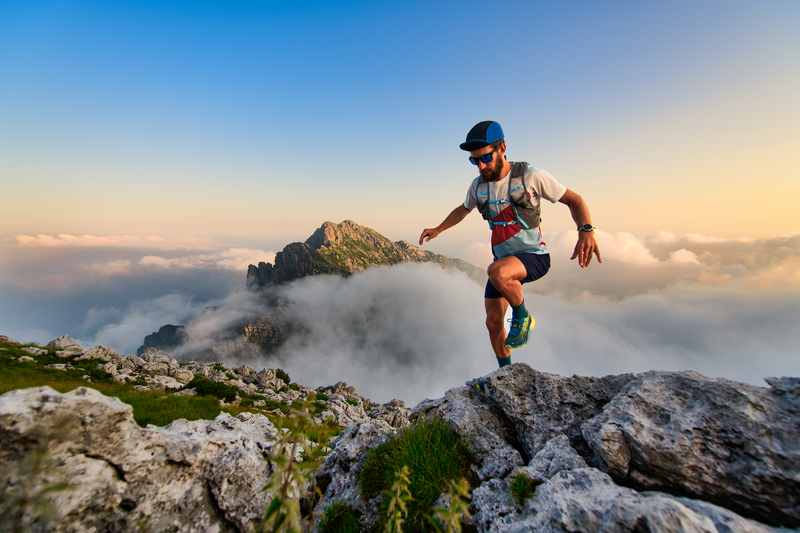
95% of researchers rate our articles as excellent or good
Learn more about the work of our research integrity team to safeguard the quality of each article we publish.
Find out more
ORIGINAL RESEARCH article
Front. Phys. , 13 December 2022
Sec. Nuclear Physics​
Volume 10 - 2022 | https://doi.org/10.3389/fphy.2022.1071269
Structural properties of materials irradiated with gamma-rays, such as mechanical and physical properties, may be modified or reduced depending on the displacement damages. Apart from increasing or decreasing that property, damage may be desirable or not. This study investigates the effects of sterilization by gamma radiation on polycaprolactone (PCL) scaffolds. Using a code-based simulation method, MCNPX provides information on primary knock-on atoms, or PKAs, that cause damage. A program has been developed called GAMMATRACK to access PKA information. These PKA data can be used as input for the SRIM code to analyze gamma damage systematically. The rate of damage caused by gamma radiation is calculated on the PCL target. The theoretical calculation method also has been used to confirm the results of the Monte Carlo method (MCNPX + SRIM code). Due to the low-energy PKAs and the thin target possibility of the displacement cascade can be ignored. It is realized that all displacements are due to single vacancies. The total number of hydrogens, carbons, and oxygen PKAs were obtained. It was found that the number of carbons PKA is more than the others, which causes three-dimensional polymer networks to be created. In the experimental analysis, it is necessary to know the appropriate depth of the sample for damage investigation. GAMMATRACK gives the gamma displacement damage graph along the length of the PCL. It shows a uniform distribution of displacement damage. The damage rate for the PCL target is calculated, and the results between the theoretical calculations and the Monte Carlo method (MCNPX + SRIM code) differ by about 17%.
Tissue engineering is a rapidly growing field in regenerative medicine and develops for the surgical repair of tissues [1, 2]. Production of tissue-engineered scaffolds as medical devices requires sterilization [3]. Gamma irradiation is a common sterilization technique because gamma-induced damage at the molecular level breaks down bacterial DNA and kills them [2, 4]. Each substance is partially affected by radiation. Gamma irradiation can affect the properties of polymers. It can induce specific changes, such as crosslinking and chain scission of polymer chains, that affect the physical, chemical, and surface properties [2, 5, 6]. The chain scission process reduces the molecular weight, and the crosslinking process leads to the formation of large 3D networks and increases the molecular weight of the polymer [1, 5]. Qualitatively, polymer chains are simultaneously linking and scission during polymer irradiation. However, the dominance of one factor over another for a given polymer depends on polymer structure, radiation doses, flux, and sample thickness [4, 5]. Therefore, materials and systems with radiation-resistant components must be developed to prevent radiation-induced degradation, which requires knowledge of degradation methods [5].
