- 1Center for Engineering in Medicine and Surgery, Massachusetts General Hospital, Harvard Medical School, Boston, MA, United States
- 2Shriners Hospitals for Children, Boston, MA, United States
- 3Department of Surgery, Beth Israel Deaconess Medical Center, Boston, MA, United States
- 4Department of Surgery, Division of Transplantation, Massachusetts General Hospital, Harvard Medical School, Boston, MA, United States
- 5Department of Electron Microscopy Research, Theodor Bilharz Research Institute, Giza, Egypt
- 6Sylvatica Biotech Inc, North Charleston, SC, United States
- 7Department of Mechanical Engineering, Carnegie Mellon University, Pittsburgh, PA, United States
- 821st Century Medicine, Fontana, CA, United States
Introduction: The current liver organ shortage has pushed the field of transplantation to develop new methods to prolong the preservation time of livers from the current clinical standard of static cold storage. Our approach, termed partial freezing, aims to induce a thermodynamically stable frozen state at high subzero storage temperatures (−10°C to −15°C), while simultaneously maintaining a sufficient unfrozen fraction to limit ice-mediated injury.
Methods and results: Using glycerol as the main permeating cryoprotectant agent, this research first demonstrated that partially frozen rat livers showed similar outcomes after thawing from either −10°C or −15°C with respect to subnormothermic machine perfusion metrics. Next, we assessed the effect of adding ice modulators, including antifreeze glycoprotein (AFGP) or a polyvinyl alcohol/polyglycerol combination (X/Z-1000), on the viability and structural integrity of partially frozen rat livers compared to glycerol-only control livers. Results showed that AFGP livers had high levels of ATP and the least edema but suffered from significant endothelial cell damage. X/Z-1000 livers had the highest levels of ATP and energy charge (EC) but also demonstrated endothelial damage and post-thaw edema. Glycerol-only control livers exhibited the least DNA damage on Terminal deoxynucleotidyl transferase dUTP nick end labeling (TUNEL) staining but also had the lowest levels of ATP and EC.
Discussion: Further research is necessary to optimize the ideal ice modulator cocktail for our partial-freezing protocol. Modifications to cryoprotective agent (CPA) combinations, including testing additional ice modulators, can help improve the viability of these partially frozen organs.
Introduction
The liver organ shortage has pushed the field of transplantation to develop bold new strategies to preserve transplantable organs. Currently, the clinical standard of preserving transplantable livers is static cold storage (SCS) at 4°C, which keeps livers viable for a maximum of 12 h [1]. Prolonging this preservation time could improve the allocation of organs in many ways. For example, it would reduce organ discard due to unacceptably long cold ischemic times, lower operating room costs by making liver transplant (LT) operations elective, enhance donor-recipient selection with human leukocyte antigen (HLA) matching and global matching programs, and make tolerance induction protocols more feasible [2–4].
Most subzero preservation efforts have centered on low cryogenic temperature ranges (<–80°C) [3]. However, recent work has investigated expanding storage of organs for transplant within the high subzero temperature range from –4°C to –20°C [2, 5–11]. These temperatures enable more metabolic depression than hypothermic SCS while also avoiding vitrification-related cryoprotectant toxicity and thermal stresses. Some examples of the potential of high subzero preservation have involved storage below the thermodynamic freezing point in the absence of ice using supercooling or isochoric supercooling (∼–4°C to –6°C for larger systems, like organs) [5, 7, 11]. While these ice-free approaches have shown great promise above –6°C, the risk of accidental ice formation nonetheless increases as a function of decreasing temperature [12]. Alternate approaches are leveraging key survival strategies of freeze-tolerant animals in nature [2, 13] to freeze whole organs down to –15°C using an approach called partial freezing [9]. Central to partial freezing success is initiating ice close to the ice nucleation temperature using ice nucleating particles since this is far less damaging than accidental ice formation. However, further progress is required to describe and address modes of ice-mediated injury, including due to recrystallization.
One potential solution to address ice-mediated damage are ice modulators. Ishine et al. showed that ice modulators such as antifreeze glycoproteins (AFGP) can have protective effects in liver freezing by inhibiting ice recrystallization and preventing ionic leakage through cell membranes at low temperatures, although the authors report damage to the endothelial layer [14]. AFGP has been shown to inhibit both ice recrystallization and ice growth below TM (the thermodynamic freezing point). These glycopeptides, which all comprise of an alanine and threonine backbone, inhibit ice growth in vivo and in vitro by irreversibly binding to multiple faces of ice crystals [15–18]. AFGPs have also been shown to raise the homogenous ice nucleation temperature (TH) by organizing water into a more ice-like state [19]. However, since the temperature range in our partial freezing protocol is well above TH [20], the issues at hand involve the role of AFGP in ice shaping and ice recrystallization inhibition. Although AFGP can shape ice into damaging spicules [21], this effect may be outweighed under our storage conditions by the ice growth and recrystallization inhibitory effects of AFGP. Also, X-1000 is a 2 kilodalton (kDa) water-soluble synthetic polyvinyl alcohol [22] that contains 20% vinyl acetate, which improves the solubility and ice-inhibiting effects of X-1000 presumably by preventing self-association between X-1000 chains. Polyvinyl alcohol is widely used in many biomedical applications as an ice modulator and is known to inhibit ice recrystallization and growth, even in small concentrations [23, 24]. Z-1000 is a synthetic polyglycerol that inhibits heterogeneous ice nucleation [25]. Normally, ice tends to crystalize around tiny impurities called nucleators. Z-1000 specifically binds these nucleators, suppressing the site for ice formation. Together X/Z-1000 has been shown to protect rat hearts during supercooling [26–28] and is functional from 0°C to temperatures below –120°C [29].
In the present study, whole rat livers were partially frozen for up to 5 days at high subzero temperatures (–10°C to –15°C) by combining ice nucleating agents (INAs) and machine perfusion for (un)loading and assessment. Further, two ice modulators, antifreeze glycoprotein (AFGP) and a polyvinyl alcohol/polyglycerol combination (X/Z-1000), were tested for their ability to overcome ice-mediated injury of rodent livers. As compared to previous efforts by Tessier et al. 2022 [9], the present study uses glycerol as the main permeating CPA. Glycerol was selected since outcomes were inferior to ethylene glycol and propylene glycol, enabling the assessment of the ice modulators ability to rescue glycerol-preserved livers from injury. Taken together, livers frozen with the inclusion of either AFGP or X/Z-1000 were compared to the control group (with glycerol as the main CPA) with primary outcomes being subnormothermic machine perfusion (SNMP) metrics, ATP, energy charge (EC), weight gain, and histology.
Materials and methods
Experimental design
Figure 1 outlines the rat liver partial freezing protocol in eight consecutive steps: 1) liver procurement, 2) preconditioning during SNMP, 3) CPA preloading during hypothermic machine perfusion (HMP), 4) loading of final storage solution and ice modulators during HMP, 5) partial freezing of rat liver, 6) thawing of rat liver, 7) unloading of CPAs during HMP, and 8) functional recovery of frozen rat livers during SNMP.
