- Department of Physics, Faculty of Science, National University of Singapore, Singapore
After the reward of more than 2 decades of pursuit on the high-Tc cuprate analog with the hope to obtain a better understanding of the mechanism of high-Tc superconductivity, the discovery of superconductivity in the infinite-layer nickelate brings more mystery to the picture than expected. Tops in the list of questions are perhaps 1) absence of superconductivity in the bulk nickelate and limited thickness of the infinite-layer phase in thin film, 2) absence of superconductivity in the La-nickelate despite it being the earliest studied rare-earth nickelate, and the role of 4
Main Text
Around 4 decades ago, the witness of superconductivity above 30 K redefined preexisting knowledge on the mechanism of superconductivity and restructured the landscape of the playground on superconductor materials [1, 2]. Understanding the high-temperature (high-Tc) superconductivity has since been one of the holy grails in physics. Several characteristic properties were discussed in the cuprate superconductor: 1) quasi-2D CuO2 square-planar lattice, 2) antiferromagnetic order and superexchange interaction, 3) spin
Thickness Dependence
Stabilization of Infinite-Layer Phase
Since the observation of superconductivity in the infinite-layer nickelate Nd0.8Sr0.2NiO2 thin film [14], apparent challenges have emerged in material synthesis [25]. On top of low reproducibility and difficulty in the fabrication of superconducting doped infinite-layer structure by many experimental groups [38, 39], a hard-nut-to-crack issue is a limited thickness from the substrate interface, which the infinite-layer phase can be stabilized [25, 26, 40]. Above ∼10 nm from the substrate interface, obvious secondary phases or off-tilt structures form instead of the infinite-layer or partially reduced perovskite phase [25]. In many cases, growing a thick film will lead to the formation of a secondary phase even at just ∼5 nm from the substrate (Figure 1A) [26]. On the other hand, if a thinner <10 nm film is grown, the entire film can be fully reduced with no observation of secondary phase even at the film surface (Figures 1C,D), except for possibly Ruddlesden-Popper (RP) stacking fault at some regions of the film (Figure 1D). The obvious strategy for obtaining the purest possible infinite-layer phase is fabricating thin films below 10 nm. In addition to the absence of secondary phases as shown in the STEM image, atomically flat surfaces with terrace-like topography can be observed on the film surface before and after topotactic reduction, as shown in the atomic-force-microscopy images in Figure 1E. Some reports suggested using SrTiO3 (STO) capping layer on top of the nickelate thin film prior to a topotactic reduction that can serve as a “backbone” to help stabilize the infinite-layer phase during topotactic reduction from the perovskite phase [25, 41]. However, such a method has not led to a thicker infinite-layer phase of >10 nm. Lattice coherency of a crystalline thin film can be seen from the X-ray diffraction (XRD) Laue fringes which originate from the constructive interference between perfect lattice layers of the thin film. To date, most reported XRD data of the perovskite phase of the doped nickelate thin film has clear Laue fringes in the vicinity of the (002) peak; however, Laue fringes are typically absent for the reduced doped infinite-layer nickelate (002) peak [16, 26, 41–43]. This may suggest the presence of nonstoichiometric oxygen at random parts of the reduced infinite-layer thin film. Significant development of secondary or perovskite phases is typically avoided after optimization in film growth conditions since no perovskite peak or defect phase peak is seen in the XRD curve.