Recently, many experimental studies have been performed to investigate the damage of sterilization by gamma-rays, which can lead to structural modification or loss of properties in polycaprolactone (PCL) scaffolds [1–4, 6, 7]. All of these studies have been the product of experimental results [2, 8–15], and the lack of theoretical damage calculations and simulations is felt in this field. In general, aliphatic polymers, such as PCL, are sensitive to radiation, and gamma radiation may affect their physical properties to some extent. In a study by Dominik de Cassan et al. [7] for PCL, the three types of X, beta, and gamma radiation were tested. The results show that irradiation led to polymer chain scission and reduced mean molecular weight
In this study, for the first time, the effect of gamma radiation from a cobalt-60 source on the PCL scaffold has been calculated using Monte Carlo methods and theoretical calculations. The primary knock-on atoms (PKAs) spectrum and their types in the PCL scaffold were investigated. It is possible to know the effect of radiation on the polymer by having the PKA’s percentage of each type of atom. A diagram of the damage along the target also can be obtained. It helps to be aware of the effect of radiation on the PCL before performing experimental analysis and also to estimate the depth of damage. The rate of gamma radiation damage was calculated with the theoretical method and compared to Monte Carlo results.
In recent years, irradiation treatment of polymers has been recognized as an effective way to modify their properties. In fact, among sterilization methods, gamma irradiation is a simple and effective method of removing the biological load. When radiation interacts with matter, it accumulates some or all of its energy, causing atoms to ionize or excite. Low ionizing radiation, known as low-linear energy transfer (LET) (such as X-rays or gamma-rays), interacts with three methods: photoelectric, Compton scattering, and pair production. Because of these processes, energy is absorbed by the target, and electrons are emitted from atoms within the target, depending on its atomic composition. Now, if these Knock-out electrons or secondary radiation, collide with the target atoms and the energy exchanged in the collisions is higher than the threshold energy [which in this case is called “displacement energy (DE)”"], These target atoms can displace adjacent atoms from their original location (called secondary atoms), leading to a cascade of subsequent nuclear collisions. These atoms are called the Primary Knock-out Atoms (PKAs). These interactions lead to structural changes such as point defects, amorphization, chain scission, and crosslinking [3, 5].
Gamma radiation is also used to modify the porous structure of tissue engineering scaffolds. Radiation causes photons to penetrate materials, break polymer chains and create free radicals. These free radicals can also be recombined to form crosslinks between adjacent molecules [4]. Therefore, ionizing radiation causes chemical reactions in polymers, leading to changes in their molecular structure and macroscopic properties. Crosslinking reduces impact strength, and the polymer becomes increasingly brittle with increasing doses. In forming low molecular weight components, gas evolution and unsaturation may occur due to chain breakage [1]. In this way, for calculating gamma radiation damage, the interaction of the secondary radiation with the PCL target can be simulated [16, 17]. This study has studied Cobalt-60 source gamma damage caused by the Compton effect. We used the results of Xcom software for the photon interaction cross-section data in PCL atoms (C6H10O2). These cross-sections are shown in Figure 1 [18]. The Cobalt-60 source has two gammas with 1.17 and 1.33 MeV energy. According to Figure 1, the probability of photoelectric absorptions and pair production at ∼1 MeV energy will be 10−8∼10−4 times lower than the Compton scattering. Therefore, we will ignore these two processes in this study.
FIGURE 1. The total cross-section of the photon interaction with matter (σγ) as a function of the photon’s energy in the Hydrogen, Carbon, and Oxygen, derived from the Xcom code [14].
