- School of Optoelectronic Science and Engineering, State Key Laboratory of Electronic Thin Films and Integrated Devices, University of Electronic Science and Technology of China (UESTC), Chengdu, China
We investigated 2 and 2.9 μm mid-infrared fiber lasers passively Q-switched by MIL-68(Al) and MIL-68(Fe), which were fabricated via the hydrothermal method. The modulation depth of MIL-68(Al) was found to be 9.12% at 1.99 μm. And the modulation depths of MIL-68(Fe) were found to be 18.89% and 15.79% at 1.99 μm and 2.87 μm, respectively. We report Q-switching pulse generation in both Tm3+-doped and Ho3+/Pr3+ co-doped fiber lasers by using the as-prepared MIL-68 (M, M = Al3+, Fe3+) as SAs. The center wavelengths were at 1.99 μm and 2.87 μm, respectively. These results indicate that MIL-68(M) has wideband nonlinear optical properties and promising application prospects in the field of optical modulators at 2- and 2.9-μm mid-infrared waveband. Work clearly accessible to a broad readership.
Introduction
Mid-infrared pulsed fiber laser sources operating in the 2 and 3 μm spectral regions have remained a research hotspot attributed to their numerous applications in remote sensing, spectroscopy, free-space communications, and laser surgery [1–6]. Compared with actively modulated pulsed lasers, the passively modulated ones with saturable absorbers, such as passively Q-switched and passively mode-locked lasers, show the merits of low cost and simple structure without the requirement of high-voltage and RF drivers. In recent years, a variety of nanomaterials with unique electronic structures and significant nonlinear optical properties have drawn great attention due to their wide application in areas such as all-optical switches, photo-detectors, optical modulators and pulsed lasers [7–13]. Among them, two-dimensional (2D) nanomaterials, such as graphene, transition metal dichalcogenides (TMDs), black phosphorus (BP), topological insulators (TIs), bismuthene, MXene, and antimonene, with their 2D planar structure, ultrafast carrier dynamics and broadband absorption, have been widely investigated for their excellent optical and optoelectronic properties [14, 15]. Especially in the field of pulsed fiber lasers, they have been successfully used as saturable absorbers for short pulse generation at various wavebands, driving the development of pulsed fiber lasers [16–32]. Some binary chalcogenides (SnS, PbS, and In2S3) have also shown saturable absorption properties [33–35]. However, they still have limitations. For example, the weak absorption of graphene makes it difficult to ensure a suitable modulation depth for pulse generation [36]. TMDs is mainly used to implement pulsed fiber lasers in the visible spectral range while the large direct band gap limits their application in the mid-infrared region [20, 37]. Although BP is the most stable allotrope of the phosphorus, it is prone to oxidation and reacts more strongly when it was exposed to water, limiting its application due to the poor stability [38]. In recent years, metal-organic frameworks materials (MOFs), microcrystalline porous materials self-assembled from metal ions or clusters and organic ligands, have received increasing attention and research due to their remarkable advantages including large surface area, ordered reticular structure, excellent electrical conductivity, excellent optical transparency [39]. MOFs have been extensively applied in the fields of chemical sensing, catalytic, gas storage, molecular magnets and nonlinear optical [40–44]. In recent years, the use of MOFs and their derived nanomaterials as saturable absorbers in fiber laser pulse generation is also being gradually investigated, such as nickel-p-benzenedicarboxylic acid MOFs (Ni-MOFs) [45, 46], zeolitic imidazolate framework-8 (ZIF-8) [47], NiO-MOF [48], rGO-Co3O4 [49].
As a typical kind of MOF, MIL-68(M) (M = Fe3+, Al3+, In3+ etc.) are built from the infinite chains of corner-sharing metal-centered octahedral MO4(OH)2 linked through hydroxyl groups and terephthalate ligands [50, 51]. The organic ligand (terephthalate) and metal atoms of MIL-68(M) are orderly assembled in a layer-by-layer manner, exhibiting 2D crystalline structure. MIL-68(M) has both triangular and hexagonal pores, demonstrating high chemical stability, high surface area and sufficient thermal stability up to 500°C [51–54]. The outstanding properties make them attractive. Currently, it has been shown that MIL-68(Al) and MIL-68(Fe) have good nonlinear optical properties at 2.8 μm [55]. However, the investigation on the nonlinear properties in other wavelength bands is still lacking.
Herein, MIL-68(Al) and MIL-68(Fe) were prepared by hydrothermal method and we investigated their nonlinear optical properties in 2 μm and 2.9 μm regions. Furthermore, pulse generation was achieved in both Tm3+-doped and Ho3+/Pr3+ co-doped fiber lasers by using MIL-68(Al) and MIL-68(Fe) as SAs, operating at 2 μm and 2.9 μm, respectively. These results indicate that MIL-68(Al) and MIL-68(Fe) can be developed as promising broadband SAs for mid-infrared pulses generation.
