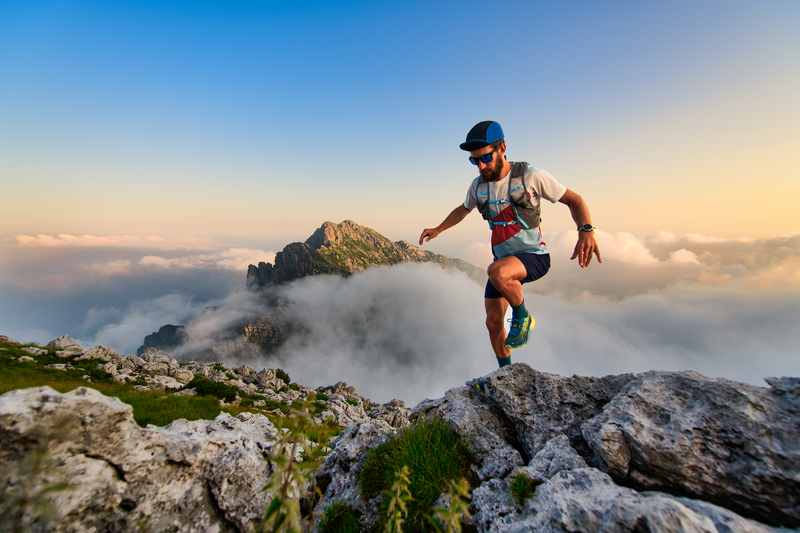
95% of researchers rate our articles as excellent or good
Learn more about the work of our research integrity team to safeguard the quality of each article we publish.
Find out more
ORIGINAL RESEARCH article
Front. Phys. , 09 December 2022
Sec. Atomic and Molecular Physics
Volume 10 - 2022 | https://doi.org/10.3389/fphy.2022.1090483
This article is part of the Research Topic Quantum Precision Measurement and Cold Atom Physics, Volume II View all 4 articles
Atomic optical filters such as Faraday anomalous dispersion optical filters (FADOFs) or similar technologies can achieve very narrow optical bandwidth close to the scale of atomic linewidth, which can be greatly reduced in cold atoms. However, limited by the number of cold atoms and the size of the cold atomic cloud, the number of atoms interacting with the laser is reduced, and the transmission remains as low as 2%. In this work, we introduce the optical pumping into the cold atomic optical filter to solve this problem. Circular polarized optical pumping can produce polarization of the atomic ensemble and induce dichromatic as well as the Faraday rotation. We demonstrate a cold-atom optical filter which operates on the 87Rb 52S1/2 (F=2) to 52P3/2 (F′=2) transition at 780 nm. The filter achieves an ultranarrow bandwidth of 6.6(4) MHz, and its peak transmission is 15.6%, which is nearly 14 times higher than that of the cold-atom optical filter realized by Faraday magneto-optic effect. This scheme can be extended to almost all kinds of atomic optical filters and may find applications in self-stabilizing laser and active optical clock.
Atomic optical filter is a device that uses the spectral characteristics of atomic resonance transition to achieve filtering function. It is mainly divided into two categories: Atomic resonance filter [1–4] (ARF) and optical rotation effect-based filter [5, 6] (such as Faraday anomalous dispersion optical filter [7, 8], FADOF). With the advantages of high peak transmission [9–12], narrow bandwidth [13–16], and excellent out-of-band suppression [17], the atomic optical filter can extract weak narrowband light signal from strong background light noise (including sunlight, scattered light, and artificial light, etc.). At present, it has played a key role in the fields of optical communication [18, 19], lidar [20, 21], and self-stabilizing laser [22, 23].
