- 1International Collaborative Laboratory of 2D Materials for Optoelectronic Science and Technology of Ministry of Education, Institute of Microscale Optoelectronics (IMO), Shenzhen University, Shenzhen, China
- 2College of Physics and Optoelectronic Engineering, Shenzhen University, Shenzhen, China
A high-speed all-optical modulator is a key device in next-generation communication systems. Due to easy fabrication and an effective modulation effect, two-dimensional (2D) material–microfiber structure all-optical modulators have aroused much attention. Graphdiyne (GDY) is an excellent nonlinear optical material and is expected to be utilized in all-optical modulators. In this work, we demonstrate a GDY-coated all-optical temporal modulator according to its saturable absorption. Under the pump pulse light of 1,064 nm, the fabricated modulator successfully modulates the continuous-wave (CW) light of 1,550 nm to the waveform of pump light with a pulse width of 4 ns and a repetition rate of 5 kHz. Our results show that GDY could be used in high-speed all-optical modulators and pave a way for the research of GDY in all-optical information processing applications.
Introduction
High-speed signal processing is an essential technology and is greatly demanded in both ultrahigh optical communications and storage systems. However, the “electrical bottleneck” in electronic–optic processing devices limits further improvement of the bit rate in communication systems. All-optical processing is a momentous technology which can avoid the process of optoelectronic signal conversion and achieve high speeds of signal processing. In fiber communication systems, the all-optical modulator, as a kind of all-optical processing device, is an important research topic for research [1–4].
A simple and convenient way to realize all-optical modulation is to utilize the nonlinear effect of 2D materials, such as saturable absorption (SA) [5–10], optical Kerr effect (OKE) [11–15], and thermo-optic effect (TOE) [16–21]. Two-dimensional materials exhibit excellent nonlinear optical properties of ultrafast carrier relaxation time [22–24], excellent thermal-optic conversion efficiency [16–21], and large third-order nonlinearity [26–28], which verify its potentiality in all-optical modulation. By taking advantage of the evanescent field in a microfiber covered with 2D materials, 2D material-coated microfibers can realize all-optical modulation. For example, in 2014, Li et al. reported a saturable absorption (SA) modulator by a graphene-cladding microfiber structure, which showed a 200-GHz modulation speed [7]. The ultrafast modulation speed is mainly owed to the intrinsic graphene response time (∼2.2 ps). In 2017, Zheng et al. successfully realized black phosphorene (BP)-coated microfiber SA modulator [6]. The BP modulator allows switch light and signal light work in the communication band together. In addition, Yu et al. reported a phase all-optical modulator based on OKE of graphene, which exhibited a fast modulation speed (10 ns) [11]. The phase all-optical modulator could also be realized by the TOE; in 2021, Tiu et al. reported an all-fiber phase modulator by using a MoWS2-rGO-coated tapered fiber [20]. In central asymmetric 2D materials, TOE-induced anisotropy refractive index change can realize polarization modulation. Wang et al. and Tiu et al. reported MXene-based and MoS2-based polarization modulator, respectively [21, 25]. The previous reports show that 2D materials have a great potential in modern all-optical modulators and deserve further investigations.
Graphdiyne, an allotrope of graphene, has been added to the 2D material family and has attracted many investigations in recent years. It has excellent physical and chemical properties [29–31] and has been reported to have abundant applications such as purification [32], energy storage and transfer [33, 34], biomedicine and therapy [35, 36], optical detectors [37, 38], and modulators [39, 40]. GDY has a natural direct bandgap of 0.44–1.42 eV, short carrier relaxation time (picoseconds scale from visible light to infrared band) [30], and excellent nonlinear optical properties (β > –1 cm GW−1) [24], which make it a good candidate to realize broadband all-optical devices. Recently, a GDY-based thermo-optical modulator has been reported by our group, and it shows a good modulation depth and wide modulation band [16]. However, the thermal-optical effect has the limitation of modulation speed. To realize high-speed GDY all-optical modulators, saturable absorption effect-based modulation is a good choice, which has not been researched until now. Therefore, it is necessary to study the feasibility of the ultrafast nonlinear GDY all-optical modulator.
Herein, we demonstrate a GDY-coated all-optical temporal modulator according to its saturable absorption effect. By employing the microfiber structure, the evanescent wave can easily interact with GDY nanosheets. The fabricated GDY modulator exhibits saturable absorption at 1,064 nm. When adding a continuous-wave (CW) light of 1,550 nm and a pulse light of 1,064 nm into the modulator together, the CW light is successfully modulated to the waveform of pulse light with a pulse width of 4 ns and a repetition rate of 5 kHz. The result shows that GDY could be used for high-speed all-optical modulators.
