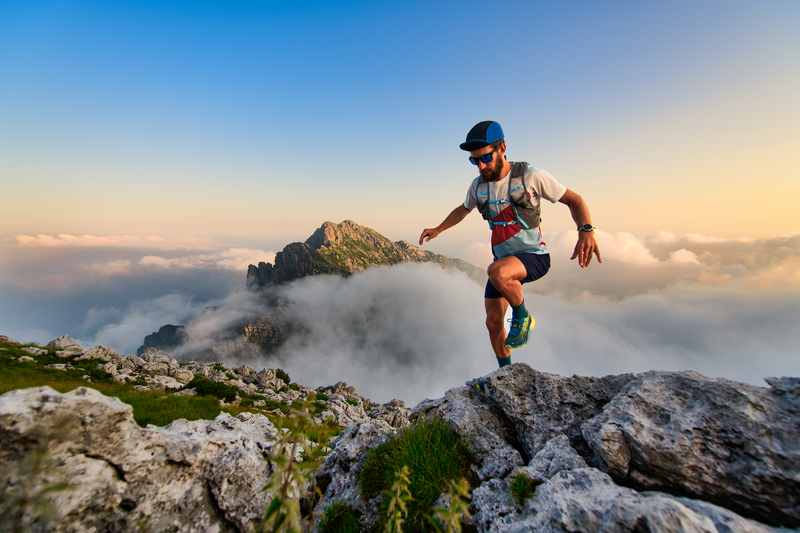
95% of researchers rate our articles as excellent or good
Learn more about the work of our research integrity team to safeguard the quality of each article we publish.
Find out more
REVIEW article
Front. Phys. , 11 May 2022
Sec. High-Energy and Astroparticle Physics
Volume 10 - 2022 | https://doi.org/10.3389/fphy.2022.813753
The CPT symmetry, which combines Charge Conjugation, Parity, and Time Reversal, is a cornerstone of our model-building method, and its probable violation will endanger the most extended tool we presently utilize to explain physics, namely local relativistic quantum fields. However, the kaon system’s conservation constraints appear to be rather severe. We will show in this paper that neutrino oscillation experiments can enhance this limit by many orders of magnitude, making them an excellent instrument for investigating the basis of our understanding of Nature. As a result, verifying CPT invariance does not evaluate a specific model, but rather the entire paradigm. Therefore, as the CPT’s status in the neutrino sector, linked or not to Lorentz invariance violation, will be assessed at an unprecedented level by current and future long baseline experiments, distinguishing it from comparable experimental fingerprints coming from non-standard interactions is critical. Whether the entire paradigm or simply the conventional model of neutrinos is at jeopardy is significantly dependent on this.
Neutrinos have the potential to take our hunt for novel physics to a new level. What makes them so difficult to detect, what allows them to travel vast distances without being halted or deflected, permits us to magnify Planck suppressed effects (or effects of equivalent scales) to levels that we can measure or constrain in future long baseline experiments. In this work, we analyze the bounds we can establish already and explore the sensitivity to CPT and Lorentz-violating interactions in a framework that allows for simple extrapolation of the constraints established to any phenomenological variation of the neutrino dispersion relation.
The cornerstone of our model-building technique is CPT symmetry, which combines Charge Conjugation, Parity, and Time Reversal. We do use local relativistic quantum field theories to describe the fundamental reality around us. As a result, the consequences of its possible violation will pose a serious danger to the most comprehensive instrument we presently have for describing physics, namely local relativistic quantum fields.
The position of CPT as a sacred cow of model building resides in the fact that the theorem behind it, the CPT Theorem [1], which states that particle and antiparticle have the same mass and, if unstable, the same lifetime, is based on only three assumptions: Lorentz invariance, Hamiltonian hermiticity, and locality, all of which have other reasons to be in the theory, far beyond the CPT theorem itself.
This is why it is critical to check the CPT symmetry. If found to be violated, then one of the three elements assumed to prove it must be false, and our entire model-building method must be reconsidered. For an implementation of a CPT violating (non-local) model of neutrinos, see [2].