The induced-dichroism-excited atomic line filter [24] (IDEALF) is a novel rotation-based filter. Due to the reverse transmission of probe laser and pump laser, only atoms with very narrow velocity groups can be excited to participate in the filtering process, which effectively reduces the influence of Doppler broadening on the filter. Therefore, many studies use this induced dichroism principle [25] to narrow the filter’s bandwidth. For example, these works, Refs. [26–30], on FADOF have improved the FADOF bandwidth from ∼ 200 MHz down to ∼ 20 MHz over the past two decades. However, it is still difficult to narrow the transmitted bandwidth of filters based on thermal atoms to the order of natural linewidth.
To overcome the Doppler effect caused by atomic motion and other factors related to atomic velocity in the process of interaction between light and atoms, the cold-atom FADOF has been studied [31]. By using laser cooling technology to slow down atoms, so as to reduce the influence of Doppler effect, they finally realized an ultranarrow-bandwidth cold-atom FADOF with bandwidth close to the natural linewidth of atomic transition. However, there is also a problem with the above research scheme, that is, the transmission is greatly sacrificed while the bandwidth of FADOF is narrowed. This is mainly because in the FADOF realized by thermal-atom scheme, the number of atoms in the vapor cell can reach 1012, while in the FADOF realized by cold-atom scheme, the number of cold atoms trapped by laser cooling technology is only about 108. Although the density of the two gas atoms is approximately the same, the length of laser traveling in the cold atom medium is much shorter than that of the thermal atom. The decrease in the number of atoms interacting with the laser is detrimental to the atomic optical filter’s transmission. Therefore, how to improve the transmission as much as possible while ensuring the ultranarrow-bandwidth of FADOF is the key to current research.
In this study, the laser cooling technology is also used to narrow the atomic filter’s bandwidth, but it is combined with the optical pumping technology [32, 33]. Different from the previous purpose of narrowing the transmitted bandwidth, we use the induced dichroism to improve the transmission of cold-atom optical filter. The relevant energy levels are shown in Figure 1.
FIGURE 1. Relevant 87Rb energy levels of cold-atom optical filter enhanced by optical pumping. The cooling laser has a red detuning of 15 MHz to the transition frequency of F=2 → F′=3. The frequency of the repumping laser refers to the F=1 → F′=2 transition. The frequencies of σ+ circularly polarized pump laser and linearly polarized probe laser both refer to the F=2 → F′=2 transition.
When the σ+ circularly polarized pump laser is applied to the cold atoms, an asymmetric distribution of atoms will be constructed on the ground state magnetic sublevels. Thus, the left and right circularly polarized components of linearly polarized incident laser have different refractive indices. For thin atomic vapor, the refractive index of the incident laser can be expressed as [34]:
Here, ρ is the density of gas atoms,
The atoms also have absorption effect on incident laser. The effective atomic absorption cross-section can be defined as Eq. 2. α(δ) = ρσ(δ) is the absorption coefficient. Then, the transmission of the filter can be written as [8, 25]:
where
Corresponding to the actual working conditions of the experiment, when the cold atomic vapor density is 2 × 1010/cm3 and the cold atomic cloud diameter is 2 mm, we obtain the theoretical simulation results shown in Figure 2. The filter displays a single 6.8 MHz passband at a peak transmission of 28.4%. This bandwidth is slightly wider than the natural linewidth of atomic transition (6.1 MHz) because we take into account the effects of saturation broadening and residual Doppler broadening. Cold-atom optical filtering enhanced by optical pumping has another advantage, that is, its optical rotation is induced by optical pumping to bring an asymmetric population of atoms on the ground state Zeeman sublevels, rather than the sublevel splitting by a magnetic field. Therefore, it does not need an additional uniform magnetic field, which is a technical innovation different from the previous cold-atom FADOF.
FIGURE 2. Calculated (A) rotation angle and (B) transmission versus probe laser detuning (corresponding to F=2 → F′=2 transition). The cold atomic vapor density is 2 × 1010/cm3, the cold atomic cloud diameter is 2 mm.