Characterization of GDY
Preparation of GDY nanosheets are illustrated as follows: First, GDY powder is exfoliated from GDY films that grow on copper foil [41]. Second, GDY nanoparticle dispersions are prepared by using the standard liquid-phase exfoliation (LPE) method [42]. A measure of 10 ml ethanol solution was added to a centrifuge tube containing 5 mg GDY powder to prepare 0.5 mg mL−1 GDY dispersions. The dispersions are then sonicated for 3 h by using the bath sonication method and centrifuged at 1,500 rpm and 3,000 rpm for 90 min to remove the unexfoliated flakes. The top 1/2 of the dispersions are collected by another tube. The prepared GDY dispersions have a concentration of 0.05 mg mL−1. In order to observe the morphology and features of GDY nanoparticle dispersions, the images of transmission electron microscopy (TEM) and high resolute transmission electron microscopy (HRTEM) are acquired and shown in Figures 1A,B, respectively. Figure 1C shows the Raman spectrum of GDY nanosheets. The Raman spectrum of GDY shows four bands: the D band (1,352 cm−1), the G band (1,585 cm−1), and two bands derived from the vibration of the conjugated diyne linkage (1932 and 2,150 cm−1). Ultraviolet and visible light (UV–vis) absorption spectroscopy is employed to characterize the optical property of GDY, as shown in Figure 1D. It is showed that GDY has a broadband absorption ranging from 600 to 1,600 nm.
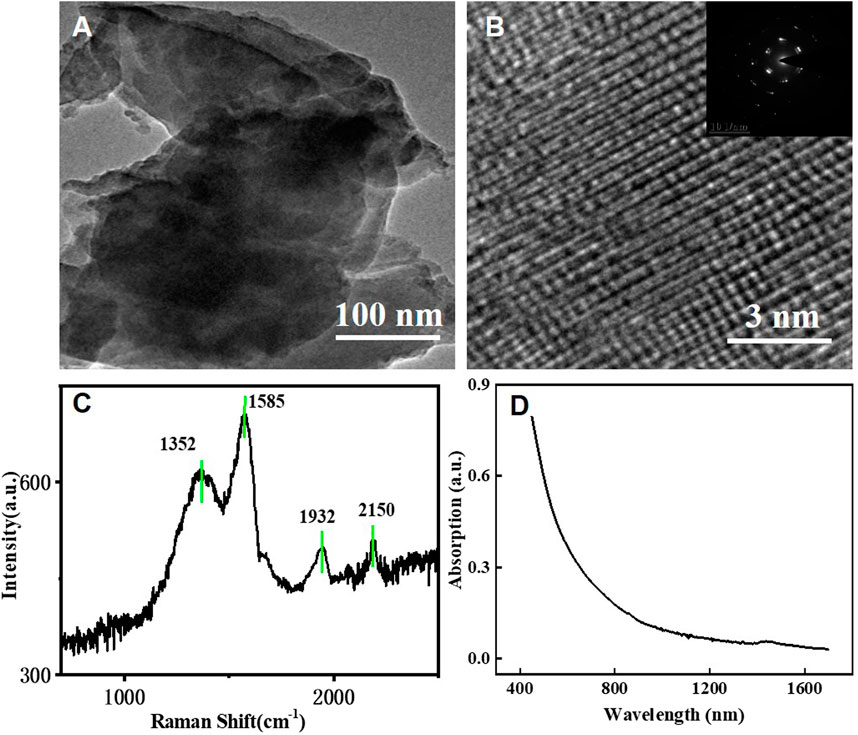
FIGURE 1. Characterizations of GDY. (A) Transmission electron microcopy (TEM) images of GDY nanosheets, (B) atomic lattice structure of GDY via high-resolution transmission electron microscopy (HRTEM) nanosheets, (C) Raman spectrum, and (D) linear absorption spectrum of GDY dispersions.