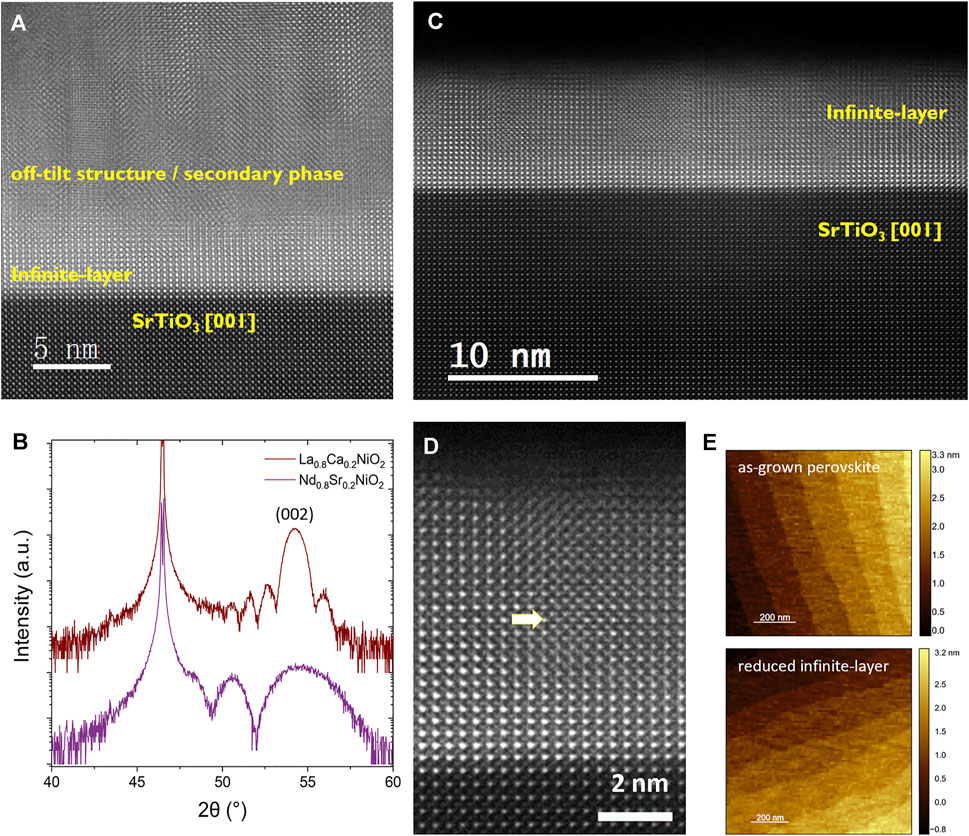
FIGURE 1. Structural properties of the reduced infinite-layer nickelate thin film. (A,C,D) STEM HAADF images. (B) XRD (002) pattern. (A) A thick 30 nm Nd0.85Sr0.15NiO2 film has infinite-layer phase stabilized up to ∼5 nm from the substrate interface. Above 5 nm, secondary phase or off-tilt structure can be seen, data is adapted from [26]. Entire Nd0.8Sr0.2NiO2 film of thinner <10 nm can be reduced to infinite-layer phase with perfect crystallinity (C,D) up to the surface, with no sign of secondary phase except for possible RP stacking fault occurs at some part of the film (D). (B) A clear Laue fringes can be observed for 8 nm Nd0.8Sr0.2NiO2 and 15 nm La0.8Ca0.2NiO2 thin films, indicating coherent infinite-layer phase. (E) Atomic-force-microscopy (AFM) images show the atomically flat terrace-like surface topography in Nd0.8Sr0.2NiO2 (6.5 nm) film surfaces before (top) and after (bottom) topotactic reduction done in the PLD chamber.
The challenge of obtaining a coherent infinite-layer phase does not affect transport-related study since zero resistance can be observed even when only a small part of the film is superconducting. Unfortunately, the same cannot be said for measurements requiring a pure phase with coherent crystallinity, especially at the film surface, such as the Angle-resolved Photoemission Spectroscopy (ARPES) or Scanning Tunneling Spectroscopy (STS). Many probing techniques which reveal crucial aspects of the superconductivity in nickelate cannot be carried out because of the lack of coherent lattice and purity in the infinite-layer phase, especially on the top surface. With much effort in optimizing film quality, we recently reported an observation of clear Laue fringes in the vicinity of XRD (002) peak of the infinite-layer phase for Nd1-xSrxNiO2 and La1-xCaxNiO2 (Figure 1B). Especially in the case of superconducting lanthanide infinite-layer nickelate, more than 30 unit-cells (uc) of a coherent infinite-layer lattice can be seen vividly from the XRD Laue fringes.
