- Materials Science Division, Argonne National Laboratory, Lemont, IL, United States
The 2019 discovery of high temperature superconductivity in layered nickelate films, Nd1-xSrNiO2, has galvanized a community that has been studying nickelates for more than 30 years both as cuprate analogs and in their own right. On the surface, infinite layer nickelates, and their multilayer analogs, should be promising candidates based on our understanding of cuprates: square planar coordination and a parent d9 configuration that places a single hole in a dx2-y2 planar orbital makes nickelates seem poised for superconductivity. But creating crystals and films of sufficient quality of this d9 configuration in Ni1+ has proven to be a synthetic challenge, only recently overcome. These crystalline specimens are opening windows that shed new light on the cuprate-nickelate analogy and reveal nuances that leave the relationship between cuprates and nickelates very much an area open to debate. This Perspective gives a qualitative, phenomenological account of these newly discovered superconductors and multilayer members of the infinite layer nickelate family. The focus is on our current understanding of electronic and magnetic properties of these materials as well as some future opportunities, explored from the viewpoint of synthetic challenges and some suggested developments in materials discovery and growth to make further progress in this rejuvenated field.
Introduction
Like the soil, mind is fertilized while it lies fallow, until a new burst of bloom ensues [1]. These words were penned by John Dewey in his 1934 work on the philosophy of art, but they express a universal experience that is common across most walks of life, especially the sciences. The materials that inspire the collection of articles in this issue, low-dimensional rare earth nickelates, are a case in point. With a story spanning more than three decades, these compelling materials have been fellow travelers with the more famous copper oxide superconductors, yet have always remained in their shadow, a “poor cousin.” That is, until now.
With superconductivity discovered in epitaxial thin films of the “infinite-layer” d9 nickelate (Nd,Sr)NiO2 by Harold Hwang [2] and his Stanford collaborators in mid-2019 and more recently by Julia Mundy [3] of Harvard in the five-layer nickelate Nd6Ni5O12, a decades-long dream has been realized. Their discoveries and other exciting new findings in d9 layered nickelate materials rest on breakthroughs in thin film and single crystal growth that have allowed old ideas to take on a new life, and renewed enthusiasm to explore the nickelates as a platform to understand high-Tc superconductivity beyond cuprates, indeed a “new burst of bloom.” The purpose of this Perspective is to provide a phenomenological account of how this nickelate renaissance has emerged as viewed through the lens of new and transformative materials developments in d9 nickel oxides, the discoveries they have enabled, and some new directions toward which they may point.
Cuprates
To appreciate the long-lived fascination with nickelates and the importance of today’s resurgence of interest, a bit of stage-setting about cuprate superconductors is essential. Understanding the physics behind the copper oxides has been a defining challenge of condensed matter physics for the 35 years since their discovery. The hundreds of thousands of papers written about cuprates underscore simultaneously their impact on the condensed matter community, and the daunting challenge to untangling both the phenomenology and mechanism behind these unconventional superconductors. Generating this challenge is an elaborate web of interactions that result in the complex phase behavior of cuprates, behavior that contains—in addition to superconductivity—magnetism, charge order, non-Fermi-liquid metals, real-space stripes (both static and dynamic) pair density waves, and perhaps other yet-to-be-discovered exotica [4]. Unraveling how these emergent phases compete or cooperate, both in the normal and superconducting state, has driven the field from its inception to today.
Superconducting cuprates emerge by doping a parent antiferromagnetic insulating parent oxide containing Cu2+, exemplified by La2CuO4 (La-214). Doping introduces holes (i.e., La2-xSr2CuO4) or electrons (i.e., Nd2-xCexCuO4) to produce first a metal, and then a superconductor. Along with sophisticated quantitative models and mechanisms constructed to explain this progression of phases, there have emerged a set of phenomenological descriptors that are considered important “ingredients” in the “recipe” for high-Tc copper oxide superconductivity. These include:
• A quasi-2D structure in which the copper ions are surrounded by a highly axially-elongated octahedral coordination polyhedron of oxygen anions.
• An orbital configuration around Cu in which the dx2-y2 orbital is highest lying. With a nominal oxidation state of Cu2+, this d9 filling promotes a Jahn-Teller distortion that generates a configuration with a single hole in this half-filled dx2-y2 orbital.
• Strong correlations that precipitate an instability in the notionally metallic half-filled dx2-y2 band of the parent, leading to an insulating, magnetic ground state (so-called “checkerboard” antiferromagnetic order)
• The relatively narrow separation between Cu 3d and O 2p states that lends a considerable hybridization between these orbitals and leads to a significant O component to the hole wave function, placing cuprates solidly in the charge transfer insulator rather than the Mott regime of the Zaanen-Sawatztky-Allen framework [5].