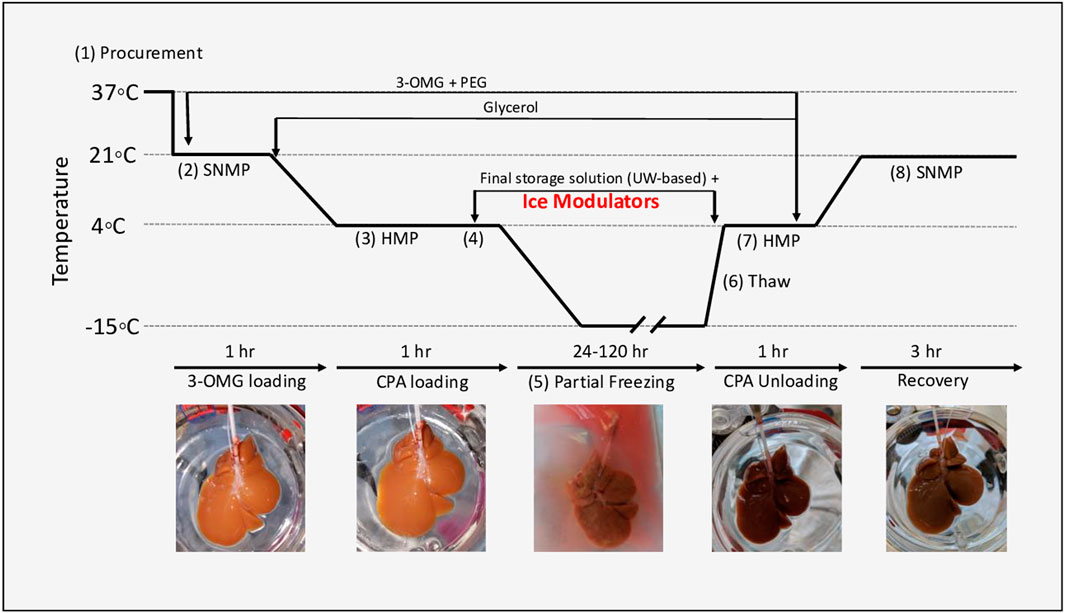
FIGURE 1. Experimental design of partial freezing, in eight consecutive steps: 1) liver procurement, 2) preconditioning during SNMP, 3) CPA preloading during hypothermic machine perfusion (HMP), 4) loading of final storage solution and ice modulators during HMP, 5) partial freezing of rat liver, 6) thawing of rat liver, 7) unloading of CPAs during HMP, and 8) functional recovery of frozen rat livers during SNMP.
Within this protocol, we first compared partially frozen livers at –10°C (n = 4 livers) and –15°C (n = 9 livers) with 12% glycerol as the main permeating CPA. Upon finding minimal differences between these two groups with respect to perfusion-based metrics, we combined them as a control and compared them to livers partially frozen with 0.5 mg/ml (0.05% w/v) of AFGP (n = 4 livers) or 0.1% X-1000/0.2% Z-1000 (total, 0.3% w/v; n = 4 livers) ice modulating agents added to the preservation solution. After freezing, liver viability on SNMP was compared between the 12% glycerol control group and the two ice modulated groups (AFGP and X/Z-1000). The AFGP protein is sourced from a company called A/F protein Inc. (please see Supplementary Table S2). Antifreeze glycoprotein from this company is purified from cold ocean teleost fish at a purity of >98%. All proteins are provided in a dry form and were stored at −20°C. Under these conditions, the company has affirmed a shelf life of 4 years. All AGFP used in this study was stored appropriately and used within a couple months after arrival.
Partial freezing protocol
The rat liver perfusion system involved perfusion through the cannulated portal vein (PV) with regulation of pressure, flow, and temperature. Detailed set-up of the perfusion system has been previously described [30]. The total rat hepatectomy protocol was approved by the Institutional Animal Care and Use Committee (IACUC) of Massachusetts General Hospital (Boston, MA, United States). Livers were procured from male Lewis rats (250–300 g, age 10–12 weeks. Charles River Laboratories, Wilmington, MA, United States) (Figure 1, step 1). The bile duct was isolated and cannulated, and the rats were heparinized with 30 U sodium heparin. PV splenic and gastric branches, as well as the hepatic artery were ligated prior to cannulation. The PV was subsequently cannulated with a 16-gauge catheter and immediately flushed with 40 ml of heparinized saline (1000 U/ml at room temperature). The liver was then freed from all peritoneal attachments, flushed with an additional 20 ml of heparinized saline. After procurement, the liver was weighed, and machine perfusion was immediately initiated.
Preconditioning during SNMP was initiated at 21°C with a flow rate of 5 ml/min (Figure 1, step 2). The flow rate was gradually increased (1 ml/min) until a maximum PV pressure of 5 mmHg, or a flow rate of 25 ml/min, was reached (whichever was reached first). The rat livers were perfused for 1 h with 500 ml of preconditioning solution consisting of Williams E, 200 U/l of insulin, 2% w/v PEG, 50 g/L BSA, 100 mM 3-O-methylglucose (3-OMG), 10,000 U/L of heparin, 24 mg/L of dexamethasone, 25 mg/ml of hydrocortisone, 40,000 ug/L of penicillin, and 40,000 U/L of streptomycin and sodium bicarbonate as needed to maintain a physiological pH (see Supplementary Table S1 for composition of all solutions).
After 1 h of preconditioning during SNMP, the temperature was decreased to 4°C at a rate of ∼1°C/min. Flow rates were gradually adjusted as necessary to ensure a maximum perfusion pressure of 3–5 mmHg during HMP. The SNMP preconditioning solution was switched to 500 ml CPA preloading solution at 4°C (consisting of Williams E, 200 U/L of insulin, 2% PEG, 50 g/L BSA, 100 mM 3-OMG, 30 mM raffinose, 3% hydroxyethyl starch (HES), 6% glycerol, 4000 U/L of heparin, 24 mg/L of dexamethasone, 25 mg/ml of hydrocortisone, 40,000 ug/L of penicillin, 40,000 U/L of streptomycin, and sodium bicarbonate as needed to maintain a physiological pH) (Figure 1, step 3; Supplementary Table S1). HMP was continued for 1 h to ensure complete equilibration of solution throughout the liver parenchyma.
After CPA preloading during HMP, rat livers were loaded with 50 ml of final storage solution (consisting of University of Wisconsin (UW) Solution (Bridge to Life Ltd., Columbia, SC, United States), 5% PEG, 50 mM trehalose, 12% v/v (1.64 M) glycerol, 1 g/L Snomax (Telemet, Hunter, NY, United States) to promote nucleation, 10 U/L of insulin, 24 mg/L of dexamethasone, sodium bicarbonate as needed to maintain a physiological pH, and either 0.5 mg/ml of AFGP or 0.1% x-1000/0.2% z-1000 (Figure 1, step 4). During the perfusion of the final storage solution the liver was perfused at a fixed flow rate of 0.5 ml/min for 30 min, resulting in a perfusion pressure of 3 mmHg.
Once the final storage solution during HMP had been perfused through the liver, the livers were placed in a storage bag with 50 ml of storage solution in a pre-cooled chiller (Engel, Schwertberg, Austria; model no. ENG65-B) for partial freezing (Figure 1, step 5). Note: the concentrations of raffinose and HES were higher in the storage solution than in the pre-loading solution because the storage solution was made with UW which contains both raffinose and HES. In the case of livers frozen with glycerol only, the chiller temperature was pre-cooled to either –10°C or –15°C, and the liver was stored at one of these temperatures for 1–5 days. Livers frozen with either of the two ice modulation candidates were stored at –15°C.
After partial freezing, livers were thawed (Figure 1, step 6) by placement in 50 ml of thawing solution consisting of Williams E, 10 U/L of insulin, 2% PEG, 50 g/L BSA, 100 mM 3-OMG, 30 mM raffinose, 3% HES, 50 mM trehalose, 5 mM L-glutathione, 200 µM cyclic AMP, 1,000 U/L of heparin, 24 mg/L of dexamethasone, 25 mg/ml of hydrocortisone, 40,000 ug/L of penicillin, and 40,000 U/L of streptomycin, which was pre-warmed to 37°C in a constant temperature bath (Thermo Fisher, Waltham, MA, United States). Livers were gently agitated until fully thawed, which required approximately 4–5 min based on superficial observation and thermal equilibration between the final thawing solution temperature and the liver surface temperature of 4°C.