Thickness Dependency and Role of Strain and Interface
Superconductivity is missing in the bulk infinite-layer nickelate [23, 44–46]. The puzzling limitation in the thickness of the infinite-layer thin film further warrants the importance of investigating the thickness dependency of the physical observables and electronic structure of the infinite-layer nickelate. The zero-resistivity critical temperature
Rare-earth Dependence
Doping Dependent Phase Diagram
The barium (Ba) hole-doped lanthanide La-cuprate La2-xBaxCuO4 was the first high-Tc cuprate synthesized, which kicked off the door to high-temperature superconductivity beyond the BCS paradigm [27]. To mimic cuprate’s square-planar structure and 3
Figure 2A shows doping-dependent superconducting dome and phase diagram in various Sr-doped and Ca-doped rare-earth (La, Pr, Nd) infinite-layer nickelate synthesized so far [15, 16, 26, 40, 41]. The first eye-catching feature is the presence of “dip” with a lower Tc for a particular doping in the Nd1-xSrxNiO2 [26, 40] and La1-xSrxNiO2 [41] thin films. Given the lack of consistency of the “dip” feature on a particular doping across different rare-earth nickelates and reports, it is presently unclear whether it is an experimental artifact or a reminiscence of certain quantum critical transitions in the system. The second observation is the expansion of the superconducting dome to a higher doping level from Nd1-xSrxNiO2, Pr1-xSrxNiO2 to La1-xCaxNiO2. Given the increased difficulty in synthesizing infinite-layer nickelate thin film at larger doping [25, 41], such observation may have a certain correlation to film crystallinity. The perovskite nickelate has a smaller in-plane lattice constant than the SrTiO3 (001) substrate, which the lattice mismatches are decreasing from NdNiO3 to LaNiO3. It has been established that a perovskite phase nickelate with good crystallinity is essential to the success in topotactic reduction to the infinite-layer phase [25]. One may suggest that perovskite La1-xCaxNiO3 and Pr1-xSrxNiO3 can be grown to have better crystallinity than the Nd1-xSrxNiO3 at a large doping regime. A similar argument could be made for the difference in superconducting dome between La1-xCaxNiO2 and La1-xSrxNiO2. The Ca2+ ion is of more similar size to the rare-earth La3+, Pr3+ and Nd3+ ions than the larger Sr2+ ion [16]. Regardless, it is also possible that different rare-earth ion and cation doping leads to a slight difference in the electronic band structure of the infinite-layer nickelate, causing a different span of superconducting domes. Another note is, so far, the doping level in the fabricated thin films is expected to follow the stoichiometry of the polycrystalline target used in the pulsed-laser-deposition (PLD) growth. It is not warranted that the doping level is accurate, and further investigation on the stoichiometry of the doped thin film shall be carried out. The third observation is the correlation between the size of the rare-earth ions and superconducting transition temperature Tc. The maximum onset temperature in resistivity, where resistivity reaches 90% of its value at 20 K, Tc,90% is roughly consistent among various reports, where La-nickelate has Tc,90% ∼9–10 K while Pr- and Nd-nickelate has Tc,90% ∼12–15 K. It is routinely explained by the increase in electronic bandwidth for a smaller rare-earth ion [14, 16].
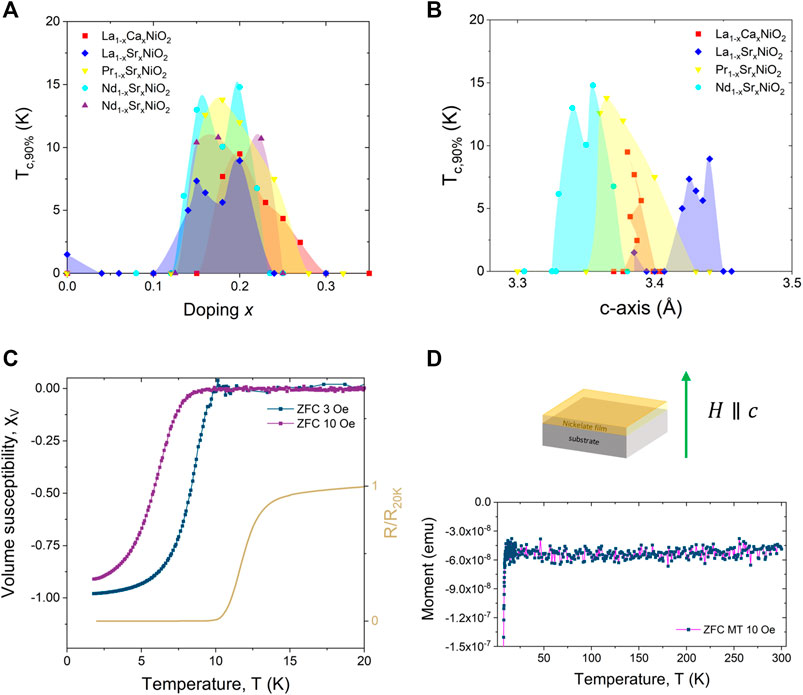
FIGURE 2. (A,B) Superconducting phase diagram of various hole doped infinite-layer nickelates, plotted as a function of hole doping levels
In addition to the difference in the superconducting Tc between La- and (Pr/Nd)- infinite-layer nickelate, the Hall coefficient (
Relevance to Lattice Constant
Since superconductivity has not been observed in the bulk infinite-layer nickelate despite that high crystallinity samples were made [44, 45], the ab-axis lattice constants of the superconducting infinite-layer thin film are epitaxially constrained to the substrate in-plane lattice constants. It might be intuitive to look for any correlation between the c-axis lattice dimension and superconducting dome of the infinite-layer nickelate (Figure 2B). At first glance, the superconducting dome is generally limited to between 3.32 Å and 3.45 Å. However, the superconducting domes between various rare-earth compounds do not completely overlap. Also, for the case of La1-xCaxNiO2, the variation in c-axis lattice constant across doping is small due to a very similar ionic size between Ca2+ ion and La3+, and has slight sample-to-sample variation at the same Ca doping [16]. It seems early to suggest any strict correlation between the superconducting dome of infinite-layer nickelate and the c-axis lattice dimension. It is worth noting that an enhancement in onset Tc was realized experimentally by applying external pressure [17], which suggests the likelihood of further increasing Tc by simulating chemical pressure through tuning in-plane lattice constants, rare-earth ions size, or dopant size. In addition, we note that there are recent theoretical calculations on the effect of in-plane lattice constant and epitaxial strain in tuning the P4/mmm - I4/mcm phase transition in RNiO2 (R = La, Pr, Nd, Eu-Lu, Y) [56, 57].