A Cuprate Analog
These ingredients have driven materials and chemistry design rules for high-Tc superconductors in the search both for other cuprate families and for non-copper based analogs. Soon after the discovery of cuprate superconductivity, attention turned to known nickelates isostructural with La-214, specifically La2-xSrxNiO4 (LSNO). Although Ni is immediately to the left of Cu on the Periodic Table, and a similar solid-state chemistry is found, the parent phase contains Ni2+, d8, rather than d9. Such d8 systems in octahedral coordination are typically high spin with holes in both the dx2-y2 and dz2 orbitals. Additionally, the lower electronegativity of Ni vs Cu means that the separation between the O 2p states and the Ni d manifold is larger, reducing hybridization. With these cuprate ingredients missing, it is perhaps unsurprising that superconductivity has not been found in LSNO. Indeed, the system remains insulating unless doped heavily to x
d9 Nickelates: Materials and Chemistry
If Ni2+ isn’t right, why not Ni1+, which would share the d9 configuration of Cu2+? Indeed, Anisimov suggested that Ni1+ in the square planar configuration found in the infinite layer compound, if doped with low-spin Ni2+, could be a superconductor [13]. In counterpoint, Pickett argued that reduced hybridization and concomitant weighting of the 3d band at EF meant that despite the isoelectronic configuration, “Ni1+ is not Cu2+.” [14] From the perspective of solid-state inorganic chemistry, Ni prefers to be in the 2+ charge state, and oxides with Ni1+ are rare. In the present context, a family of layered d9 compounds has taken center stage (Figure 1A). This homologous series, Rn+1NinO2n+2 (R = La,Pr,Nd), first described by Lacorre [15], is characterized by interleaving blocks of n NiO2 layers separated by R2O2 fluorite blocks. It is noteworthy that the n = 1 member of the series is isostructural with the well-known T′ phase of the superconducting cuprates, (Nd,Ce)2CuO4. The remainder of this Perspective focuses on this class of d9 nickelates in both bulk and thin-film forms, their growth, properties, and impact on high Tc superconductivity.
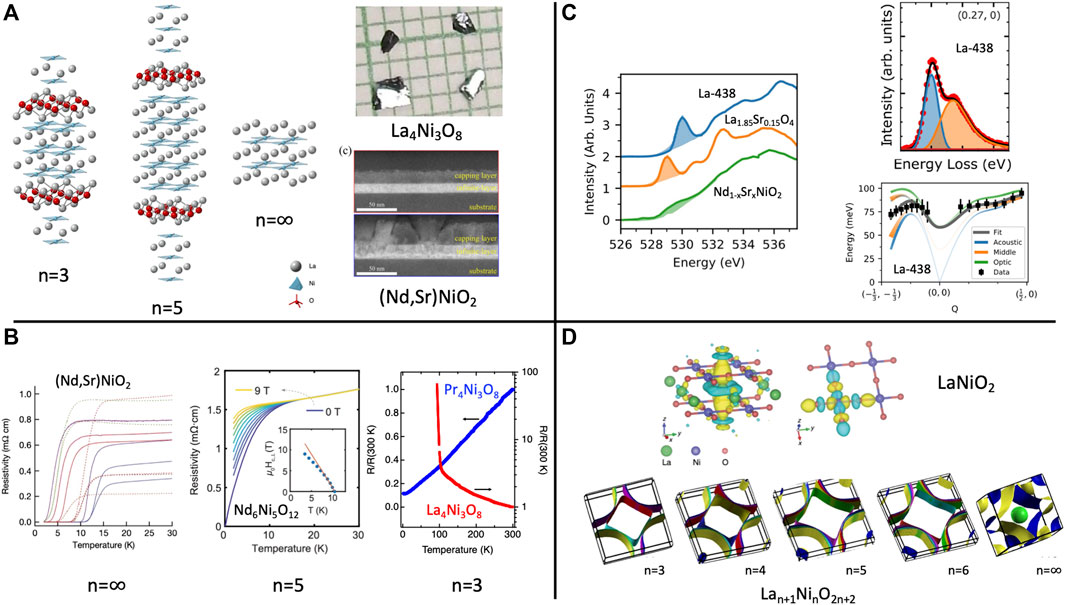
FIGURE 1. Overview of d9 nickelates structure and properties (A) left: members of the layered d9 nickelate family showing square planar NiO2 sheets separated by fluorite-like R2O2 layers; right top: single crystals of La4Ni3O8; right bottom: cross-sectional images of PLD-grown (Nd,Sr)NiO2 films [46]. (B) transport properties showing superconductivity in thin film infinite layer (Nd,Sr)NiO2 [2] and five-layer Nd6Ni5O12 [3] and competing ground states in single crystal bulk trilayer nickelates, metallic Pr4Ni3O8 and charge-stripe insulating La4Ni3O8. Note logarithmic scale for La-438. (C) Electronic structure of nickelates. left: prominent O K-edge prepeak in cuprates and trilayer La4Ni3O8 appears suppressed in (Nd,Sr)NiO2 films [49]. right: RIXS data reveal a magnon whose dispersion can be fit to yield the nearest-neighbor Ni-O superexchange constant [49]. (D) DFT calculations of electronic structure of d9 layered nickelates. top: Wannier orbitals calculated for LaNiO2 showing both dx2-y2 and dz2 configurations [51]; bottom: calculated Fermi surface vs. layer number for the Lan+1NinO2n+2 series [52].