Preparation and characterization of MIL-68(Al) and MIL-68(Fe)
The synthesis of MIL-68(Al) and MIL-68(Fe) is the same as the literature [55]. The morphology of MIL-68(Al) crystals was observed by transmission electron microscopy (TEM), as shown in Figure 1A. According to the TEM image, the prepared MIL-68(Al) are clusters of needlelike crystals with different lengths [56]. Figure 1B shows the XRD pattern of MIL-68(Al) crystals. All the characteristic peaks of the MIL-68(Al) material are similar to those previous literatures [57, 58].
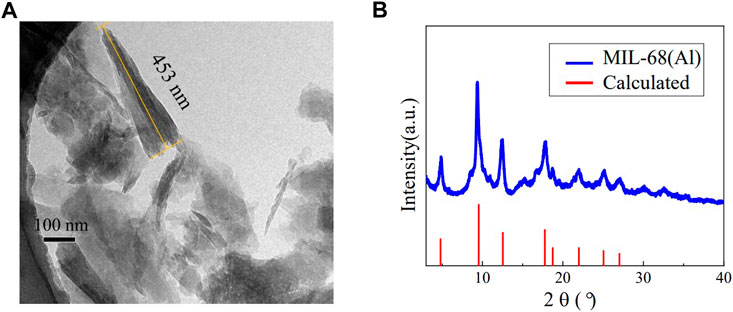
FIGURE 1. (A) TEM image of MIL-68(Al) with a 100 nm scale; (B) XRD pattern of as-synthesized MIL-68(Al).
Similarly, the as-prepared MIL-68 (Fe) sample was also characterized. Figure 2A shows the TEM image and the size of the as-synthesized MIL-68(Fe) is about 1–3 μm. As shown in Figure 2B, the positions of the typical peaks of the XRD pattern matched well with the previous work, indicating that the crystal lattice parameters (cell length and angle) are the same but differ in relative intensity. However, the relative intensities are different influenced by the meritocratic orientation [59].
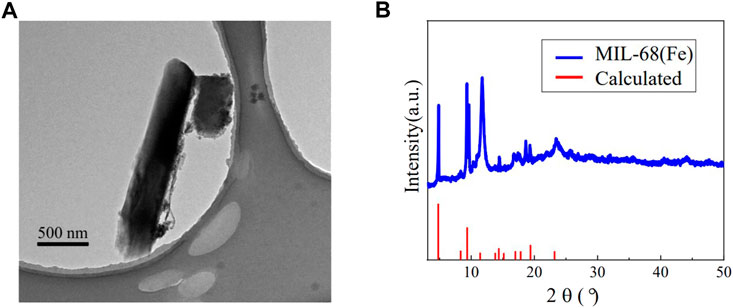
FIGURE 2. (A) TEM image of MIL-68(Fe) with a 500 nm scale; (B) XRD pattern of as-synthesized MIL-68(Fe).
Figure 3 shows the measurement setup of the nonlinear absorptions. A self-made laser which generates pulse duration of 1.42 ps at 1.99 μm was used as one of the laser sources, as shown in Figure 3A. An optical output coupler with 50:50 fiber-pigtailed was used to simultaneously detect the reference signal and absorption. MIL-68(Al) or MIL-68(Fe) were coated on a CaF2 window plate (F1) and the F2 was an uncoated CaF2 window plate. Two detectors (D1 and D2) were used to measure the average powers. In addition, we used a homemade mode-locked fiber laser operating at 2.87 μm as the other laser source. The repetition rate is 18.39 MHz and a pulse duration is ∼20 ps. The balanced twin detector system was elaborated in Ref. [19], as shown in Figure 3B.
The parameters of the SAs were fitted with the following formula:
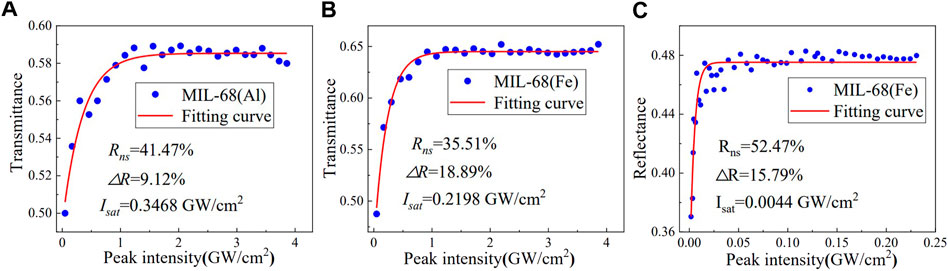
FIGURE 4. Transmittance and reflectance of the samples as a function of pulse peak intensity. (A) MIL-68(Al) at 1.99 μm; (B) MIL-68(Fe) at 1.99 μm; (C) MIL-68(Fe) at 2.87 μm.