Observation of Meissner Effect
Two main phenomena characterize superconductivity: 1) zero electrical resistivity, 2) Meissner effect. The first experimental report of the discovery of superconductivity in Nd0.8Sr0.2NiO2 thin film provided multiple resistivity-temperature (
Meissner effect is routinely seen in the magnetic susceptibility measurement: 1) a negative slope in the
The superconducting transition temperature observed in the
Pairing Symmetry
Dominant d-wave Gap
The infinite-layer nickelate is a sister of the high-Tc cuprate, which hosts a dominant
Considering the role of nickelate superconductivity in illuminating the origin of high-Tc superconductivity in cuprates, a detailed experimental study on the pairing symmetry of nickelate is crucial. The first experimental report on the superconducting gap symmetry of the infinite-layer nickelate is a single-particle-tunneling experiment on Nd0.8Sr0.2NiO2 film surface which detected signals correspond to
Fully Gapped Pairing and Isotropic Upper Critical Field
While the tunneling experiment did not lead to a complete answer of the nickelate’s pairing order, the existence of a fully gapped
Data Availability Statement
The original contributions presented in the study are included in the article/Supplementary Material, further inquiries can be directed to the corresponding author.
Author Contributions
All authors listed have made a substantial, direct, and intellectual contribution to the work and approved it for publication.
Funding
This research is supported by the Agency for Science, Technology, and Research (A*STAR) under its Advanced Manufacturing and Engineering (AME) Individual Research Grant (IRG) (A1983c0034) and the Singapore National Research Foundation (NRF) under the Competitive Research Programs (CRP Grant No. NRF-CRP15-2015-01) and by the Ministry of Education, Singapore, under its MOE Tier 2 grant (Grant no. MOE-T2EP50121-0018).
Conflict of Interest
The authors declare that the research was conducted in the absence of any commercial or financial relationships that could be construed as a potential conflict of interest.
Publisher’s Note
All claims expressed in this article are solely those of the authors and do not necessarily represent those of their affiliated organizations, or those of the publisher, the editors and the reviewers. Any product that may be evaluated in this article, or claim that may be made by its manufacturer, is not guaranteed or endorsed by the publisher.
Acknowledgments
We thank S. W. Zeng for the fruitful discussion and revision of this article. We acknowledge Elbert E. M. Chia, C. Z. Diao, W. Escoffier, M. Goiran, S. K. Goh, J. X. Hu, H. Jani, Z. S. Lim, C. J. Li, P. Nandi, G. J. Omar, M. Pierre, D. Preziosi, S. K. Sudheesh, M. Salluzzo, C. S. Tang, Andrew T. S. Wee, K. Y. Yip, X. M. Yin, P. Yang, Z. T. Zhang for discussions.
