- 1College of Physics and Optoelectronic Engineering, Shenzhen University, Shenzhen, China
- 2Shenzhen Nuoan Technology Co. LTD, Shenzhen, China
- 3School of Electronic and Communication Engineering, Shenzhen Polytechnic, Shenzhen, China
- 4College of Liberal Arts and Sciences, National University of Defense Technology, Changsha, China
The high repetition rate, widely tunable, picosecond mid-infrared laser plays an important role in various fields. In this paper, the single-pass optical parametric generator (OPG) pumped by Yb-fiber laser based on MgO: PPLN is proposed. The Yb-fiber laser is mode locked by a nonlinear amplifying loop mirror (NALM) with an all-polarization-maintaining (PM) structure. A maximum power of 6.2 W was obtained with a repetition rate of 15.8 MHz and pulse duration of 18 ps after amplification by cascaded fiber. Based on the principle of nonlinear frequency down-conversion, the tunable mid-infrared output wavelength of 2.76–3.98 μm was realized by varying the crystal grating periods and temperature. The maximum power was 500 mW at 3.22 μm, corresponding to a quantum conversion efficiency of 25%, and the relative power fluctuation was measured as 0.37% over 30 min.
Introduction
The mid-infrared band of 2.5–5 μm is in the transmission window of the atmosphere and covers the absorption spectrum of most molecules, playing a wide range of roles in the environmental, medical, communication, and defense fields (1), (2). In particular, the high repetition rate (kHz and above) and widely tunable mid-infrared picosecond laser has important applications in trace gas analysis, material characterization, minimally invasive laser therapy, and optical frequency comb due to its short pulse interval and high peak power (3), (4), (5). At present, different methods have been proposed to obtain mid-infrared lasers, including gas lasers (6), fiber lasers (7, 8), quantum cascade lasers (9), nonlinear frequency conversion (10), (11), (12), etc. The nonlinear frequency conversion is the main means to produce mid-infrared picosecond and femtosecond pulses, such as optical parametric oscillator (OPO), optical parametric generator (OPG), and difference frequency generator (DFG). OPG has a single-pass structure, which not need to introduce pulse-synchronized signal light, and build a complex resonant cavity. With the features of a simple structure, wide tuning range, and low cost, it has attracted much attention in the preparation of current mid-infrared light sources.
The common mid-infrared nonlinear crystals include KTP (13), KTA (14), ZGP (15), BGSe (16), and PPLN (17), (18). Among these crystals, PPLN, as a widely used and mature quasi-phase-matching crystal, overcomes the optical walk-off effect in the frequency conversion process, has a high nonlinear coefficient (d33 = 27.2 p.m./V), a wide transparency range (0.5–5 μm), and no two-photon absorption for the conventional near-IR pumping sources. In particular, MgO: PPLN, by adding 5% MgO to PPLN, can significantly improve the optical refractive threshold and optical damage of the crystal while retaining a high nonlinear coefficient. The wide tuning and high efficiency mid-infrared output can be achieved in the crystal by different grating period designs as well as temperature tuning.
Recently, there have been many studies on mid-infrared generation based on MgO: PPLN crystal. J. Wueppen’s group presented the OPG system using MgO: PPLN pumped by a 1,064 nm Nd: YVO4 laser, a wide tuning range of 2.3–2.8 µm laser output was achieved with a repetition rate of 20 MHz (19). S. Parsa et al. proposed a Yb-fiber pumped picosecond OPO at 80 MHz based on MgO: PPLN, an average power of 2 W was achieved at a continuous tuning range of 2,198–4,028 nm, and measured the power stability better than 0.31–0.46% rms over 1 h (20). Jin Guangyong et al. reported a single resonant MgO: PPLN-OPO and single-pass MgO: PPLN-OPA pumped by fiber laser respectively, and they obtained output power of 3.02 W at 3.8 μm with a repetition rate of 140 kHz (21). Chen Fei et al. presented a MgO: PPLN-based OPO pumped by an all-fiber Yb-doped fiber laser. A maximum power of 79.1 W at 1,064.1 nm was obtained with a repetition rate of 300 kHz and pulse duration of 200 ns (22). However, most of these studies focus on the OPO approach and pumping with solid-state laser. The single-pass OPG system using fiber laser pumping requires a higher pumping threshold, but the structure is simpler, and the pump source could be constructed with all-PM components, which greatly reduces the size of the system and provides a more stable operation.