It must be emphasized however that testing the predictions of CPT conservation is not identical, strictly speaking, to constraining CPT violation. Comparing the masses of particles and antiparticles can be used to test CPT conservation. Indeed, these mass discrepancies can be regarded observables that violate the CPT symmetry. However, only in the context of a certain specific model of CPT violation, can a bound from distinct observables be interpreted and compared.
Since the inception of the Standard Model, experimental evidence of CPT violation has been sought, primarily because of its enormous potential ramifications. Figure 1 contains a summary of the findings. The most rigorous (in terms of relative precision) limit comes from the neutral kaon system [5], which appears to be so solid that it leaves little room for entertaining the idea of a Universe in which the CPT symmetry is broken.
FIGURE 1. Lorentz and CPT violation breakdown can be described in a model-independent and general way via realistic Lagrangian effective field theory. The resulting effective field theory framework is known as the Standard Model Extension (SME). In this figure the relative experimental precision and SME coefficients for various quantities is shown [3]. Dark blue: existing experimental values, orange: SME coefficient. Courtesy of Eberhard Widmann.
This notion, on the other hand, could not be more fallacious. The strength of this limit is totally artificial, arising solely from two reasons. First our endeavor to obtain dimensionless bounds and second, the specific choice we have made for the denominator.
Although it can sometimes be interesting and even useful to provide a bound in a dimensionless fashion, we have no model of CPT violation to date, therefore the selection of the scale to use is arbitrary. By using the Planck scale, we may have gotten a far more restrictive (and equally significant) constraint. As a result, until we have a thorough theory of CPT violation to answer the question of what scale is appropriate for this problem, the most we can say is
Furthermore, because the kaon is not an elementary particle and its mass is controlled by QCD, the accurate statement we can draw about this bound is that QCD is CPT invariant.
Lepton probes are required to evaluate CPT violations in elementary particles. Electrons were used in such a test to obtain
Besides, as the kaon is a boson, the parameter that enters the Lagrangian is its mass squared, hence the constraint above should be rewritten as
while that of the electrons becomes
At this point, it becomes clear not only that neutrinos can already improve this bound by several orders of magnitude, providing the world’s best bound on CPT invariance [4, 6] but also that neutrinos do offer the possibility to improve it several orders of magnitude in the near future.
So far we have seen CPT violation in the mass differences, CPT violation in the absolute mass scale can be bounded using cosmological data like the matter power spectrum [7] and although this bound is not competitive with the electron bound today, it will significantly improve in the coming years. It should be noted however that this bound as well that the one on the mass difference, is free of charge contamination effects.
Besides the contamination-free aspect of it, there are numerous reasons to believe that neutrinos could be an appropriate probe for CPT violation: quantum gravity is thought to be non-local, allowing for a possible CPT violation. Its effects, on the other hand, are expected to be Planck suppressed, i.e.
In summary, if there is a sector where CPT can show up, this sector is indeed neutrino physics. Amazingly enough, this sector not only also offers the best world bounds but also through the ongoing and planned neutrino experiments can improve it significantly.
CPT violation in the neutrino sector could have significant ramifications. One of the most attractive ones being the generation of the baryon asymmetry of the Universe. If CPT is not conserved, the baryon asymmetry can be generated in equilibrium. The Sakharov conditions do not need to be fulfilled.
Besides, the fact that the mass squared is the parameter entering the dispersion relation, E2 = p2 + m2, and the natural parameter in relativistic kinematics further motivates its choice as the CPT violating observable.
As a result, deviations from the conventional dispersion relation can be used to investigate CPT violations caused by Lorentz invariance breaking and those violations of CPT that have another origin, for example, non-locality. Therefore, we will start our analysis by assuming a CPT scenario, reduced only to a CPT violating spectrum, like the one shown in Figure 2 In this case, we will extract the bounds by an overall fit of the experiments, using only those experiments where the neutrino and antineutrino data can be separated. Earlier constraints were determined with neutrinos and antineutrinos having the same mass ordering. It is worth noting that differing mass orderings for neutrinos and antineutrinos would also indicate CPT violation, even if the mass difference is the same.
FIGURE 2. The figure illustrates a CPT violating spectrum. A possible overall shift between the neutrino and antineutrinos is omitted since oscillation experiments can’t test it. As commented before, the cosmological matter power matter spectrum can do it.