Bulk Materials
The “infinite layer” nickelate LaNiO2 (n =
Hayward reported bulk synthesis of LNO and NdNiO2 (NNO) using NaH as a reducing agent [22, 23]. The synthesis led to mixed phases with excess O, stacking faults, etc. NNO synthesized in this way was insulating and paramagnetic, not the hoped-for antiferromagnetism found in cuprate parent phases. Hayward also successfully doped 10% Sr and Ca into LNO, but he reported no physical properties due to contamination by metallic Ni byproducts [24]. More recently, Li et al. [25] synthesized bulk Nd1-xSrxNiO2 (x = 0, 0.2, 0.4) using CaH2 to reduce the perovskite parent. The samples were insulating and paramagnetic. Contemporaneously, Wang et al. [26] made the x = 0.0, 0.1, 0.2 compositions and found no evidence for the mixed phase behavior found by Hayward. Samples were insulating, and neutron diffraction revealed the absence of long-range magnetic order above 3 K in the parent phase. Some theoretical considerations implicate H− insertion into the infinite layer nickelates during the reduction process [27, 28]. Such interstitial species could dramatically impact the electronic band structure; however, no direct experimental evidence for this interstitial has been reported in the superconducting films.
The multilayer analogs of “infinite layer” Rn+1NinO2n+2 (R = La,Pr,Nd) shown in Figure 1A are synthesized by reduction of the corresponding Ruddlesden-Popper phase, Rn+1NinO3n+1. In bulk materials, samples with La, Pr, and Nd have been synthesized with n ≤ 3 [29–31]. Unlike the infinite layer materials, these compounds can be synthesized at 300°C using dilute mixtures of H2 in inert gas, or using NaH in organic solvents at a more mild 150°C, as shown by Poltavets [30]. The ease of reduction can be traced to the mixed-valent nature of this family, which implies a fraction of Ni2+ for all n. Given the square planar coordination, it was conceivable that the divalent Ni could enter as low spin, satisfying the conditions placed by Anisimov for superconductivity [13].
Searching for cuprate analogs, particular attention was paid to the n = 3 material, La4Ni3O8 (La-438), which formally contains Ni1+ and Ni2+ in a 2:1 ratio. Work by Poltavets and Greenblatt [29] revealed a semiconductor to metal transition at T = 105 K, which also had a magnetic component. Speculation at the time was that either a CDW or SDW was the cause of this transition, and band structure calculations corroborated this q-space mechanism by showing a nested Fermi surface [29]. Other suggestions included charge order among the planes [32]—outer planes Ni1+ and inner plane Ni2+—or a spin-state transition mediated by inter-plane coupling through partially-occupied dz2 orbitals [33]. It was the successful growth of single crystals at Argonne National Laboratory, using a high oxygen pressure floating zone furnace followed by H2 reduction, that opened a new view on La-438: we showed unambiguously using x-ray and neutron diffraction that the transition results from real space charge- and spin-stripe formation, leading to an insulating ground state (Figure 1B, right), with a three-cell repeat set by the hole concentration: 1/3 hole doped into a Ni1+ background [34, 35]. The correspondence to the stripes found in hole-doped Ni2+ LSNO (including the diagonal propagation vector) became obvious in hindsight. DFT calculations show that the stripe formation is accompanied by a buckling of the outer Ni-O layers, a prediction verified by high-resolution diffraction measurements [36]. μSR studies by Bernal were found to be consistent with this stripe model [37].