To investigate the nonlinear absorption (NLA) property of GDY, the open-aperture (OA) Z-scan technique was applied by a nanosecond fiber laser operating at 1,064 nm. The OA Z-scan curve of the GDY is shown in Figure 2A. The normalized transmittance increases as the GDY approaches the focal position (z = 0), and the peak of the curves increase with the increase in the incident power of the laser. These curves show the typical saturable absorption (SA) characteristic [43]. The relationship between normalized transmittance of GDY dispersions and input peak intensity is shown in Figure 2B. The saturation intensity and modulation depth are basic parameters used to evaluate the suitability of 2D materials. The most common SA model of single-photon absorption was used to fit the relationship between T(I) and input peak intensity, shown as follows [44]:
where As, I, Is, and Ans are the modulation depth, incident light intensity, saturable intensity, and non-saturable loss, respectively. As and Is are fitted to be 22.19% and 282.1 kW/cm2, respectively.
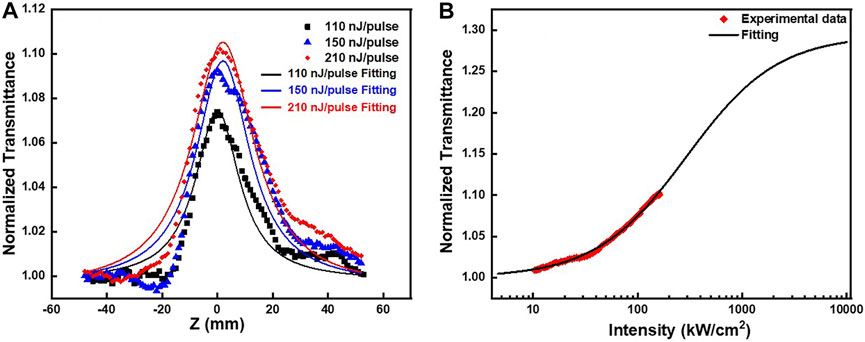
FIGURE 2. Nonlinear optical characterization of GDY. (A) Open-aperture Z-scan results of GDY nanosheet dispersions at 1,064 nm. (B) Relationship between normalized transmittance of GDY dispersions and input peak intensity.
Results and Discussion
Saturable Absorption Behavior of GDY-Coated Microfiber
In this work, we prepared a GDY-coated microfiber structure by the optical-deposited approach used in Ref. [18]. First, by extending a part of the standard single-mode fiber (SMF-28) with flame heating, the microfiber with a diameter of 1.5 μm was manufactured. Then, we injected a 1,550 nm continuous light with 10 mW power into the prepared microfiber and dropped GDY dispersions onto the microfiber surface. To obtain better optical deposition results, we diluted the concentration of dispersions from 0.05 to 0.01 mg mL−1. Afterward, due to the gradient force of the evanescent field, GDY would be gradually absorbed to the surface of the microfiber. Finally, the fabricated GDY-coated microfiber is shown in Figure 3B. We found that the deposition length of GDY is about 170 μm, and the transmittance of the device is about 4%. We measured the input power-dependent transmittance of the GDY-coated microfiber through a 1,064 nm nanosecond pulse laser to study the nonlinear SA effect. The schematic diagram of the measurement setup is shown in Figure 3A. A nanosecond pulse laser (Aaoxiu ASNSFL-1064–500-FA-B) was first congregated by using a variable optical attenuator (VOA) for the purpose of constantly adjusting the optical power injected into the sample. The pulse width and repetition rate are set as 3 ns and 5 kHz, respectively. The pulse pump light was split into two parts by using a 10:90 optical coupler (OC); the 10% part was employed to monitor the input power (measured by photodetector 1 and optical power meter 1, PD1/OPM1), while the 90% part was injected into the GDY-coated microfiber sample measured by PD2 and OPM2. By gradually adjusting the VOA, the measured transmittance of the sample is shown in Figure 3C, which exhibits an SA effect. A modulation depth (MD) was fitted to 4.06% from the measurement result. The SA effect is mainly generated by GDY materials, which shows a capability to realize all-optical modulation combining with the microfiber.