The scheme of the experimental setup is presented in Figure 3. The first step of our study is to prepare cold 87Rb atoms by magneto-optical trap (MOT). We lock the cooling laser and repumping laser to F=2 → F′=3 and F=1 → F′=1 transitions using the standard saturated absorption spectroscopy technique. Then, we red-detune the former by 15 MHz for cooling and blue-detune the latter towards F′=2 for repumping. After frequency stabilization, the cooling and repumping lasers are combined and then divided into six beams with the same power, which are injected into the optical windows of the vacuum system in pairs (The power of each cooling laser is about 6 mW, and each repumping laser is about 1.5 mW, the diameter of each laser beam is about 10 mm). The vacuum system (vacuum level: 2 × 10−9 Torr) contains thin 87Rb atomic vapor. The gradient magnetic field of MOT is provided by a pair of coils with current in opposite directions. Finally, we trap around 2 × 108 atoms in the MOT with a temperature of around 200 μK. The diameter of the cold atomic cloud is about 2 mm, the density of the cold atomic vapor is about 1010/cm3.
FIGURE 3. (A) Schematic of the experimental setup. NDF: neutral density filter; GT: Glan-Taylor prism; PD: photo-electric detector; L1 and L2: plane-convex lenses; QWP: quarter-wave plate; M’: mirror with reflectivity of 10% and transmission of 90%; MOT: magneto-optical trap. The gradient magnetic field coils and geomagnetic field compensation coils of MOT are not shown in figure. (B) Relevant time sequence diagram of the experiment.
Next, we carry out the construction of cold-atom optical filter and its pump light path and probe light path. Using the modulation transfer spectrum (MTS) frequency stabilization technology, the 780 nm probe laser is locked to the F=2 → F′=3 transition, and then red-shifted 267 MHz to F′=2 by an acousto-optic modulator (AOM). After frequency stabilization, the probe laser is firstly transmitted to the neutral density filter (NDF) to adjust the laser power. Then, it passes through the cold atomic cloud trapped in the vacuum system, a pair of Glan-Taylor prisms (GTPs) with orthogonal polarization direction, and then enters the photo-electric detector (PD), which measures the transmitted signal of the cold-atom optical filter. On the other side, a beam is divided from the 780 nm cooling laser, it is used as the pump laser for this study, and its frequency is adjusted to the F=2 → F′=2 transition by an AOM. This pump laser passes through a half-wave plate (HWP), a polarizing beam splitter (PBS), a pair of plane-convex lenses (L1 and L2), and a quarter-wave plate (QWP), then reflected by a mirror with reflectivity of 10% and transmission of 90% (M’). The reflected pump laser overlaps with the probe laser in reverse and passes through the cold atomic cloud trapped in the vacuum system. Here, HWP and PBS are used to adjust the pump laser power and turn the pump laser into a pure linearly polarized light. L1 and L2 are used to expand the pump laser beam, letting the pump laser completely contains cold atomic cloud when passing through the vacuum system, so as to improve the efficiency of optical pumping. QWP is used to convert the linearly polarized pump laser into a standard σ+ circularly polarized pump laser.
Setting 2 s as a cycle, the MOT (cooling laser and gradient magnetic field) is switched off at 1.5 s. Then, after a time interval of 1 ms, turn off the pump laser and immediately turn on the probe laser to measure the transmitted signal of the cold-atom optical filter. The repumping laser is kept on during the whole cooling and pumping process, in order to return the atoms from F=1 to F=2. The AOM placed on the probe light path can be used for fast switching the probe laser. Under the experimental conditions shown in Table 1, we use the above AOM to generate frequency detuning near the probe laser resonance frequency, discretely measure the transmission values at multiple frequency points, and then draw them in combination. Thus, the transmission spectrum of the cold-atom optical filter which operates on the F=2 → F′=2 transition is obtained (see the black square points in Figure 4). Using Lorentz fitting (blue curve), it can be concluded that the transmitted bandwidth of the cold-atom optical filter realized by optical pumping method is 6.6(4) MHz, and the peak transmission is 15.6%.
