A Density Functional Theory Study on the Kinetics of Seven-Member Ring Formation in Polyaromatic Hydrocarbons. Combust Flame (2020) 217:152–74. doi:10.1016/j.combustflame.2020.03.032
44. Stahl F, von Ragué Schleyer P, Bettinger HF, Kaiser RI, Lee YT, Schaefer HF. Reaction of the Ethynyl Radical, C2H, with Methylacetylene, Ch 3 Cch, under Single Collision Conditions: Implications for Astrochemistry. J Chem Phys (2001) 114(8):3476–87. doi:10.1063/1.1331360
45. Allamandola LJ, Tielens GGM, Barker JR. Interstellar Polycyclic Aromatic Hydrocarbons: The Infrared Emission Bands, the Excitation/Emission Mechanism, and the Astrophysical Implications. Astrophys J Suppl Ser (1989) 71:733. doi:10.1086/191396
46. Reddy VS, Ghanta S, Mahapatra S. First Principles Quantum Dynamical Investigation Provides Evidence for the Role of Polycyclic Aromatic Hydrocarbon Radical Cations in Interstellar Physics. Phys Rev Lett (2010) 104(11):111102. doi:10.1103/PhysRevLett.104.111102
47. Ghanta S, Reddy VS, Mahapatra S. Theoretical Study of Electronically Excited Radical Cations of Naphthalene and Anthracene as Archetypal Models for Astrophysical Observations. Part I. Static Aspects. Phys Chem Chem Phys (2011) 13(32):14523–30. doi:10.1039/C1CP21083A
48. Allamandola LJ, Hudgins DM, Sandford SA. Modeling the Unidentified Infrared Emission with Combinations of Polycyclic Aromatic Hydrocarbons. Astrophys J (1999) 511(2):L115–L119. doi:10.1086/311843
49. Pathak A, Rastogi S. Theoretical Spectra of Pahs in Modeling Astrophysical Ir Features. Adv Space Res (2007) 40(11):1620–7. doi:10.1016/j.asr.2007.08.011
50. Roy T, Ghosal S, Thimmakondu VS. Six Low-Lying Isomers of C11h8 Are Unidentified in the Laboratory–A Theoretical Study. J Phys Chem A (2021) 125(20):4352–64. doi:10.1021/acs.jpca.1c02247
51. Nagy A, Fulara J, Maier JP. Formation of Aromatic Structures from Chain Hydrocarbons in Electrical Discharges: Absorption and Fluorescence Study of C11h9+ and C11h9• Isomers in Neon Matrices. J Am Chem Soc (2011) 133(49):19796–806. doi:10.1021/ja206522s
52. Robinson AG, Winter PR, Ramos C, Zwier TS. Ultraviolet Photochemistry of Diacetylene: Reactions with Benzene and Toluene. J Phys Chem A (2000) 104(45):10312–20. doi:10.1021/jp001427v
53. Nagy A, Garkusha I, Fulara J, Maier JP. Electronic Spectroscopy of Transient Species in Solid Neon: The Indene-Motif Polycyclic Hydrocarbon Cation Family C9HY+(Y= 7–9) and Their Neutrals. Phys Chem Chem Phys (2013) 15(44):19091–101. doi:10.1039/c3cp52172a
54. Khatun M, Majumdar RS, Anoop A. A Global Optimizer for Nanoclusters. Front Chem (2019) 7:644. doi:10.3389/fchem.2019.00644
55. Krishnan R, Binkley JS, Seeger R, Pople JA. Self-Consistent Molecular Orbital Methods. Xx. A Basis Set for Correlated Wave Functions. J Chem Phys (1980) 72:650–4. doi:10.1063/1.438955
56. Clark T, Chandrasekhar J, Spitznagel GW, Schleyer PR. Efficient Diffuse Function Augmented Basis Sets for Anion Calculations. III. The 3-21+G Basis Set for First Row Elements, Li-F. J Comput Chem (1983) 4:294–301. doi:10.1002/jcc.540040303
57. Becke AD. Density-Functional Exchange-Energy Approximation with Correct Asymptotic Behavior. Phys Rev A (1988) 38(6):3098–100. doi:10.1103/PhysRevA.38.3098
58. Lee C, Yang W, Parr RG. Development of the Colle-Salvetti Correlation-Energy Formula into a Functional of the Electron Density. Phys Rev B (1988) 37(2):785–9. doi:10.1103/PhysRevB.37.785
59. Grimme S, Steinmetz M, Korth M. How to Compute Isomerization Energies of Organic Molecules with Quantum Chemical Methods. J Org Chem (2007) 72(6):2118–26. doi:10.1021/jo062446p
60. Chai J-D, Head-Gordon M. Long-Range Corrected Hybrid Density Functionals with Damped Atom–Atom Dispersion Corrections. Phys Chem Chem Phys (2008) 10(44):6615–20. doi:10.1039/B810189B
61. Grimme S, Antony J, Ehrlich S, Krieg H. A Consistent and Accurate Ab Initio Parametrization of Density Functional Dispersion Correction (Dft-D) for the 94 Elements H-Pu. J Chem Phys (2010) 132(15):154104. doi:10.1063/1.3382344
62. Karton A, Martin JML. Explicitly Correlated Benchmark Calculations on C8h8 Isomer Energy Separations: How Accurate Are Dft, Double-Hybrid, and Composite Ab Initio Procedures? Mol Phys (2012) 110(19-20):2477–91. doi:10.1080/00268976.2012.698316
63. Frisch MJ, Trucks GW, Schlegel HB, Scuseria GE, Robb MA, Cheeseman JR, et al. Gaussian 09 Rev. D.01. Wallingford, CT: Gaussian, Inc. (2009).
