- 1Key Laboratory of Earth and Planetary Physics, Institute of Geology and Geophysics, Chinese Academy of Sciences, Beijing, China
- 2France-China Joint Laboratory for Evolution and Development of Magnetotactic Multicellular Organisms, Chinese Academy of Sciences, Beijing, China
- 3College of Earth and Planetary Sciences, University of Chinese Academy of Sciences, Beijing, China
- 4Beijing National Observatory of Space Environment, Institute of Geology and Geophysics, Chinese Academy of Sciences, Beijing, China
The elimination of geomagnetic field (GMF), also called hypomagnetic field (HMF), is one of the major environmental hazards faced by deep-space astronauts and the workers in magnetically shielded rooms on Earth. We previously reported that long-term HMF exposure impaired adult hippocampal neurogenesis (AHN) and cognition by reducing endogenous reactive oxygen species (ROS) levels in adult neural stem cells (aNSCs). In addition to the aNSCs themselves, adult neurogenesis is also regulated by the local environment, i.e., the neurogenic niche. Neurogenic niche is mainly composed of astrocyte, microglia, and vascular system. However, whether the HMF exposure affects the neurogenic niche in hippocampus remains unknown. In this study, we investigated the effects of the HMF exposure on the neurogenic niche and adult neurogenesis in hippocampus, as well as the cognitive function in mice. The HMF is simulated by using the newly upgraded double-wrapped coils, different with our previous coils, which are capable of providing a very low-strength static magnetic field and identical electromagnetic field background between the HMF group and the GMF group. Here, we for the first time clearly revealed that 8-week HMF exposure significantly induced microglia activation and increased the number of astrocytes in hippocampal dentate gyrus (DG), suggesting the abnormalities in the neurogenic niche. Meanwhile, 8-week HMF exposure also markedly reduced proliferation and differentiation of aNSCs in the DG, and impaired the cognitive behavior of mice, consistent with our previous findings. In addition, we also found that 8-week HMF exposure significantly induced anxiety-like behaviors of mice. In summary, this study indicates that 8-week HMF exposure induces the neurogenic niche abnormalities, contributing to the AHN impairments, thus leads to the cognitive dysfunction and anxiety-like behaviors in mice.
Introduction
Hypomagnetic field (HMF), space radiation, and microgravity are major environmental hazards faced by deep-space astronauts. On Earth, some staff also need to work in some artificial HMF environments such as magnetically shielded rooms. Thus, studying the effects of HMF exposure on living organisms is a unique platform for humans to understand the adaptability of life to extreme environment. In addition to the harm of space radiation on living organisms due to the absence of geomagnetic field (GMF) [1–7], the HMF itself has also many adverse influences on animal development, physiology, circadian rhythms, learning and memory, etc. [8–15]. However, the neural mechanisms involved in the cognitive dysfunctions are poorly understood. Adult hippocampal neurogenesis (AHN) plays important regulatory roles in hippocampal-dependent cognition and spatial orientation, which involves proliferation and differentiation of adult neural stem cells (aNSCs), survival and integration of newborn neurons into pre-existing neuronal networks [16,17]. We previously reported that long-term HMF exposure could cause the impairments of AHN in mice, thus leading to their cognitive dysfunction. That is the first time to demonstrate that HMF has negative effect on AHN and cognitive function by modulating the cellular reactive oxygen species (ROS) in vivo [18]. We also found that long-term HMF exposure increased cellular ROS in the dentate gyrus (DG) and cornu ammonis (CA) regions of the hippocampus by modulating the key genes expression of the oxidative stress and antioxidant defense [19]. In addition to the above findings, adult neurogenesis is also regulated by the local environment, i.e., the neurogenic niche. The neurogenic niche is the microenvironment supporting the neural progenitor cells, which mainly includes astrocytes, microglia, and the vascular system. It is a key factor affecting many aspects of neurogenesis, including the proliferation of aNSCs, the differentiation into different lineages, and the survival of newborn cells [20,21]. Astrocytes participate in the regulation of synaptic integration of hippocampal newborn neurons, which is crucial for the maturation of dendritic cells and the functional integration of new neurons, as well as the survival of these cells in the critical period of maturation [22–24]. Microglia are the immune cells in the brain, which play an important role in removing cell debris and promoting adult neurogenesis. Static microglia are highly branched, which can phagocytize and clear the newly apoptotic cells of subgranular zone (SGZ) [25,26]. While under the condition of injury or disease, microglia become active, whose cell body is hypertrophic and amebic-like, and start the inflammatory reaction by secreting proinflammatory cytokines and phagocytizing dead cells [27–29]. Phagocytic microglia can be regarded as a sensor of local cell death to regulate the dynamic balance between cell proliferation and survival in neurogenesis [30]. However, whether the HMF exposure affects the neurogenic niche in hippocampus remains unknown.
In this study, we investigated the effects of the HMF exposure on the neurogenic niche, neurogenesis and cognition by using double-wrapped coils. Compared with the helmholtz coils, the advantages of double-wrapped coils are that GMF and HMF groups have similar ohmic heating and magnetomechanical effects, and easy to double-blind operation. Here, for the first time, we found that 8-week HMF exposure significantly altered the morphological activation of microglia and increased the number of astrocytes, which suggested that the 8-week HMF exposure impaired the balance of AHN microenvironment. We again confirmed that 8-week HMF exposure indeed inhibited the proliferation and differentiation of aNSCs, thus led to the anxiety-like and cognitive dysfunction behaviors in mice.
Materials and methods
Animals
Adult 7-week-old male mice (C57BL/6J) were housed in the experimental coils to acclimate to the exposure environment for 1 week, then started the HMF/GMF exposure experiments. The mice were randomly allocated to the GMF and HMF exposure groups. Brain samples for proliferation and differentiation of aNSCs were collected at 0, 4, 8, and 12 weeks, respectively. After determining the time point at which the HMF exposure had explicit negative effect on hippocampal neurogenesis, behavioral tests and neurogenic niche experiments were performed. All animals were housed under a constant temperature (23 ± 1°C) with a 12:12 h light: dark cycle. Water and food were provided ad libitum. Ten mice in each group were used for the behavioral tests. And four mice in each group were used for immunofluorescence analysis. The body weight of mice and the relative volume of DG area in hippocampus were comparable between the GMF- and HMF-exposed mice during experimental duration (Supplementary Figure S1). All procedures and husbandry were performed according to protocols approved by the Institutional Animal Care and Use Committee at the Institute of Geology and Geophysics (IGG), Chinese Academy of Science.