Tm: Fiber Q-switched laser
Experiment setup
The experimental setup of the passively Q-switched Tm3+-doped fiber laser is depicted in Figure 5. An all-fiber-integrated ring cavity configuration was adopted. The pump source was a 12 W commercial 793 nm diode laser (BWT). The gain fiber was a 5.8 m double-clad Tm3+-doped fiber (Coractive, 4 dB/m absorption at790 nm) which has a diameter of 128 μm and a numerical aperture (NA) of 0.22. The gain fiber was pumped via a
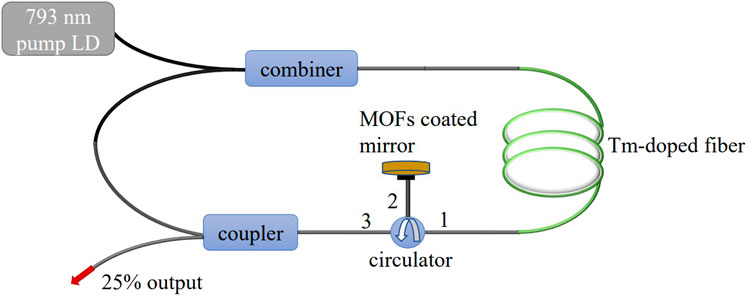
FIGURE 5. Experimental setup of the passively Q-switched Tm3+-doped fiber laser based on the MOFs SA.
Results and discussion
The MIL-68(Al)-based passively Q-switched operation self-stared when the pump power was increased to 2.06 W, as shown in Figure 6A. A repetition rate of 38.81 kHz and a pulse duration of 5.73 μs were obtained. As the pump power gradually increased to 2.57 W, the pulse sequence maintained a stable Q-switched state with a repetition rate of 42.44 kHz and the minimum pulse duration of 2.13 μs. Once the pump power exceeded 2.57 W, the pulse trains started to grow erratic and then faded away. Nevertheless, stable Q-switching operation could be recaptured when the pump power was decreased, demonstrating that the MIL-68(Al) was not damaged by the photothermal effect [19, 32]. The high optical damage threshold of MIL-68(Al) was also confirmed [55]. The Q-switched output power increased from 1.37 mW to 3.08 mW and the maximum pulse energy was 0.073 μJ, as displayed in Figure 6B. Accordingly, the highest peak power was calculated to be 0.034 W. The inset shows that the center wavelength of the pulsed laser is 1988.5 nm. The repetition rate increased from 38.81 kHz to 42.44 kHz as the pump power increased, as displayed in Figure 6C. Specifically, the pulse duration decreased from 5.73 μs to 2.13 μs. As shown in the inset, the signal-to-noise ratio (SNR) of 41.1 dB was measured at the frequency of 42.44 kHz, indicating a stable Q-switched operation.
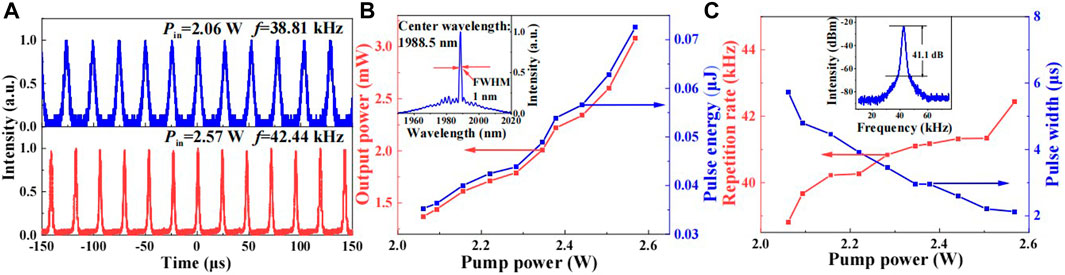
FIGURE 6. (A) Q-switched pulse trains at the launched pump power of 2.06 W and 2.57 W; (B) Output power and single-pulse energy as functions of the pump power, inset: optical spectra of the Q-switched pulses; (C) repetition rate and pulse width as functions of the pump power, inset: RF spectra of the Q-switched pulses.
When MIL-68(Al) was replaced with MIL-68(Fe) in the same experimental setup, the MIL-68(Fe)-based passively Q-switched operation self-started as the pump power raised to 1.59 W, as shown in Figure 7A. The repetition rate was 18.89 kHz. Stable Q-switching operation without any adjustment was sustained until the launched pump power of 2.79 W. The repetition rate and pulse duration were 50.32 kHz and 3.37 µs, respectively. With further increasing the launched pump power, the Q-switching began unstable and then disappeared. When we reduced the pump power to less than 2.79 W again, stable Q-switched operation could be observed again. As the launched pump power rising from 1.59 W to 2.79 W, the Q-switched output power and pulse energy both increased, as shown in Figure 7B. The maximum output power of 13.3 mW and the maximum pulse energy of 0.26 μJ were received. Accordingly, the highest peak power of 0.078 W was obtained. The center wavelength was 1973.3 nm, as shown in the inset. Figure 7C shows the variation of Q-switched pulses over the same pump range. The repetition rate raised from 18.89 kHz to 50.32 kHz while the pulse duration decreased from 5.72 μs to 3.37 μs. The measured signal-to-noise ratio (SNR) was 52.5 dB at the frequency of 50.32 kHz, indicating a stable Q-switched operation.