Radiation damage is a microscopic defect that changes materials’ properties [19]. A parameter usually used to relate displacement damage is the total number of displacements per atom (DPA). As a damage-based exposure unit, DPA introduces the number of primary and secondary atoms displaced from their original sites as a consequence of particle bombardment [20].To calculate DPA caused by the gamma-ray and estimate the effect of sterilization on the PCL scaffold, the radiation chamber of the Cobalt-60 source where medical equipment is sterilized was simulated by MCNPX code. This chamber is a cylinder of 19 cm in length and 14 cm in diameter, which is kept in the Atomic Energy Organization of Iran. PCL scaffold with 0.05 cm thickness was simulated in this chamber and exposed to Cobalt-60 gamma radiation. The source activity was 4,200 Ci. One MCNPX output, Ptrac, saves the whole history of particles through the MCNPX run [21]. GAMMATRACK code was written by MATLAB R2014b [22] software and designed to calculate the damage caused by the gamma-rays. It reads the MCNPX output via Ptrac and provides information on primary knock-on atoms, or PKAs. It calculates energy and stores the type, initial location, and direction cosines of PKAs, and uses it as input for SRIM. SRIM is another popular code used to calculate damage on a target. SRIM input is called trim.dat, which is shown in Table 1 [23, 24]. Finally, the Monte Carlo SRIM code simulates the transport of PKAs in the PCL scaffold. It calculates damage caused by gamma radiation (sterilization effect) in a displacement unit per angstrom-ion [25]. The GAMMATRACK program flowchart is shown in Figure 2 to explain the operations performed within the system (Table 1; Table 2; Table 3).
TABLE 3. Comparison between the DPA production rate estimated by theoretical calculation and Monte Carlo results.
The theoretical calculation of gamma radiation damage has been done for more confidence of the Monte Carlo results. The rate of displacement damage of all atoms in matter is calculated by Eq. 1.
Where N is the atomic lattice density, Φ (Ee) is the electron flux, and σD(Ee) is the cross-section of the electron-nucleus displacement. The displacement rate is measured in DPA, which shows how many times an atom has been displaced from its location in a unit of time. The damage unit is DPA/m3s [17, 26, 27]. The flux of Compton electrons in the matter is obtained from Eq. 2:
Where σc is the cross-section of the Compton scattering, Φy is the gamma-ray flux, and NPCL is the atomic density of PCL [17, 26].
The Klein-Nishina formula, which describes the differential scattering cross-section in gamma-ray interaction with electrons, is defined by relation (3) [17, 26].
In Eq. 3,
Using Eq. 4, the total Compton scattering cross-section, σc, can be calculated:
The energy transferred from the electron to the nucleus is explained by Eq. 6 as a function of the electron scattering angle (θ):
In Eq. 6, Ee is the electron’s energy transferred in Compton scattering [26]. The displacement cross-section is the probability of displacement of atoms by bombarding particles introduced by Eq. 7, where νD (T) is the displacement probability that shows the average number of atoms displaced by a PKA and dσs/dT is the differential scattering cross-section of the electron.
In Eq. 7, the maximum transferable energy from the electron to the nucleus is the upper, and the minimum energy required for the displacement to occur (displacement energy (Ed)) is set to the lower limit of the integral [26, 28]. From Eq. 6, it is possible to determine the maximum transferable energy (TM) for head-on collisions (θ = π), which is used in Eq. 8, α = Z/137, and β is Particle velocity in units of c. The function F(β) is represented by Eq. 9, in which ER is the Rydberg energy [28].
The threshold displacement energy is the energy that a recoil needs to overcome the target’s lattice forces and to move more than one atomic spacing away from its original site. The typical value for fragile materials like polymers, a value as low as 2–5 eV, maybe more accurate [23, 24, 29]. This study considers a 5-eV displacement threshold for the PCL scaffold.
Table 2 shows the minimum, average, and maximum energy of each hydrogen, carbon, and oxygen PKAs by the GAMMATRACK program. The average energy of PKAs is in the range ≈ of 300 eV. Investigations show that oxygen and carbon atoms can knock out other atoms in the PCL structure and create a series of displacements; their energy must be higher in the 1 keV range [23, 29]. At energies higher than 450 eV, the probability of creating a displacement cascade with the hydrogen atoms becomes possible [23, 29]. Nevertheless, as can be seen in Figure 3, the population of these PKAs is negligible. Therefore, the possibility of cascading is ruled out.
FIGURE 3. Energy spectrum of PKAs in PCL samples, for each type of primary knocked-out atoms (hydrogen, carbon, and oxygen) sterilized with cobalt-60 gamma-ray source and 5 eV threshold energy by Monte Carlo method.