REFERENCES
1. Wu MK, Ashburn JR, Torng CJ, Hor PH, Meng RL, Gao L, et al. Superconductivity at 93 K in a New Mixed-phase Y-Ba-Cu-O Compound System at Ambient Pressure. Phys Rev Lett (1987) 58:908–10. doi:10.1103/physrevlett.58.908
2. Azuma M, Hiroi Z, Takano M, Bando Y, Takeda Y. Superconductivity at 110 K in the Infinite-Layer Compound (Sr1-xCax)1-yCuO2. Nature (1992) 356:775–6. doi:10.1038/356775a0
3. Keimer B, Kivelson SA, Norman MR, Uchida S, Zaanen J. From Quantum Matter to High-Temperature Superconductivity in Copper Oxides. Nature (2015) 518:179–86. doi:10.1038/nature14165
4. Botana AS, Bernardini F, Cano A. Nickelate Superconductors: An Ongoing Dialog between Theory and Experiments. J Exp Theor Phys (2021) 132:618–27. doi:10.1134/s1063776121040026
5. Jiang M, Berciu M, Sawatzky GA. Critical Nature of the Ni Spin State in Doped NdNiO2. Phys Rev Lett (2020) 124:207004. doi:10.1103/physrevlett.124.207004
6. Pickett WE. The Dawn of the Nickel Age of Superconductivity. Nat Rev Phys (2021) 3:7–8. doi:10.1038/s42254-020-00257-3
7. Botana AS, Norman MR. Similarities and Differences between LaNiO2 and CaCuO2 and Implications for Superconductivity. Phys Rev X (2020) 10:011024. doi:10.1103/physrevx.10.011024
8. Rice TM. Electronic Structure of Possible Nickelate Analogs to the Cuprates. Phys Rev B - Condens Matter Mater Phys (1999) 59:7901.
9. Maeno Y, Hashimoto H, Yoshida K, Nishizaki S, Fujita T, Bednorz JG, et al. Superconductivity in a Layered Perovskite without Copper. Nature (1994) 372:532–4. doi:10.1038/372532a0
10. Bernardini F, Olevano V, Blase X, Cano A. Infinite-Layer Fluoro-Nickelates as D9 Model Materials. J Phys Mater (2020) 3:035003. doi:10.1088/2515-7639/ab885d
11. Lee KW, Pickett WE. Infinite-Layer LaNiO2: Ni1+ Is Not Cu2+ [33]. Phys Rev B - Condens Matter Mater Phys (2004) 70:1. doi:10.1103/physrevb.70.165109
12. Chaloupka J, Khaliullin G. Orbital Order and Possible Superconductivity in LaNiO3/LaMO3 Superlattices. Phys Rev Lett (2008) 100:016404. doi:10.1103/PhysRevLett.100.016404
13. Zhang J, Botana AS, Freeland JW, Phelan D, Zheng H, Pardo V, et al. Large Orbital Polarization in a Metallic Square-Planar Nickelate. Nat Phys (2017) 13:864–9. doi:10.1038/nphys4149
14. Li D, Lee K, Wang BY, Osada M, Crossley S, Lee HR, et al. Superconductivity in an Infinite-Layer Nickelate. Nature (2019) 572:624–7. doi:10.1038/s41586-019-1496-5
15. Osada M, Wang BY, Lee K, Li D, Hwang HY. Phase Diagram of Infinite Layer Praseodymium Nickelate Pr1-XSrxNiO2 Thin Films. Phys Rev Mater (2020) 4:1. doi:10.1103/physrevmaterials.4.121801
16. Zeng SW, Li CJ, Chow LE, Cao Y, Zhang ZT, Tang CS, et al. Superconductivity in Infinite-Layer Lanthanide Nickelates. Sci Adv (2022) 8:eabl9927. doi:10.1126/sciadv.abl9927
17. Wang NN, Yang MW, Chen KY, Yang Z, Zhang H, Zhu ZH, et al. Pressure-Induced Monotonic Enhancement of Tc to over 30 K in the Superconducting Pr0.82Sr0.18NiO2 Thin Films. arXiv [Preprint] (2021). Available from: https://arXiv.org/abs/2109.12811.