Double-wrapped coils
In this study, we modified and established double-wrapped coils with the support from professional engineers according to [31]. Double-wrapped coils were composed of three nested sets (X, Y, and Z) of orthogonal square coils corresponding to the north-south, east-west and vertical components of magnetic field. High central field uniformity was achieved by using the four-coil design similar to the Merritt square coils [32]. The four-coil design consisted of four coaxial square coils, parallel and coaxial to each other, and the coil skeleton was purely made up of nonmagnetic materials (Figure 1A). Two sets of coils were powered by the same power supply and each coil contained two matched sets of windings to allow operation in active or sham mode. In active mode, currents in paired windings were parallel, leading to summation of generated magnetic fields. In sham mode, currents ran antiparallel, yielding no measurable external field, but with similar ohmic heating and magneto-mechanical effects as in active mode [31]. The two sets of coils can be randomly selected, one as the HMF group and the other for the GMF control. The double-wrapped coils modified in this study enabled authentic double-blind experiments. Because the advantage of double-wrapped coils, it has been already used in many studies [33–37]. In this study, we used the double-wrapped coils to test the effects of HMF exposure on the neurogenic niche and adult neurogenesis in hippocampus, as well as the cognitive function in mice under double-blind experimental conditions. The coils were installed at Beijing National Observatory of Space Environment that is far from residential districts to reduce anthropogenic environmental interference, such as the background extremely low-frequency electric field (ELF-EF). The strength of static magnetic field and the power frequency electromagnetic field within the HMF and GMF cages were measured by Mag-13MS sensors combined with the Spectramag-6 (Bartington Instruments, Witney, United Kingdom) during the experiments. The HMF had a high uniformity with a strength of 31.1 ± 2.0 nT (mean ± SEM). For the GMF control, the magnetic field strength was 55,547.8 ± 1.5 nT (Figure 1B). The ELF-EF intensity at 50 Hz within the HMF and GMF cages was mutually comparable, reduced by 1–2 orders of magnitude compared to our previous study (Table 1).
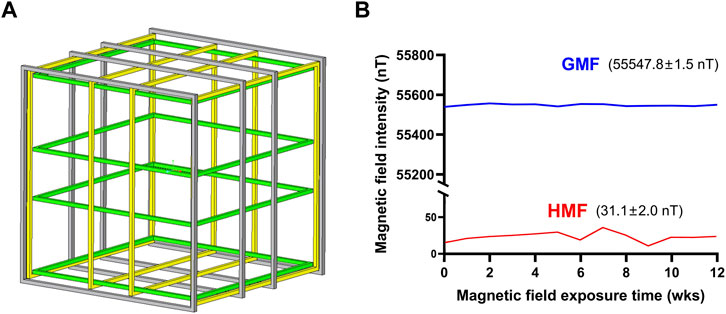
FIGURE 1. (A) Schematic diagram of double-wrapped coil system. (B) The parameters of magnetic fields in experimental duration. HMF means hypomagnetic field. GMF means geomagnetic field; wks means weeks.

TABLE 1. The square root of power spectral density (PSD) at 50 Hz within the GMF and HMF cages in this study and our previous study. DW, double-wrapped coils in this study; pre, helmholtz coils used in our previous study [18].
BrdU administration and brain slices
Mice were injected at a dose of 200 mg/kg BrdU at 2 h or 4 weeks before perfusion (proliferation or differentiation sampling). Mice were perfused and euthanized after anesthesia with isoflurane. The brains were dissected and properly fixed in 4% PFA and then dehydrated in 30% sucrose buffer. The brains were consecutively sectioned on the coronal plane with a thickness of 40 µm using a freezing microtome. Serials sections were stored in 96-well plates filled with the cryoprotectant solution for next immunofluorescence steps.
Immunofluorescence staining
Tissue sections (40-μm thick) were preblocked with blocking buffer PBS++ (PBS containing 3% donkey serum and 0.3% Triton X-100) for 1 h at 37°C, followed by incubation with primary antibodies diluted in blocking buffer for 24 h at 4°C. After washing 3 times, sections were incubated with the secondary antibody for 1 h at 37°C. To detect BrdU incorporation, BrdU-labeled brain sections were pretreated with 2 M HCl for 20 min at 37°C and neutralized with 0.1 M borate buffer (pH 8.5) for 30 min, and subjected to immunofluorescence analyses. All sections were counterstained with DAPI (Sigma-Aldrich, #D9542). The following primary antibodies were used for immunostaining: Rat anti-BrdU (1:1000, Abcam, #ab6326), Goat anti-GFAP (1:1000, Abcam, #ab53544), Mouse anti-SOX2 (1:1000, Abcam, #ab79351), Mouse anti-NeuN (1:500, Millipore, #MAB377), Rabbit anti-S100β (1:1000, Abcam, #ab52642). The following fluorescent secondary antibodies were used: Donkey anti-rat 488 (1:500, Invitrogen, #A21208), Donkey anti-rabbit 488 (1:500, Abcam, #ab150073), Donkey anti-goat 568 (1:500, Invitrogen, #A11057), Donkey anti-rabbit 568 (1:500, Abcam, #ab175470), Donkey anti-rabbit 647 (1:500, Abcam, #ab150075), Donkey anti-mouse 647 (1:500, Invitrogen, #A31571). After staining, sections were mounted, coverslipped, and then maintained at 4°C in the dark until imaging. Images were acquired on an FV1000 Olympus confocal system with a multitrack configuration.
Quantification and fate mapping of BrdU+ cells in the dentate gyrus
For quantification of BrdU+ cells, Iba1+ cells, GFAP+ cells, and the phenotype of BrdU+ cells (double labeling with either GFAP, SOX2, S100β, or NeuN), 1 in 12 serial sections of the whole hippocampus (from Bregma −1.5 to −3.5 mm) were used. All indicated cells in the brain sections (5–6 sections per mouse) were counted inside the section center between 5-μm guard zones of the section surfaces under an FV1000 Olympus microscope. The data were presented as a number of cells in a cubic millimeter of the dentate gyrus. For measurement of relative DG volume, 1 in 12 serial sections of the whole hippocampus (from Bregma −1.5 to −3.5 mm) were stained with DAPI to visualize the nuclei. The same amount of sections (5 sections per mouse) with relative same brain anatomy was used to measure granule cell layer volume under an FV1000 Olympus microscope, and the data were presented as relative DG volume among the selected sections. Four individuals in each group for the immunofluorescence experiments.
Open-field test
The open field test box and video recording equipment (VCR) were prepared and installed beforehand. The marked mice were placed in the central area of the open field maze, and allowed to move freely to explore. The video device was switched on after 5 min to record the exploration activity for 10 min. After each test, the inner environment of the test box was thoroughly cleaned and disinfected with 75% alcohol solution to remove the residual odor of the last mouse. Then the next open-field experiment was carried out after the alcohol was volatilized and the operation was repeated. Finally, Panlab smart 3.0 software was used to analyze the exploration activity of each mouse recorded by VCR.
Object location task and novel object recognition task tests
Object location task (OLT) and novel object recognition task (NORT) tests are commonly surveyed as indicators of the cognitive functions of specific brain regions. OLT primarily evaluates hippocampal-related spatial learning and NORT evaluates non-spatial learning of object identity, which relies on multiple brain regions [38,39]. The experimental procedures were described in detail elsewhere [40]. The two tests exploited the inherent preference of mice for the novelty to reveal memory for previously encountered objects. The percentage of the time spent exploring new locations or new objects in the total time spent exploring new and old locations or objects was counted as preference index (PI). If PI > 0.5, it indicates that mice have exploration preference for novel locations or objects.