After thawing, CPAs and INAs were unloaded during HMP (Figure 1, step 7). The thawed livers were perfused at 4°C with thawing solution for 60 min with an initial flow rate of 2 ml/min. Flows were increased so as to keep a maximum pressure of 3 mmHg. After 60 min, the perfusion temperature was increased to 21°C, and the perfusion solution was changed to 750 ml of SNMP recovery solution (consisting of Williams E, 20 U/L of insulin, 2% PEG, 50 g/L BSA, 5 mM L-glutathione, 200uM cyclic AMP, 1,000 U/L of heparin, 24 mg/L of dexamethasone, 25 mg/ml of hydrocortisone, 40000 ug/L of penicillin, and 40000 U/l of streptomycin; Supplementary Table S1), discarding the first 50–100 ml to ensure complete CPA removal. Functional recovery of frozen rat livers during SNMP with recovery solution continued for 3 h (Figure 1, step 8) with a maximum intrahepatic perfusion pressure of 5 mmHg and a maximum flow rate of 25 ml/min.
Viability assessment
Perfusate measurements were done hourly during the SNMP recovery period. Time zero (t = 0) was defined as being at approximately 5 min of HMP, and the first outflow samples were taken at this time (flow, 2 ml/min). PV and infrahepatic vena cava (IVC) oxygen partial pressures and lactate levels were measured with a Cg4+ i-STAT cartridge (catalog no. 03P85-50) and handheld blood analyzer. Similarly, potassium and other electrolytes were measured in IVC samples using a Chem 8+ i-STAT cartridge (catalog no. 09P31-26) with the same blood analyzer (catalog no. WD7POC012; Abbott, Chicago, IL, United States).
Rat liver weight was measured directly after procurement, prior to freezing, after thawing, and after viability testing. Weight gain was calculated as the percentage increase at the end of recovery compared to the liver weight after procurement. Vascular resistance was calculated by dividing the perfusion pressure in the PV by the flow rate per gram of liver using the weight of the liver after procurement as the reference standard weight. Oxygen consumption rates were calculated as (pO2in–pO2out)*F/W where pO2in and pO2out were the oxygen contents per ml of inflowing and outflowing perfusate, respectively, and the difference between them multiplied by the perfusion rate (F, in ml/min) provided the total oxygen uptake per minute. This value was then normalized by liver weight (W) to calculate the oxygen uptake per minute, per gram of liver. After thawing and SNMP recovery, rat liver tissue was either flash frozen in liquid nitrogen or fixed in 10% formalin, embedded in paraffin, sectioned, and stained with Hematoxylin and Eosin (H&E). Terminal deoxynucleotidyl transferase dUTP nick end labeling (TUNEL) was also performed on rat liver tissue after freezing to detect DNA breaks as an indicator of apoptosis [7]. Liver tissue that was flash frozen was used to quantify ATP and energy charge (EC) by the Shriners Hospitals for Children Mass Spectrometry Core (Boston, MA, United States).
Statistical analysis was performed with Prism eight software (GraphPad Software, San Diego, CA, United States) with a significance level of 0.05. Analysis of variance (ANOVA), followed by Tukey’s post hoc test (ANOVA/Tukey) was used for the comparison of the time-course perfusion data. ATP and EC in the –10°C vs. –15°C group were compared using unpaired, two-tailed t-tests.
Results
Comparison of partially frozen rat livers at –10°C vs. –15°C with 12% glycerol
Pooled results for livers stored for 1 and 5 days at –10°C (n = 4) or –15°C (n = 9) with a cocktail containing 12% glycerol were compared 1 h after CPA unloading and 3 h after recovery with SNMP at 21°C. There was no statistically significant difference between the two groups with respect to oxygen consumption (Figure 2A), PV lactate (Figure 2B), and vascular resistance (Figure 2C) on two-way ANOVA/Tukey testing. Similarly, there were no statistically significant differences between –10°C and –15°C livers with respect to percent weight gain (Figure 2D), ATP (Figure 2E), EC (Figure 2F), and perfusate potassium (Supplementary Figure S1A) by unpaired, two-tailed t-testing. Thus, perfusion metrics of rat livers partially frozen with 12% glycerol at –10°C and –15°C were not statistically different, and as a result, were grouped together to form a more robust control group (n = 13) for comparison against the ice modulator groups. However, it should be noted that differences between –10°C and –15°C were noted in histology by our blinded pathologist, as described further below.
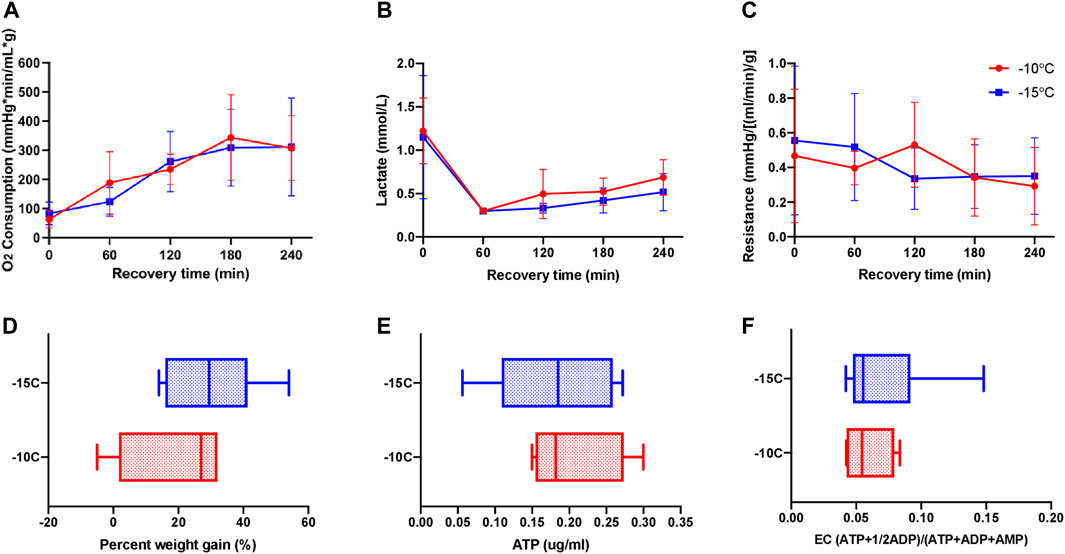
FIGURE 2. Perfusion metrics comparing partially frozen rat livers stored for 1 (n = 6) and 5 (n = 7) days at –10°C (n = 4) vs. –15°C (n = 9) with 12% glycerol and 4 h of recovery during SNMP revealed no functional differences between the groups. (A) Oxygen consumption, defined as outflow oxygen minus inflow oxygen, adjusted for flow rate and liver weight, (B) inflow lactate, (C) resistance, defined as the perfusion pressure in the PV divided by the flow rate and adjusted for liver weight after procurement, (D) percent weight gain, defined as percentage increase of liver weight at the end of recovery compared to liver weight after procurement, (E) ATP, (F) energy charge. Two-way ANOVA, followed by Tukey’s post hoc test for (A–C). Unpaired two-tailed t-test for (D–F). Boxes: median with interquartile range. Whiskers: min to max. Significance level: p < 0.05.
Comparison of partially frozen control rat livers to livers frozen with AFGP or X/Z-1000 ice modulators
There was no statistically significant difference between the three groups with respect to oxygen consumption based on two-way ANOVA/Tukey testing (Figure 3A). Control glycerol-only livers had the lowest mean perfusate lactate (1.17 ± 0.61 mmol/L) at time zero. Over the first hour of SNMP, there was a decrease in lactate among all three groups. There were no significant differences between the groups at any time point according to the two-way ANOVA/Tukey test (Figure 3B). X/Z-1000 frozen livers had significantly higher mean vascular resistance {1.44 ± 0.37 mmHg/[(ml/min)/g]} at 0 h compared to both 12% glycerol control livers {0.528 ± 0.39 mmHg/[(ml/min)/g], p-value 0.0315} and AFGP livers (0.413 ± 0.27, p-value 0.0215) (by ANOVA/Tukey). However, these differences were no longer significant at the remaining time points as the resistance levels converged over time (Figure 3C). Further, there was no statistical significant difference in cumulative bile production for all three group (Supplementary Figure S2).