In this paper, we demonstrated a mid-infrared OPG regime pumped by an all-PM mode-locked Yb-fiber laser. A 2.76–3.98 μm widely tunable mid-infrared output was obtained and a maximum output power of 500 mW at 3.22 μm was achieved. Moreover, the relative power fluctuation was measured as 0.37% over 30 min. The results illustrated a simple method to obtained wide-tunable mid-infrared source with a stable output.
Experimental setup
Figure 1 illustrates the experimental setup of mid-infrared single-pass OPG pumped by Yb-fiber laser. The pump source was composed of a fiber oscillator and amplifier. As shown in Figure 1A, the oscillator was based on the principle of NALM, using the interference of the Sagnac loop in the resonant cavity to act as a saturable absorber to achieve mode-locking (23). In contrast to the real saturable absorbers, the NALM would benefit passive mode-locking with considerably high damage threshold and extremely short response time (24), (25). The Yb-doped fiber (PM-YDF-6/125, Nufern) with a length of 1.5 m was used for the gain medium to provide enough nonlinear and pulse amplification. The fiber Bragg grating (FBG) was used for end-face reflection in the laser cavity, with a bandwidth of 5 nm and a reflectivity of 99%. The 50:50 output coupler (OC) was used to make the laser propagating in the coupler interference with the maximum contrast, so that NALM showed better strength permeability. The Phase shifter (PS) was used to provide π/2 non-reciprocal phase shift in the cavity, which could effectively reduce the mode-locking threshold.
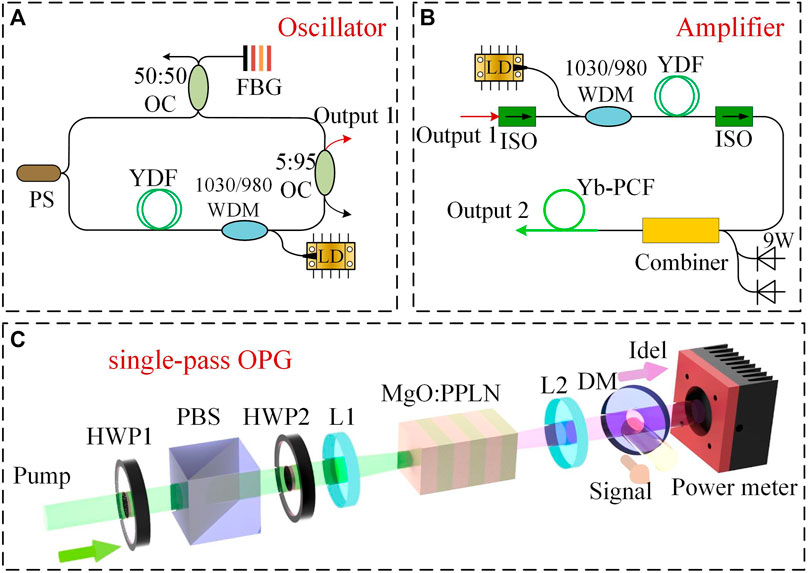
FIGURE 1. Experimental of fiber laser pumped MgO: PPLN OPG. (A) Fiber oscillator. (B) Cascaded fiber amplifier. (C) Mid-infrared generation using the OPG system.
Then the pulse from the oscillator was steered into the amplifier for power boosting, which would serve as a pump source in the subsequent OPG. As shown in Figure 1B, the amplifier adopted a cascaded fiber amplification structure which was composed of the pre-amplifier and main amplifier. The pre-amplifier was pumped by a single-mode laser diode (LD) at 976 nm, with a maximum output power of 680 mW, and 100 mW output power was achieved in 1.2 m YDF. The main amplifier was pumped by two identical multi-mode LDs at 976 nm, each with a maximum output power of 9 W, and 6.2 W output power was obtained in 2 m photonic crystal fiber (PM-PCF-14/135, NKT Photonics).