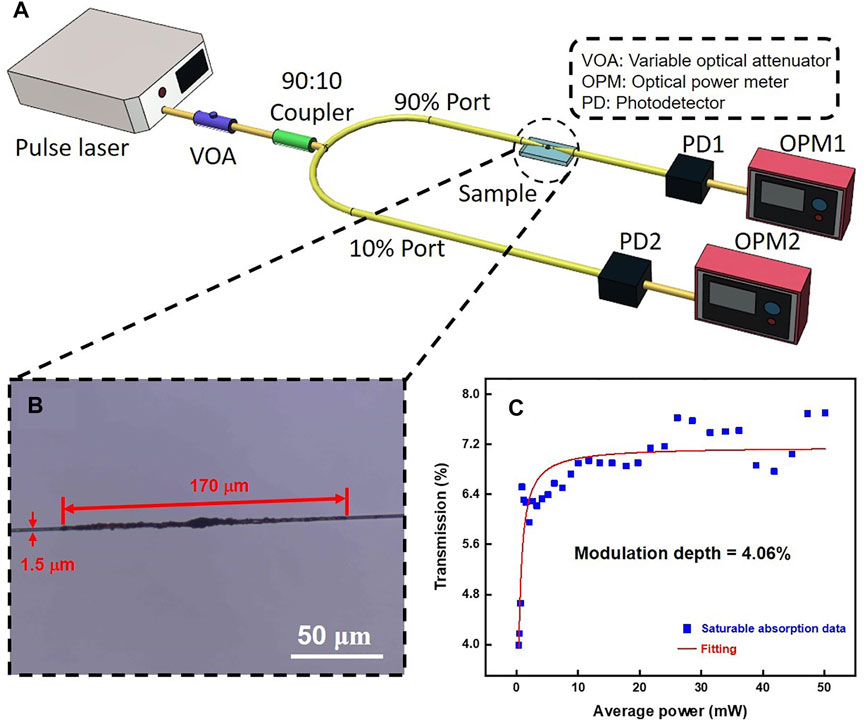
FIGURE 3. (A) Sketch diagram of the measurement system for GDY-coated microfiber SA effect, (B) optical microscope image of the GDY-coated microfiber, and (C) saturated absorption characteristic curve of the GDY-coated microfiber.
Ultrafast All-Optical Modulator of GDY
The GDY-coated microfiber exhibits an SA effect, which shows that all-optical modulation for the sample might be feasible. Theoretically, the mechanism of modulation based on saturable absorption can be described as follows: According to Pauli blocking, if the intensity of pump light is adequately strong, GDY can generate an SA effect and become transparent. Successively, the signal can pass through and show temporal modulation. The modulation speed is determined by the time of the excited carriers relaxing in GDY [24]. Therefore, the all-optical modulation experiment and its result are studied and shown in Figure 4. The sketch diagram of the experiment system is shown in Figure 4A. The nanosecond laser (3 ns/5 kHz) is used as a pump light to modulate the CW light (signal light, centered at 1550 nm). The VOA is employed to control the power of the pump light. A 10:90 OC was used to divide the pump light into two parts: the 10% part was used to observe the waveform of the pump power, which was measured by PD2 (Thorlabs DET08CFC/M) and an oscilloscope (Tektronix MDO 3014), and the 90% part was combined with CW light via a wavelength division multiplexer (WDM) and then coupled into the GDY-coated microfiber. After the sample, an optical band-pass filter (BPF, centered wavelength of 1,550 nm and a bandwidth of 0.25 nm) was used to filter out the pump light. Finally, the PD1 and oscilloscope were employed to measure the modulated signals. The pump light and signal light were turned on simultaneously, the signal light power was set to 0.4 mW, and the pump light power was increased from 0 to 4 mW for the modulation to occur. Figure 4B shows the measured experimental results of the all-optical modulation.
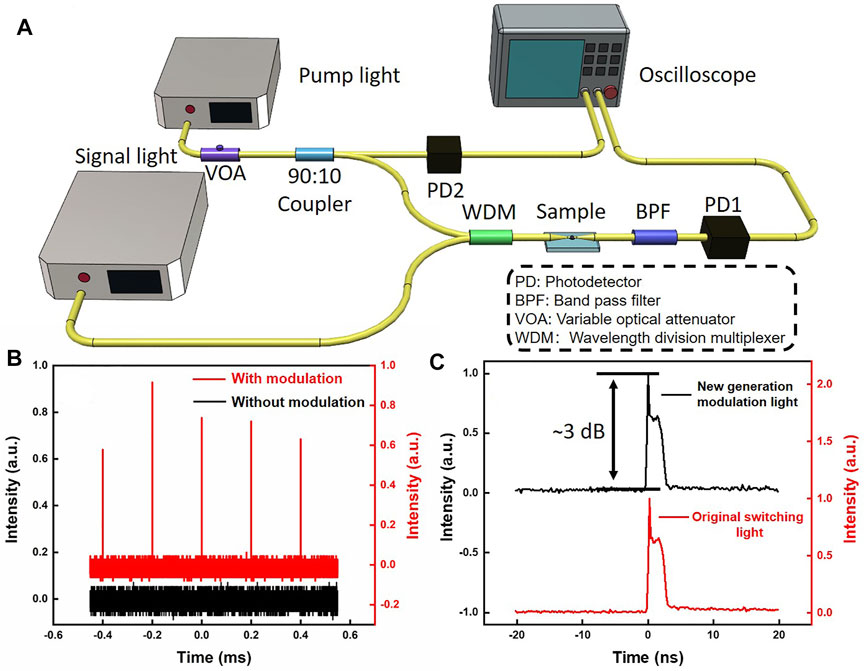
FIGURE 4. (A) Schematic diagram of the experiment setup for all-optical modulation. (B) Waveforms of the output light after the band pass filter (BPF) with CW light on or without CW light. (C) Single-pulse waveform comparison between the original pump/switching light and the new modulated light.