18. Zhou X, Zhang X, Yi J, Qin P, Feng Z, Jiang P, et al. Antiferromagnetism in Ni‐Based Superconductors. Adv Mater (2021) 34 2106117. doi:10.1002/adma.202106117
19. Gu Q, Li Y, Wan S, Li H, Guo W, Yang H, et al. Single Particle Tunneling Spectrum of Superconducting Nd1-XSrxNiO2 Thin Films. Nat Commun (2020) 11:6027. doi:10.1038/s41467-020-19908-1
20. Pan GA, Segedin DF, LaBollita H, Song Q, Nica EM, Goodge BH, et al. Superconductivity in a Quintuple-Layer Square-Planar Nickelate. Nat Mater (2022) 21:160–4. doi:10.1038/s41563-021-01142-9
21. Gao Q, Zhao Y, Zhou XJ, Zhu Z. Preparation of Superconducting Thin Films of Infinite-Layer Nickelate Nd0.8Sr0.2NiO2. Chin Phys. Lett. (2021) 38:5. doi:10.1088/0256-307x/38/7/077401
22. Cui Y, Li C, Li Q, Zhu X, Hu Z, Yang YF, et al. NMR Evidence of Antiferromagnetic Spin Fluctuations in Nd0.85Sr0.15NiO2. Chin Phys. Lett. (2021) 38. doi:10.1088/0256-307x/38/6/067401
23. Wang BX, Zheng H, Krivyakina E, Chmaissem O, Lopes PP, Lynn JW, et al. Synthesis and Characterization of Bulk Nd1-XSrxNi O2 and Nd1-XSrxNi O3. Phys Rev Mater (2020) 4:1. doi:10.1103/physrevmaterials.4.084409
24. Zeng SW, Yin XM, Li CJ, Tang CS, Han K, Huang Z, et al. Observation of Perfect Diamagnetism and Interfacial Effect on the Electronic Structures in Infinite Layer Nd0.8Sr0.2NiO2 Superconductors. Nat Commun (2022) 13:743. doi:10.1038/s41467-022-28390-w
25. Lee K, Goodge BH, Li D, Osada M, Wang BY, Cui Y, et al. Aspects of the Synthesis of Thin Film Superconducting Infinite-Layer Nickelates. APL Mater (2020) 8:041107. doi:10.1063/5.0005103
26. Zeng S, Tang CS, Yin X, Li C, Li M, Huang Z, et al. Phase Diagram and Superconducting Dome of Infinite-Layer Nd1−xSrxNiO2 Thin Films. Phys Rev Lett (2020) 125:147003. doi:10.1103/physrevlett.125.147003
27. Bednorz JG, Müller KA. Possible highT C Superconductivity in the Ba?La?Cu?O System. Z Physik B - Condensed Matter (1986) 64:189–93. doi:10.1007/bf01303701
28. Choi M-Y, Lee K-W, Pickett WE. Role of 4f States in Infinite-Layer NdNiO2. Phys Rev B (2020) 101:20503. doi:10.1103/physrevb.101.020503
29. Zhang R, Lane C, Singh B, Nokelainen J, Barbiellini B, Markiewicz RS, et al. Magnetic and F-Electron Effects in LaNiO2 and NdNiO2 Nickelates with Cuprate-like 3dx2−y2 Band. Commun Phys (2021) 4:1. doi:10.1038/s42005-021-00621-4
30. Norman MR. Entering the Nickel Age of Superconductivity. Physics (College. Park. Md). (2020) 13:1. doi:10.1103/physics.13.85
32. Kitatani M, Si L, Janson O, Arita R, Zhong Z, Held K. Nickelate Superconductors—A Renaissance of the One-Band Hubbard Model. Npj Quan Mater (2020) 5:6. doi:10.1038/s41535-020-00260-y
33. Liu Z, Xu C, Cao C, Zhu W, Wang ZF, Yang J. Doping Dependence of Electronic Structure of Infinite-Layer NdNiO2. Phys Rev B (2021) 103:1. doi:10.1103/physrevb.103.045103
34. Sawatzky GA. Superconductivity Seen in a Non-magnetic Nickel Oxide. Nature (2019) 572:592–3. doi:10.1038/d41586-019-02518-3
35. Zhang Y-H, Vishwanath A. Type-II T − J Model in Superconducting Nickelate Nd 1 − X Sr X NiO 2. Phys Rev Res (2020) 2:1. doi:10.1103/physrevresearch.2.023112
36. Botana AS, Lee K-W, Norman MR, Pardo V, Pickett WE. Low Valence Nickelates: Launching the Nickel Age of Superconductivity. Front Phys (2022) 9:813532. doi:10.3389/fphy.2021.813532
37. Nomura Y, Arita R. Superconductivity in Infinite-Layer Nickelates. arXiv [Preprint] (2021). Available from: https://arXiv.org/abs/2107.12923.
38. Si L, Xiao W, Kaufmann J, Tomczak JM, Lu Y, Zhong Z, et al. Topotactic Hydrogen in Nickelate Superconductors and Akin Infinite-Layer Oxides ABO_{2}. Phys Rev Lett (2020) 124:166402. doi:10.1103/PhysRevLett.124.166402
39. Li Y, Sun W, Yang J, Cai X, Guo W, Gu Z, et al. Impact of Cation Stoichiometry on the Crystalline Structure and Superconductivity in Nickelates. Front Phys (2021) 9:1. doi:10.3389/fphy.2021.719534
40. Li D, Wang BY, Lee K, Harvey SP, Osada M, Goodge BH, et al. Superconducting Dome in Nd 1- X Sr X NiO 2 Infinite Layer Films. Phys Rev. Lett (2020) 1.