Finally, the pump beam was focused into the MgO: PPLN crystal by a spherical lens (L1, f = 75 mm). As shown in Figure 1C, the crystal was housed in an oven with a tuning range of 25–200°C and an accuracy of 0.1°C. The dimensions of the MgO: PPLN crystal were 10 × 1 × 25 mm3 (Width × Height × Length), and the grating periods were 28.3–30.5 μm with a step of 0.5 μm. Both end surfaces of the crystal were antireflection coated for three bands from 1,000 to 1,100 nm, 1,300–1700 nm, and 2,600–5,000 nm. The half-wave plate (HWP1) and polarization prism (PBS) form a power attenuator to change the pump power. The polarization was adjusted to be perpendicular by the HWP2 to satisfy the type-0 phase-matching condition (26). The generated signal light and idler light were collimated by a calcium fluoride Lens (L2, f = 75 mm), and then separated by a dichroic mirror (DM) to obtain a pure mid-infrared laser. The DM has a high transmittance (>95%) at 3–4 μm, and high reflectivity (>99.7%) at both 1.03μm and 1.4–1.7 μm. The mid-infrared output power was detected by a thermal power sensor (Thorlabs S401C).
Results and discussions
Output characteristics of the pump laser
When the pump power of LD was set to 150 mW, the oscillator achieved self-started mode-locking. As shown in Figure 2A, via using an optical spectrum analyzer (AQ6370D, Yokogawa), the measurement wavelength was centered at 1,032.5 nm with a 3-dB bandwidth of 0.28 nm. As depicted in Figure 2B, after amplification by cascaded gain fiber, the spectrum was broadened due to the influence of self-phase modulation (SPM), and the corresponding 10-dB bandwidth was 10 nm. Note that the highest power was at about 1,037 nm. Figure 2C gives the performance of the amplifier, it can be seen that the maximum output power reached 6.2 W at 16 W pump power. The corresponding pulse duration of 18 ps can be deduced from the measured autocorrelation trace by an autocorrelator (PulseCheck, APE), as illustrated in Figure 2D. In Figures 2E,F, via using an oscilloscope (MDO3032, Tektronix) and an RF spectrum analyzer (MDO32, Tektronix), we measured the time interval between adjacent pulses and pulse repetition rate of 63.2 ns and 15.8 MHz, respectively. The RF spectrum was very clean with a signal-to-noise ratio of 52 dB (RBW = 100 kHz).
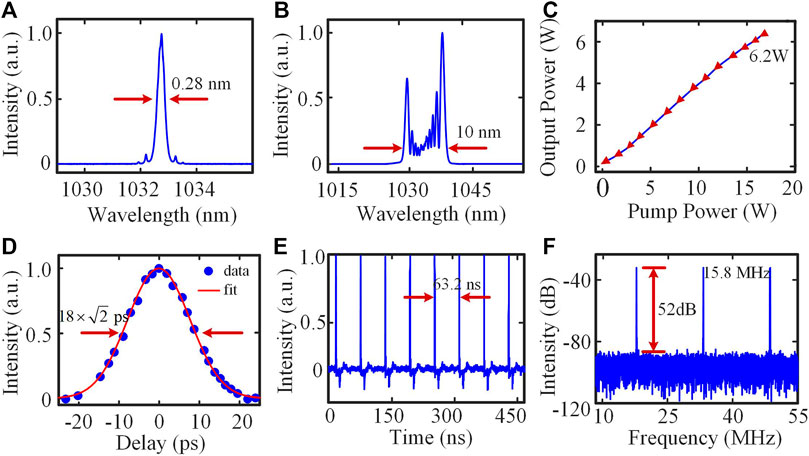
FIGURE 2. Characterization of the pump pulse. (A) and (B) are the measured spectrum of the output pulses before and after YDFL amplification. (C) Power curve. (D) Autocorrelation trace. (E) and (F) are the measured time interval between adjacent pulses and pulse repetition rate.
Mid-infrared output characteristics
In order to obtain a widely tunable mid-infrared output, we investigated the relationship between the output wavelength and the crystal temperature under different grating periods using the Fourier transform optical spectrum analyzer (Thorlabs OSA207C). As depicted in Figure 3A, the wavelength decreased with the increase of temperature and grating period. When the temperature was set to 40°C and the grating period was 28.3 μm, the maximum wavelength could be obtained at 3.98 μm. Continue to raise the temperature to 130°C and adjust the grating period to 30.5 μm, corresponding to a minimum wavelength of 2.76 μm. Figure 3B shows the corresponding spectral profile at grating periods of 30.5 and 28.3 μm, respectively.