It is worth noting that to exclude the pump power leak induced by BPF limits, a simple way was used to implement in this experiment as follows: If the pump light was kept on and the signal light was turned off, the oscilloscope cannot detect any pulse signal, as shown in the black curve of Figure 4B; if both pump light and signal light were turned on, the modulation pulse signal is detected, as shown in the red curve of Figure 4B. To further study the pulse quality of modulated CW light, the single-pulse waveform between the pump light and the modulated CW light were compared, as shown in Figure 4C. It can be seen that the modulated signal light is highly similar to the original pump light but with a slightly broadened pulse (from 3 to 4 ns). The similar pulse waveform and the same pulse repetition rate between the modulated light and the pump light demonstrated the success of the modulation.
Performance Discussions of GDY All-Optical Modulator
Table 1 summarizes the comparison among different kinds of nonlinear all-optical modulators with typical 2D materials. We can easily observe that the SA modulator had an absolutely fast modulation speed but a relative low MD compared with other types of modulators [11, 18]. In our work, the modulator shows a 4 ns modulation pulse in 5 kHz and 3 dB MD. A higher pump power may induce the damage of the modulator. Therefore, the maximum MD of our modulator is measured by 3 dB. Otherwise, the microfiber diameter can directly influence the evanescent field energy. The smaller the diameter, the larger the evanescent field overflow ratio. In our experiment, the microfiber diameter is similar to that in Refs. [5, 8], in which the evanescent field energy at this diameter can achieve 20% of input power in the fiber [5] and make it conducive to the interaction between GDY and the evanescent field. The modulator shows 4 mW power consumption, which is relatively lower than BP and graphene optical Kerr effect (OKE) modulators. Also, our modulator exhibited a comparable modulation speed with graphene OKE and BP modulator in the nanosecond scale but much lower than the graphene SA modulator. That was properly limited by the pulse width of the pump light and sensitivity of the detectors. According to the Paul blocking principle, the modulation speed of the SA modulator is limited by the carrier relaxation time of 2D materials theoretically. Thus, the experimental modulation speed in our work does not achieve its maximum modulation speed. The carrier relaxation time of such types of 2D materials is shown in Table 1. The result shows that GDY as an SA modulator is expected to have a faster modulation rate than graphene. Therefore, it is worth studying further the high modulation speed of the GDY SA modulator. Our work preliminarily proves the feasibility of GDY as an SA modulator in experiments and provides experimental supports for the faster GDY SA modulator research.
Conclusion
In conclusion, we demonstrated a GDY-coated microfiber temporal modulator based on saturable absorption for the first time. By employing the SA effect of GDY, the CW light of 1,550 nm was successfully modulated by the pulse light of 1,064 nm. The modulated light had a pulse duration of 4 ns and a repetition frequency of 5 kHz. The results showed that GDY could be used in all-optical modulators and pave a way for the research of GDY in all-optical information processing applications.
Data Availability Statement
The original contributions presented in the study are included in the article/Supplementary Material, further inquiries can be directed to the corresponding author.
Author Contributions
KZ, ZQ, and SH have contributed equally to this work. SL and KZ contributed to conception and design of the study. KZ and ZQ organized the database. SH and ZQ performed the statistical analysis. KZ wrote the first draft of the manuscript. KZ, SH, ZQ, and WB wrote sections of the manuscript. All authors contributed to manuscript revision, read, and approved the submitted version.
Funding
The authors are grateful for financial support from the China Postdoctoral Science Foundation (2020M672763) and the Science and Technology Innovation Commission of Shenzhen (JCYJ20170302153323978 and JCYJ20170410171958839).