41. Osada M, Wang BY, Goodge BH, Harvey SP, Lee K, Li D, et al. Nickelate Superconductivity without Rare-Earth Magnetism: (La,Sr)NiO2. Adv Mater (2021) 33:1. doi:10.1002/adma.202104083
42. Osada M, Wang BY, Goodge BH, Lee K, Yoon H, Sakuma K, et al. A Superconducting Praseodymium Nickelate with Infinite Layer Structure. Nano Lett (2020) 20:5735–40. doi:10.1021/acs.nanolett.0c01392
43. Tam CC, Choi J, Ding X, Agrestini S, Nag A, Huang B, et al. Charge Density Waves in Infinite-Layer NdNiO$_2$ Nickelates. arXiv [Preprint] (2021). Available from: https://arXiv.org/abs/2112.04440.
44. Li Q, He C, Si J, Zhu X, Zhang Y, Wen HH. Absence of Superconductivity in Bulk Nd1-XSrxNiO2. Commun Mater (2020) 1:16. doi:10.1038/s43246-020-0018-1
45. Puphal P, Wu Y, Fürsich K, Lee H, Pakdaman M, Bruin JAN, et al. Topotactic Transformation of Single Crystals : From Perovskite to Infinite-Layer Nickelates. Sci Adv (2021) 7:eabl8091. doi:10.1126/sciadv.abl8091
46. He C, Ming X, Li Q, Zhu X, Si J, Wen H-H. Synthesis and Physical Properties of Perovskite Sm1−x Sr X NiO3 (X = 0, 0.2) and Infinite-Layer Sm0.8Sr0.2NiO2 Nickelates. J Phys Condens Matter (2021) 33:265701. doi:10.1088/1361-648x/abfb90
47. Goodge BH, Li D, Lee K, Osada M, Wang BY, Sawatzky GA, et al. Doping Evolution of the Mott-Hubbard Landscape in Infinite-Layer Nickelates. Proc Natl Acad Sci U S A (2021) 118:1. doi:10.1073/pnas.2007683118
48. Miller C, Botana AS. Cupratelike Electronic and Magnetic Properties of Layered Transition-Metal Difluorides from First-Principles Calculations. Phys Rev B(2020) 101:195116. doi:10.1103/physrevb.101.195116
49. Takamatsu T, Kato M, Noji T, Koike Y. Low-Temperature Synthesis of the Infinite-Layer Compound LaNiO2 Using CaH2 as Reductant. Phys C Supercond Its Appl (2010) 470:2009. doi:10.1016/j.physc.2009.10.132
50. Ikeda A, Manabe T, Naito M. Improved Conductivity of Infinite-Layer LaNiO2 Thin Films by Metal Organic Decomposition. Physica C: Superconductivity (2013) 495:134–40. doi:10.1016/j.physc.2013.09.007
51. Ren X, Gao Q, Zhao Y, Luo H, Zhou X, Zhu Z. Superconductivity in Infinite-Layer Pr$_(0.8)$Sr$_(0.2)$NiO$_2$ Films on Different Substrates. arXiv [Preprint] (2021). Available from: https://arXiv.org/abs/2109.05761.
52. Adhikary P, Bandyopadhyay S, Das T, Dasgupta I, Saha-Dasgupta T. Orbital-Selective Superconductivity in a Two-Band Model of Infinite-Layer Nickelates. Phys Rev B (2020) 102:1. doi:10.1103/physrevb.102.100501
53. Hepting M, Li D, Jia CJ, Lu H, Paris E, Tseng Y, et al. Electronic Structure of the Parent Compound of Superconducting Infinite-Layer Nickelates. Nat Mater (2020) 19:381–5. doi:10.1038/s41563-019-0585-z
54. Jiang P, Si L, Liao Z, Zhong Z. Electronic Structure of Rare-Earth Infinite-Layer RNi O2(R=La,Nd). Phys Rev B (2019) 100:1. doi:10.1103/physrevb.100.201106
55. Wu X, Di Sante D, Schwemmer T, Hanke W, Hwang HY, Raghu S, et al. Robust Dx2-Y2-Wave Superconductivity of Infinite-Layer Nickelates. Phys Rev B (2020) 101:1. doi:10.1103/physrevb.101.060504
56. Bernardini F, Bosin A, Cano A. Geometric Effects in the Infinite-Layer Nickelates. arXiv [Preprint] (2021). Available from: https://arXiv.org/abs/2110.13580.
57. Xia C, Wu J, Chen Y, Chen H. Dynamical Structural Instability and a New Crystal-Electronic Structure of Infinite-Layer Nickelates. arXiv [Preprint] (2021). Available from: https://arXiv.org/abs/2110.12405.