We were also able to grow the Pr analog, Pr4Ni3O8 (Pr-438) [38]. As shown in Figure 1B (right), Pr-438 is metallic. Pr3+ is somewhat smaller than La3+, and this conceivably leads to a wider bandwidth than that of La-438, favoring the metallic ground state rather than the charge- and spin-stripes found for La-438. Notably, however, RIXS measurements on Pr-438 show the same broadened magnon dispersion as found in La-438 (see below) despite no long-range magnetic order, implying that stripe correlations are present here as well. As discussed below, Pr-438 possesses many of the ingredients for cuprate superconductivity. However, its hole concentration is slightly beyond the cuprate superconducting dome, and it remains normal. DFT calculations [39] and ARPES measurements [40] substantiate this picture, revealing an electronic structure like that of overdoped cuprates. Unfortunately, attempts to dope electrons into Pr-438 and shift the hole concentration into the superconducting dome have thus far proven unsuccessful.
Thin Films
Thin film synthesis of LNO was achieved by Kawai et al. [41, 42] using CaH2 reduction of a perovskite precursor film. It was found to be insulating. This result was subsequently confirmed by Kaneko et al. [43] and Ikeda et al. [44, 45] Regrettably, these groups do not seem to have pursued hole doping into the parent LNO or the effect of using other rare earths than La on the A site. It thus came as a remarkable surprise in August 2019, when the Stanford team led by Harold Hwang reported superconductivity at
Following on the direction suggested by the bulk trilayer nickelates, a strategy of growing multilayer thin-film nickelates has been pursued by Julia Mundy’s group at Harvard through another tour de force in materials growth [3]. While in infinite layer (Nd,Sr)NiO2 and the putative trilayer (Pr,Ce)4Ni3O8 the doping is provided by aliovalent substitution on the A site, it is also possible to self-dope the higher order members of the Rn+1NinO2n+2 series, as the formal Ni oxidation state follows (n+1)/n. Thus, by stacking more layers, the average Ni oxidation state will progressively move into the superconducting dome of the cuprates and the infinite-layer nickelates, entering perhaps at n = 4 and certainly by n = 5 (See Figure 2). This hypothesis proved to be correct, as shown in Figure 1B (middle). Here the resistivity of the five-layer material, Nd6Ni5O12 (nominal Ni oxidation state = 6/5), is shown as a function of temperature and field. A broad superconducting transition is found with Tc (H = 0) around 10 K, making this multilayer, self-doped material the second class of d9-derived nickelate superconductors and validating a unified a picture of nickelate and cuprate superconducting phase diagrams controlled by d electron count.
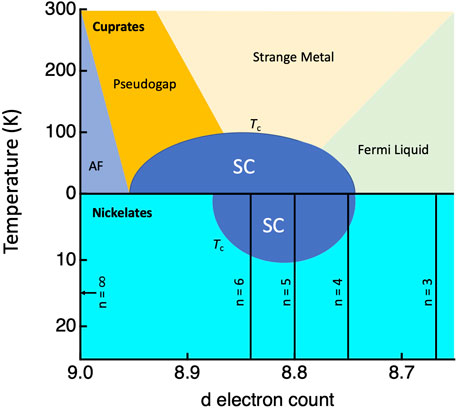
FIGURE 2. Schematic unified electronic phase diagram of d9 nickelates and cuprates as a function of d electron count. Inspired by Refs. [3, 4] and [38].
d9 Nickelates: Physics
We now turn from materials and chemistry to physics to overview the current thinking about the question of how nickelates compare and contrast with the cuprates. Inspired by the cuprate ingredients discussed above, we touch on magnetism and electronic structure and the role of hybridization on the hole wavefunction.
Magnetism
The cuprate parent phase is an antiferromagnetic insulator. As mentioned above, this does not seem to be the case for the infinite layer nickelates, which in bulk are paramagnetic [23, 25, 26]. Nonetheless, Ni L3-edge RIXS measurements on the superconducting films by Lu et al. [48] find a magnon spectrum consistent with the spin-1/2 antiferromagnetic square lattice found in cuprates. The nearest-neighbor exchange, J1 = 63 meV, is roughly half that found in copper oxide materials. Mark Dean’s group at Brookhaven National Lab has analyzed similar experiments on single crystals of trilayer La-438 (Figure 1C, right), finding a comparable value of J1 = 69 meV, although the nominal doping concentration is quite different [49]. A broadened magnon spectrum observed by this group is consistent with the spin stripes seen by neutrons. It is currently impossible to synthesize the equivalent hole concentration in the trilayer material in bulk or to reach the parent (e.g., Pr3CeNi3O8), but DFT calculations indicate the antiferromagnetic square lattice as the ground state of this putative parent phase [39].