Below we summarize the neutrino samples considered, indicating in each case the neutrino or antineutrino parameters they are sensitive to.
• solar neutrino data [9–17]: θ12,
• neutrino mode in long–baseline experiments K2K [18], MINOS [19, 20], T2K [21, 22] and NOνA [23, 24]: θ23,
• KamLAND reactor antineutrino data [25]:
• short–baseline reactor antineutrino experiments Daya Bay [26], RENO [27] and Double Chooz [28]:
• antineutrino mode in long–baseline experiments1 MINOS [19, 20] and T2K [21, 22]:
Because all potential values of δ or
improving the older bounds for all parameters, except for sin2θ13, that remains unchanged.
It is important to notice at this point that the limit on
Besides, its importance and absolute relevance in itself, a dedicated experimental analysis and the extraction of the corresponding bound on CPT violation, stands in the way of claiming the discovery of CP violation in the neutrino sector. Although the T2K collaboration has claimed experimental evidence of CP violation at the 3σ level [30] and the NoνA experiment will follow soon [31], this claim, which is very robust in front of several new physics scenarios, assumes that neutrino and antineutrino oscillation parameters in vacuum are identical, i.e., CPT conservation. A symmetry which has not been tested to the level needed to support such an assumption, as shown in [6].
Unfortunately, we are still nowhere close to claiming experimental proof of CP violation in the neutrino system, no matter how enticing and thrilling it may be. CPT violation, a way more fascinating phenomena that challenges our explanation of Nature in terms of local relativistic quantum field theory, should be checked before. Otherwise, the T2K and NOvA findings may be explained by a difference in oscillation parameters in the neutrino and antineutrino sectors, which is perfectly compatible with all available neutrino evidence thus far.
Lorentz’s symmetry violations do not always induce CPT violations in the mass spectrum, as the ones considered so far. Lorentz–violating neutrinos and its corresponding antineutrinos can be effectively described by the following Lagrangian density [32].
where
The observable effect on the left-handed neutrinos is governed by the combinations,
which are constant hermitian matrices in the flavor space that can modify the standard vacuum Hamiltonian. The first combination is relevant for CPT–violating neutrinos, whereas the second combination is only relevant for CPT-even Lorentz-violating neutrinos.
To make transparent the phenomenology we are looking for we will look at the isotropic component of the Lorentz–violating terms, and therefore we will fix the (μ, ν) indices to 0. We will also focus on the oscillation phenomenology. To simplify our notation, from now on, we will denote the parameters
Explicitly, one can write the Lorentz–violating contribution to the full oscillation Hamiltonian as
with
Note that the effect of aαβ–induced Lorentz violation in neutrino oscillations is proportional to the neutrino baseline L, while the terms corresponding to cαβ induce new contributions proportional to LE. In the latter, the factor −4/3 arises from the non–observability of the Minkowski trace of cL, which forces the components xx, yy, and zz to be related to the 00 component.
Then, if we focus on the first term in Eq. 9, we notice that this Hamiltonian looks very similar to the one corresponding to nonstandard interactions (NSI) in the neutrino propagation
where the NSI term is parametrized as
Here Ne corresponds to the electron number density along the neutrino trajectory and the parameters
There are, however, significant distinctions between the two scenarios [36]. The sort of CPT violation examined here is an inherent effect that occurs even in vacuum, whereas NSI during neutrino propagation is an exotic matter effect that does not play a role while in vacuum. Nonetheless, the equivalency in Eq. 12 allows for the investigation of CPT-violating parameters in long baseline experiments, in a manner similar to how NSI is treated in neutrino propagation.
As we have seen, neutrino-oscillation observations have ultrahigh sensitivity to Lorentz and CPT violation, due to their interferometric nature. Novel mixing properties of neutrino and antineutrino flavor states are predicted in most of the Standard Model extensions that can give rise to these phenomena. Non-canonical energy dependencies of the oscillation phase, energy-dependent mixing angles in vacuum, direction-dependent oscillation probabilities due to the loss of rotational invariance, time-dependent oscillation probabilities in Earth-based experiments arising from laboratory motion, and mixing between neutrinos and antineutrinos are all examples of Lorentz- and CPT-violating signatures that can and must be searched for in the coming experiments. For an updated collection of bounds see [37].