FIGURE 4. Transmission spectrum of the cold-atom optical filter (corresponding to F=2 → F′=2 transition). The black square points represent the transmission spectrum of the cold-atom optical filter realized by optical pumping method. The red circular points represent the transmission spectrum of the cold-atom optical filter realized by Faraday magneto-optical effect. The blue and red curves are the Lorentz fitting curves respectively. Each point is obtained by averaging 20 measurements.
We also measure the transmission spectrum of the cold-atom optical filter realized by Faraday magneto-optical effect under the same transition. The pump laser is blocked during the whole process. The homogeneous magnetic field (around 4 Gs) along the probe laser direction is generated by a pair of energized coils. We still keep the MOT off at 1.5 s, then turn on the probe laser and homogeneous magnetic field at 1.501 s to measure the cold-atom FADOF’s transmitted signal. According to the same data recording and processing method, we get the transmission spectrum, seeing the red circular points in Figure 4, the red curve is its Lorentz fitting curve. From the comparison, it can be clearly seen that the cold-atom optical filters implemented by the two different schemes all have an ultranarrow bandwidth close to the natural linewidth of atomic transition. However, the peak transmission of the cold-atom optical filter realized by optical pumping method (15.6%) is nearly 14 times higher than that of the cold-atom optical filter realized by Faraday magneto-optical effect (1.14%), which is a significant progress made in this study.
Apart from narrow bandwidth and high peak transmission, figure of merit [35, 36] (FOM) is another important quality measure for the filter, defined as FOM = Tmax/ENBW. Tmax is the maximum transmission of the filter, ENBW = ∫T(ν)dν/Tmax is the equivalent noise bandwidth, and ν is the optical frequency. After calculation, the cold-atom optical filter realized by optical pumping method in this study has a FOM value of 23.6 GHz−1, which is 14 times larger than the largest FOM achieved by thermal vapor atomic optical filter published to date [37]. Here, we list the peak transmission, ENBW, and FOM parameters for a variety of filters given in the literature, as shown in Table 2.
To investigate the influence of pump laser power on the peak transmission of cold-atom optical filter, we vary its power under the probe laser power kept at 3 μW. From Figure 5A, it can be seen that the transmission increases with the pump laser power and reaches the maximum when the pump laser power is 1 mW. After that, continue to increase the pump laser power, due to the pump laser exerts a greater force on the cold atoms, resulting in the diffusion and displacement of cold atomic cloud (see the upper right inset in Figure 5A), the transmission of the cold-atom optical filter decreases rapidly. Figure 5B shows that when the pump laser power is 1 mW, the intensity of the transmitted signal gradually becomes saturated with the increase of the probe laser power. Since the intensity of the incident laser increases linearly with the probe laser power, the measured transmission points show a trend of first increasing and then decreasing [31, 41]. From the green fitting curve, we can conclude that when the probe laser power is about 2 μW, the transmission of the filter reaches a maximum.
FIGURE 5. (A) Dependence of the peak transmission on pump laser power. The probe laser power is kept at 3 μW. The upper right inset is an image of cold atomic cloud observed when the external incident laser power is high. (B) Dependence of the transmitted signal and peak transmission on probe laser power. The pump laser power is kept at 1 mW, and the green curve is the fitting curve of the green points.
Finally, we use a simple and direct method to verify the effect of optical pumping. Before the probe laser enters the cold-atom optical filter, let it pass through a quarter-wave plate, which can convert the linearly polarized probe laser into circularly polarized. Then, removing the two GTPs and using the PD to receive the absorption signal of trapped cold atoms. In combination with the energy levels shown in Figure 1, rotating the above quarter-wave plate, when the probe laser is σ− circularly polarized, it can be absorbed by the atoms located on the ground state F=2, mF = +2 level, making the atoms transition to the excited state F′=2, mF′ = +1. However, when the probe laser is σ+ circularly polarized, because there is no excited state energy level can transition, the absorption of light by cold atoms will be greatly reduced.