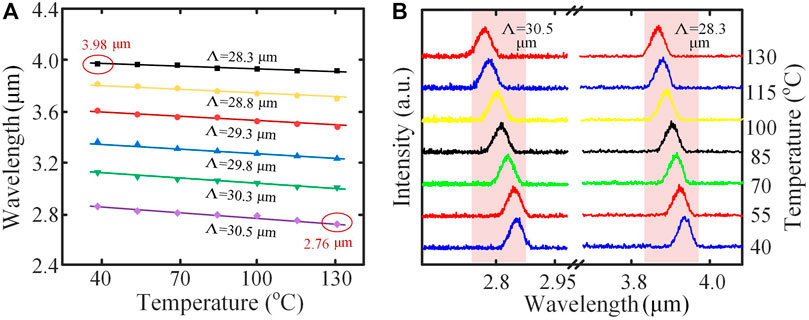
FIGURE 3. (A) Wavelength-tuning performance of the mid-infrared output. (B) Mid-infrared spectra at grating periods of 30.5 and 28.3 μm.
As observed in Figure 4A, we measured the output power of the mid-infrared at different wavelengths under 6.2 W pump power, and it can be seen that there was a maximum power of 500 mW at 3.22 μm, corresponding a grating period of 29.8 μm and temperature of 115°C. The quantum conversion efficiency
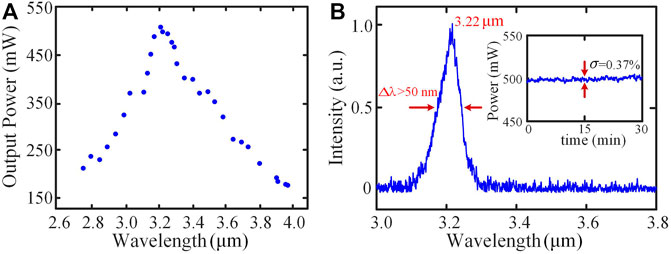
FIGURE 4. (A) Output power of mid-infrared at different wavelengths. (B) The spectra and power fluctuation of mid-infrared at maximum power.
Conclusion
In conclusion, we proposed a single-pass mid-infrared OPG regime pumped by an all-PM Yb-fiber laser at 1,032.5 nm based on multi-grating MgO: PPLN. The Yb-fiber laser was mode-locked by NALM with a nine-figure configuration, followed by a cascaded fiber amplification system to achieve an average power of 6.2 W pulse output, with a repetition rate of 15.8 MHz and pulse duration of 18 ps. By varying the crystal grating periods (28.3–30.5 μm) and temperature (40–130°C), the widely tunable (2.76–3.98 μm), picosecond mid-infrared laser at 15.8 MHz was obtained. Corresponding to a maximum output power of 500 mW at 3.22 μm and a quantum conversion efficiency of 25%. The whole system has high stability due to the all-PM fiber structure of the pump source, and the relative power fluctuation was 0.37% over 30 min. We believe that our results are meaningful in terms of mid-infrared generation in an all-PM fiber laser-pumped OPG system.
Data availability statement
The original contributions presented in the study are included in the article/Supplementary Materials, further inquiries can be directed to the corresponding author.
Author contributions
All authors listed have made a substantial, direct, and intellectual contribution to the work and approved it for publication.
Funding
This work was supported by the National Natural Science Foundation of China (12074264, 61905148); Natural Science Foundation of Guangdong Province, China (2022A1515010759); Guangdong Basic and Applied Basic Research Foundation (2020A1515110740); Department of Education of Guangdong Province (2022KTSCX310); Shenzhen Science and Technology Project of China (JCYJ20190808141011530, JCYJ20190808174201658, JCYJ20190808160205460); the Grant of Shenzhen Polytechnic (6022310027K, 6022312060K).
Conflict of interest
Author JY was employed by Shenzhen Nuoan Technology Co. LTD.
The remaining authors declare the research was conducted in the absence of any commercial or financial relationships that could be construed as a potential conflict of interest.
Publisher’s note
All claims expressed in this article are solely those of the authors and do not necessarily represent those of their affiliated organizations, or those of the publisher, the editors and the reviewers. Any product that may be evaluated in this article, or claim that may be made by its manufacturer, is not guaranteed or endorsed by the publisher.