Conflict of Interest
The authors declare that the research was conducted in the absence of any commercial or financial relationships that could be construed as a potential conflict of interest.
Publisher’s Note
All claims expressed in this article are solely those of the authors and do not necessarily represent those of their affiliated organizations, or those of the publisher, the editors, and the reviewers. Any product that may be evaluated in this article, or claim that may be made by its manufacturer, is not guaranteed or endorsed by the publisher.
Acknowledgments
The authors thank Dr. Cong Wang for his valuable advice.
References
1. Yang H, Wang Y, Tiu ZC, Tan SJ, Yuan L, Zhang H. All-Optical Modulation Technology Based on 2D Layered Materials. Micromachines (2022) 13(1):92. doi:10.3390/mi13010092
2. Zhang A, Wang Z, Ouyang H, Lyu W, Sun J, Cheng Y, et al. Recent Progress of Two-Dimensional Materials for Ultrafast Photonics. Nanomaterials (2021) 11(7):1778. doi:10.3390/nano11071778
3. Liu W, Liu M, Liu X, Wang X, Deng HX, Lei M, et al. Recent Advances of 2D Materials in Nonlinear Photonics and Fiber Lasers. Adv Opt Mater. (2020) 8(8):1901631. doi:10.1002/adom.201901631
4. Liu M, Wei Z-W, Luo A-P, Xu W-C, Luo Z-C. Recent Progress on Applications of 2D Material-Decorated Microfiber Photonic Devices in Pulse Shaping and All-Optical Signal Processing. Nanophotonics (2020) 9(9):2641–71. doi:10.1515/nanoph-2019-0564
5. Li E, Jiang M, Li D, Wang R, Kang X, Wang T, et al. All-optical Ti3C2Tx Modulator Based on a sandwich Structure. Appl Opt (2022) 61(4):925–30. doi:10.1364/AO.445975
6. Zheng J, Tang X, Yang Z, Liang Z, Chen Y, Wang K, et al. Few-Layer Phosphorene-Decorated Microfiber for All-Optical Thresholding and Optical Modulation. Adv Opt Mater (2017) 5(9):1700026. doi:10.1002/adom.201700026
7. Li W, Chen B, Meng C, Fang W, Xiao Y, Li X, et al. Ultrafast All-Optical Graphene Modulator. Nano Lett (2014) 14(2):955–9. doi:10.1021/nl404356t
8. Zhu J, Cheng X, Liu Y, Wang R, Jiang M, Li D, et al. Stimulated Brillouin Scattering Induced All-Optical Modulation in Graphene Microfiber. Photon Res (2019) 7(1):8–13. doi:10.1364/PRJ.7.000008
9. Zhang D, Guan H, Zhu W, Yu J, Lu H, Qiu W, et al. All Light-Control-Light Properties of Molybdenum Diselenide (MoSe_2)-Coated-Microfiber. Opt Express (2017) 25(23):28536–46. doi:10.1364/OE.25.028536
10. Li D, Chen Z, Chen G, Hu S, Wang Y, Qiu W, et al. Reduced Graphene Oxide Wrapped on Microfiber and its Light-Control-Light Characteristics. Opt Express (2017) 25(5):5415–25. doi:10.1364/OE.25.005415
11. Yu S, Wu X, Chen K, Chen B, Guo X, Dai D, et al. All-Optical Graphene Modulator Based on Optical Kerr Phase Shift. Optica (2016) 3(5):541–4. doi:10.1364/optica.3.000541
12. Wu L, Dong Y, Zhao J, Ma D, Huang W, Zhang Y, et al. Kerr Nonlinearity in 2D Graphdiyne for Passive Photonic Diodes. Adv Mater (2019) 31(14):1807981. doi:10.1002/adma.201807981
13. Wu L, Jiang X, Zhao J, Liang W, Li Z, Huang W, et al. MXene‐Based Nonlinear Optical Information Converter for All‐Optical Modulator and Switcher. Laser Photon Rev (2018) 12(12):1800215. doi:10.1002/lpor.201800215
14. Shi B, Miao L, Wang Q, Du J, Tang P, Liu J, et al. Broadband Ultrafast Spatial Self-phase Modulation for Topological Insulator Bi2Te3 Dispersions. Appl Phys Lett (2015) 107(15):151101. doi:10.1063/1.4932590