To illustrate this point, we can analyze the dominant channel, νμ → νe, at the peak of the DUNE neutrino flux 3 GeV [38]. Notice that the transition probability peaks at this energy, while the survival probability has a dip. Results are presented in Figures 3, 4. The reference to DUNE is not gratuitous, other forthcoming neutrino experiments, most notably T2HK [39] (or its version with a detector in Korea T2HKK [40]) and the European Spallation Source ν-Beam (ESSνB) [41] do not offer such a sensibility. The reason behind is clear, the degeneracies between the NSNI parameters, as well as the generalized mass hierarchy degeneracy, significantly limit the prospects of these experiments as a potential cancellation between leading order terms in the appearance channel may occur when ϵeτ = cos θ23ϵeμ
FIGURE 3. Upper-panel: oscillation probability as a function of energy for the νμ → νe channel with standard matter effects (black) and a non-diagonal CPT-violating parameter (red). Lower-panel: the absolute difference between the above-mentioned standard oscillation and the CPT-violating oscillation probability. Each column represents a separate (unique) non-diagonal CPT-violating parameter that is set to ±2.0, ×, 10–23 GeV and is different from zero. The smallness of the parameters is just another proof of the exquisite sensitivity of neutrino oscillation experiments to tiny violations of CPT and Lorentz invariance. The DUNE baseline (1,300 km) has been assumed, with a vertical line indicating the peak energy of the neutrino beam in DUNE.
FIGURE 4. Same results as in Figure 3, now for the diagonal parameters in the electron and muon sectors. Notice, however, that the benchmark values used for the diagonal CPT–violating parameters here are five times larger than the ones assumed in Figure 3. Still, the level of sensitive is impressive.
A similar, but strongly model dependent way, to look for violations of Lorentz invariance, which may or may not be associated with CPT violation is through an effectively modified dispersion relation. There are a variety of methods to change the dispersion relation, and the majority of these are simple to include into the Hamiltonian and therefore easily bounded. Depending on their energy dependence, they will give rise to different spectral distortions. For example, a neutrino energy given by,
where the E, p and m stand for the neutrino energy, momentum and mass, respectively, and A is precisely a dimension-full Lorentz breaking parameter. The subscript i, makes it transparent that this parameter is non-universal.
In the two generation case, this dispersion relation gives rise to the following transition and survival probabilities
where θ is the mixing angle and
Clearly the different energy dependencies allow for a clear distinction between the standard term and the new one. Even more, extragalactic neutrinos and their time-of-flight data and flavor ratios as well as astrophysical neutrinos do exhibit an enormous potential for probing such effects, which are so dependent on the propagation distance, see for example [42] and references therein.
Also, models with extra dimensions can be bounded using neutrinos. In this context, the light neutrino masses might be explained by a mechanism analogous to the seesaw mechanism in the setting of additional dimensions. Because right-handed neutrinos are Standard Model singlets, a scenario in which the Standard Model fields are restricted to live in the brane while a number of right-handed neutrinos propagate in the additional dimensions, may be considered natural. The scenario is similar to the type-I seesaw model, except that the right-handed neutrinos’ zero modes come along with towers of extremely heavy Kaluza-Klein modes, which might explain why the light neutrino masses are suppressed. In this setting, the mixing among the left- and right-handed neutrinos, induce non-unitarity effects in the neutrino mixing matrix that depend on the model’s parameters.
Other models of extra dimensions, can also be phenomenologically described by a modified dispersion relation as the one shown before and therefore can also be incorporated into the Hamiltonian and bounded.
Despite the exquisite sensitivities to some parameters that will be reached with the current and next generation of oscillation and high energy astrophysical experiments, some Lorentz- and CPT-violating operators are unobservable in these experiments, as they leave flavor-oscillation and velocity properties of neutrinos unchanged. They do, however, have an impact on the phase space accessible for neutrinos in the final state of nuclear processes, for example. Modern beta-decay endpoint measurements, which are used to calculate the absolute neutrino mass scale, are also well adapted to searching for such operators due to their great accuracy. These particle-decay measurements have the one and only known source of experimental sensitivity to such effects, which may have gone undetected by other approaches and hence can be rather substantial.