64. Henkel S, Huynh Y-a., Neuhaus P, Winkler M, Sander W. Tunneling Rearrangement of 1-Azulenylcarbene. J Am Chem Soc (2012) 134(32):13204–7. doi:10.1021/ja3050376
65. West PR, Mooring AM, McMahon RJ, Chapman OL. Benzobicyclo[4.1.0]Hepta-2,4,6-Trienes. J Org Chem (1986) 51(8):1316–20. doi:10.1021/jo00358a029
66. Bonvallet PA, McMahon RJ. Generation, Characterization, and Rearrangements of 4, 5-Benzocyclohepta-1, 2, 4, 6-Tetraene. J Am Chem Soc (2000) 122(38):9332–3. doi:10.1021/ja001291e
67. Xie Y, Schreiner PR, Schleyer Pv. R, Schaefer HF. The Naphthylcarbene Potential Energy Hypersurface. J Am Chem Soc (1997) 119(6):1370–7. doi:10.1021/ja9517072
68. Trozzolo AM, Wasserman E, Yager WA. Geometric Isomers of Methylenes. J Am Chem Soc (1965) 87(1):129–30. doi:10.1021/ja01079a030
69. Scott LT, Brunsvold WR. Double-Bond Isomers of Aromatic Compounds. 1-Methylene-1, 2-Dihydronaphthalene. J Org Chem (1979) 44(4):641. doi:10.1021/jo01318a039
70. Bartmess JE, Griffith SS. Tautomerization Energetics of Benzoannelated Toluenes. J Am Chem Soc (1990) 112(8):2931–6. doi:10.1021/ja00164a014
71. Henkel S, Pollok CH, Schleif T, Sander W. Azulenylcarbenes: Rearrangements on the C11h8 Potential Energy Surface. Chem Eur J (2016) 22(35):12479–86. doi:10.1002/chem.201601390
72. Woodcock HL, Moran D, Brooks BR, Schleyer Pv. R, Schaefer HF. Carbene Stabilization by Aryl Substituents. Is Bigger Better? J Am Chem Soc (2007) 129(12):3763–70. doi:10.1021/ja068899t
73. Bauschlicher CW, Ricca A, Boersma C, Allamandola LJ. The Nasa Ames Pah Ir Spectroscopic Database: Computational Version 3.00 with Updated Content and the Introduction of Multiple Scaling Factors. Astrophys J Suppl Ser (2018) 234(2):32. doi:10.3847/1538-4365/aaa019
Keywords: C11H8, carbene, PAHs, PyAR, DFT
Citation: Roy T, Satpati S, Thimmakondu VS and Ghosal S (2022) Theoretical Investigation on C11H8 Bicyclic Carbene and Allene Isomers. Front. Phys. 10:907466. doi: 10.3389/fphy.2022.907466
Received: 29 March 2022; Accepted: 26 May 2022;
Published: 22 June 2022.
Edited by:
Maria Rutigliano, Institute for Plasma Science and Technology (CNR), ItalyReviewed by:
Timothy Schmidt, University of New South Wales, AustraliaDaniel Aranda, University of Malaga, Spain
Copyright © 2022 Roy, Satpati, Thimmakondu and Ghosal. This is an open-access article distributed under the terms of the Creative Commons Attribution License (CC BY). The use, distribution or reproduction in other forums is permitted, provided the original author(s) and the copyright owner(s) are credited and that the original publication in this journal is cited, in accordance with accepted academic practice. No use, distribution or reproduction is permitted which does not comply with these terms.
*Correspondence: Venkatesan S. Thimmakondu, dnRoaW1tYWtvbmR1c2FteUBzZHN1LmVkdQ==; Subhas Ghosal, c3ViaGFzLmdob3NhbEBjaC5uaXRkZ3AuYWMuaW4=
†These authors have contributed equally to the manuscript