Statistical analysis
All data in this study were collected in a double-blind manner. The number of various types of cells were counted by Imaris Viewer and Image J, and different groups were randomly numbered for statistical analyses to ensure the objectivity and reliability of the experimental results. Unpaired t-test was used to analyze the significance of the difference between groups using GraphPad Prism 8.3.0 software. Differences were considered statistically significant at p < 0.05.
Results
Long-term hypomagnetic field exposure induced adult hippocampal neurogenesis inhibition
To assess whether the exposure to HMF affects the proliferation of aNSCs, we examined the proliferation of aNSCs in the DG of adult mice at 0, 4, 8, and 12 weeks after the GMF- or HMF-exposure. Compared with the GMF control, a significant reduction in the number of BrdU+ proliferating cells was observed in HMF-exposed mice after 8-week (p = 0.0201) and 12-week (p = 0.0001) HMF exposure (Figure 2). We further assessed the phenotypes of the BrdU+ proliferating cells in the DG of mice. Compared with the GMF control, BrdU+GFAP+SOX2+ type 1 aNSCs were significantly reduced in HMF-exposed mice after 8-week HMF exposure (p = 0.0314), while no significant change in the number of BrdU+GFAP−SOX2+ type 2 aNSCs after 8-week HMF exposure (Figure 3). The results suggested that 8-week HMF exposure primarily affected proliferating type 1 aNSCs.
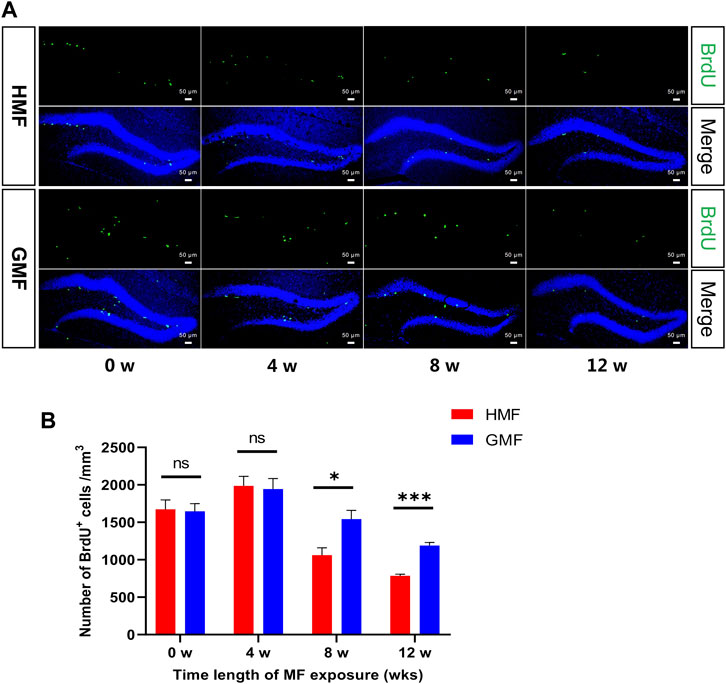
FIGURE 2. The 8-, and 12-week hypomagnetic field (HMF) exposure significantly decreased the proliferation of aNSCs in DG of hippocampus. (A) Representative images of BrdU+ cells in the DG at 0-, 4-, 8-, and 12-week of GMF- or HMF-exposure. (B) Quantification of numbers of BrdU+ cells in GMF- and HMF-exposed mice (P8w = 0.0201, P12w = 0.0001). There were 4 mice in each group at each time point. Data are expressed as mean ± SEM. *p < 0.05; ***p < 0.001. GMF means geomagnetic field; wks means weeks.
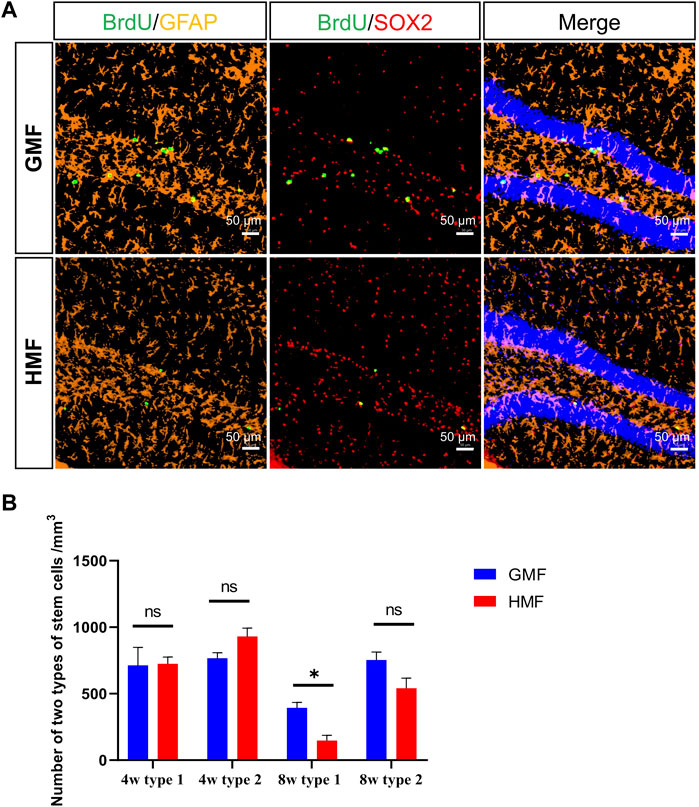
FIGURE 3. The 8-week hypomagnetic field (HMF) exposure significantly decreased the proliferation of type 1 aNSCs in DG of hippocampus. (A) Representative images of BrdU+GFAP+SOX2+ cells (type 1 aNSCs) and BrdU+GFAP−SOX2+ cells (type 2 aNSCs) in the DG at 8-week GMF- or HMF-exposure. (B) Comparison of two types of aNSCs between GMF- and HMF-exposed mice (P8w = 0.0314). There were 4 mice in each group at each time point. Data are expressed as mean ± SEM. ns means no significance. *p < 0.05. GMF means geomagnetic field. 4w means 4-week magnetic fields exposure; 8w means 8-week magnetic fields exposure.
We further analyzed the numbers of the newborn neurons and astrocytes after the differentiation from aNSCs. BrdU-colabeled with NeuN and S100β were used to specifically identify the newborn neurons and astrocytes, respectively. Compared to the GMF-exposed control, we found the numbers of newborn neurons (p = 0.022) and astrocytes (p = 0.0016) significantly decreased after 12-week HMF exposure (Figure 4). The results indicated that long-term HMF exposure significantly decreased the neuronal/astrocytic differentiation of aNSCs.