The effects of operators of mass dimension d ≥ 4 in effective field theories, other Lorentz- and CPT-violating, commonly referred to as counter-shaded, may also be explored using double beta decay experiments’ striking low background sensitivity. Lorentz- and CPT-violating phase-space corrections may cause different distortions of a specific region of the two-neutrino double beta decay spectrum, similar to the single beta decay signals mentioned above. Furthermore, the relevant operator breaks CPT symmetry while retaining T invariance, resulting in a novel CP violation source in the neutrino sector. Finally, in the lack of a Majorana mass term, the mixing of neutrinos and antineutrinos caused by Lorentz violation can cause neutrinoless double beta decay. Because of these characteristics, neutrinoless double beta decay studies can detect Lorentz invariance and CPT symmetry.
Unmeasured neutrino properties, as well as tension between different neutrino experiments, offer a lot of promise for new neutrino physics findings in the next decade. The unique chance to verify Lorentz and CPT symmetry in the neutrino sector is one of the ongoing and upcoming experimental initiatives in this context. These closely related invariance concepts are a cornerstone of modern physics. Nonetheless, it is commonly recognized that an underlying framework that provides a coherent account of both quantum and gravitational physics will need, at least to some extent, deviations from already known theoretical underpinnings. In particular, in this setting, a variety of theoretical developments to address Nature, like string theory, have the potential to break both Lorentz and CPT invariance. Such effects would produce quantifiable signals at currently accessible energy scales, providing us a fascinating new path for studying the physics that hides beyond the Standard Model, which might emerge at the Planck scale.
The author confirms being the sole contributor of this work and has approved it for publication.
The author declares that the research was conducted in the absence of any commercial or financial relationships that could be construed as a potential conflict of interest.
All claims expressed in this article are solely those of the authors and do not necessarily represent those of their affiliated organizations, or those of the publisher, the editors, and the reviewers. Any product that may be evaluated in this article, or claim that may be made by its manufacturer, is not guaranteed or endorsed by the publisher.
I do thank Eberhard Widmann for generously speeding up his own project to be able to share the CPT bounds figure shown here. I also thank Joe Lykken, Pablo Martinez, Mehedi Masud, Jordi Salvado, Christoph Ternes and Mariam Tortola for their collaboration on CPT and all that during these years. GB acknowledges support from the MEC and FEDER (EC) grant PID2020-113334 GB-I00/AEI/10.13039/501100011033 and the Generalitat Valenciana under grant PROMETEOII/2017/033.
1The K2K experiment took only data in neutrino mode. The NOνA experiment has not yet published data in antineutrino mode.
2These components are defined in the Sun–centered celestial equatorial frame.
2. Barenboim G, Lykken J. A Model of CPT Violation for Neutrinos. Phys Lett B (2003) 554:73–80. doi:10.1016/S0370-2693(02)03262-8
4. Barenboim G, Ternes CA, Tórtola M. Neutrinos, DUNE and the World Best Bound on CPT Invariance. Phys Lett B (2018) 780:631–7. doi:10.1016/j.physletb.2018.03.060
6. Barenboim G, Tórtola CAM, Ternes CA. CPT and CP, an Entangled Couple. J High Energ Phys (2020) 2020:155. doi:10.1007/JHEP07(2020)155
7. Barenboim G, Salvado J. Cosmology and CPT Violating Neutrinos. Eur Phys J C (2017) 77(11):766. doi:10.1140/epjc/s10052-017-5347-y
8. Barenboim G, Ternes CA, Tórtola M. New Physics vs New Paradigms: Distinguishing CPT Violation from NSI. Eur Phys J C (2019) 79(5):390. doi:10.1140/epjc/s10052-019-6900-7