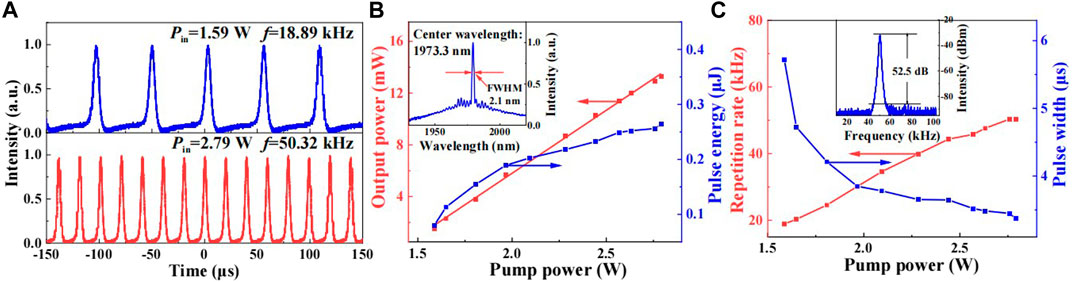
FIGURE 7. (A) Q-switched pulse trains at the launched pump power of 1.59 W and 2.79 W; (B) Output power and single-pulse energy as functions of the pump power, inset: optical spectra of the Q-switched pulses; (C) repetition rate and pulse width as functions of the pump power, inset: RF spectra of the Q-switched pulses.
Mode-locked operation of the two fiber lasers were not observed in our experiments. This may be related to the parameters of SAs and/or the current laser resonator design. By comparing selected all-fiber Q-switched lasers operating in the 2 μm region in Table 1, the minimum pulse width of MIL-68(Al) in this work is better and the maximum pulse energy of MIL-68(Fe) measured in this system is higher than most of materials reported previously [20–22, 30, 31]. Compared with traditional SESAM, MOFs has the characteristics of simple manufacturing process and compared with low-dimensional materials like BP and TMDs, MOFs exhibits better physiochemical stability with high laser damage threshold, as well as the high temperature stability (∼500°C). In addition, the pulse width obtained by using MIL-68(Al) as SA is narrower and the pulse energy is higher compared to the one when using MIL-68(Fe) as SA, while the average output power and peak power obtained by using MIL-68(Fe) as SA are both higher compared to the ones when using MIL-68(Al) as SA.
Ho3+/Pr3+: Fiber Q-switched laser
Experiment setup
We have also studied the optical performance in the 3-μm waveband of MIL-68(Fe). We established the MIL-68(Fe) SAs enabled passively Q-switched mid-infrared fiber laser. Figure 8 shows the experimental setup of the passively Q-switched fiber laser based on MIL-68(Fe). The pump light was a commercial laser diodes (LD) (Eagleyard Photonics) operating at 1,150 nm. A 5.5 m long Ho3+/Pr3+ co-doped ZBLAN fiber (FiberLabs) was used as the gain fiber. The core diameter is 10 µm and numerical aperture (NA) is 0.2. The front end of fiber was vertically cut to the fiber axis to provide a 4% feedback. A dichroic mirror (DM) which has a high reflectivity at 2.8 μm and high transparency at 1,150 nm was 45°placed to be used as the output coupler. An anti-reflection CaF2 plano-convex lens (L1:
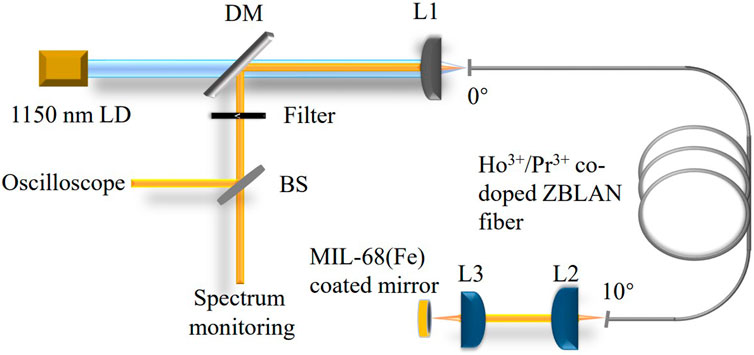
FIGURE 8. Experimental setup of the passively Q-switched Ho3+/Pr3+-doped ZBLAN fiber laser based on the MOFs SA.
Results and discussion
The fiber laser started Q-switching operation when the incident pump power was increased to 0.46 W, as shown in Figure 9A. The repetition rate and pulse duration were 182.0 kHz and 1.26 μs. The Q-switching operation can be maintained until the pump power of 1.26 W. The shortest pulse width of 497 ns was obtained with a repetition rate of 189.9 kHz. Figure 9B shows the Q-switched average output power and pulse energy over the same incident pump power. The output power increased linearly with the pump power and the maximum output power was 107.2 mW. The maximum pulse energy of 0.56 μJ and peak power of 1.14 W were obtained. The inset shows the Q-switched pulse spectrum and the center wavelength locates at 2,864.3 nm. The full width at half maxima (FWHM) was 1.6 nm. The repetition rate raised from 182.0 kHz to 189.9 kHz while the pulse width decreased from 1.26 μs to 497 ns, as displayed in Figure 9C. The radio-frequency (RF) spectrum with a signal-to-noise ratio (SNR) of 33.1 dB was measured at the repetition rate of 189.9 kHz, as shown in the inset of Figure 9C.