15. Zhang J, Yu X, Han W, Lv B, Li X, Xiao S, et al. Broadband Spatial Self-phase Modulation of Black Phosphorous. Opt Lett (2016) 41(8):1704–7. doi:10.1364/ol.41.001704
16. Zhang K, Bao W, Chen X, Lu S. Graphdiyne-Deposited Microfiber Structure All-Optical Modulator at the Telecommunication Band. Opt Express (2021) 29(23):38915–23. doi:10.1364/oe.443217
17. Zhang Z, Chen G, Yang M, Ou Y, Luo L, Lu D, et al. Resonance-enhanced All-Optical Modulation of WSe2-Based Micro-resonator. Nanophotonics (2019) 9(8):2387–96. doi:10.1515/nanoph-2019-0425
18. Wang Y, Zhang F, Tang X, Chen X, Chen Y, Huang W, et al. All-Optical Phosphorene Phase Modulator with Enhanced Stability under Ambient Conditions. Laser Photon Rev (2018) 12(6):1800016. doi:10.1002/lpor.201800016
19. Gan X, Zhao C, Wang Y, Mao D, Fang L, Han L, et al. Graphene-Assisted All-Fiber Phase Shifter and Switching. Optica (2015) 2(5):468–71. doi:10.1364/optica.2.000468
20. Tan SJ, Ahmad H, Tiu ZC. All‐fibre Phase Shifter Based on Tapered Fibre Coated with MoWS 2 ‐rGO. IET Optoelectronics (2021) 15(6):264–9. doi:10.1049/ote2.12042
21. Ahmad H, Lim HS, MatJafri MZ, Ge YQ, Zhang H, Tiu ZC. All-fiber Optical Polarization Modulation System Using MoS2 as Modulator. Infrared Phys Techn (2019) 102:103002. doi:10.1016/j.infrared.2019.103002
22. Dawlaty JM, Shivaraman S, Chandrashekhar M, Rana F, Spencer MG. Measurement of Ultrafast Carrier Dynamics in Epitaxial Graphene. Appl Phys Lett (2008) 92(4):042116. doi:10.1063/1.2837539
23. Wang K, Szydłowska BM, Wang G, Zhang X, Wang JJ, Magan JJ, et al. Ultrafast Nonlinear Excitation Dynamics of Black Phosphorus Nanosheets from Visible to Mid-infrared. ACS Nano (2016) 10(7):6923–32. doi:10.1021/acsnano.6b02770
24. Guo J, Shi R, Wang R, Wang Y, Zhang F, Wang C, et al. Graphdiyne‐Polymer Nanocomposite as a Broadband and Robust Saturable Absorber for Ultrafast Photonics. Laser Photon Rev (2020) 14(4):1900367. doi:10.1002/lpor.201900367
25. Wang C, Xu J, Wang Y, Song Y, Guo J, Huang W, et al. MXene (Ti2NTx): Synthesis, Characteristics and Application as a Thermo-Optical Switcher for All-Optical Wavelength Tuning Laser. Sci China Mater (2021) 64(1):259–65. doi:10.1007/s40843-020-1409-7
26. Cheng JL, Vermeulen N, Sipe JE. Third Order Optical Nonlinearity of Graphene. New J Phys (2014) 16:053014. doi:10.1088/1367-2630/16/5/053014
27. Zhang M, Wu Q, Zhang F, Chen L, Jin X, Hu Y, et al. 2d Black Phosphorus Saturable Absorbers for Ultrafast Photonics. Adv Opt Mater (2019) 7(1):1800224. doi:10.1002/adom.201800224
28. Guo J, Wang Z, Shi R, Zhang Y, He Z, Gao L, et al. Graphdiyne as a Promising Mid‐Infrared Nonlinear Optical Material for Ultrafast Photonics. Adv Opt Mater. (2020) 8(10):2000067. doi:10.1002/adom.202000067
29. Xu J, Qian X. Graphdiyne: Electronics, Thermoelectrics, and Magnetism Applications. In: Graphdiyne: Fundamentals and Applications in Renewable Energy and Electronics. (2022). Weinheim, Germany: Wiley-VCH. p. 315–39. doi:10.1002/9783527828470.ch8
30. Gao X, Liu H, Wang D, Zhang J. Graphdiyne: Synthesis, Properties, and Applications. Chem Soc Rev (2019) 48(3):908–36. doi:10.1039/c8cs00773j
31. Huang C, Li Y, Wang N, Xue Y, Zuo Z, Liu H, et al. Progress in Research into 2D Graphdiyne-Based Materials. Chem Rev (2018) 118(16):7744–803. doi:10.1021/acs.chemrev.8b00288