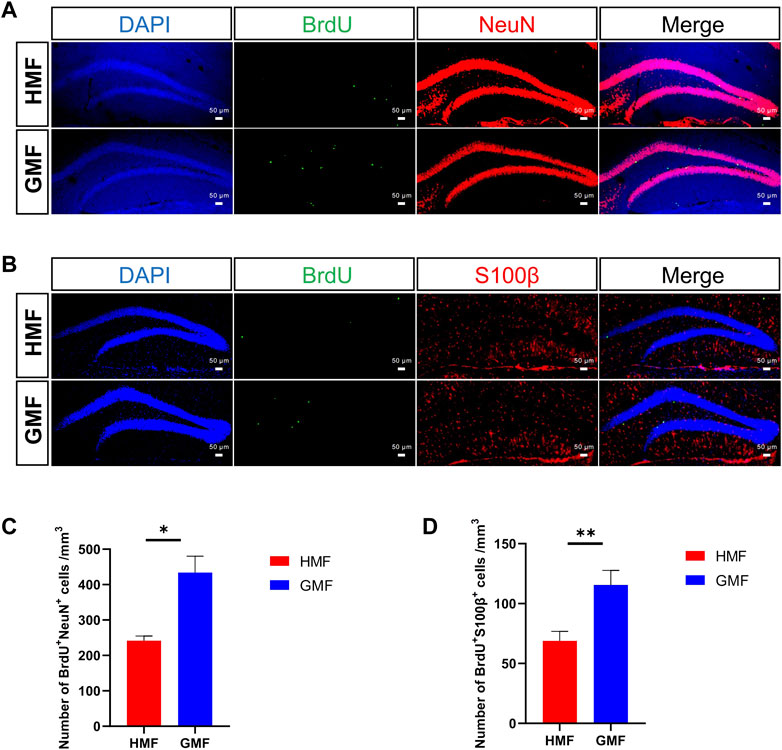
FIGURE 4. Long-term hypomagnetic field (HMF) exposure decreased the neuronal and astrocytic differentiation of aNSCs in DG of hippocampus. (A) Representative images of BrdU+ NeuN+ cells (newborn neurons) in the DG after 12-week of GMF- or HMF-exposure. (B) Representative images of BrdU+ S100β+ cells (newborn astrocytes) in the DG after 12-week of GMF- or HMF-exposure. (C) Quantification of numbers of BrdU+ NeuN+ cells in GMF- and HMF-exposed mice (p = 0.022). (D) Quantification of numbers of BrdU+ S100β+ cells in GMF- and HMF-exposed mice (p = 0.0016). There were 4 mice in each group. Data are expressed as mean ± SEM. *p < 0.05; **p < 0.01. GMF means geomagnetic field.
Long-term hypomagnetic field exposure induced abnormal neurogenic niche of adult neural stem cells
Maintenance of homeostasis of the neurogenic niche in the DG is quite important to normal AHN [41]. We then examined the effect of the exposure to HMF on the morphological activation of microglia and the number of astrocytes in DG area. Compared to GMF-exposed mice, some microglia morphologies were altered (cytoplasmic hypertrophy and fewer and thicker branches) in the DG of HMF-exposed mice after 8-week HMF exposure, suggesting that microglia were activated by the HMF exposure (Figure 5); In addition, the number of astrocytes in the DG in HMF-exposed mice was significantly increased (p = 0.0008), compared to the GMF-exposed mice (Figure 6). These results indicated that 8-week HMF exposure altered the homeostasis of the neurogenic niche of aNSCs.
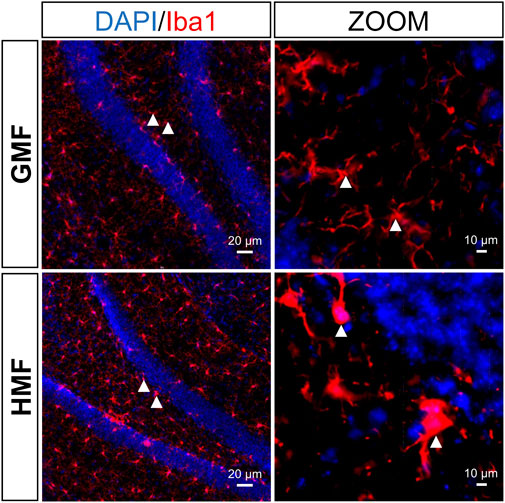
FIGURE 5. The hypomagnetic field (HMF) led to partial activation of microglia in the DG of hippocampus. Representative images of microglia in the hippocampal neurogenic niche under different magnetic field treatments. Microglia (Iba1-labeled, red), hippocampal cells (DAPI-labeled, blue).
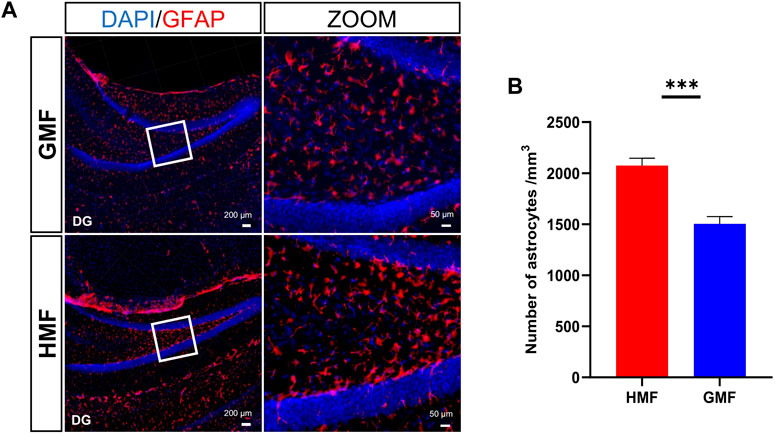
FIGURE 6. The hypomagnetic field (HMF) increased the number of astrocytes in the DG of hippocampus. (A) The representative images of astrocytes in the hippocampal neurogenic niche under different magnetic field treatments, astrocytes (GFAP-labeled, red), hippocampal cells (DAPI-labeled, blue). (B) Quantitative statistical analysis of the number of astrocytes in the DG area of hippocampus under different magnetic field treatments (p = 0.0008). There were 4 mice in each group. Data are expressed as mean ± SEM. ***p < 0.001.
Long-term hypomagnetic field exposure induced anxiety-like behavior of mice
To assess whether the long-term HMF exposure affects the locomotion and anxiety-like behaviors in mice, we mainly analyzed the movement distances of mice in the open field and the exploration time in the central area. The result showed no significant difference in the movement distances between the GMF group and the HMF group at 0-week and 8-week, indicating that the 8-week HMF exposure had no significant effect on the movement ability of mice. However, the exploration time in the central area significantly decreased in HMF-exposed mice after 8-week HMF exposure (p = 0.0002), suggesting that the HMF group mice exhibited significant anxiety-like behavior. While at 0 week, there was no significant difference between HMF- and GMF- groups (Figure 7).