Figure 3 shows the PCL scaffold’s energy spectrum of hydrogen, carbon, and oxygen PKAs by the GAMMATRACK program. The highest molecular percentage in materials with organic molecules like polymers is related to the carbon and hydrogen chains. The radiation-induced ionization process first involves the breakage of covalent bonds that require less energy to break and decompose into free radicals [30, 31]. In this case, the carbon-hydrogen bond is opened, and radicals are created, which are unstable particles that can form bonds with other radicals. These ions are the reason for chemical interactions between the molecules at varying concentrations. As a result, many polymer strands are created, forming a three-dimensional polymer network that can enhance mechanical, thermal, and chemical resistance properties [30]. In the sterilization process with gamma radiation, the carbon that requires less energy to be removed is separated from the hydrogen atom by receiving energy and forms a short and robust carbon-carbon bond. Hence the possible damage could be considered an advantage in a sense.
As shown in Figure 3 or Table 2, the percentage of carbon PKAs is higher than that of oxygen and hydrogen. Therefore, forming a three-dimensional polymer network is promising for the PCL target. A 3D network enables the polymer scaffold to adsorb better and trap water molecules. It helps the PCL scaffolds’ performance by keeping the wound moist for extended periods [1, 32, 33]. Figure 4 shows the homogeneity of PKAs’ two-dimensional distribution that is uniformly dispersed along the target for each type of hydrogen, carbon, and oxygen PKAs in the PCL target. However, it should be noted that to better display the atoms along the target, the x-axis display has been exaggerated.
FIGURE 4. PKA’s 2D-distributions in PCL samples, sterilized with cobalt-60 gamma-ray source and 5 eV threshold energy by Monte Carlo method.
As shown in Figure 5, developing a three-dimensional profile of displacement damage can lead to vacancies in the target or replacement collisions. These displacements are due to single vacancies, and replacement collisions have no role in the total displacements.
FIGURE 5. Three-dimensional profile of displacements for gamma-ray cobalt-60 source on an PCL sample.
Figure 6 shows the number of atoms displaced in the PCL target by secondary radiation and compares the theoretical computation with the results obtained from the Monte Carlo method. Since the PCL scaffold is thin, it is expected that the damage will spread uniformly along the target. This subject will be helpful in the next stage of the investigation, experimental analysis, and it assures us of the accuracy of the sampling. As shown in Figure 6, the damage is mainly caused by the displacement of carbon atoms; the related effects explained earlier must be considered. The damage rate value in DPA/year is shown in Table 3. DPA is the most relevant correlation parameter for damage calculation purposes and gives equivalent dose values for different types of irradiations. It is well known that the damage rate plays a vital role in irradiation-induced swelling, creeping, and solute segregation [20]. It will talk about the effects of this damage rate on actual samples by experimental analysis in the subsequent works. The theoretical calculation and Monte Carlo results differ by ∼17%. To determine the difference between the theoretical and Monte Carlo techniques, two reasons are described. In our work, there are two parameters, lattice and surface binding energies, which were chosen as the default values of SRIM. These numbers can be changed for each type of target. Although the LAMMPS simulator, a classical molecular dynamics code focused on materials modeling, was not used in this study, our recent studies show the ability of this software to model polymers well. Calculating the appropriate inputs for the PCL scaffold using the LAMMPS simulator is suggested [34, 35]. Another reason for the difference may be that the SRIM code uses an approximation to consider the structure of polymers. The strength of SRIM is for crystalline structures, and it approximates amorphous-crystalline polymers structure with its default assumptions. With all these interpretations, the obtained results for the damage rate are in good agreement with each other and give us a reliable prediction of property change due to irradiation of the PCL target. The GAMMATRACK code is successfully extended for the gamma radiation, and it is shown that it can be used for damage calculations by the gamma sterilization method. The previous study has been about calculating the damage caused by neutrons by an extended code called AMTRACK. AMTRACK can be used as an input of SRIM codes to analyze neutron primary radiation damage for different purposes systematically [19, 25, 36, 37]. GAMMATRACK was developed to have a complete neutron-gamma damage code.