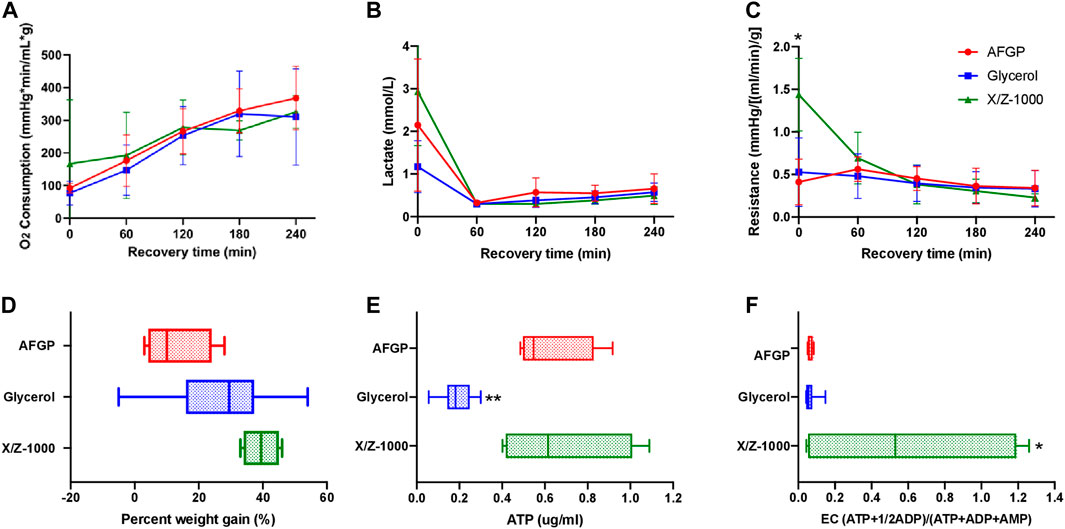
FIGURE 3. Perfusion metrics comparing rat livers partially frozen at –15°C with AFGP (n = 4) or with X/Z-1000 ice modulators (n = 4) versus 12% glycerol control (n = 13), with 4 h of recovery during SNMP. There was higher t = 0 resistance and energy charge in X/Z-1000 livers, and lower ATP levels in glycerol livers. (A) Oxygen consumption, defined as outflow oxygen minus inflow oxygen, adjusted for flow rate and liver weight, (B) portal vein lactate, (C) vascular resistance, defined as the perfusion pressure in the PV divided by the flow rate and adjusted for liver weight after procurement, (D) percent weight gain, defined as percentage increase of liver weight at the end of recovery compared to liver weight after procurement, (E) ATP, (F) energy charge. Stars denote statistical significance (two-way ANOVA, followed by Tukey’s post hoc test for (A–C), one-way ANOVA, followed by Tukey’s post hoc test for (D–F): *0.01 < p < 0.05; **0.001 < p < 0.01. Error bars represent standard deviation. Boxes: median with interquartile range. Whiskers: min to max.
Final mean weight gain for the glycerol-only control, the +AFGP, and the +X/Z-1000 groups were 26.9 ± 15.3%, 12.8 ± 13.2%, and 39.5 ± 5.69% respectively. Livers frozen with AFGP had the least edema, significantly less compared to X/Z-1000 livers (p-value 0.0294 by one-way ANOVA/Tukey; Figure 3D). Mean ATP for the glycerol control group, AFGP, and X/Z-1000 were 0.187 ± 0.075, 0.624 ± 0.20, and 0.680 ± 0.32 ug/ml respectively. ATP concentrations were significantly lower for livers stored with glycerol only compared with AFGP (p = 0.0057) and X/Z-1000 (p = 0.0023) (ANOVA/Tukey). There was no significant difference in ATP levels between AFGP and X/Z-1000 frozen livers (p = 0.91) (Figure 3E). Finally, mean EC for the glycerol control group, AFGP, and X/Z-1000 was 0.066 ± 0.032, 0.063 ± 0.015, and 0.591 ± 0.62 (ATP+1/2ADP)/(ATP + ADP + AMP) respectively. EC was higher in livers stored with X/Z-1000 and glycerol compared to glycerol alone (p = 0.0159) and to glycerol plus AFGP (p = 0.0428) (one-way ANOVA/Tukey). There was no significant difference in EC between AFGP and glycerol frozen livers (p = 0.99) (Figure 3F). Finally, there was no significant difference in perfusate potassium after 1 h of perfusion (used as a marker of cellular damage) between AFGP, X/Z-1000, and glycerol frozen livers (Supplementary Figure S1B).
H&E staining of rat liver parenchyma following both 1 and 5 days partial freezing showed variable sinusoidal, hepatocellular, and endothelial cell damage in all groups. In X/Z-1000 frozen livers, H&E showed better preservation of sinusoidal patency due to less hepatocyte cell swelling than seen in the other groups after 1 day of storage, which deteriorated somewhat after 5 days of storage. Endothelial patency also deteriorated between days 1 and 5, with hydropic changes and accumulation of intracytoplasmic vacuoles and focal endothelial cell destruction specially in the central venules (after 1 day: Figure 4A; after 5 days, Figure 4E). After 1 day of storage, glycogen staining was decreased in X/Z-1000 livers, but not in the AFGP or glycerol-only groups. AFGP frozen livers exhibited cellular swelling at the expense of sinusoidal patency and suffered from endothelial cell layer compression and disruption that was mild after 1 day (Figure 4B) and considerably worse after 5 days (Figure 4F) of storage. Livers frozen with only glycerol at –10°C had sinusoidal damage, endothelial cell loss, central vein endothelial cell disruption (Figure 4D), and massively swollen hepatocytes (Figure 4H). Decreasing the storage temperature of these control glycerol livers to –15°C worsened the parenchymal edema as noted by the H&E pathology after either 1 (Figure 4C) or 5 (Figure 4G) days of frozen storage.
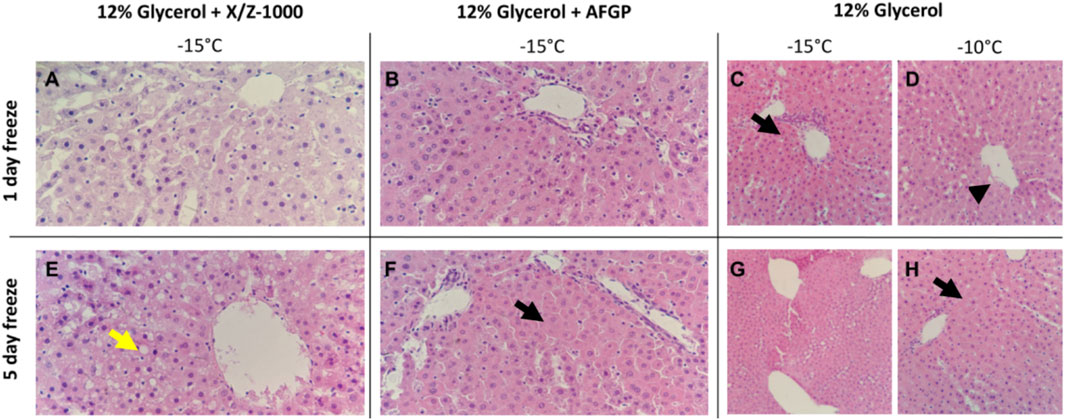
FIGURE 4. H&E staining of rat liver parenchyma after partial freezing for 1 day (A–D) and 5 days, (E–H) with X/Z-1000, AFGP ice modulators versus 12% glycerol only (40X). Yellow arrow indicates intracytoplasmic vacuoles. Black arrows indicate hepatocyte swelling and compressed sinusoids. Arrowhead indicates endothelial cell destruction.