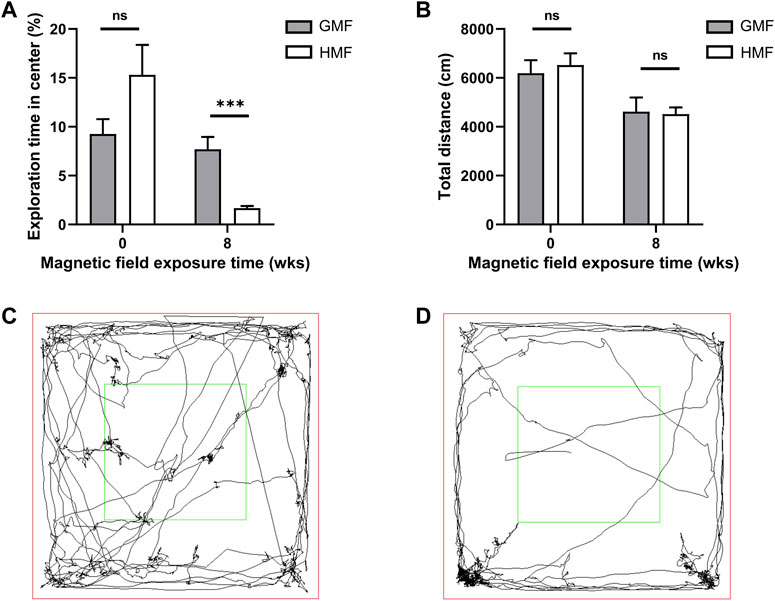
FIGURE 7. Exposure to the hypomagnetic field (HMF) induced anxiety-like behaviors in mice. (A) Comparison of the proportions of the exploration time in the central area of the open field maze to the total exploration time in mice exposed to different magnetic fields (P8w = 0.0002). (B) Comparison of total travel distances of mice exposed to different magnetic fields in the open field maze. (C) The representative image of the trajectory of mice in the open field maze after 8-week GMF exposure. (D) The representative image of the trajectory of mice in the open field maze after 8-week HMF exposure. There were 10 mice in each group at each time point. ns, no significance. Data are expressed as mean ± SEM. ***p < 0.001. GMF means geomagnetic field; wks means weeks.
Long-term hypomagnetic field exposure impaired the cognitive function of mice
We analyzed the percentage of the time spent exploring new locations or new objects in the total time in NOL and NORT tests. As shown in Figure 8, GMF-exposed mice spent relatively more time exploring the new object or new location, while the time exploring the new object or new location of HMF-exposed mice significantly reduced (p < 0.0001). The results showed that HMF-exposed mice have less exploration preference for novel objects or locations than ones in the GMF group. These data suggested the HMF exposure impaired the spatial memory of mice.
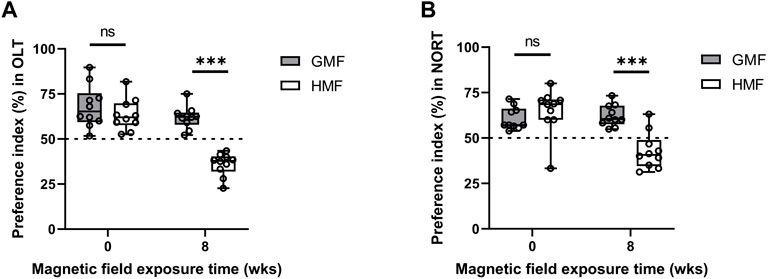
FIGURE 8. Exposure to hypomagnetic field (HMF) impaired hippocampal-dependent cognition. (A) Comparison of preference index in novel location recognition test between the GMF-exposed group and HMF-exposed group (P8w < 0.0001). (B) Comparison of preference index in novel object recognition test between the GMF-exposed group and HMF-exposed group (P8w < 0.0001). Boxplots show the median value (horizontal line), box limits indicate the 25th and 75th percentiles (box size covers the central 50% of the data), whiskers show extreme values of data distribution (maximal and minimal values within total range). There were 10 mice in each group at each time point. ns means no significance. ***p < 0.001. GMF means geomagnetic field; wks means weeks.
Discussion
In this study, the findings support that 8-week HMF exposure impairs AHN and hippocampal-dependent cognitive function of mice (Figures 2–4, 8), which are consistent with our previous results [18]. These results strongly confirm that long-term HMF can indeed cause AHN impairment and cognitive dysfunction of mice, seemly regardless of small electromagnetic field differences. Importantly, we observed the increased number of astrocytes and partial activation of microglia in the neurogenic niche after 8-week HMF exposure for the first time (Figures 5, 6), indicating that the homeostasis of the neurogenic niche was altered by the 8-week HMF exposure. Neurogenic niche cells are essential to neurogenesis as they provide structural and nutritional support, and modulate fundamental stem cell fate decisions [42–44]. In the health condition, astrocytes and microglia promote developmental synapse formation and pruning [25,30,45,46]. While an increase in the number of astrocytes serves as a delicate indicator of the action of noxious influences upon the CNS and may lead to excessive pruning of dendrites [41]. In general, the proliferation process means the production of many reactive astrocytes and GFAP content is a strong indication of reactive astrocyte remodeling [47,48]. And the reactive astrocytes are commonly detected in many neurodegenerative diseases and in the aging hippocampus [49–51]. Furthermore, microglia are regarded as the macrophages of the CNS, which play critical roles in neural development, homeostasis and neuroinflammation and age-related neurological dysfunction. The activation of microglia often implies the occurrence of neuroinflammation, and the increase of microglial activation and inflammation is closely related to the proliferation of aNSCs and the decline of neuronal maturity [52,53], and ultimately result in cognitive decline [54–56]. Therefore, the increased number of astrocytes and the activation of microglia demonstrate that the long-term HMF disrupts the homeostasis of the neurogenic niche, contributing to the neurogenesis impairments in hippocampus. Further evidence about some inflammatory cytokines and growth factors related to the niche cells in the DG, such as tumor necrosis factor (TNF), astrocyte-derived IL-33 and IL-1, will be investigated in the future.
In addition, we found that the HMF exposure significantly induced anxiety-like behaviors of mice as evidenced in the open field test. Previous studies revealed short-time HMF exposure (72 h) induced a significant increase in anxiety-like behaviors of mice and the oxidative stress may be one of the factors triggering anxious behavior [14,57,58]. Intriguingly, we previously reported that HMF exposure increased ROS content in the whole hippocampus by modulating the key genes expression of the oxidative stress and antioxidant defense, except the subgranular zone of dentate gyrus [19], which suggests that oxidative stress in the hippocampus may contribute to the anxiety-like behavior found in this study. Besides, other studies suggested that decreased neurogenesis or ablation of neurogenesis usually led to an increased anxiety-like behavior [59,60]. And the recovery of anxiety also requires the inevitable involvement of neurogenesis [61–63]. For example, Mohammad et al. showed that enhancing adult neurogenesis could alleviate the anxiety behaviors [64]. Thus, oxidative stress and reduced neurogenesis induced by HMF exposure may lead to the anxiety-like behavior in mice. The effect of amygdala on anxiety cannot be ruled out, which has also been shown to be closely related to anxiety [65–70].