Under different polarization states of the probe laser, the absorptivity change actually measured in this study is shown in Figure 6, which is indeed consistent with the above analysis. As the probe laser polarization changes from σ− to σ+, the absorptivity decreases gradually, and vice versa. If the optical pumping does not work or the effect is not obvious, the atoms will be evenly distributed on all the sublevels of the ground state F=2, then there will be no significant difference in absorptivity. In addition, it should be noted that when the probe laser polarization is σ+, the absorptivity is still 50% instead of 0. This may be because the optical pumping does not accumulate all the atoms to F=2, mF = +2 level, and there are still a small number of atoms distributed in other Zeeman sublevels, which results in the absorption of σ+ circularly polarized probe laser. And this is also the reason for the deviation between the theoretical simulation “transmission” and the experimental measurement “transmission”. But in any case, this study achieves a good optical pumping effect, which also greatly improves the transmission of the cold-atom optical filter.
In conclusion, using laser cooling and optical pumping technologies, we demonstrate a cold-atom optical filter which operates on the 87Rb F=2 → F′=2 transition at 780 nm, with an ultranarrow bandwidth of 6.6(4) MHz and a peak transmission of 15.6%. The scheme we proposed effectively solves the problem of the decrease of filter transmission due to the loss of atom number. Experimental results show that, under the same transition, the peak transmission of our achieved cold-atom optical filter (15.6%) is nearly 14 times higher than that of the cold-atom optical filter realized by Faraday magneto-optic effect (1.14%).
We can further improve the transmission of cold-atom optical filter by increasing the optical thickness (OD), which is specifically influenced by the density of gas atoms and the length of atomic medium [42]. An ultranarrow-bandwidth filter with high transmission might reveal active optical frequency standard [43] without an additional locked pump laser. In this case, the pump laser will not disturb the center frequency of the output laser, which is determined by the atomic transmission. Furthermore, trapping atoms in MOT on chip [44, 45] can expand optical frequency standard’s applications in the future, for its less cost and complexity. And the problem of cold-atom filter operating in pulse mode may be solved by using optical molasses to prepare cold atoms. Finally, a cold-atom optical filter using narrower natural linewidth has the potential to be applied in more ultranarrow-bandwidth-required fields.
The original contributions presented in the study are included in the article/supplementary material, further inquiries can be directed to the corresponding authors.
BL, WZ, and JC conceived the idea of the cold-atom optical filtering enhanced by optical pumping. XG performed the experiments and carried out the theoretical calculations. XG wrote the manuscript. TS, JM, and JZ provided revisions. All authors contributed equally to the discussions of the results.
This research was funded by the National Natural Science Foundation of China (61771067, 11704361); Innovation Program for Quantum Science and Technology (2021ZD0303200); Wenzhou Key Scientific and Technological Innovation R&D Project (Application No. 2019ZG0029); Wenzhou Major Science & Technology Innovation Key Project (Grant No. ZG2020046); China Postdoctoral Science Foundation (BX2021020).
We give our best wishes on the occasion of Yiqiu Wang’s 90th birthday. The authors have learned a lot from Wang throughout their 10 years of collaboration and communication. For their research on cold atoms and optical filter, authors most frequently refer to Wang’s book Laser Cooling and Trapping of Atoms, and Principle of Quantum Frequency Standard. Wang’s rigorous academic attitude and profound professional knowledge are worth learning from each of us. We sincerely wish Wang to be healthy and happy.
The authors declare that the research was conducted in the absence of any commercial or financial relationships that could be construed as a potential conflict of interest.
All claims expressed in this article are solely those of the authors and do not necessarily represent those of their affiliated organizations, or those of the publisher, the editors and the reviewers. Any product that may be evaluated in this article, or claim that may be made by its manufacturer, is not guaranteed or endorsed by the publisher.