References
1. Schliesser A, Picqué N, Hänsch TW. Mid-infrared frequency combs. Nat Photon (2012) 6:440–9. doi:10.1038/nphoton.2012.142
2. Yan M, Luo PL, Iwakuni K, Millot G, Hansch TW, Picque N. Mid-infrared dual comb spectroscopy with electro-optic modulators. Light Sci Appl (2017) 6:e17076. doi:10.1038/lsa.2017.76
3. Dixit N, Mahendra R, Naraniya OP, Gupta A. High repetition rate mid-infrared generation with singly resonant optical parametric oscillator using multi-grating periodically poled MgO:LiNbO3. Opt Laser Technol (2010) 42:18–22. doi:10.1016/j.optlastec.2009.04.012
4. Petrov KP, Goldberg L, Burns WK, Curl RF, Tittle FK. Detection of CO in air by diode-pumped 4.6-μm difference-frequency generation in quasi-phase-matched LiNbO3. Opt Lett (1996) 21:86–8. doi:10.1364/OL.21.000086
5. Li XH, Xu WS, Wang YM, Zhang XL, Hui ZQ, Zhang H, et al. Optical-intensity modulators with PbTe thermoelectric nanopowders for ultrafast photonics. Appl Mater Today (2022) 28:101546. doi:10.1016/j.apmt.2022.101546
6. Cui YL, Zhou ZY, Huang W, Li ZX, Wang ZF. Quasi-all-fiber structure CW mid-infrared laser emission from gas-filled hollow-core silica fibers. Opt Laser Technol (2020) 121:105794. doi:10.1016/j.optlastec.2019.105794
7. Bawden N, Sapir OH, Jackson SD, Ottaway DJ. Ultrafast 3.5 µm fiber laser. Opt Lett (2021) 46:1636–9. doi:10.1364/OL.418162
8. Sujecki S, Sojka L, Pawlik EB, Tang Z, Furniss D, Seddon AB, et al. Modelling of a simple Dy3+ doped chalcogenide glass fibre laser for mid-infrared light generation. Opt Quant Electron (2010) 42:69–79. doi:10.1007/s11082-010-9421-z
9. Goekden B, Bai Y, Bandyopadhyay N, Slivken S, Razeghi M. Broad area photonic crystal distributed feedback quantum cascade lasers emitting 34 W at λ∼4.36 μm. Appl Phys Lett (2010) 13:131112. doi:10.1063/1.3496043
10. Huang JY, Lin HF, Guo CY, Wang JT, Yang JB, Yan PG. Enhanced cascaded up-conversion from periodically poled lithium niobate in a double-pass configuration. Infrared Phys Technol (2022) 124:104206. doi:10.1016/j.infrared.2022.104206
11. Henderson A, Stafford R. Low threshold, singly-resonant CW OPO pumped by an all-fiber pump source. Opt Express (2006) 14:767–72. doi:10.1364/OPEX.14.000767
12. Wang XC, Wang YH, Zheng H, Liu HZ, Yu JY, Wang ZJ, et al. Wide-tunable mid infrared intra-cavity optical parametric oscillator based on multi-period MgO:PPLN. Curr Opt Photon (2021) 5:59–65. doi:10.3807/COPP.2021.5.1.059
13. Tiihonen M, Pasiskevicius V, Fragemann A, Canalias C, Laurell F. Ultrabroad gain in an optical parametric generator with periodically poled KTiOPO4. Appl Phys B (2006) 85:73–7. doi:10.1007/s00340-006-2253-7
14. Dong C, Xu H, Wang X, Yang F, Shen Y, Bo Y, et al. High energy picosecond mid-infrared optical parametric amplifier based on KTiOAsO4 crystal. Infrared Phys Technol (2019) 97:440–3. doi:10.1016/j.infrared.2019.01.015
15. Sanchez D, Hemmer M, Baudish M, Cousin SL, Zawilski K, Schunemann P, et al. 7 μm, ultrafast, sub-millijoule-level mid-infrared optical parametric chirped pulse amplifier pumped at 2 μm. Optica (2016) 3:147–50. doi:10.1364/OPTICA.3.000147
16. Zhang JW, Wang Q, Hao JJ, Liu HY, Yao JY, Li Z, et al. Broadband, few-cycle mid-infrared continuum based on the intra-pulse difference frequency generation with BGSe crystals. Opt Express (2020) 28:37903–9. doi:10.1364/OE.411664
17. Prakash O, Lim HH, Kim BJ, Pandiyan K, Cha M, Rhee BK. Collinear broadband optical parametric generation in periodically poled lithium niobate crystals by group velocity matching. Appl Phys B (2008) 92:535–41. doi:10.1007/s00340-008-3140-1
18. Ding X, Zhang SM, Ma HM, Pang M, Yao JQ, Li Z. Continuous-wave mid-infrared intracavity singly resonant optical parametric oscillator based on periodically poled lithium niobate. Chin Phys B (2008) 17:02111674–06. doi:10.1088/1674-1056/17/1/03
19. Wueppen J, Jungbluth B, Taubner T, Loosen P. Ultrafast tunable mid IR source. In: Proceedings of the International Conference on Infrared Millimeter and Terahertz Waves; 02-07 October 2011; Houston, TX, USA (2011).