To calculate the gamma radiation sterilization effect on the PCL scaffold (its modification and improvement or degradation effects) and evaluate PKAs characteristics, the GAMMATRACK code was developed. A technique has been developed to evaluate DPA using the Monte Carlo method (MCNPX and SRIM codes). A theoretical method was also developed to ensure the result of the Monte Carlo method. Using these methods, damage distribution was obtained along the PCL target, showing the displacements’ uniformity. The distribution function of displacement values is a symmetric and uniform Gaussian distribution (R-Square = 0.94539) with mean value = 2.998E-7, and sigma = 2.88594E-8, which indicates the uniformity of the damage along the target, compared to the asymmetric and wider distribution graph corresponds to more variance. Uniformity means sampling will not have complicated in SEM and TEM experimental analyzes, and one can perform better in experimental analyses and minimize trial and error for sampling. The displacement damage was due to vacancies (its rate was calculated in DPA/year) because gamma damage is induced into the matter by electrons (secondary radiation). The electron has low-energy recoils and is more efficient at generating point defects. In contrast, high-energy recoils such as neutron irradiation cause cascade damage [20]. It was found that at this level of gamma radiation energy and the low thickness of the target, the occurrence of a displacement cascade is almost impossible. Nevertheless, according to the damage rate in the sterilization method with gamma radiation, the damaged microstructure leads to essential changes in a material’s physical and mechanical properties. Experimental studies of gamma-ray sterilization show that it can change the molecular structures of the polymer by chain scission, which reduces tensile strength and elongation, or crosslinking, which increases tensile strength but reduces elongation. Crosslinking can generate a 3D crosslink network that can improve the polymer properties while crystallinity and hence density specification may also be altered as chain scission continues [1, 30]. In this study, the number of hydrogen, carbon and oxygen PKAs was obtained. It shows that the percentage of carbon PKAs is more than those of oxygen and hydrogen. Therefore, forming a robust C-C bond with a 3D polymer network is promising. The 3D network increases the PCL scaffolds’ performance for keeping the wound moist for a longer time.
A novel method for investigating the impact of sterilization by gamma radiation is extended. It helps to have foreknowledge about gamma radiation’s definite and possible effects on samples. By considering these effects and optimizing the sample to prevent radiation-induced degradation, we can save time and money and have more efficient experimental studies. In the following article, the structural analyzes of several different PCL samples sterilized by gamma-rays will be investigated, and these results will be compared to experimental results.
The original contributions presented in the study are included in the article/Supplementary Material, further inquiries can be directed to the corresponding author.
The authors declare that they have no known competing financial interests or personal relationships that could have appeared to influence the work reported in this paper. MH, SH, AM, and ES.
The authors declare that the research was conducted in the absence of any commercial or financial relationships that could be construed as a potential conflict of interest.
All claims expressed in this article are solely those of the authors and do not necessarily represent those of their affiliated organizations, or those of the publisher, the editors and the reviewers. Any product that may be evaluated in this article, or claim that may be made by its manufacturer, is not guaranteed or endorsed by the publisher.
1. Rediguieri CF, Sassonia RC, Dua K, Kikuchi IS, de Jesus Andreoli Pinto T. Impact of sterilization methods on electrospun scaffolds for tissue engineering. Eur Polym J (2016) 82:181–95. doi:10.1016/j.eurpolymj.2016.07.016