9. Cleveland BT, Daily T, Davis R, Distel JR, Lande K, Lee CK, et al. Measurement of the Solar Electron Neutrino Flux with the Homestake Chlorine Detector. ApJ (1998) 496:505–26. doi:10.1086/305343
10. Kaether F, Hampel W, Heusser G, Kiko J, Kirsten T. Reanalysis of the Gallex Solar Neutrino Flux and Source Experiments. Phys Lett B (2010) 685:47–54. doi:10.1016/j.physletb.2010.01.030
14. Abe K, Hayato Y, Iida T, Ikeda M, Iyogi K, Kameda J, et al. Search for Differences in Oscillation Parameters for Atmospheric Neutrinos and Antineutrinos at Super-kamiokande. Phys Rev Lett (2011) 107:241801. doi:10.1103/PhysRevLett.107.241801
16. Aharmim B, et al. Retraction: Geometric Ginzburg-Landau Theory for Faceted Crystals in One Dimension: From Coarsening to Chaos through a Driving Force [Phys. Rev. E 79, 011115 (2009)]. Phys Rev E Stat Nonlin Soft Matter Phys (2010) 81:039901. doi:10.1103/PhysRevE.81.039901
22. Abe K, Amey J, Andreopoulos C, Antonova M, Aoki S, Ariga A, et al. Combined Analysis of Neutrino and Antineutrino Oscillations at T2K. Phys Rev Lett (2017) 118(15):151801. doi:10.1103/PhysRevLett.118.151801
27. Choi JH, Choi WQ, Choi Y, Jang HI, Jang JS, Jeon EJ, et al. Observation of Energy and Baseline Dependent Reactor Antineutrino Disappearance in the RENO Experiment. Phys Rev Lett (2016) 116(21):211801. doi:10.1103/PhysRevLett.116.211801
29. de Salas PF, Forero DV, Ternes CA, Tórtola M, Valle JWF. Status of Neutrino Oscillations 2018: 3σ Hint for normal Mass Ordering and Improved CP Sensitivity. Phys Lett B (2018) 782:633–40. doi:10.1016/j.physletb.2018.06.019
32. Alan Kostelecký V, Mewes M. Lorentz andCPTviolation in Neutrinos. Phys Rev D (2004) 69:016005. doi:10.1103/PhysRevD.69.016005
33. Kostelecký VA, Mewes M. Neutrinos with Lorentz-Violating Operators of Arbitrary Dimension. Phys Rev D (2012) 85:096005. doi:10.1103/PhysRevD.85.096005
34. Kostelecký VA, Russell N. Data Tables for Lorentz andCPTviolation. Rev Mod Phys (2011) 83:11–31. doi:10.1103/RevModPhys.83.11
36. Barenboim G, Masud M, Ternes CA, Tórtola M. Exploring the Intrinsic Lorentz-Violating Parameters at DUNE. Phys Lett B (2019) 788:308–15. doi:10.1016/j.physletb.2018.11.040
37. Kostelecký VA, Russell N. Data Tables for Lorentz andCPTviolation. Rev Mod Phys (2011) 83:11–31. doi:10.1103/RevModPhys.83.11
Keywords: CPT symmetry, neutrino properties, lorentz violation, fundamental symmetries, discrete symmetries
Citation: Barenboim G (2022) Some Aspects About Pushing the CPT and Lorentz Invariance Frontier With Neutrinos. Front. Phys. 10:813753. doi: 10.3389/fphy.2022.813753
Received: 12 November 2021; Accepted: 02 March 2022;
Published: 11 May 2022.
Edited by:
Omar G. Miranda, Instituto Politécnico Nacional de México (CINVESTAV), MexicoReviewed by:
Heinrich Päs, Technical University Dortmund, GermanyCopyright © 2022 Barenboim. This is an open-access article distributed under the terms of the Creative Commons Attribution License (CC BY). The use, distribution or reproduction in other forums is permitted, provided the original author(s) and the copyright owner(s) are credited and that the original publication in this journal is cited, in accordance with accepted academic practice. No use, distribution or reproduction is permitted which does not comply with these terms.
*Correspondence: Gabriela Barenboim, Z2FicmllbGEuYmFyZW5ib2ltQHV2LmVz
Disclaimer: All claims expressed in this article are solely those of the authors and do not necessarily represent those of their affiliated organizations, or those of the publisher, the editors and the reviewers. Any product that may be evaluated in this article or claim that may be made by its manufacturer is not guaranteed or endorsed by the publisher.
Research integrity at Frontiers
Learn more about the work of our research integrity team to safeguard the quality of each article we publish.