TUNEL staining was observed in both the endothelium lining the portal veins as well as scattered in the sinusoidal endothelial cells (SEC) after 1 and 5 days of frozen storage in the presence of X/Z-1000 (Figures 5A,E). AFGP frozen livers had primarily portal vein endothelial staining with mild SEC staining after 1 day (Figure 5B), but after 5 days, TUNEL hepatocyte nuclear staining was noted together with the SEC (Figure 5F). Compared to the other groups, AFGP frozen livers at –15°C and 5 days of partial freezing had the most TUNEL staining. Glycerol only livers stored at –10°C had remarkably less TUNEL staining compared to X/Z-1000 and AFGP livers with only mild few scattered sinusoidal staining after 1 day (Figure 5D), and 5 days (Figure 5H) of storage. As in the H&E results, decreasing the storage temperature of glycerol only livers to –15°C did not exacerbate TUNEL staining after 1 day (Figure 5C) or 5 days (Figure 5G) of storage.
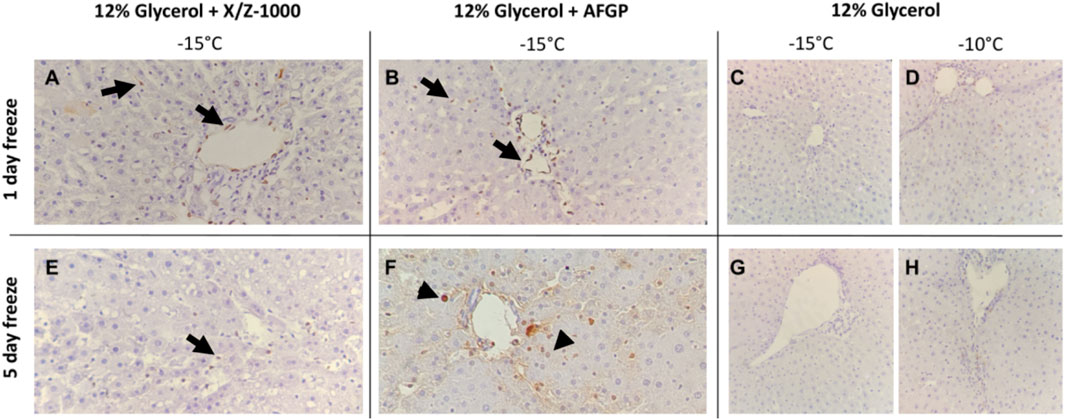
FIGURE 5. Terminal deoxynucleotidyl transferase dUTP nick end labeling (TUNEL) staining of rat liver parenchyma after partial freezing for 1 day (A–D) and 5 days (E–H) with X/Z-1000, AFGP ice modulators versus 12% glycerol only (40X). Black arrows indicate endothelial cell staining. Arrowheads indicate hepatocyte staining.
Discussion
Extending the preservation time of donor organs would be a tremendous asset for the field of transplantation and beyond. For liver transplantation, lengthening the allograft preservation time from the current standard of SCS at 4°C would reduce organ discard and the high costs of unplanned surgeries, decrease organ rejection rates by improving donor-recipient matches or enable immune tolerance induction protocols, and possibly even open avenues for global matching programs. Further, preserved whole human organs would be an invaluable resource for research, including for drug discovery and uncovering disease etiology [3, 31].
Prior cryogenic organ preservation efforts have typically (although not universally [32]) encountered either lethal [33, 34] or unacceptably damaging [29, 35–37] amounts of extracellular ice, or the challenge of introducing the enormous concentrations of CPA needed to preclude such damage [29, 38, 39]. So far, these difficult challenges have not been adequately overcome, and therefore, other approaches should be investigated and may produce practical results more rapidly. Our current approach utilizes high subzero (−4°C to −20°C) but not deep cryogenic temperatures to extend organ preservation time. In this capacity, we explored an approach called partial freezing, whereby we combined preservation at high subzero temperatures (–10°C and –15°C) with machine perfusion to maximize the benefits of metabolic rate depression and ex-vivo organ recovery/assessment [9]. While glycerol-treated, partially frozen livers that were stored for 5-fold longer than clinical controls showed some favorable outcomes, further studies are required to fully describe and address ice-mediated damage. Ice modulators have been shown to modify ice crystal shape and inhibit ice recrystallization, potentially decreasing ice-induced cellular damage. In the context of the partial freezing of rat livers, ice modulators may provide an additional layer of protection from these types of ice-related cellular damage.
This study first demonstrated that rat livers frozen at –10°C versus –15°C with glycerol were statistically similar regarding perfusion metrics (Figure 2). However, the reduction in storage temperature from –10°C to –15°C can have implications for organ viability on a cellular level. Indeed, previous efforts that used propylene glycol as the main permeating CPA of partially frozen livers [9], showed storage temperature can impact viability outcomes at the cellular level. As water freezes, solutes are excluded from the ice crystals, which increases the osmolality and reduces the freezing point of the unfrozen water fraction. Thus, a lower freezing temperature results in more ice and a higher level of osmotic shift [40]. In our case, 12% v/v glycerol (1.64 M), equates to 1.86 molality. According to the freezing point depression approximation, freezing point is lowered by about 1.86°C for every one osmolal increase in concentration. Adding the 0.3 osmolal contribution of the glycerol vehicle solution, the melting point of our storage media should be in the vicinity of –4°C. At –10°C, about 60% of the water in the solution will be converted to ice, and at –15°C, about 73% of the water will be frozen out, which is a significant increase. Although the membrane stabilizing saccharides, trehalose and raffinose [41] were employed, they do not enter cells and therefore do not nominally protect the inner membrane leaflet or reduce cell shrinkage induced by water extraction during freezing.
A major aim of this research was to assess the effect of two ice modulators, AFGP and X/Z-1000, on partially frozen rat livers compared to glycerol controls (Figure 3). As compared to previous efforts by Tessier et al. 2022 [9], the present study uses glycerol as the main permeating CPA and postulated that ice modulators may rescue glycerol-preserved livers from ice-mediated injury. AFGP plays a role in ice shaping and ice recrystallization inhibition, while X/Z-1000 inhibits ice recrystallization and influences ice nucleation events. AFGP frozen livers had the least amount of edema and high levels of ATP. However, the AFGP-mediated ice modulation had adverse effects on endothelial cells, which was reflected in both H&E and TUNEL staining, particularly after prolonged storage (Figures 4, 5). As the duration of freezing increased from 1 to 5 days, the AFGP TUNEL staining expanded from predominately endothelial damage to more prominent hepatocyte and sinusoidal cellular damage as well. AFGP has an established role in dynamic ice shaping, ice recrystallization inhibition, and hysteretic freezing point depression [17, 42, 43]. Since endothelial cells would make direct contact with the ice, it is possible that AFGP may be causing less favorable ice crystal shapes that are disrupting the endothelial cells of the liver. In a study by Rubinsky et al., antifreeze proteins resulted in the killing of all red blood cells during freezing despite the use of directional solidification methods that normally minimize ice damage [21, 44]. Yet, AFGP may offer protection to hepatocytes through its other mechanism of action, ice recrystallization inhibition. It should be noted that AFGP freezing point depression is typically limited to 1–2°C, which is smaller than the difference between our solutions’ TMs and our chosen storage temperatures and therefore was not able to contribute a protective effect in these experiments. Isothermal freeze fixation [34] could be useful in future studies for relating the details of ice distribution and characteristics to observed outcomes [45].