Electronic Structure
DFT calculations show that the broad features of the infinite layer nickelate Fermi surface to be much like that of the copper oxides, except for the rare earth d-band hole pocket that self-dopes the former [14, 50]. The size of this hole pocket decreases with doping into the superconducting dome. The Wannier orbitals shown in Figure 1D (top) bear strong resemblance to the one-electron dx2-y2 and dz2 orbitals expected from simple crystal field considerations [51]. Looking at the multilayer systems [Figure 1 (bottom)], the number of bands in the dx2-y2 manifold increases with n, as expected [52].
Whether the nickelates lie in the Mott-Hubbard or charge transfer regime remains a significant controversy in the field. Since before even the earliest mentions of superconductivity in infinite layer nickelates, a Mott picture has been favored due to the larger 3d- O 2p separation [14]. Modern DFT calculations on the d9 nickelates support this view [53–56], which leads to less metal-ligand hybridization, and to the inference that Mott physics should be a better descriptor than a charge-transfer insulator model, distancing the d9 nickelates from cuprates. Experimental support for this picture comes from STEM-EELS measurements that find NdNiO2 lies in the Mott regime, and that with doping, holes appear on the Ni and Nd d bands as well as on O [53]. Many-body DMFT calculations have come down on both sides of this divide, but recent calculations seem to favor the Mott picture rather than charge-transfer, which is found for the isostructural cuprate analog CaCuO2 [56]. Experimentally, the prominent O 2p K-edge prepeak found in cuprates that signifies strong 3d- O 2p hybridization is greatly suppressed in the infinite layer materials, although less so in the case of the trilayer material (Figure 1C, left). Adding to the uncertainty, the Brookhaven group argues from O K-edge RIXS and cluster calculations that neither limit is appropriate for the trilayer La-438; rather, this nickelate is better described as having a mixed Mott-Hubbard/charge-transfer character with significant hole character on O, explaining the large superexchange mentioned above in this material [57]. The jury is still out on hybridization in the d9 nickelates, but it seems that oxygen states cannot be ignored in a proper description of these materials.
To date, the gold-standard for probing the low-energy physics of copper oxide superconductors, Angle-Resolved Photoemission Spectroscopy (ARPES), has not been applied to the thin film superconducting nickelates. It has, however, been measured on single crystals of the trilayer metal Pr-438 by the Dessau group. In unpublished data, they find a Fermi surface consistent with that of overdoped BSCCO and a large mass enhancement signaling strong correlations [40].
Finally, the crystal field is expected to strongly favor the low-spin configuration on Ni2+. This configuration has indeed been confirmed in the trilayer materials by x-ray absorption spectroscopy, which also shows a strong in plane orbital polarization [38]. Both findings stand as analogous to the cuprate electronic state.
Outlook
To conclude this Perspective, a discussion of open questions and potential directions to pursue is appropriate. Here are a few materials-inspired questions that could direct research in the near term.
Why aren’t Bulk d9 Nickelates Superconducting?
To date, no superconductivity has been found in bulk infinite layer phases at doping levels inside the superconducting dome of thin film specimens or in bulk multilayer systems (n = 2,3) that lie outside the dome. This does not mean it is impossible, so what might be the problem? It could be as simple as having the wrong lattice constant. The films are slightly compressively strained vs. freestanding bulk materials, and so perhaps pressure could tip the balance. Unfortunately, pressure studies on Nd0.8Sr0.2NiO2 to 50 GPa show no sign of metallic, let alone superconducting, behavior [25]. Concerning is the structural perfection [2, 46, 53] found in the ultra-thin films that is probably unlikely to be found in bulk materials. Oxygen defects may become disordered or be insufficiently ordered during the low-T reduction. The complex process that takes RNiO3 to RNiO2 passes through multiple known (and perhaps unknown) ordered O vacancy structures [19, 21, 58]. And while single crystals have been made by CaH2 reduction of Nd0.8Ca0.2NiO3 grown at high P, and they are more metallic than powder samples, there is a low-temperature localization transition and no superconductivity [59]. This may reflect a true Ca content in the crystals that is insufficient to reach the superconducting dome. Further work here is called for.
The message is that we simply may not have optimized materials growth of bulk samples to the same level of perfection as the films. Desperately needed is a direct synthesis of the d9 nickelate families that does not involve the perovskite reduction process. Such a method per force must be low temperature, as the infinite layer materials begin to disorder and to decompose at or below ∼300°C, depending on the rare earth. Such a creative breakthrough in synthesis and growth could be game-changing.
Can Trilayer Materials Be Made SC?