2. Cottam E, Hukins DW, Lee K, Hewitt C, Jenkins MJ. Effect of sterilisation by gamma irradiation on the ability of polycaprolactone (PCL) to act as a scaffold material. Med Eng Phys (2009) 31(2):221–6. doi:10.1016/j.medengphy.2008.07.005
3. Bosworth L, Gibb A, Downes S. Gamma irradiation of electrospun poly (ε-caprolactone) fibers affects material properties but not cell response. J Polym Sci B Polym Phys (2012) 50(12):870–6. doi:10.1002/polb.23072
4. Augustine R, Saha A, Jayachandran VP, Thomas S, Kalarikkal N. Dose-dependent effects of gamma irradiation on the materials properties and cell proliferation of electrospun polycaprolactone tissue engineering scaffolds. Int J Polymeric Mater Polymeric Biomater (2015) 64(10):526–33. doi:10.1080/00914037.2014.977900
6. Bhaskar P, Bosworth LA, Wong R, O'brien MA, Kriel H, Smit E, et al. Cell response to sterilized electrospun poly (ɛ-caprolactone) scaffolds to aid tendon regeneration in vivo. J Biomed Mater Res (2017) 105(2):389–97. doi:10.1002/jbm.a.35911
7. de Cassan D, Hoheisel AL, Glasmacher B, Menzel H. Impact of sterilization by electron beam, gamma radiation and X-rays on electrospun poly-(ε-caprolactone) fiber mats. J Mater Sci Mater Med (2019) 30(4):42. doi:10.1007/s10856-019-6245-7
8. Walo M, Przybytniak G, Nowicki A, Swieszkowski W. Radiation-induced effects in gamma-irradiated PLLA and PCL at ambient and dry ice temperatures. J Appl Polym Sci (2011) 122(1):375–83. doi:10.1002/app.34079
9. El Salmawi KM. Gamma radiation-induced crosslinked PVA/chitosan blends for wound dressing. J Macromolecular Sci A (2007) 44(5):541–5. doi:10.1080/10601320701235891
10. El-Zaiat S, El-Ghandoor H, Sharaf F. Interferometric investigation of the effects, on the refractive index of PVA, of doping with lead acetate and gamma irradiation. Opt Laser Tech (1997) 29(3):117–9. doi:10.1016/s0030-3992(96)00059-x
11. Ilčin M, Hola O, Bakajova B, Kucerik J. FT-IR study of gamma-radiation induced degradation of polyvinyl alcohol (PVA) and PVA/humic acids blends. J Radioanal Nucl Chem (2010) 283(1):9–13. doi:10.1007/s10967-009-0321-2
12. Jeun J-P, Jeon YK, Nho YC, Kang PH. Effects of gamma irradiation on the thermal and mechanical properties of chitosan/PVA nanofibrous mats. J Ind Eng Chem (2009) 15(3):430–3. doi:10.1016/j.jiec.2009.02.001
13. Dorati R, Colonna C, Serra M, Genta I, Modena T, Pavanetto F, et al. γ-Irradiation of PEGd, lPLA and PEG-PLGA multiblock copolymers: I. Effect of irradiation doses. AAPS PharmSciTech (2008) 9(2):718. doi:10.1208/s12249-008-9103-3
14. Mendieta-Barrañon I, Chanes-Cuevas OA, Serrano-Bello J. Physicochemical and tissue response of PLA nanofiber scaffolds sterilized by different techniques. Odovtos-International J Dental Sci (2019) 169–80.
15. Valente T, Silva DM, Gomes PS, Fernandes MH, Santos JD, Sencadas V. Effect of sterilization methods on electrospun poly (lactic acid)(PLA) fiber alignment for biomedical applications. ACS Appl Mater Inter (2016) 8(5):3241–9. doi:10.1021/acsami.5b10869
16. Toijer E. Assessment of primary damage and copper precipitation in cast iron in repository conditions (2014).
17. Leo WR. Techniques for nuclear and particle physics experiments: A how-to approach. Springer Science & Business Media (2012).
18.National Institute of Standard and Technology (NIST), U.S.D.O.C., Physical measurement laboratory. Available at: https://www.nist.gov/pml/xcom-photon-cross-sections-database.
19. Ardekani SFG, Hadad K. Evaluation of radiation damage in belt-line region of VVER-1000 nuclear reactor pressure vessel. Prog Nucl Energ (2017) 99:96–102. doi:10.1016/j.pnucene.2017.05.003
20. Li M, Mokhov N. Experience with moving from dpa to changes in materials properties. Morschach, Switzerland: ICFA Advanced Beam Dynamics Workshop (2010).
21. Pelowitz DB. Mcnpx 2.7 e extensions. Los Alamos, NM United States: Los Alamos National Lab.LANL (2011).
22. Attaway S. Matlab: A practical introduction to programming and problem solving. Butterworth-Heinemann (2013).
23. Ziegler J, Biersack J, SRIM-2013 software package (2013). Available at: http://www.srim.org.
24. Ziegler JF, Biersack JP. The stopping and range of ions in matter. In: Treatise on heavy-ion science. Springer (1985). p. 93–129.