1. Marling JB, Nilsen J, West LC, Wood LL. An ultrahighQ isotropically sensitive optical filter employing atomic resonance transitions. J Appl Phys (1979) 50(2):610–4. doi:10.1063/1.326074
2. Shay TM, Chung YC. Ultrahigh-resolution, wide-field-of-view optical filter for the detection of frequency-doubled Nd:YAG radiation. Opt Lett (1988) 13(6):443–5. doi:10.1364/ol.13.000443
3. Gelbwachs JA. Atomic resonance filters. IEEE J Quan Electron (1988) 24(7):1266–77. doi:10.1109/3.963
4. Gelbwachs JA. Active wavelength-shifting in atomic resonance filters. IEEE J Quan Electron (1990) 26(6):1140–7. doi:10.1109/3.108112
5. Menders J, Searcy P, Roff K, Korevaar E. Blue cesium Faraday and Voigt magneto-optic atomic line filters. Opt Lett (1992) 17(19):1388–90. doi:10.1364/ol.17.001388
6. Billmers RI, Gayen SK, Squicciarini MF, Contarino VM, Scharpf WJ. Experimental demonstration of an excited-state Faraday filter operating at 532 nm. Opt Lett (1995) 20(1):106–8. doi:10.1364/ol.20.000106
7. Ohman Y. On some new auxiliary instruments in astrophysical research VI. A tentative monochromator for solar work based on the principle of selective magnetic rotation. Stockholms Obs Ann (1956) 19(4):9–11.
9. Yin B, Shay TM. Faraday anomalous dispersion optical filter for the Cs 455 nm transition. IEEE Photon Technol Lett (1992) 4(5):488–90. doi:10.1109/68.136496
10. Wang Y, Zhang X, Wang D, Tao Z, Zhuang W, Chen J. Cs Faraday optical filter with a single transmission peak resonant with the atomic transition at 455 nm. Opt Express (2012) 20(23):25817–25. doi:10.1364/oe.20.025817
11. Ling L, Bi G. Isotope 87Rb Faraday anomalous dispersion optical filter at 420 nm. Opt Lett (2014) 39(11):3324–7. doi:10.1364/ol.39.003324
12. Xue X, Pan D, Zhang X, Luo B, Chen J, Guo H. Faraday anomalous dispersion optical filter at 133Cs weak 459 nm transition. Photon Res (2015) 3(5):275–8. doi:10.1364/prj.3.000275
13. BloomLiuMenders SHCSJ, Benson K, Korevaar E. Ultranarrow line filtering using a Cs Faraday filter at 852 nm. Opt Lett (1991) 16(11):846–8. doi:10.1364/ol.16.000846
14. Zielińska JA, Beduini FA, Godbout N, Mitchell MW. Ultranarrow Faraday rotation filter at the Rb D1 line. Opt Lett (2012) 37(4):524–6. doi:10.1364/ol.37.000524
15. Wang Y, Zhang S, Wang D, Tao Z, Hong Y, Chen J. Nonlinear optical filter with ultranarrow bandwidth approaching the natural linewidth. Opt Lett (2012) 37(19):4059–61. doi:10.1364/ol.37.004059
16. Zentile MA, Whiting DJ, Keaveney J, Adams CS, Hughes IG. Atomic Faraday filter with equivalent noise bandwidth less than 1 GHz. Opt Lett (2015) 40(9):2000. doi:10.1364/ol.40.002000
17. Dick DJ, Shay TM. Ultrahigh-noise rejection optical filter. Opt Lett (1991) 16(11):867–9. doi:10.1364/ol.16.000867
18. Tang J, Wang Q, Li Y, Zhang L, Gan J, Duan M, et al. Experimental study of a model digital space optical communication system with new quantum devices. Appl Opt (1995) 34(15):2619–22. doi:10.1364/ao.34.002619
19. Zhang J, Gao G, Wang B, Guan X, Yin L, Chen J, et al. Background noise resistant underwater wireless optical communication using Faraday atomic line laser and filter. J Lightwave Technol (2022) 40(1):63–73. doi:10.1109/jlt.2021.3118447