X/Z-1000 was the second ice modulator combination assessed in this study. X/Z-1000 frozen livers had the highest ATP and by far the highest EC but suffered from the highest SNMP resistance at t = 0 and had the most edema after recovery (Figure 3). On staining, X/Z-1000 frozen livers had less TUNEL staining compared to AFGP frozen livers, but still exhibited sinusoidal endothelial staining in excess of that seen for the glycerol only group (Figures 4, 5). X/Z-1000 livers had a large variation in both ATP and EC levels, which could potentially be explained by the competing mechanisms of action of X-1000/Z-1000 with the potent ice nucleator, Snomax [25]. Snomax would tend to reduce the number of ice crystals and, therefore, to increase their mean size and the range of grain sizes. This might relate to the larger observed size of the sinusoids and to stochastic differences in local nucleation and ice crystal size that affected the consistency of hepatocyte viability. While X/Z-1000 is appealing for its high ATP and EC, the high level of edema after partial freezing is concerning and might also be related to vascular damage caused by larger local intravascular or interstitial ice grains, which would be consistent with previous observations by Rubinsky et al. relating injury in frozen livers to vascular distension by intravascular ice [46]. A future direction related to this ice modulator should be to explore the use of X-1000, which is a recrystallization inhibitor, without the use of Z-1000, which is an antinucleator. On the other hand, total vascular distension should have been similar in all groups, as dictated by the phase diagram of glycerol-water solutions, and yet edema was more moderate in the glycerol-only group. In any case, X/Z-1000 seems promising for use with the isolation or preservation of isolated hepatocytes, for which maintenance of high ATP/EC would be the main goal.
Finally, glycerol-only control livers had the lowest lactate levels at t = 0 and minimal TUNEL staining (Figures 3–5). However, these livers also had very low ATP and EC compared to the ice modulator groups. A potential biological reason for this difference is that glycerol induces glycerol kinase to convert glycerol to glycerol-3-phosphate, which is an ATP dependent pathway. Thus, the activity of glycerol as a CPA could depress ATP levels [47]. Indeed, our other studies have confirmed replacing glycerol with other permeating CPAs, such as ethylene glycol or propylene glycol may be more promising [9]. However, the extraordinary and unexpected ability of both ice modulators to prevent low ATP has no clear explanation, but it seemed that utilization of glycogen to generate ATP and lactate was more effective in the X/Z-1000 group and to a lesser extent in the AFGP group, based on more intense glycogen staining (suggesting less glycogen metabolism) in the glycerol-only group. It would be interesting to see if structurally unrelated small molecule ice recrystallization inhibitors (IRIs) [48] would have a similar effect and also better protect the vascular system. Without the effects of the ice modulators preventing damaging ice recrystallization, glycerol-only livers also consistently had moderate parenchymal swelling and endothelial cell damage on H&E staining, despite adding Snomax and 3-OMG to rescue endothelial cells from partial freezing injury. Thus, the concept of adding ice modulation to the basic methodology for high temperature freezing appears to be well supported. Finally, all liver groups cleared lactate over the 2-h perfusion and (while incurring hepatocellular damage) had viable H&E histology after perfusion, meeting two criteria for transplantation [49, 50]. Thus, future experiments transplanting these partially frozen livers after SNMP can be conducted to assess if perfusion performance corelates with in vivo hepatic function.
Overall, the ideal ice modulator combination to enhance the partial freezing protocol would retain the positive effects of high ATP and high EC seen in X/Z-1000, the low levels of edema with AFGP, without the cellular damage to endothelial and sinusoidal cells seen with both ice modulator groups. Thus, future directions to expand the preservation of livers for transplantation with the partial freezing approach depend on both modifications to the freezing protocol as well as the ice modulator combination. Moreover, increasing the CPA concentration to decrease ice formation at lower temperatures and expanding on efforts to improve the loading and unloading of CPAs with machine perfusion, as is already being pursued [9], could improve liver viability. Also, in addition to glycerol, ethylene glycol, and propylene glycol tested herein and in Tessier et al [9]., there are other permeating CPAs that could be tested in the partial freezing protocol such as dimethyl sulfoxide, N-methylformamide, urea, or combinations thereof [38, 51, 52]. Finally, altering the base solution from UW to a lower potassium carrying solution could decrease the transmembrane osmotic stress in the unfrozen water fraction.
In summary, this research incorporated ice modulators into the rat liver partial freezing protocol to prolong the preservation time of livers. We demonstrated that AFGP and X/Z-1000 ice modulators can have beneficial effects on partially frozen rat liver ATP and EC levels, respectively. However, further work on elucidating the optimal ice modulator cocktail is necessary as AFGP livers had high levels of endothelial DNA damage and X/Z-1000 livers suffered from post-freeze edema. Modifications to CPA combinations, as well as improvements to machine perfusion CPA loading and unloading, can help improve the viability of these partially frozen organs.
Data availability statement
The original contributions presented in the study are included in the article/Supplementary Material, further inquiries can be directed to the corresponding authors.
Ethics statement
All research complies with all relevant ethical regulations and the experimental protocol was approved by the Institutional Animal Care and Use Committee (IACUC) of Massachusetts General Hospital (Boston, MA, United States).
Author contributions
ST, CP, SC, MiT, GF, MeT, and KU conceived and designed the study; ST, CP, and SC performed the experiments; ST, OH, EH, LW, HY, JM, GF, MeT, and KU analyzed and interpreted data; ST, CP, and OH performed statistical analysis; ST and OH wrote the manuscript; all authors participated in critical revision of the manuscript for intellectual content; all authors contributed to the preparation of the manuscript.
Funding
This research was funded from the United States National Institutes of Health (R01DK114506, R01DK096075, R01DK107875, and R21GM143659) and NSF ATP-Bio ERC grant (NSF 1941543). Further, we gratefully acknowledge funding to SNT from NIH (K99/R00 HL1431149; R01HL157803), American Heart Association (18CDA34110049), Harvard Medical School Eleanor and Miles Shore Fellowship, and the Claflin Distinguished Scholar Award on behalf of the MGH Executive Committee on Research. We gratefully acknowledge research support to OH by the American Liver Foundation (2019 Hans Popper Memorial Postdoctoral Research Fellowship) and the American College of Surgeons (Grant number 1123-39991 scholarship endowment fund).
Acknowledgments
We thank the MGH Cell Resource Core for assistance with perfusion equipment and the Mass Spectrometry Special Shared Facilities at Shriners Hospitals for Children (Boston) for quantification of tissue adenylate levels. Finally, we are grateful for our collaboration on high subzero organ preservation with Sylvatica Biotech, Inc.
Conflict of interest
Author MiT was employed by the company Sylvatica Biotech, Inc. and GF was employed by the company 21st Century Medicine. MGH-affiliated authors declare competing interests. MT, KU, ST, LW, SC, and CP have patent applications relevant to this study. KU has a financial interest in Organ Solutions, a company focused on developing organ preservation technology. MT, KU, and ST serve on the Scientific Advisory Board for Sylvatica Biotech Inc., a company focused on developing high subzero organ preservation technology. Competing interests for MGH investigators are managed by the MGH and Partners HealthCare in accordance with their conflict-of-interest policies.
The remaining authors declare that the research was conducted in the absence of any commercial or financial relationships that could be construed as a potential conflict of interest.
Publisher’s note
All claims expressed in this article are solely those of the authors and do not necessarily represent those of their affiliated organizations, or those of the publisher, the editors and the reviewers. Any product that may be evaluated in this article, or claim that may be made by its manufacturer, is not guaranteed or endorsed by the publisher.