58. Lu H, Rossi M, Nag A, Osada M, Li DF, Lee K, et al. Magnetic Excitations in Infinite-Layer Nickelates. Science (2021) 373:213–6. doi:10.1126/science.abd7726
59. Krieger G, Martinelli L, Zeng S, Chow LE, Kummer K, Arpaia R, et al. Charge and Spin Order Dichotomy in NdNiO2 Driven by SrTiO3 Capping Layer. arXiv [Preprint] (2021). Available from: https://arXiv.org/abs/2112.03341.
60. Rossi M, Osada M, Choi J, Agrestini S, Jost D, Lee Y, et al. A Broken Translational Symmetry State in an Infinite-Layer Nickelate. arXiv [Preprint] (2021). Available from: https://arXiv.org/abs/2112.02484.
61. Lin JQ, Villar Arribi P, Fabbris G, Botana AS, Meyers D, Miao H, et al. Strong Superexchange in a D9-δ Nickelate Revealed by Resonant Inelastic X-Ray Scattering. Phys Rev Lett (2021) 126:1. doi:10.1103/physrevlett.126.087001
62. Zhang GM, Yang YF, Zhang FC. Self-Doped Mott Insulator for Parent Compounds of Nickelate Superconductors. Phys Rev B (2020) 101:1. doi:10.1103/physrevb.101.020501
63. Wang Z, Zhang GM, Yang YF, Zhang FC. Distinct Pairing Symmetries of Superconductivity in Infinite-Layer Nickelates. Phys Rev B (2020) 102:1. doi:10.1103/physrevb.102.220501
64. Tsuei CC, Kirtley JR. Pairing Symmetry in Cuprate Superconductors. Rev Mod Phys (2000) 72:969–1016. doi:10.1103/revmodphys.72.969
65. Wu X, Jiang K, Di Sante D, Hanke W, Schnyder AP, Hu J, et al. Surface S-Wave Superconductivity for Oxide-Terminated Infinite-Layer Nickelates. arXiv [Preprint] (2020). Available from: https://arXiv.org/abs/2008.06009.
66. Wang BY, Li D, Goodge BH, Lee K, Osada M, Harvey SP, et al. Isotropic Pauli-Limited Superconductivity in the Infinite-Layer Nickelate Nd0.775Sr0.225NiO2. Nat Phys (2021) 17:473–7. doi:10.1038/s41567-020-01128-5
67. Yuan HQ, Singleton J, Balakirev FF, Baily SA, Chen GF, Luo JL, et al. Nearly Isotropic Superconductivity in (Ba,K)Fe2As2. Nature (2009) 457:565–8. doi:10.1038/nature07676
68. Martin C, Tillman ME, Kim H, Tanatar MA, Kim SK, Kreyssig A, et al. Nonexponential London Penetration Depth of FeAs-Based Superconducting RFeAsO(0.9)F(0.1) (R = La, Nd) Single Crystals. Phys Rev Lett (2009) 102:247002. doi:10.1103/PhysRevLett.102.247002
69. Chow LE, Kunniniyil Sudheesh S, Nandi P, Zeng SW, Zhang ZT, Du XM, et al. Pairing Symmetry in Infinite-Layer Nickelate Superconductor. arXiv [Preprint] (2022). Available from: https://arxiv.org/abs/2201.10038.
70. Harvey SP, Wang BY, Fowlie J, Osada M, Lee K, Lee Y, et al. Evidence for Nodal Superconductivity in Infinite-lLyer Nickelates. arXiv [Preprint] (2022). Available from: https://arxiv.org/abs/2201.12971.
Keywords: infinite-layer nickelates, nickelate superconductivity, pairing symmetry, meissner effect, lanthanide nickelate, thickness dependence, rare earth magnetism
Citation: Chow LE and Ariando A (2022) Infinite-Layer Nickelate Superconductors: A Current Experimental Perspective of the Crystal and Electronic Structures. Front. Phys. 10:834658. doi: 10.3389/fphy.2022.834658
Received: 13 December 2021; Accepted: 10 January 2022;
Published: 03 March 2022.
Edited by:
Junjie Zhang, Shandong University, ChinaReviewed by:
Xingjiang Zhou, Institute of Physics (CAS), ChinaCopyright © 2022 Chow and Ariando. This is an open-access article distributed under the terms of the Creative Commons Attribution License (CC BY). The use, distribution or reproduction in other forums is permitted, provided the original author(s) and the copyright owner(s) are credited and that the original publication in this journal is cited, in accordance with accepted academic practice. No use, distribution or reproduction is permitted which does not comply with these terms.
*Correspondence: A. Ariando, YXJpYW5kb0BudXMuZWR1LnNn