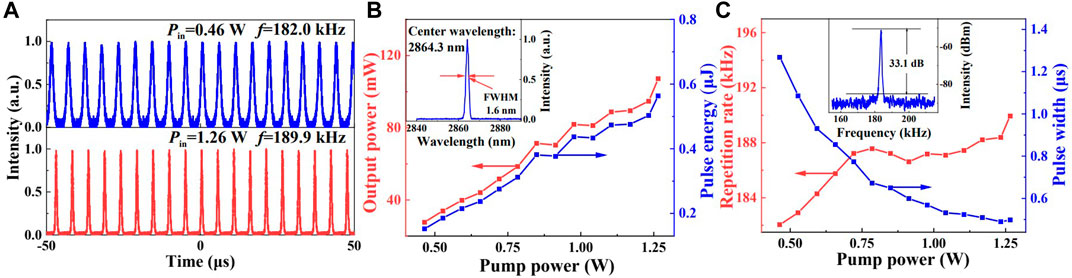
FIGURE 9. (A) Q-switched pulse trains at the launched pump power of 0.46 W and 1.26 W; (B) Output power and single-pulse energy as functions of the pump power, inset: optical spectra of the Q-switched pulses; (C) repetition rate and pulse width as functions of the pump power, inset: RF spectra of the Q-switched pulses.
In contrast to the output characteristics of 2.9 µm Q-switched Ho3+ and Ho3+/Po3+ co-doped fiber lasers modulated by the typical 2D materials, a stable passive Q-switched laser with a short pulse width of 497 ns, which is the shortest one, as far as we know, was investigated based on a novel MIL-68(Fe)-SA. As can be seen in Table 2, The generated pulse peak power manifested the advantages compared with materials like graphene [17], PtSe2 [19], WS2 [23], antimonene [32] etc. In addition, the MIL-68(Al) and MIL-68(Fe) SAs used in our experiments were stored in the thermostat for two to 3 months and then the Q-switched laser experiments were repeated. Stable Q-switching pulses can still be achieved, although the pulse performances are slightly different at a given pump power. The results show that MIL-68(Al) and MIL-68(Fe) are SA materials with long-term stability in the mid-infrared spectral range.
Conclusion
In summary, MIL-68(Al) and MIL-68(Fe) were fabricated by hydrothermal method and the saturable absorption properties were characterized under 1.99 µm and 2.87 µm laser irradiation, respectively. We developed Tm3+-doped fiber laser operating at 1988.5 nm using MIL-68(Al) as SA. In addition, we developed MIL-68(Fe) Q-switched Tm3+-doped and Ho3+/Pr3+ co-doped fiber lasers, operating at 1.98 µm and 2,864.3 nm, with pulse durations of 3.37 µs and 467 ns, respectively. Our results show the potential of MIL-68 (M, M = Al3+, Fe3+) with excellent optical properties and extraordinary opportunities for application in mid-infrared spectral region.
Data availability statement
The original contributions presented in the study are included in the article/supplementary materials, further inquiries can be directed to the corresponding author.
Author contributions
All authors listed have made a substantial, direct, and intellectual contribution to the work and approved it for publication.
Funding
National Natural Science Foundation of China (61875033 and 61421002); Science and Technology Planning Project of Sichuan Province (2022NSFSC1790 and 2020ZHCG0087). Fundamental Research Funds for the Central Universities (YGX2019J051).
Conflict of interest
The authors declare that the research was conducted in the absence of any commercial or financial relationships that could be construed as a potential conflict of interest.
Publisher’s note
All claims expressed in this article are solely those of the authors and do not necessarily represent those of their affiliated organizations, or those of the publisher, the editors and the reviewers. Any product that may be evaluated in this article, or claim that may be made by its manufacturer, is not guaranteed or endorsed by the publisher.
References
1. Godard A. Infrared (2–12 μm) solid-state laser sources: A review. Comptes Rendus Physique (2007) 8(10):1100–28. doi:10.1016/j.crhy.2007.09.010
2. Boulnois JL. Photophysical processes in recent medical laser developments: A review. Laser Med Sci (1986) 1(1):47–66. doi:10.1007/BF02030737
3. Ebrahim-Zadeh M, Sorokina IT. Mid-infrared coherent sources and applications NATO science for peace and security series B: Physics and biophysics. Springer (2008). p. 467–6122.