Supplementary material
The Supplementary Material for this article can be found online at: https://www.frontiersin.org/articles/10.3389/fphy.2022.1033613/full#supplementary-material
Abbreviations
3-OMG, 3-O-methyl-d-glucose; ANOVA, analysis of variance; AFGP, antifreeze glycoprotein; BSA, bovine serum albumin; CPA, cryoprotective agent; EC, energy charge; H&E, hematoxylin and Eosin; HES, hydroxyethyl starch; HLA, human leukocyte antigen; HMP, hypothermic machine perfusion; INAs, ice nucleating agents; IRIs, ice recrystallization inhibitors; IVC, infrahepatic vena cava; IACUC, Institutional Animal Care and Use Committee; LT, liver transplant; M, molarity; PEG, polyethylene glycol; X/Z-1000, polyvinyl alcohol/polyglycerol; PV, Portal Vein; SCS, static cold storage; SEC, sinusoidal endothelial cells; SNMP, subnormothermic machine perfusion; TUNEL, terminal deoxynucleotidyl transferase dUTP nick end labeling; UW, University of Wisconsin; WE, Williams E.
References
1. Ray K. Preserving the liver for transplantation. Nat Rev Gastroenterol Hepatol (2018) 15(6):327. doi:10.1038/s41575-018-0024-7
2. Taylor MJ, Weegman B, Baicu S, Giwa S. New approaches to cryopreservation of cells, tissues, and organs. Transfus Med Hemother (2019) 46(3):197–215. doi:10.1159/000499453
3. Giwa S, Lewis JK, Alvarez L, Langer R, Roth AE, Church GM, et al. The promise of organ and tissue preservation to transform medicine. Nat Biotechnol (2017) 35(6):530–42. doi:10.1038/nbt.3889
4. Yamada K, Sykes M, Sachs DH. Tolerance in xenotransplantation. Curr Opin Organ Transpl (2017) 22(6):522–8. doi:10.1097/mot.0000000000000466
5. Berendsen TA, Bruinsma BG, Puts CF, Saeidi N, Usta OB, Uygun BE, et al. Supercooling enables long-term transplantation survival following 4 days of liver preservation. Nat Med (2014) 20(7):790–3. doi:10.1038/nm.3588
6. Bruinsma BG, Berendsen TA, Izamis ML, Yeh H, Yarmush ML, Uygun K. Supercooling preservation and transplantation of the rat liver. Nat Protoc (2015) 10(3):484–94. doi:10.1038/nprot.2015.011
7. de Vries RJ, Tessier SN, Banik PD, Nagpal S, Cronin SEJ, Ozer S, et al. Supercooling extends preservation time of human livers. Nat Biotechnol (2019) 37(10):1131–6. doi:10.1038/s41587-019-0223-y
8. de Vries RJ, Tessier SN, Banik PD, Nagpal S, Cronin SEJ, Ozer S, et al. Subzero non-frozen preservation of human livers in the supercooled state. Nat Protoc (2020) 15(6):2024–40. doi:10.1038/s41596-020-0319-3
9. Tessier SN, de Vries RJ, Pendexter CA, Cronin SEJ, Ozer S, Hafiz EOA, et al. Partial freezing of rat livers extends preservation time by 5-fold. Nat Commun (2022) 13(1):4008. doi:10.1038/s41467-022-31490-2
10. Que W, Hu X, Fujino M, Terayama H, Sakabe K, Fukunishi N, et al. Prolonged cold ischemia time in mouse heart transplantation using supercooling preservation. Transplantation (2020) 104(9):1879–89. doi:10.1097/tp.0000000000003089
11. Powell-Palm MJ, Charwat V, Charrez B, Siemons B, Healy KE, Rubinsky B. Isochoric supercooled preservation and revival of human cardiac microtissues. Commun Biol (2021) 4(1):1118. doi:10.1038/s42003-021-02650-9
12. Zachariassen KE, Kristiansen E. Ice nucleation and antinucleation in nature. Cryobiology (2000) 41(4):257–79. doi:10.1006/cryo.2000.2289
13. Tessier SN, Weng L, Moyo WD, Au SH, Wong KHK, Angpraseuth C, et al. Effect of ice nucleation and cryoprotectants during high subzero-preservation in endothelialized microchannels. ACS Biomater Sci Eng (2018) 4(8):3006–15. doi:10.1021/acsbiomaterials.8b00648
14. Ishine N, Rubinsky B, Lee CY. Transplantation of mammalian livers following freezing: Vascular damage and functional recovery. Cryobiology (2000) 40(1):84–9. doi:10.1006/cryo.1999.2225
15. Celik Y, Drori R, Pertaya-Braun N, Altan A, Barton T, Bar-Dolev M, et al. Microfluidic experiments reveal that antifreeze proteins bound to ice crystals suffice to prevent their growth. Proc Natl Acad Sci U S A (2013) 110(4):1309–14. doi:10.1073/pnas.1213603110
16. Kuiper MJ, Morton CJ, Abraham SE, Gray-Weale A. The biological function of an insect antifreeze protein simulated by molecular dynamics. Elife (2015) 4:e05142. doi:10.7554/elife.05142
17. Weng L, Stott SL, Toner M. Molecular dynamics at the interface between ice and poly(vinyl alcohol) and ice recrystallization inhibition. Langmuir (2018) 34(17):5116–23. doi:10.1021/acs.langmuir.7b03243
18. Naullage PM, Lupi L, Molinero V. Molecular recognition of ice by fully flexible molecules. J Phys Chem C (2017) 121(48):26949–57. doi:10.1021/acs.jpcc.7b10265
19. Weng L, Swei A, Toner M. Role of synthetic antifreeze agents in catalyzing ice nucleation. Cryobiology (2018) 84:91–4. doi:10.1016/j.cryobiol.2018.08.010
20. Eickhoff L, Dreischmeier K, Zipori A, Sirotinskaya V, Adar C, Reicher N, et al. Contrasting behavior of antifreeze proteins: Ice growth inhibitors and ice nucleation promoters. J Phys Chem Lett (2019) 10(5):966–72. doi:10.1021/acs.jpclett.8b03719
21. Ishiguro H, Rubinsky B. Mechanical interactions between ice crystals and red blood cells during directional solidification. Cryobiology (1994) 31(5):483–500. doi:10.1006/cryo.1994.1059
22. Wowk B, Leitl E, Rasch CM, Mesbah-Karimi N, Harris SB, Fahy GM. Vitrification enhancement by synthetic ice blocking agents. Cryobiology (2000) 40(3):228–36. doi:10.1006/cryo.2000.2243
23. Burkey AA, Riley CL, Wang LK, Hatridge TA, Lynd NA. Understanding poly(vinyl alcohol)-mediated ice recrystallization inhibition through ice adsorption measurement and pH effects. Biomacromolecules (2018) 19(1):248–55. doi:10.1021/acs.biomac.7b01502
24. Hasan M, Fayter AER, Gibson MI. Ice recrystallization inhibiting polymers enable glycerol-free cryopreservation of microorganisms. Biomacromolecules (2018) 19(8):3371–6. doi:10.1021/acs.biomac.8b00660
25. Wowk B, Fahy GM. Inhibition of bacterial ice nucleation by polyglycerol polymers. Cryobiology (2002) 44(1):14–23. doi:10.1016/s0011-2240(02)00008-1
26. Amir G, Horowitz L, Rubinsky B, Yousif BS, Lavee J, Smolinsky AK. Subzero nonfreezing cryopresevation of rat hearts using antifreeze protein I and antifreeze protein III. Cryobiology (2004) 48(3):273–82. doi:10.1016/j.cryobiol.2004.02.009
27. Amir G, Rubinsky B, Basheer SY, Horowitz L, Jonathan L, Feinberg MS, et al. Improved viability and reduced apoptosis in sub-zero 21-hour preservation of transplanted rat hearts using anti-freeze proteins. J Heart Lung Transpl (2005) 24(11):1915–29. doi:10.1016/j.healun.2004.11.003