20. Popescu A, Walther T. On an ESFADOF edge-filter for a range resolved Brillouin-lidar: The high vapor density and high pump intensity regime. Appl Phys B (2010) 98(4):667–75. doi:10.1007/s00340-009-3857-5
21. Yang Y, Cheng X, Li F, Hu X, Lin X, Gong S. A flat spectral Faraday filter for sodium lidar. Opt Lett (2011) 36(7):1302–4. doi:10.1364/ol.36.001302
22. Wanninger P, Valdez EC. Diode-laser frequency stabilization based on the resonant Faraday effect. IEEE Photon Technol Lett (1992) 4(1):94–6. doi:10.1109/68.124888
23. Tao Z, Hong Y, Luo B, Chen J, Guo H. Diode laser operating on an atomic transition limited by an isotope 87Rb Faraday filter at 780 nm. Opt Lett (2015) 40(18):4348–51. doi:10.1364/ol.40.004348
24. Gayen SK, Billmers RI, Contarino VM, Squicciarini MF, Scharpf WJ, Yang G, et al. Induced-dichroism-excited atomic line filter at 532 nm. Opt Lett (1995) 20(12):1427–9. doi:10.1364/ol.20.001427
25. He Z, Zhang Y, Wu H, Yuan P, Liu S. Theoretical model for an atomic optical filter based on optical anisotropy. J Opt Soc Am B (2009) 26(9):1755–9. doi:10.1364/josab.26.001755
26. Turner LD, Karaganov V, Teubner PJO, Scholten RE. Sub-Doppler bandwidth atomic optical filter. Opt Lett (2002) 27(7):500–2. doi:10.1364/ol.27.000500
27. Cerè A, Parigi V, Abad M, Wolfgramm F, Predojević A, Mitchell MW. Narrowband tunable filter based on velocity-selective optical pumping in an atomic vapor. Opt Lett (2009) 34(7):1012–4. doi:10.1364/ol.34.001012
28. Liu S, Zhang Y, Wu H, Yuan P. Ultra-narrow bandwidth atomic filter based on optical-pumping-induced dichroism realized by selectively saturated absorption. Opt Commun (2012) 285(6):1181–4. doi:10.1016/j.optcom.2011.10.042
29. Zhang X, Tao Z, Zhu C, Hong Y, Zhuang W, Chen J. An all-optical locking of a semiconductor laser to the atomic resonance line with 1 MHz accuracy. Opt Express (2013) 21(23):28010–8. doi:10.1364/oe.21.028010
30. Zhuang W, Hong Y, Gao Z, Zhu C, Chen J. Ultranarrow bandwidth nonlinear Faraday optical filter at rubidium D2 transition. Chin Opt Lett (2014) 12(10):101204. doi:10.3788/col201412.101204
31. Zhuang W, Zhao Y, Wang S, Fang Z, Fang F, Li T. Ultranarrow bandwidth Faraday atomic filter approaching natural linewidth based on cold atoms. Chin Opt Lett (2021) 19(3):030201. doi:10.3788/col202119.030201
32. Liu S, Zhang Y, Wu H, Yuan P. Atomic filter with large scale tunability. J Opt Soc Am B (2011) 28(5):1100–3. doi:10.1364/josab.28.001100
33. Luo B, Yin L, Xiong J, Chen J, Guo H. Induced-Dichroism-Excited atomic line filter at 1529 nm. IEEE Photon Technol Lett (2018) 30(17):1551–4. doi:10.1109/lpt.2018.2860947
34. Labeyrie G, Miniatura C, Kaiser R. Large Faraday rotation of resonant light in a cold atomic cloud. Phys Rev A (Coll Park) (2001) 64(3):033402. doi:10.1103/physreva.64.033402
35. Zentile MA, Keaveney J, Mathew RS, Whiting DJ, Adams CS, Hughes IG. Optimization of atomic Faraday filters in the presence of homogeneous line broadening. J Phys B: Mol Opt Phys (2015) 48(18):185001. doi:10.1088/0953-4075/48/18/185001