20. Parsa S, Kumar SC, Nandy B, Zadeh M. Yb-fiber-pumped, high-beam-quality, idler-resonant mid-infrared picosecond optical parametric oscillator. Opt Express (2019) 27:25436–44. doi:10.1364/OE.27.025436
21. Chen B, Wu S, Yu Y, Wu C, Wang Y, Jin G. 3.8 μm mid-infrared optical parametric amplifier based on MgO:PPLN crystal. Infrared Phys Technol (2020) 111:103448. doi:10.1016/j.infrared.2020.103448
22. He Y, Ji Y, Wan H, Yu D, Zhang K, Pan Q, et al. High-power mid-infrared pulse MgO:PPLN optical parametric oscillator pumped by linearly polarized Yb-doped all-fiber laser. Opt Laser Technol (2022) 146:107545–5. doi:10.1016/j.optlastec.2021.107545
23. Mayer S, Grosinger W, Fellinger J, Winkler G, Perner LW, Droste S, et al. Flexible all-PM NALM Yb:fiber laser design for frequency comb applications: Operation regimes and their noise properties. Opt Express (2020) 28:18946. doi:10.1364/OE.394543
24. Hänsel W, Hoogland H, Giunta M, Schmid S, Steinmetz T, Doubek R, et al. All polarization-maintaining fiber laser architecture for robust femtosecond pulse generation. Appl Phy B (2017) 123:331–40. doi:10.1007/978-3-319-64346-5_18
25. Li XH, An MQ, Li G, Han YH, Guo PL, Chen EC, et al. MOF-derived porous dodecahedron rGO-Co3O4 for robust pulse generation. Adv Mater Inter (2022) 9:2101933. doi:10.1002/admi.202101933
26. Myers LE, Eckardt RC, Fejer MM, Byer RL, Bosenberg WR, Pierce JW. Quasi-phase-matched optical parametric oscillators in bulk periodically poled LiNbO3. J Opt Soc Am B (1995) 12:2102–16. doi:10.1364/JOSAB.12.002102
Keywords: mid-infrared laser, optical parametric generator, ultrafast, MgO: PPLN, mode-lock laser
Citation: Li B, Yin J, Wang J, Yang J and Yan P (2022) 2.76–3.98 μm picosecond mid-infrared optical parametric generation in a muti-grating MgO: PPLN. Front. Phys. 10:1082520. doi: 10.3389/fphy.2022.1082520
Received: 28 October 2022; Accepted: 14 November 2022;
Published: 29 November 2022.
Edited by:
Houkun Liang, Sichuan University, ChinaReviewed by:
Haroldo Hattori, University of New South Wales, AustraliaXiaohui Li, Shaanxi Normal University, China
Copyright © 2022 Li, Yin, Wang, Yang and Yan. This is an open-access article distributed under the terms of the Creative Commons Attribution License (CC BY). The use, distribution or reproduction in other forums is permitted, provided the original author(s) and the copyright owner(s) are credited and that the original publication in this journal is cited, in accordance with accepted academic practice. No use, distribution or reproduction is permitted which does not comply with these terms.
*Correspondence: Peiguang Yan, eWFucGdAc3p1LmVkdS5jbg==