25. Mohammadi A, Hamidi S, Asadabad MA. The use of the SRIM code for calculation of radiation damage induced by neutrons. Nucl Instr Methods Phys Res Section B: Beam Interactions Mater Atoms (2017) 412:19–27. doi:10.1016/j.nimb.2017.08.036
27. Chen S, Bernard D. Attenuation of atomic displacement damage in the heavy reflector of the PERLE experiment and application to EPR. Nucl Eng Des (2019) 353:110205. doi:10.1016/j.nucengdes.2019.110205
28. Thompson M. Defects and radiation damage in metals. London and New York: Cambridge univ. Press (1969). p. 367.
29. Ziegler JF, Ziegler MD, Biersack JP. SRIM–The stopping and range of ions in matter. Nucl Instr Methods Phys Res Section B: Beam Interactions Mater Atoms (2010) 268(11-12):1818–23. doi:10.1016/j.nimb.2010.02.091
30. Naikwadi AT, Sharma BK, Bhatt KD, Mahanwar PA. Gamma radiation processed polymeric materials for high performance applications: A review. Front Chem (2022) 10:837111. doi:10.3389/fchem.2022.837111
31. Carey FA, Sundberg RJ. Advanced organic chemistry: Part A: Structure and mechanisms. Springer Science & Business Media (2007).
32. Negut I, Dorcioman G, Grumezescu V. Scaffolds for wound healing applications. Polymers (2020) 12(9):2010. doi:10.3390/polym12092010
33. Li J, Zhang T, Pan M, Xue F, Lv F, Ke Q, et al. Nanofiber/hydrogel core–shell scaffolds with three-dimensional multilayer patterned structure for accelerating diabetic wound healing. J Nanobiotechnol (2022) 20(1):28–18. doi:10.1186/s12951-021-01208-5
34. Thompson AP, Aktulga HM, Berger R, Bolintineanu DS, Brown WM, Crozier PS, et al. LAMMPS-a flexible simulation tool for particle-based materials modeling at the atomic, meso, and continuum scales. Comput Phys Commun (2022) 271:108171. doi:10.1016/j.cpc.2021.108171
35. Bone MA, Howlin BJ, Hamerton I, Macquart T. AutoMapper: A python tool for accelerating the polymer bonding workflow in LAMMPS. Comput Mater Sci (2022) 205:111204. doi:10.1016/j.commatsci.2022.111204
36. Amirkhani MA, Asadi Asadabad M, Hassanzadeh M, Mirvakili SM, Mohammadi A. Calculation of dpa rate in graphite box of Tehran Research Reactor (TRR). Nucl Sci Tech (2019) 30(6):92–13. doi:10.1007/s41365-019-0621-3
Keywords: gamma sterilization method, SRIM code, MCNPX, primary knock-on atoms, Xcom code, PCL scaffold, gamma radiation damage
Citation: Hoseini M, Hamidi S, Mohammadi A and Salehi E (2022) A novel method for investigation of the impact of sterilization by gamma radiation on polycaprolactone scaffold. Front. Phys. 10:1071269. doi: 10.3389/fphy.2022.1071269
Received: 16 October 2022; Accepted: 28 November 2022;
Published: 13 December 2022.
Edited by:
David Mascali, Laboratori Nazionali del Sud (INFN), ItalyReviewed by:
Luciano Pandola, Universities and Research, ItalyCopyright © 2022 Hoseini, Hamidi, Mohammadi and Salehi. This is an open-access article distributed under the terms of the Creative Commons Attribution License (CC BY). The use, distribution or reproduction in other forums is permitted, provided the original author(s) and the copyright owner(s) are credited and that the original publication in this journal is cited, in accordance with accepted academic practice. No use, distribution or reproduction is permitted which does not comply with these terms.
*Correspondence: S. Hamidi, cy1oYW1pZGlAYXJha3UuYWMuaXI=
†Present address A. Mohammadi, Materials and Nuclear Fuel Research School, Nuclear Science and Technology Research Institute, Tehran, Iran
Disclaimer: All claims expressed in this article are solely those of the authors and do not necessarily represent those of their affiliated organizations, or those of the publisher, the editors and the reviewers. Any product that may be evaluated in this article or claim that may be made by its manufacturer is not guaranteed or endorsed by the publisher.
Research integrity at Frontiers
Learn more about the work of our research integrity team to safeguard the quality of each article we publish.