In this study, the accumulation period of HMF effect on the proliferation of aNSCs was 8 weeks of HMF exposure (Figure 2), different with 4 weeks in our previous study [18]. Furthermore, HMF exposure caused a significant decrease in the number of type 1 aNSCs in this study, whereas the numbers of both type 1 and type 2 aNSCs were decreased in our previous study [18]. We could not determine whether these differences were caused by differences in the stability of the static magnetic field and background electromagnetic noise of the two different coil systems.
In summary, this study delineates that long-term HMF exposure causes cognitive dysfunction and the anxiety-like behavior in the CNS, which are closely associated with the neurogenic niche abnormalities to reduce adult neurogenesis in hippocampus. These findings demonstrate that geomagnetic field is an important environmental factor for normal adult neurogenesis in mammals on Earth. Furthermore, this study helps us to assess the health risks of the HMF environment in deep space exploration and to develop effective protective strategies or potential treatments.
Data availability statement
The raw data supporting the conclusion of this article will be made available by the authors, without undue reservation.
Ethics statement
The animal study was reviewed and approved by Institutional Animal Care and Use Committee at the Institute of Geology and Geophysics (IGG), Chinese Academy of Science.
Author contributions
LT conceived and supervised the study; YL and YF performed experiments; AZ performed the magnetic field operation and environmental data measurements; LT and YL performed data analysis and wrote the manuscript with contributions from all coauthors. All authors read and approved the final manuscript.
Funding
This work was supported by National Natural Science Foundation of China (Nos 42074073, 41621004) and the Strategic Priority Research Program of the Chinese Academy of Sciences (No. XDA17010501).
Acknowledgments
The authors would like to thank Bingfang Zhang for her guidance on the immunofluorescence technique, thank Yu’an Du for help with measuring environmental parameters, and thank Jianxun Shen for discussing the manuscript.
Conflict of interest
The authors declare that the research was conducted in the absence of any commercial or financial relationships that could be construed as a potential conflict of interest.
Publisher’s note
All claims expressed in this article are solely those of the authors and do not necessarily represent those of their affiliated organizations, or those of the publisher, the editors and the reviewers. Any product that may be evaluated in this article, or claim that may be made by its manufacturer, is not guaranteed or endorsed by the publisher.
Supplementary material
The Supplementary Material for this article can be found online at: https://www.frontiersin.org/articles/10.3389/fphy.2022.1075198/full#supplementary-material
References
1. Parihar VK, Allen B, Tran KK, Macaraeg TG, Chu EM, Kwok SF, et al. What happens to your brain on the way to mars. Sci Adv (2015) 1(4):e1400256. doi:10.1126/sciadv.1400256
2. Parihar VK, Allen BD, Caressi C, Kwok S, Chu E, Tran KK, et al. Cosmic radiation exposure and persistent cognitive dysfunction. Sci Rep (2016) 6:34774. doi:10.1038/srep34774
3. Parihar VK, Maroso M, Syage A, Allen BD, Angulo MC, Soltesz I, et al. Persistent nature of alterations in cognition and neuronal circuit excitability after exposure to simulated cosmic radiation in mice. Exp Neurol (2018) 305:44–55. doi:10.1016/j.expneurol.2018.03.009
4. Pradhan S, Sehgal VK, Bandyopadhyay K, Panigrahi P, Parihar C, Jat S. Radiation interception, extinction coefficient and use efficiency of wheat crop at various irrigation and nitrogen levels in a semi-arid location. Indian J Plant Physiol (2018) 23(3):416–25. doi:10.1007/s40502-018-0400-x
5. Acharya MM, Baulch JE, Klein PM, Baddour AAD, Apodaca LA, Kramar EA, et al. New concerns for neurocognitive function during deep space exposures to chronic, low dose-rate, neutron radiation. eNeuro (2019) 6. ENEURO.0094, 19.2019(4). Epub 2019/08/07. doi:10.1523/ENEURO.0094-19.2019
6. Clement GR, Boyle RD, George KA, Nelson GA, Reschke MF, Williams TJ, et al. Challenges to the central nervous system during human spaceflight missions to mars. J Neurophysiol (2020) 123(5):2037–63. doi:10.1152/jn.00476.2019
7. Michalettou TD, Michalopoulos I, Costes SV, Hellweg CE, Hada M, Georgakilas AG. A meta-analysis of the effects of high-let ionizing radiations in human gene expression. Life (2021) 11(2):115. doi:10.3390/life11020115
8. Choleris E, Del Seppia C, Thomas AW, Luschi P, Ghione G, Moran GR, et al. Shielding, but not zeroing of the ambient magnetic field reduces stress-induced analgesia in mice. Proc R Soc Lond B (2002) 269(1487):193–201. doi:10.1098/rspb.2001.1866
9. Wang XB, Xu ML, Li B, Li DF, Jiang JC. Long-term memory was impaired in one-trial passive avoidance task of day-old chicks hatching from hypomagnetic field space. Chin Sci. Bull. (2003) 48(22):2454–7.