4. Skorczakowski M, Swiderski J, Pichola W, Nyga P, Zajac A, Maciejewska M, et al. Mid-infrared Q-switched Er: YAG laser for medical applications. Laser Phys Lett (2010) 7(7):498–504. doi:10.1002/lapl.201010019
5. Halmer D, Thelen S, Hering P, Murtz M. Online monitoring of ethane traces in exhaled breath with a difference frequency generation spectrometer. Appl Phys B (2006) 85(2):437–43. doi:10.1007/s00340-006-2288-9
6. Scherer JJ, Paul JB, Jost HJ, Fischer ML. Mid-IR difference frequency laser-based sensors for ambient CH4, CO, and N2O monitoring. Appl Phys B (2013) 110(2):271–7. doi:10.1007/s00340-012-5244-x
7. Sun Z, Martinez A, Wang F. Optical modulators with 2D layered materials. Nat Photon (2016) 10:227–38. doi:10.1038/nphoton.2016.15
8. Xia F, Mueller T, Lin Y, Valdes-Garcia A, Avouris P. Ultrafast graphene photodetector. Nat Nanotechnol (2009) 4:839–43. doi:10.1038/nnano.2009.292
9. Zhang X, Ouyang H, Miao R, Sui Y, Hao H, Tang Y, et al. Anisotropic nonlinear optical properties of a SnSe flake and a novel perspective for the application of all-optical switching. Adv Opt Mater (2019) 7(18):1900631. doi:10.1002/adom.201900631
10. Keller U. Recent developments in compact ultrafast lasers. Nature (2003) 424:831–8. doi:10.1038/nature01938
11. Mu H, Wang Z, Yuan J, Xiao S, Chen C, Chen Y, et al. Graphene–Bi2Te3 heterostructure as saturable absorber for short pulse generation. ACS Photon (2015) 2:832–41. doi:10.1021/acsphotonics.5b00193
12. Li X, Xu W, Wang Y, Zhang X, Hui Z, Zhang H, et al. Optical-intensity modulators with PbTe thermoelectric nanopowders for ultrafast photonics. Appl Mater Today (2022) 28(8):101546. doi:10.1016/j.apmt.2022.101546
13. Zhang C, Li X, Chen E, Liu H, Shum P, Chen X. Hydrazone organics with third-order nonlinear optical effect for femtosecond pulse generation and control in the L-band. Opt Laser Technology (2022) 151(9):108016. doi:10.1016/j.optlastec.2022.108016
14. Ma C, Wang C, Gao B, Adams J, Wu G, Zhang H. Recent progress in ultrafast lasers based on 2D materials as a saturable absorber. Appl Phys Rev (2019) 6(4):041304. doi:10.1063/1.5099188
15. Jiang T, Yin K, Wang C, You J, Ouyang H, Miao R, et al. Ultrafast fiber lasers mode-locked by two-dimensional materials: Review and prospect. Photon Res (2020) 8(1):78–90. doi:10.1364/PRJ.8.000078
16. Zhang M, Kelleher EJR, Torrisi F, Sun Z, Hasan T, Popa D, et al. Tm-doped fiber laser mode-locked by graphene-polymer composite. Opt Express (2012) 20(22):25077–84. doi:10.1364/OE.20.025077
17. Wei C, Zhu XH, Wang F, Xu Y, Balakrishnan K, Song F, et al. Graphene Q-switched 2.78 μm Er3+-doped fluoride fiber laser. Opt Lett (2013) 38:3233–6. doi:10.1364/OL.38.003233
18. Tiu ZC, Ooi SI, Guo J, Zhang H, Ahmad H. Review: Application of transition metal dichalcogenide in pulsed fiber laser system. Mater Res Express (2019) 6:082004. doi:10.1088/2053-1591/ab2257
19. Wei C, Chi H, Jiang S, Zheng L, Zhang H, Liu Y. Long-term stable platinum diselenide for nanosecond pulse generation in a 3-µm mid-infrared fiber laser. Opt Express (2020) 28(22):33758–66. doi:10.1364/OE.410110
20. Woodward RI, Howe RCT, Runcorn TH, Hu G, Torrisi F, Kelleher EJR, et al. Wideband saturable absorption in few-layer molybdenum diselenide (MoSe2) for Q-switching Yb-Er- and Tm-doped fiber lasers. Opt Express (2015) 23:20051. doi:10.1364/oe.23.020051
21. Ahmad H, Reduan SA, Aidit SN, Yusoff N, Maah MJ, Ismail MF, et al. Ternary MoWSe2 alloy saturable absorber for Passively Q-switched thulium-doped fiber laser with silver-nanoparticle film as the saturable absorber for operation at 2.0 μm Yb-Er- and Tm-doped fiber laser. Opt Commun (2019) 437:355–62. doi:10.1016/j.optcom.2019.01.009
22. Ahmad H, Sharbirin AS, Ismail MF. Molybdenum tungsten disulphide (MoWS2) as a saturable absorber for a passively Q-switched thulium/holmium-co doped fiber laser. J Mod Opt (2019) 66:1163–71. doi:10.1080/09500340.2019.1609612
23. Wei C, Luo H, Zhang H, Li C, Xie J, Li J. Passively Q-switched mid-infrared fluoride fiber laser around 3 μm using a tungsten disulfide (WS2) saturable absorber. Laser Phys Lett (2016) 13:105108. doi:10.1088/1612-2011/13/10/105108
24. Chen Y, Jiang G, Chen S, Guo Z, Yu X, Zhao C, et al. Mechanically exfoliated black phosphorus as a new saturable absorber for both Q-switching and mode-locking laser operation. Opt Express (2015) 23(10):12823–33. doi:10.1364/OE.23.012823