28. Amir G, Rubinsky B, Horowitz L, Miller L, Leor J, Kassif Y, et al. Prolonged 24-hour subzero preservation of heterotopically transplanted rat hearts using antifreeze proteins derived from arctic fish. Ann Thorac Surg (2004) 77(5):1648–55. doi:10.1016/j.athoracsur.2003.04.004
29. Fahy GM, Wowk B, Pagotan R, Chang A, Phan J, Thomson B, et al. Physical and biological aspects of renal vitrification. Organogenesis (2009) 5(3):167–75. doi:10.4161/org.5.3.9974
30. Watson CJE, Jochmans I. From "gut feeling" to objectivity: Machine preservation of the liver as a tool to assess organ viability. Curr Transpl Rep (2018) 5(1):72–81. doi:10.1007/s40472-018-0178-9
31. de Vries RJ, Yarmush M, Uygun K. Systems engineering the organ preservation process for transplantation. Curr Opin Biotechnol (2019) 58:192–201. doi:10.1016/j.copbio.2019.05.015
32. Campbell BK, Hernandez-Medrano J, Onions V, Pincott-Allen C, Aljaser F, Fisher J, et al. Restoration of ovarian function and natural fertility following the cryopreservation and autotransplantation of whole adult sheep ovaries. Hum Reprod (2014) 29(8):1749–63. doi:10.1093/humrep/deu144
33. Pegg DE. The relevance of ice crystal formation for the cryopreservation of tissues and organs. Cryobiology (2020) 93:3–11. doi:10.1016/j.cryobiol.2020.01.005
34. Hunt CJ. Studies on cellular structure and ice location in frozen organs and tissues: The use of freeze-substitution and related techniques. Cryobiology (1984) 21(4):385–402. doi:10.1016/0011-2240(84)90077-4
35. Hamilton R, Holst HI, Lehr HB. Successful preservation of canine small intestine by freezing. J Surg Res (1973) 14(4):313–8. doi:10.1016/0022-4804(73)90033-4
36. Lin JH, Carroll PM. The effect of calcium and magnesium on frozen rat uteri, and the calcium content of uteri frozen by various procedures. Cryobiology (1968) 5(2):105–8. doi:10.1016/s0011-2240(68)80151-8
37. Taylor MJ, Pegg DE. The effect of ice formation on the function of smooth muscle tissue stored at −21 or −60 °C. Cryobiology (1983) 20(1):36–40. doi:10.1016/0011-2240(83)90057-3
38. Fahy GM, Wowk B, Wu J, Phan J, Rasch C, Chang A, et al. Cryopreservation of organs by vitrification: Perspectives and recent advances. Cryobiology (2004) 48(2):157–78. doi:10.1016/j.cryobiol.2004.02.002
39. Farrant J. Mechanism of cell damage during freezing and thawing and its prevention. Nature (1965) 205(4978):1284–7. doi:10.1038/2051284a0
40. Libbrecht KG. Physical dynamics of ice crystal growth. Annu Rev Mater Res (2017) 47(1):271–95. doi:10.1146/annurev-matsci-070616-124135
41. Van den Ende W. Multifunctional fructans and raffinose family oligosaccharides. Front Plant Sci (2013) 4:247. doi:10.3389/fpls.2013.00247
42. Urbańczyk M, Gora J, Latajka R, Sewald N. Antifreeze glycopeptides: From structure and activity studies to current approaches in chemical synthesis. Amino Acids (2017) 49(2):209–22. doi:10.1007/s00726-016-2368-z
43. Bang JK, Lee J, Murugan R, Lee S, Do H, Koh H, et al. Antifreeze peptides and glycopeptides, and their derivatives: Potential uses in biotechnology. Mar Drugs (2013) 11(6):2013–41. doi:10.3390/md11062013
44. Mugnano JA, Wang T, Layne JR, DeVries AL, Lee RE. Antifreeze glycoproteins promote intracellular freezing of rat cardiomyocytes at high subzero temperatures. Am J Physiology-Regulatory Integr Comp Physiol (1995) 269(2):R474–9. doi:10.1152/ajpregu.1995.269.2.r474
45. Hunt CJ, Taylor MJ, Pegg DE. Freeze-substitution and isothermal freeze-fixation studies to elucidate the pattern of ice formation in smooth muscle at 252 K (-21°C). J Microsc (1982) 125(2):177–86. doi:10.1111/j.1365-2818.1982.tb00335.x
46. Rubinsky B, Lee C, Bastacky J, Onik G. The process of freezing and the mechanism of damage during hepatic cryosurgery. Cryobiology (1990) 27(1):85–97. doi:10.1016/0011-2240(90)90055-9
47. Robinson J, Newsholme EA. Some properties of hepatic glycerol kinase and their relation to the control of glycerol utilization. Biochem J (1969) 112(4):455–64. doi:10.1042/bj1120455
48. Briard JG, Poisson JS, Turner TR, Capicciotti CJ, Acker JP, Ben RN. Small molecule ice recrystallization inhibitors mitigate red blood cell lysis during freezing, transient warming and thawing. Sci Rep (2016) 6:23619. doi:10.1038/srep23619
49. Tolboom H, Pouw R, Uygun K, Tanimura Y, Izamis ML, Berthiaume F, et al. A model for normothermic preservation of the rat liver. Tissue Eng (2007) 13(8):2143–51. doi:10.1089/ten.2007.0101
50. Nösser M, Gassner JMGV, Moosburner S, Wyrwal D, Claussen F, Hillebrandt KH, et al. Development of a rat liver machine perfusion system for normothermic and subnormothermic conditions. Tissue Eng A (2020) 26(1-2):57–65. doi:10.1089/ten.tea.2019.0152
51. Katenz E, Vondran FWR, Schwartlander R, Pless G, Gong X, Cheng X, et al. Cryopreservation of primary human hepatocytes: The benefit of trehalose as an additional cryoprotective agent. Liver Transpl (2007) 13(1):38–45. doi:10.1002/lt.20921
Keywords: antifreeze glycoprotein, polyvinyl alcohol, polyglycerol, cryopreservation, partial freezing, machine perfusion
Citation: Tessier SN, Haque O, Pendexter CA, Cronin SEJ, Hafiz EOA, Weng L, Yeh H, Markmann JF, Taylor MJ, Fahy GM, Toner M and Uygun K (2022) The role of antifreeze glycoprotein (AFGP) and polyvinyl alcohol/polyglycerol (X/Z-1000) as ice modulators during partial freezing of rat livers. Front. Phys. 10:1033613. doi: 10.3389/fphy.2022.1033613
Received: 31 August 2022; Accepted: 05 December 2022;
Published: 22 December 2022.
Edited by:
Rafael C. Bernardi, Auburn University, United StatesReviewed by:
Xingyuan Jiao, The First Affiliated Hospital of Sun Yat-sen University, ChinaGhazaleh Gharib, University of Southern Denmark, Denmark
Copyright © 2022 Tessier, Haque, Pendexter, Cronin, Hafiz, Weng, Yeh, Markmann, Taylor, Fahy, Toner and Uygun. This is an open-access article distributed under the terms of the Creative Commons Attribution License (CC BY). The use, distribution or reproduction in other forums is permitted, provided the original author(s) and the copyright owner(s) are credited and that the original publication in this journal is cited, in accordance with accepted academic practice. No use, distribution or reproduction is permitted which does not comply with these terms.
*Correspondence: Mehmet Toner, bWVobWV0X3RvbmVyQGhtcy5oYXJ2YXJkLmVkdQ==; Korkut Uygun, a3V5Z3VuQG1naC5oYXJ2YXJkLmVkdQ==