10. Zhang B, Lu H, Xi W, Zhou X, Xu S, Zhang K, et al. Exposure to hypomagnetic field space for multiple generations causes amnesia in Drosophila melanogaster. Neurosci Lett (2004) 371(2-3):190–5. doi:10.1016/j.neulet.2004.08.072
11. Binhi VN, Sarimov RM. Zero magnetic field effect observed in human cognitive processes. Electromagn Biol Med (2009) 28(3):310–5. Epub 2009/12/17. doi:10.3109/15368370903167246
12. Mo WC, Liu Y, Cooper HM, He RQ. Altered development of Xenopus embryos in a hypogeomagnetic field. Bioelectromagnetics (2012) 33(3):238–46. doi:10.1002/bem.20699
13. Binhi VN, Prato FS. Biological effects of the hypomagnetic field: An analytical review of experiments and theories. Plos One (2017) 12(6):e0179340. doi:10.1371/journal.pone.0179340
14. Ding HM, Wang X, Mo WC, Qin LL, Wong S, Fu JP, et al. Hypomagnetic fields cause anxiety in adult male mice. Bioelectromagnetics (2019) 40(1):27–32. doi:10.1002/bem.22155
15. Hu PD, Mo WC, Fu JP, Liu Y, He RQ. Long-term hypogeomagnetic field exposure reduces muscular mitochondrial function and exercise capacity in adult male mice. Prog Biochem Biophys (2020) 47(5):426–38. doi:10.16476/j.pibb.2020.0047
16. Oomen CA, Bekinschtein P, Kent BA, Saksida LM, Bussey TJJWIRCS. Adult hippocampal neurogenesis and its role in cognition. Wires Cogn Sci (2014) 5(5):573–87. doi:10.1002/wcs.1304
17. Semenov MV. Adult hippocampal neurogenesis is a developmental process involved in cognitive development. Front Neurosci (2019) 13:159. doi:10.3389/fnins.2019.00159
18. Zhang B, Wang L, Zhan A, Wang M, Tian L, Guo W, et al. Long-term exposure to a hypomagnetic field attenuates adult hippocampal neurogenesis and cognition. Nat Commun (2021) 12(1):1174–17. doi:10.1038/s41467-021-21468-x
19. Tian LX, Luo YK, Zhan AS, Ren J, Qin HF, Pan YX. Hypomagnetic field induces the production of reactive oxygen species and cognitive deficits in mice Hippocampus. Int J Mol Sci (2022) 23(7):3622. doi:10.3390/ijms23073622
20. Platel JC, Dave KA, Gordon V, Lacar B, Rubio ME, Bordey A. Nmda receptors activated by subventricular zone astrocytic glutamate are critical for neuroblast survival prior to entering a synaptic network. Neuron (2010) 65(6):859–72. doi:10.1016/j.neuron.2010.03.009
21. Cao X, Li LP, Qin XH, Li SJ, Zhang M, Wang Q, et al. Astrocytic adenosine 5 '-Triphosphate release regulates the proliferation of neural stem cells in the adult Hippocampus. Stem Cells (2013) 31(8):1633–43. doi:10.1002/stem.1408
22. Song SH, Stevens CF, Gage FH. Astroglia induce neurogenesis from adult neural stem cells. Nature (2002) 417(6884):39–44. doi:10.1038/417039a
23. Lie DC, Colamarino SA, Song HJ, Desire L, Mira H, Consiglio A, et al. Wnt signalling regulates adult hippocampal neurogenesis. Nature (2005) 437(7063):1370–5. doi:10.1038/nature04108
24. Sultan S, Li L, Moss J, Petrelli F, Cassé F, Gebara E, et al. Synaptic integration of adult-born hippocampal neurons is locally controlled by astrocytes. Neuron (2015) 88(5):957–72. doi:10.1016/j.neuron.2015.10.037
25. Sierra A, Encinas JM, Deudero JJ, Chancey JH, Enikolopov G, Overstreet-Wadiche LS, et al. Microglia shape adult hippocampal neurogenesis through apoptosis-coupled phagocytosis. Cell Stem Cell (2010) 7(4):483–95. doi:10.1016/j.stem.2010.08.014
26. Artegiani B, Lyubimova A, Muraro M, van Es JH, van Oudenaarden A, Clevers H. A single-cell rna sequencing study reveals cellular and molecular dynamics of the hippocampal neurogenic niche. Cell Rep (2017) 21(11):3271–84. doi:10.1016/j.celrep.2017.11.050
27. Mallat M, Marin-Teva JL, Cheret C. Phagocytosis in the developing cns: More than clearing the corpses. Curr Opin Neurobiol (2005) 15(1):101–7. doi:10.1016/j.conb.2005.01.006
28. Ajami B, Bennett JL, Krieger C, Tetzlaff W, Rossi FMV. Local self-renewal can sustain cns microglia maintenance and function throughout adult life. Nat Neurosci (2007) 10(12):1538–43. doi:10.1038/nn2014
30. Diaz-Aparicio I, Paris I, Sierra-Torre V, Plaza-Zabala A, Rodriguez-Iglesias N, Marquez-Ropero M, et al. Microglia actively remodel adult hippocampal neurogenesis through the phagocytosis secretome. J Neurosci (2020) 40(7):1453–82. doi:10.1523/Jneurosci.0993-19.2019
31. Kirschvink JL. Uniform magnetic-fields and double-wrapped coil systems - improved techniques for the design of bioelectromagnetic experiments. Bioelectromagnetics (1992) 13(5):401–11. doi:10.1002/bem.2250130507
32. Merritt R, Purcell C, Stroink G. Uniform magnetic field produced by three, four, and five square coils. Rev Scientific Instr (1983) 54(7):879–82. doi:10.1063/1.1137480
33. Zapka M, Heyers D, Hein CM, Engels S, Schneider N-L, Hans J, et al. Visual but not trigeminal mediation of magnetic compass information in a migratory bird. Nature (2009) 461(7268):1274–7. doi:10.1038/nature08528
34. Hein CM, Engels S, Kishkinev D, Mouritsen H. Robins have a magnetic compass in both eyes. Nature (2011) 471(7340):E11–2. discussion E2-3. doi:10.1038/nature09875
35. Engels S, Schneider NL, Lefeldt N, Hein CM, Zapka M, Michalik A, et al. Anthropogenic electromagnetic noise disrupts magnetic compass orientation in a migratory bird. Nature (2014) 509(7500):353–6. doi:10.1038/nature13290
36. Mouritsen H. Long-distance navigation and magnetoreception in migratory animals. Nature (2018) 558(7708):50–9. doi:10.1038/s41586-018-0176-1
37. Wang CX, Hilburn IA, Wu D-A, Mizuhara Y, Cousté CP, Abrahams JN, et al. Transduction of the geomagnetic field as evidenced from alpha-band Activity in the human brain. eNeuro (2019) 6. ENEURO.0483, 18.2019(2). doi:10.1523/ENEURO.0483-18.2019
38. Broadbent NJ, Gaskin S, Squire LR, Clark RE. Object recognition memory and the rodent Hippocampus. Learn Mem (2010) 17(1):5–11. doi:10.1101/lm.1650110
39. Barker GRI, Warburton EC. When is the Hippocampus involved in recognition memory? J Neurosci (2011) 31(29):10721–31. doi:10.1523/Jneurosci.6413-10.2011
40. Denninger JK, Smith BM, Kirby ED. Novel object recognition and object location behavioral testing in mice on a budget. J Vis Exp (2018) 141:e58593. doi:10.3791/58593
41. Terreros-Roncal J, Moreno-Jiménez E, Flor-García M, Rodríguez-Moreno C, Trinchero MF, Cafini F, et al. Impact of neurodegenerative diseases on human adult hippocampal neurogenesis. Science (2021) 374(6571):1106–13. doi:10.1126/science.abl5163
42. Alonso M. Neurogenic niche. In: MD Binder, N Hirokawa, and U Windhorst, editors. Encyclopedia of neuroscience. Berlin, Heidelberg: Springer Berlin Heidelberg (2009). p. 2682–5.