Based on simple d count as the key parameter, the trilayer materials are overdoped by a bit (Figure 2). Substituting on the Pr site of Pr-438 with a tetravalent ion like Ce4+ could in principle adjust the hole count into the dome. Despite extensive efforts, we have failed in this endeavor (A small amount of Ce4+ could be substituted into La-438, causing the metal-insulator transition to shift down from 105 K by 25 K). Other methods could include electric field gating or K-dosing (e.g., in an ARPES chamber) as done for cuprates and iridates [60, 61]. So far no one has succeeded at these strategies. A contrarian approach has been suggested by Dessau: while the focus has been on trying to increase the electron count to bring Pr-438 into the dome, another possibility is that the dz2 band takes up some holes that need to be in the dx2-y2 band, and that hole doping is really needed to tune into superconductivity. However, the recent report of superconductivity in n = 5 Nd6Ni5O12 films by Mundy et al. [3] (Figures 1B, 2) argues that the electron doping argument is appropriate, although this does not rule out other approaches. Even in the absence of superconductivity, the trilayer materials may hold other interesting stories. For instance, how does the stripe phase of La-438 evolve into the correlated metal Pr-438 at T = 0 K?
What Other Prospects May Be out There?
We have argued in this Perspective that materials synthesis and crystal growth advances have led the way in the rebirth of nickelates. Largely this has emerged as we access and gain better control over materials with Ni in extreme oxidation states spanning from +1 to +4. These synthesis advances have largely been applied to known materials. We should also look to the possibility of other materials — either known or unknown — in the quest for a more complete picture of nickelate superconductivity and the more general understanding of the physics of these highly correlated materials. Hybrid materials such as layered oxide-chalcogenides stand out as an interesting direction to pursue.
As demonstrated by the intense study of LSNO and related Ni2+ materials and the long history of the RNiO3 Ni3+ perovskites, the rare Earth nickelates as an extended family are more than just the d9 Ni1+ materials, and many open questions remain. What is the magnetic ground state of the rare Earth perovskites? Do dynamic fluctuations exist in the metallic phase of LaNiO3? How does dimensionality evolution from LSNO to LaNiO3 influence the transition from a real space charge stripe insulator to a metal? Many if not all such questions stand at a better place to be answered than they did even a few years ago because of renewed interest and capability in growing crystals under high pressure either by zone techniques or high fugacity solution growth in anvil cells or optimizing thin film growth. We are already seeing new and yet unresolved physics in these crystals: a broad maximum in magnetic susceptibility unknown in powders is universally found in LaNiO3 crystals grown independently by multiple groups around the world [62–64]. Evidence for a polar distortion in perovskite nickelates is growing [65]. A coupled CDW/SDW in the trilayer Ruddlesden-Popper phase La4Ni3O10 replaces the charge stripes in single layer La2-xSrxNiO4, hinting at an evolution between q-space and real space driven instabilities tuned by dimensionality [66].
The nickelate field had already established its longevity and importance to condensed matter science well before superconductivity was discovered in 2019, and the next few years should prove exciting as we learn more about superconducting and non-superconducting systems alike. As we master the synthesis and growth of a wider range of nickel oxides in extreme oxidation states, it is certain that new answers to questions both old and new will be found and that unexpected surprises are virtually guaranteed to emerge.
There is much work to be done. So, let the fields lay fallow, but not for too long.
Data Availability Statement
The original contributions presented in the study are included in the article/Supplementary Materials, further inquiries can be directed to the corresponding author.
Author Contributions
This Perspective was conceived and written by JFM.
Funding
This work was sponsored by the U.S. Department of Energy, Office of Science, Basic Energy Sciences, Materials Science and Engineering Division.
Conflict of Interest
The author declares that the research was conducted in the absence of any commercial or financial relationships that could be construed as a potential conflict of interest.
Publisher’s Note
All claims expressed in this article are solely those of the authors and do not necessarily represent those of their affiliated organizations, or those of the publisher, the editors, and the reviewers. Any product that may be evaluated in this article, or claim that may be made by its manufacturer, is not guaranteed or endorsed by the publisher.
Acknowledgments
The author thanks Mike Norman and Daniel Phelan for helpful discussions in the preparation of this manuscript.