32. Jiao Y, Du A, Hankel M, Zhu Z, Rudolph V, Smith SC. Graphdiyne: A Versatile Nanomaterial for Electronics and Hydrogen Purification. Chem Commun (2011) 47(43):11843–5. doi:10.1039/c1cc15129k
33. Du Y, Zhou W, Gao J, Pan X, Li Y. Fundament and Application of Graphdiyne in Electrochemical Energy. Acc Chem Res (2020) 53(2):459–69. doi:10.1021/acs.accounts.9b00558
34. Zuo Z, Li Y. Emerging Electrochemical Energy Applications of Graphdiyne. Joule (2019) 3(4):899–903. doi:10.1016/j.joule.2019.01.016
35. Hao X, Hu F, Gu Y, Yang H, Li C, Guo C. Molecularly Assembled Graphdiyne with Atomic Sites for Ultrafast and Real-Time Detection of Nitric Oxide in Cell Assays. Biosens Bioelectron (2022) 195:113630. doi:10.1016/j.bios.2021.113630
36. Liu J, Chen C, Zhao Y. Progress and Prospects of Graphdiyne‐Based Materials in Biomedical Applications. Adv Mater (2019) 31(42):1804386. doi:10.1002/adma.201804386
37. Zhang Y, Huang P, Guo J, Shi R, Huang W, Shi Z, et al. Graphdiyne‐Based Flexible Photodetectors with High Responsivity and Detectivity. Adv Mater (2020) 32(23):2001082. doi:10.1002/adma.202001082
38. Jin Z, Zhou Q, Chen Y, Mao P, Li H, Liu H, et al. Graphdiyne:ZnO Nanocomposites for High‐Performance UV Photodetectors. Adv Mater (2016) 28(19):3697–702. doi:10.1002/adma.201600354
39. Jin X, Bao W, Zhang H, Zheng Z, Zhang M. Four-Wave Mixing in Graphdiyne-Microfiber Based on Synchronized Dual-Wavelength Pulses. Photon Res (2022) 10(2):503. doi:10.1364/PRJ.444938
40. Ma W, Yin P, Li M, Sui L, Wang T, Liu Z, et al. Graphdiyne-Decorated Microfiber Based Soliton and Noise-like Pulse Generation. Nanophotonics (2021) 10(16):3967–77. doi:10.1515/nanoph-2021-0299
41. Li G, Li Y, Liu H, Guo Y, Li Y, Zhu D. Architecture of Graphdiyne Nanoscale Films. Chem Commun (2010) 46(19):3256–8. doi:10.1039/b922733d
42. Guo Z, Zhang H, Lu S, Wang Z, Tang S, Shao J, et al. From Black Phosphorus to Phosphorene: Basic Solvent Exfoliation, Evolution of Raman Scattering, and Applications to Ultrafast Photonics. Adv Funct Mater (2015) 25(45):6996–7002. doi:10.1002/adfm.201502902
43. Sheik-Bahae M, Said AA, Wei T-H, Hagan DJ, Van Stryland EW. Sensitive Measurement of Optical Nonlinearities Using a Single Beam. IEEE J Quan Electron. (1990) 26(4):760–9. doi:10.1109/3.53394
Keywords: graphdiyne, saturable absorption, all-optical modulator, microfiber, two-dimensional materials
Citation: Zhang K, Qiu Z, He S, Bao W and Lu S (2022) Graphdiyne-Coated Microfiber All-Optical Temporal Modulator Based on Saturable Absorption. Front. Phys. 10:893023. doi: 10.3389/fphy.2022.893023
Received: 09 March 2022; Accepted: 21 March 2022;
Published: 05 May 2022.
Edited by:
Yunzheng Wang, Singapore University of Technology and Design, SingaporeReviewed by:
Zian Cheak Tiu, INTI International University, MalaysiaCong Wang, Technical University of Denmark, Denmark
Qing Wu, Harbin University of Science and Technology, China
Copyright © 2022 Zhang, Qiu, He, Bao and Lu. This is an open-access article distributed under the terms of the Creative Commons Attribution License (CC BY). The use, distribution or reproduction in other forums is permitted, provided the original author(s) and the copyright owner(s) are credited and that the original publication in this journal is cited, in accordance with accepted academic practice. No use, distribution or reproduction is permitted which does not comply with these terms.
*Correspondence: Shunbin Lu, c2h1bmJpbl9sdUBzenUuZWR1LmNu