25. Qin ZP, Xie GQ, Ma JG, Yuan P, Qian LJ. 2.8 μm all-fiber Q-switched and mode-locked lasers with black phosphorus. Photon Res (2018) 6:1074–8. doi:10.1364/PRJ.6.001074
26. Dou Z, Song Y, Tian J, Liu J, Yu Z, Fang X. Mode-locked ytterbium-doped fiber laser based on topological insulator: Bi_2Se_3. Bi2se3 Opt Express (2014) 22(20):24055–61. doi:10.1364/OE.22.024055
27. Guo B, Wang SH, Wu ZX, Wang ZX, Wang DH, Huang H, et al. Sub-200 fs soliton mode-locked fiber laser based on bismuthene saturable absorber. Opt Express (2018) 26(18):22750–60. doi:10.1364/OE.26.022750
28. Wu Q, Jin X, Chen S, Jiang X, Hu Y, Jiang Q, et al. MXene-based saturable absorber for femtosecond mode-locked fiber lasers. Opt Express (2019) 27(7):10159–70. doi:10.1364/OE.27.010159
29. Wei C, Zhou L, Wang D, Chi H, Huang H, Zhang H, et al. MXene-Ti3C2Tx for watt-level high-efficiency pulse generation in a 2.8 μm mid-infrared fiber laser. Photon Res (2020) 8(6):972–7. doi:10.1364/PRJ.388930
30. Ahmad H, Kamely AA, Yusoff N, Bayang L, Samion MZ. Generation of Q-switched pulses in Thulium doped and Thulium/Holmium-co-doped fiber lasers using MAX phase (Ti3AlC2). Sci Rep (2020) 10:9233. doi:10.1038/s41598-020-66141-3
31. Ahmad H, Ismail NN, Aidit SN, Reduan S, Samion M, Yusoff N. 2.08 μm Q-switched holmium fiber laser using niobium carbide-polyvinyl alcohol (Nb2C-PVA) as a saturable absorber. Opt Commun (2021) 490:126888. doi:10.1016/j.optcom.2021.126888
32. Luo HY, Tian XL, Gao Y, Wei RF, Li JF, Qiu JR, et al. Antimonene: A long-term stable two-dimensional saturable absorption material under ambient conditions for the mid-infrared spectral region. Photon Res (2018) 6:900–7. doi:10.1364/PRJ.6.000900
33. Xie Z, Zhang F, Liang Z, Fan T, Li Z, Jiang X, et al. Revealing of the ultrafast third-order nonlinear optical response and enabled photonic application in two-dimensional tin sulfide. Photon Res (2019) 7(5):494–502. doi:10.1364/PRJ.7.000494
34. Liu X, Li X, Tang Y, Zhang S. PbS nanoparticles saturable absorber for ultrafast pulse generation in 2-µm fiber laser. Opt Lett (2020) 45(1):161–4. doi:10.1364/OL.45.000161
35. Ma X, Chen W, Tong L, Liu S, Dai W, Ye S, et al. In2S3-based saturable absorber for passively harmonic mode-locking in 2μm region. Opt Laser Technology (2022) 145:107476. doi:10.1016/j.optlastec.2021.107476
36. Martinez A, Sun Z. Nanotube and graphene saturable absorbers for fibre lasers. Nat Photon (2013) 7(11):842–5. doi:10.1038/nphoton.2013.304
37. Luo Z, Huang Y, Zhong M, Li Y, Wu J, Xu B, et al. 1-1.5-and 2-μm fiber lasers Q-switched by a broadband few-layer MoS2 saturable absorber. J Lightwave Technol (2014) 32:4679–86. doi:10.1109/JLT.2014.2362147
38. Favron A, Gaufrès E, Fossard F, Phaneuf-L’Heureux A, Tang NYW, Lévesque PL, et al. Photooxidation and quantum confinement effects in exfoliated black phosphorus. Nat Mater (2015) 14(8):826–32. doi:10.1038/nmat4299
39. Yaghi OM, O’Keeffe M, Ockwig NW, Chae HK, Eddaoudi M, Kim J. Reticular synthesis and the design of new materials. Nature (2003) 423(6914):705–14. doi:10.1038/nature01650
40. Qu F, Jiang H, Yang M. Designed formation through a metal organic framework route of ZnO/ZnCo2O4 hollow core–shell nanocages with enhanced gas sensing properties. Nanoscale (2016) 8:16349–56. doi:10.1039/C6NR05187A
41. Furukawa H, Cordova K, O’Keeffe M, Yaghi O. The chemistry and applications of metal-organic frameworks. Science (2013) 341(6149):1230444. doi:10.1126/science.1230444
42. Kuppler RJ, Timmons DJ, Fang QR, Li JR, Makal TA, Young MD, et al. Potential applications of metal-organic frameworks. Coord Chem Rev (2009) 253(23):3042–66. doi:10.1016/j.ccr.2009.05.019
43. Silva P, Vilela S, Tome J, Almeida PF. Multifunctional metal–organic frameworks: From academia to industrial applications. Chem Soc Rev (2015) 44(19):6774–803. doi:10.1039/c5cs00307e
44. Evans OR, Lin W. Crystal engineering of NLO materials based on metal-organic coordination networks. Acc Chem Res (2002) 35(7):511–22. doi:10.1021/ar0001012
45. Jiang X, Zhang L, Liu S, Zhang Y, He Z, Li W, et al. Ultrathin metal-organic framework: An emerging broadband nonlinear optical material for ultrafast photonics. Adv Opt Mater (2018) 6(16):1800561. doi:10.1002/adom.201800561