Reference
2. Li D, Lee K, Wang BY, Osada M, Crossley S, Lee HR, et al. Superconductivity in an Infinite-Layer Nickelate. Nature (2019) 572:624–7. doi:10.1038/s41586-019-1496-5
3. Pan GA, Segedin DF, LaBollita H, Song Q, Nica EM, Goodge BH, et al. Superconductivity in a Quintuple-Layer Square-Planar Nickelate. Nat Mater (2021). doi:10.1038/s41563-021-01142-9
4. Keimer B, Kivelson SA, Norman MR, Uchida S, Zaanen J, Uchida S, et al. From Quantum Matter to High-Temperature Superconductivity in Copper Oxides. Nature (2015) 518:179–86. doi:10.1038/nature14165
5. Zaanen J, Sawatzky GA, Allen JW. Band Gaps and Electronic Structure of Transition-Metal Compounds. Phys Rev Lett (1985) 55:418–21. doi:10.1103/physrevlett.55.418
6. Shinomori S, Okimoto Y, Kawasaki M, Tokura Y. Insulator-Metal Transition in La2-xSrxNiO4. J Phys Soc Jpn (2002) 71:705–8. doi:10.1143/jpsj.71.705
7. Cheong S-W, Hwang HY, Chen CH, Batlogg B, Rupp LW, Carter SA. Charge-Ordered States in (La,Sr)2NiO4for Hole Concentrationsnh=1/3 and 1/2. Phys Rev B (1994) 49:7088–91. doi:10.1103/physrevb.49.7088
8. Katsufuji T, Tanabe T, Ishikawa T, Yamanouchi S, Tokura Y, Kakeshita T, et al. Commensurability Effect on the Charge Ordering ofLa2−xSrxNiO4. Phys Rev B (1999) 60:R5097–R5100. doi:10.1103/physrevb.60.r5097
9. Tranquada JM, Buttrey DJ, Sachan V. Incommensurate Stripe Order inLa2−xSrxNiO4withx=0.225. Phys Rev B (1996) 54:12318–23. doi:10.1103/physrevb.54.12318
10. Wochner P, Tranquada JM, Buttrey DJ, Sachan V. Neutron-Diffraction Study of Stripe Order inLa2NiO4+δwithδ=215. Phys Rev B (1998) 57:1066–78. doi:10.1103/physrevb.57.1066
11. Boothroyd AT, Prabhakaran D, Freeman PG, Lister SJS, Enderle M, Hiess A, et al. Spin Dynamics in Stripe-orderedLa5/3Sr1/3NiO4. Phys Rev B (2003) 67:100407. doi:10.1103/physrevb.67.100407
12. Yoshizawa H, Kakeshita T, Kajimoto R, Tanabe T, Katsufuji T, Tokura Y. Stripe Order at Low Temperatures inLa2−xSrxNiO4with0.289≲x≲0.5. Phys Rev B (2000) 61:R854–R857. doi:10.1103/physrevb.61.r854
13. Anisimov VI, Bukhvalov D, Rice TM. Electronic Structure of Possible Nickelate Analogs to the Cuprates. Phys Rev B (1999) 59:7901–6. doi:10.1103/physrevb.59.7901
14. Lee K-W, Pickett WE. Infinite-LayerLaNiO2: Ni1+is notCu2+. Phys Rev B (2004) 70:165109. doi:10.1103/physrevb.70.165109
15. Lacorre P. Passage from T-type to T′-type Arrangement by Reducing R4Ni3O10 to R4Ni3O8 (R = La, Pr, Nd). J Solid State Chem (1992) 97:495–500. doi:10.1016/0022-4596(92)90061-y
16. Crespin M, Isnard O, Dubois F, Choisnet J, Odier P. LaNiO2: Synthesis and Structural Characterization. J Solid State Chem (2005) 178:1326–34. doi:10.1016/j.jssc.2005.01.023
17. Crespin M, Levitz P, Gatineau L. Reduced Forms of LaNiO3perovskite. Part 1.-Evidence for New Phases: La2Ni2O5and LaNiO2. J Chem Soc Faraday Trans 2 (1983) 79:1181–94. doi:10.1039/f29837901181
18. Levitz P, Crespin M, Gatineau L. Reduced Forms of LaNiO3perovskite. Part 2.-X-ray Structure of LaNiO2and Extended X-ray Absorption fine Structure Study: Local Environment of Monovalent Nickel. J Chem Soc Faraday Trans 2 (1983) 79:1195–203. doi:10.1039/f29837901195
19. Moriga T, Usaka O, Nakabayashi I, Kinouchi T, Kikkawa S, Kanamaru F. Characterization of Oxygen-Deficient Phases Appearing in Reduction of the Perovskite-type LaNiO3 to La2Ni2O5. Solid State Ionics (1995) 79:252–5. doi:10.1016/0167-2738(95)00070-m
20. Moriga T, Usaka O, Nakabayashi I, Hirashima Y, Kohno T, Kikkawa S, et al. Reduction of the Perovskite-type LnNiO3 (Ln=Pr, Nd) to Ln3Ni3O7 with Monovalent Nickel Ions. Solid State Ionics (1994) 74:211–7. doi:10.1016/0167-2738(94)90212-7
21. Moriga T, Usaka O, Imamura T, Nakabayashi I, Matsubara I, Kinouchi T, et al. Synthesis, Crystal Structure, and Properties of Oxygen-Deficient Lanthanum Nickelate LaNiO3−x(0 ≤x≤ 0.5). Bcsj (1994) 67:687–93. doi:10.1246/bcsj.67.687
22. Hayward MA, Green MA, Rosseinsky MJ, Sloan J. Sodium Hydride as a Powerful Reducing Agent for Topotactic Oxide Deintercalation: Synthesis and Characterization of the Nickel(I) Oxide LaNiO2. J Am Chem Soc (1999) 121:8843–54. doi:10.1021/ja991573i
23. Hayward MA, Rosseinsky MJ. Synthesis of the Infinite Layer Ni(I) Phase NdNiO2+x by Low Temperature Reduction of NdNiO3 with Sodium Hydride. Solid State Sci (2003) 5:839–50. doi:10.1016/s1293-2558(03)00111-0
24. Hayward MAThe Synthesis and Characterisation of Some Novel Reduced Transition Metal Oxides. [PhD thesis]. Oxford, United Kingdom: University of Oxford (1999).