43. Vainchtein ID, Molofsky AV. Astrocytes and microglia: In sickness and in health. Trends Neurosci (2020) 43(3):144–54. doi:10.1016/j.tins.2020.01.003
44. Vicidomini C, Guo NN, Sahay A. Communication, cross talk, and signal integration in the adult hippocampal neurogenic niche. Neuron (2020) 105(2):220–35. doi:10.1016/j.neuron.2019.11.029
45. Weber B, Barros LF. The astrocyte: Powerhouse and recycling center. Cold Spring Harb Perspect Biol (2015) 7(12):a020396. doi:10.1101/cshperspect.a020396
46. Allen NJ, Eroglu C. Cell biology of astrocyte-synapse interactions. Neuron (2017) 96(3):697–708. doi:10.1016/j.neuron.2017.09.056
47. Liddelow SA, Guttenplan KA, Larke LEC, Bennett FC, Bohlen CJ, Schirmer L, et al. Neurotoxic reactive astrocytes are induced by activated microglia. Nature (2017) 541(7638):481–7. doi:10.1038/nature21029
48. Escartin C, Galea E, Lakatos A, O'Callaghan JP, Petzold GC, Serrano-Pozo A, et al. Reactive astrocyte nomenclature, definitions, and future directions. Nat Neurosci (2021) 24(3):312–25. doi:10.1038/s41593-020-00783-4
49. Li KY, Li JT, Zheng JL, Qin S. Reactive astrocytes in neurodegenerative diseases. Aging Dis (2019) 10(3):664–75. doi:10.14336/Ad.2018.0720
50. Chun H, Im H, Kang YJ, Kim Y, Shin JH, Won W, et al. Severe reactive astrocytes precipitate pathological hallmarks of alzheimer's disease via H2o2- production. Nat Neurosci (2020) 23(12):1555–66. doi:10.1038/s41593-020-00735-y
51. Baumert R, Brose N, Eroglu C. Astrocytic traffic jams in the aging brain. Nat Aging (2022) 2(8):681–3. doi:10.1038/s43587-022-00270-4
52. Monje ML, Toda H, Palmer TDJS. Inflammatory blockade restores adult hippocampal neurogenesis. Science (2003) 302(5651):1760–5. doi:10.1126/science.1088417
53. Appel JR, Ye SY, Tang FL, Sun D, Zhang HS, Mei L, et al. Increased microglial activity, impaired adult hippocampal neurogenesis, and depressive-like behavior in microglial VPS35-depleted mice. J Neurosci (2018) 38(26):5949–68. doi:10.1523/Jneurosci.3621-17.2018
54. Ransohoff RM, Perry VH. Microglial physiology: Unique stimuli, specialized responses. Annu Rev Immunol (2009) 27:119–45. doi:10.1146/annurev.immunol.021908.132528
55. Li QY, Barres BA. Microglia and macrophages in brain homeostasis and disease. Nat Rev Immunol (2018) 18(4):225–42. doi:10.1038/nri.2017.125
56. Skok M. Mesenchymal stem cells as a potential therapeutic tool to cure cognitive impairment caused by neuroinflammation. World J Stem Cell (2021) 13(8):1072–83. doi:10.4252/wjsc.v13.i8.1072
57. Bouayed J, Rammal H, Soulimani R. Oxidative stress and anxiety: Relationship and cellular pathways. Oxid Med Cel Longev (2009) 2(2):63–7. doi:10.4161/oxim.2.2.7944
58. Bouayed J, Bohn T. Exogenous antioxidants-double-edged swords in cellular redox state health beneficial effects at physiologic doses versus deleterious effects at high doses. Oxid Med Cel Longev (2010) 3(4):228–37. doi:10.4161/oxim.3.4.12858
59. Pristera A, Saraulli D, Farioli-Vecchioli S, Strimpakos G, Costanzi M, di Certo MG, et al. Impact of N-tau on adult hippocampal neurogenesis, anxiety, and memory. Neurobiol Aging (2013) 34(11):2551–63. doi:10.1016/j.neurobiolaging.2013.05.010
60. Wang J, Ware K, Bedolla A, Allgire E, Turcato FC, Weed M, et al. Disruption of sonic hedgehog signaling accelerates age-related neurogenesis decline and abolishes stroke-induced neurogenesis and leads to increased anxiety behavior in stroke mice. Transl Stroke Res (2022) 13(5):830–44. doi:10.1007/s12975-022-00994-w
61. Mateus-Pinheiro A, Pinto L, Bessa JM, Morais M, Alves ND, Monteiro S, et al. Sustained remission from depressive-like behavior depends on hippocampal neurogenesis. Transl Psychiatry (2013) 3:e210. doi:10.1038/tp.2012.141
62. Anacker C, Hen R. Adult hippocampal neurogenesis and cognitive flexibility - linking memory and mood. Nat Rev Neurosci (2017) 18(6):335–46. doi:10.1038/nrn.2017.45
63. Drew MR, Huckleberry KA. Modulation of aversive memory by adult hippocampal neurogenesis. Neurotherapeutics (2017) 14(3):646–61. doi:10.1007/s13311-017-0528-9
64. Mohammad H, Marchisella F, Ortega-Martinez S, Hollos P, Eerola K, Komulainen E, et al. Jnk1 controls adult hippocampal neurogenesis and imposes cell-autonomous control of anxiety behaviour from the neurogenic niche. Mol Psychiatry (2018) 23(2):362–74. doi:10.1038/mp.2016.203
65. Davis M. The role of the amygdala in fear and anxiety. Annu Rev Neurosci (1992) 15(1):353–75. doi:10.1146/annurev.ne.15.030192.002033
66. McHugh SB, Deacon RMJ, Rawlins JNP, Bannerman DM. Amygdala and ventral Hippocampus contribute differentially to mechanisms of fear and anxiety. Behav Neurosci (2004) 118(1):63–78. doi:10.1037/0735-7044.118.1.63
67. Juranek J, Filipek PA, Berenji GR, Modahl C, Osann K, Spence MA. Association between amygdala volume and anxiety level: Magnetic resonance imaging (mri) study in autistic children. J Child Neurol (2006) 21(12):1051–8. doi:10.1177/7010.2006.00237
68. Ressler KJ. Amygdala activity, fear, and anxiety: Modulation by stress. Biol Psychiatry (2010) 67(12):1117–9. doi:10.1016/j.biopsych.2010.04.027
69. Forster GL, Novick AM, Scholl JL, Watt MJ. The role of the amygdala in anxiety disorders. In: The amygdala: A discrete multitasking manager (2012). p. 61–102.
Keywords: hypomagnetic field, double-wrapped coils, neurogenic niche, adult hippocampal neurogenesis, cognitive function
Citation: Luo Y, Zhan A, Fan Y and Tian L (2022) Effects of hypomagnetic field on adult hippocampal neurogenic niche and neurogenesis in mice. Front. Phys. 10:1075198. doi: 10.3389/fphy.2022.1075198
Received: 20 October 2022; Accepted: 14 November 2022;
Published: 25 November 2022.
Edited by:
Guijun Wan, Nanjing Agricultural University, ChinaReviewed by:
Ying Liu, Beijing University of Chinese Medicine, ChinaFrank Barnes, University of Colorado Boulder, United States
Copyright © 2022 Luo, Zhan, Fan and Tian. This is an open-access article distributed under the terms of the Creative Commons Attribution License (CC BY). The use, distribution or reproduction in other forums is permitted, provided the original author(s) and the copyright owner(s) are credited and that the original publication in this journal is cited, in accordance with accepted academic practice. No use, distribution or reproduction is permitted which does not comply with these terms.
*Correspondence: Lanxiang Tian, dGlhbmx4QG1haWwuaWdnY2FzLmFjLmNu