46. Zhang Q, Jiang X, Zhang M, Jin X, Zhang H, Zheng Z. Wideband saturable absorption in metal–organic frameworks (MOFs) for mode-locking Er- and Tm- doped fiber lasers. Nanoscale (2020) 12(7):4586–90. doi:10.1039/C9NR09330C
47. Dong L, Chu H, Li Y, Zhao S, Li D. Broadband optical nonlinearity of zeolitic imidazolate framework-8 (ZIF-8) for ultrafast photonics. J Mater Chem C Mater (2021) 9(28):8912–9. doi:10.1039/D1TC01665B
48. Zhang C, Liu J, Gao Y, Li X, Lu H, Wang Y, et al. Porous nickel oxide micron polyhedral particles for high-performance ultrafast photonics. Opt Laser Technology (2022) 146(40):107546. doi:10.1016/j.optlastec.2021.107546
49. Li X, An M, Li G, Han Y, Guo P, Chen E, et al. MOF-derived porous dodecahedron rGO-Co3O4 for robust pulse generation. Adv Mater Inter (2022) 9(5):2101933. doi:10.1002/admi.202101933
50. Barthelet K, Marrot J, Ferey G, Riou D. III(OH){O2C–C6H4–CO2}.(HO2C–C6H4–CO2H)x(DMF)y(H2O)z (or MIL-68), a new vanadocarboxylate with a large pore hybrid topology : Reticular synthesis with infinite inorganic building blocks? Chem Commun (2004) 5:520–1. doi:10.1039/b312589k
51. Volkringer C, Meddouri M, Loiseau T, Guillou N, Marrot J, Ferey G, et al. The kagome topology of the gallium and indium metal-organic framework types with a MIL-68 structure: Synthesis, XRD, solid-state NMR characterizations, and hydrogen adsorption. Inorg Chem (2008) 47(24):11892–901. doi:10.1021/ic801624v
52. Yang Q, Vaesen S, Vishnuvarthan M, Ragon F, Serre C, Vimont A, et al. Probing the adsorption performance of the hybrid porous MIL-68(Al): A synergic combination of experimental and modelling tools. J Mater Chem (2012) 22(10):10210–20. doi:10.1039/c2jm15609a
53. Fateeva A, Horcajada P, Devic T, Serre C, Marrot J, Grenèche JM, et al. Synthesis, structure, characterization, and redox properties of the porous MIL-68(Fe) solid. Eur J Inorg Chem (2010) 2010(24):3789–94. doi:10.1002/ejic.201000486
54. Seoane B, Sebastian V, Tellez C, Coronas J. Crystallization in THF: The possibility of one-pot synthesis of mixed matrix membranes containing MOF MIL-68(Al). CrystEngComm (2013) 15(45):9483–90. doi:10.1039/c3ce40847g
55. Zheng L, Wei C, Zhou H, Sun F, Gao A, Wang D, et al. Mid-infrared optical switches enabled by metal-organic frameworks for compact high-power nanosecond laser sources at 3 µm. Opt Express (2022) 30(8):12409–19. doi:10.1364/OE.455854
56. Jiang Y, Zhang B, Li J, Sun Y, Wang X, Ma P, et al. One-step fabrication of hydrophilic MIL-68(Al)/Chitosan-coated melamine sponge for vortex-assisted solid-phase extraction of parabens in water samples. Talanta (2021) 1(224):121799. doi:10.1016/j.talanta.2020.121799
57. Tehrani MS, Zare-Dorabei R. Competitive removal of hazardous dyes from aqueous solution by MIL-68(Al): Derivative spectrophotometric method and response surface methodology approach. Spectrochimica Acta A: Mol Biomol Spectrosc (2016) 160:8–18. doi:10.1016/j.saa.2016.02.002
58. Embrechts H, Kriesten M, Ermer M, Peukert W, Hartmann M, Distaso M. In situ Raman and FTIR spectroscopic study on the formation of the isomers MIL-68(Al) and MIL-53(Al). RSC Adv (2020) 10:7336–48. doi:10.1039/c9ra09968a
Keywords: metal-organic frameworks, mid-infrared, pulsed fiber lasers, saturable absorber, MIL-68(Al), MIL-68(Fe)
Citation: Zheng L, Sun F, Zhou H, Gao A, Liu W, Wang D, Wei C and Liu Y (2022) MIL-68(Al) and MIL-68(Fe) as broadband optical modulators for Q-switching fiber lasers operating at 2 and 2.9 μm. Front. Phys. 10:1066166. doi: 10.3389/fphy.2022.1066166
Received: 10 October 2022; Accepted: 18 November 2022;
Published: 01 December 2022.
Edited by:
Houkun Liang, Sichuan University, ChinaCopyright © 2022 Zheng, Sun, Zhou, Gao, Liu, Wang, Wei and Liu. This is an open-access article distributed under the terms of the Creative Commons Attribution License (CC BY). The use, distribution or reproduction in other forums is permitted, provided the original author(s) and the copyright owner(s) are credited and that the original publication in this journal is cited, in accordance with accepted academic practice. No use, distribution or reproduction is permitted which does not comply with these terms.
*Correspondence: Chen Wei, Y3dlaUB1ZXN0Yy5lZHUuY24=