36. Kiefer W, Löw R, Wrachtrup J, Gerhardt I. Na-Faraday rotation filtering: The optimal point. Sci Rep (2014) 4(1):6552. doi:10.1038/srep06552
37. Logue FD, Briscoe JD, Pizzey D, Wrathmall SA, Hughes IG. Better magneto-optical filters with cascaded vapor cells. Opt Lett (2022) 47(12):2975–8. doi:10.1364/ol.459291
38. Hu Z, Sun X, Liu Y, Fu L, Zeng X. Temperature properties of Na dispersive Faraday optical filter at D1 and D2 line. Opt Commun (1998) 156(4-6):289–93. doi:10.1016/s0030-4018(98)00461-1
39. Zhang Y, Jia X, Ma Z, Wang Q. Potassium Faraday optical filter in line-center operation. Opt Commun (2001) 194(1-3):147–50. doi:10.1016/s0030-4018(01)01222-6
40. Luo B, Yin L, Xiong J, Chen J, Guo H. Signal intensity influences on the atomic Faraday filter. Opt Lett (2018) 43(11):2458–61. doi:10.1364/ol.43.002458
41. Zheng B, Cheng H, Meng Y, Xiao L, Wan J, Liu L. Observation of Faraday rotation in cold atoms in an integrating sphere. Chin Phys Lett (2014) 31(7):073701. doi:10.1088/0256-307x/31/7/073701
42. Wan J, Wang X, Zhang X, Meng Y, Wang W, Sun Y, et al. Quasi-one-dimensional diffuse laser cooling of atoms. Phys Rev A (Coll Park) (2022) 105(3):033110. doi:10.1103/physreva.105.033110
43. Zhuang W, Chen J. Active Faraday optical frequency standard. Opt Lett (2014) 39(21):6339–42. doi:10.1364/ol.39.006339
44. Nshii CC, Vangeleyn M, Cotter JP, Griffin PF, Hinds EA, Ironside CN, et al. A surface-patterned chip as a strong source of ultracold atoms for quantum technologies. Nat Nanotechnol (2013) 8(5):321–4. doi:10.1038/nnano.2013.47
Keywords: optical filter, cold atom, optical pumping, induced circular dichroism, ultranarrow bandwidth
Citation: Guan X, Zhuang W, Shi T, Miao J, Zhang J, Chen J and Luo B (2022) Cold-atom optical filtering enhanced by optical pumping. Front. Phys. 10:1090483. doi: 10.3389/fphy.2022.1090483
Received: 05 November 2022; Accepted: 29 November 2022;
Published: 09 December 2022.
Edited by:
Andrea Bertoldi, ParisTech Institut d’Optique Graduate School, FranceReviewed by:
Lu Zhou, East China Normal University, ChinaCopyright © 2022 Guan, Zhuang, Shi, Miao, Zhang, Chen and Luo. This is an open-access article distributed under the terms of the Creative Commons Attribution License (CC BY). The use, distribution or reproduction in other forums is permitted, provided the original author(s) and the copyright owner(s) are credited and that the original publication in this journal is cited, in accordance with accepted academic practice. No use, distribution or reproduction is permitted which does not comply with these terms.
*Correspondence: Wei Zhuang, emh1YW5nd2VpQG5pbS5hYy5jbg==; Bin Luo, bHVvYmluQGJ1cHQuZWR1LmNu
Disclaimer: All claims expressed in this article are solely those of the authors and do not necessarily represent those of their affiliated organizations, or those of the publisher, the editors and the reviewers. Any product that may be evaluated in this article or claim that may be made by its manufacturer is not guaranteed or endorsed by the publisher.
Research integrity at Frontiers
Learn more about the work of our research integrity team to safeguard the quality of each article we publish.