25. Li Q, He C, Si J, Zhu X, Zhang Y, Wen H-H. Absence of Superconductivity in Bulk Nd1−xSrxNiO2. Commun Mater (2020) 1:16. doi:10.1038/s43246-020-0018-1
26. Wang B-X, Zheng H, Krivyakina E, Chmaissem O, Lopes PP, Lynn JW, et al. Synthesis and Characterization of bulk Nd1-x Srx NiO2 and Nd1-x Srx NiO3. Phys Rev Mat (2020) 4:084409. doi:10.1103/physrevmaterials.4.084409
27. Si L, Xiao W, Kaufmann J, Tomczak JM, Lu Y, Zhong Z, et al. Topotactic Hydrogen in Nickelate Superconductors and Akin Infinite-Layer Oxides ABO2. Phys Rev Lett (2020) 124:166402. doi:10.1103/physrevlett.124.166402
29. Poltavets VV, Lokshin KA, Nevidomskyy AH, Croft M, Tyson TA, Hadermann J, et al. Bulk Magnetic Order in a Two-DimensionalNi1+/Ni2+(d9/d8) Nickelate, Isoelectronic with Superconducting Cuprates. Phys Rev Lett (2010) 104:206403. doi:10.1103/physrevlett.104.206403
30. Blakely CK, Bruno SR, Poltavets VV. Low-Temperature Solvothermal Approach to the Synthesis of La4Ni3O8 by Topotactic Oxygen Deintercalation. Inorg Chem (2011) 50:6696–6700. doi:10.1021/ic200677p
31. Sakurai Y, Chiba N, Kimishima Y, Uehara M. Electronic and Magnetic Properties of La4Ni3−xCuxO8 and Nd4−ySmyNi3O8. Physica C: Supercond (2013) 487:27–30. doi:10.1016/j.physc.2013.02.002
32. Wu H. Charge-spin-orbital States in the Tri-layered Nickelate La4Ni3O8: an Ab Initio Study. New J Phys (2013) 15:023038. doi:10.1088/1367-2630/15/2/023038
33. Pardo V, Pickett WE. Quantum Confinement Induced Molecular Correlated Insulating State inLa4Ni3O8. Phys Rev Lett (2010) 105:266402. doi:10.1103/physrevlett.105.266402
34. Zhang J, Chen Y-S, Phelan D, Zheng H, Norman MR, Mitchell JF. Stacked Charge Stripes in the quasi-2D Trilayer Nickelate La4Ni3O8. Proc Natl Acad Sci USA (2016) 113:8945–50. doi:10.1073/pnas.1606637113
35. Zhang J, Pajerowski DM, Botana AS, Zheng H, Harriger L, Rodriguez-Rivera J, et al. Spin Stripe Order in a Square Planar Trilayer Nickelate. Phys Rev Lett (2019) 122:247201. doi:10.1103/physrevlett.122.247201
36. Botana AS, Pardo V, Pickett WE, Norman MR. Charge Ordering in Ni1+/Ni2+ Nickelates: La4Ni3 O8 and La3Ni2 O6. Phys Rev B (2016) 94:081105. doi:10.1103/physrevb.94.081105
37. Bernal OO, MacLaughlin DE, Morris GD, Ho P-C, Shu L, Tan C, et al. Charge-stripe Order, Antiferromagnetism, and Spin Dynamics in the Cuprate-Analog Nickelate La4Ni3O8. Phys Rev B (2019) 100:125142. doi:10.1103/physrevb.100.125142
38. Zhang J, Botana AS, Freeland JW, Phelan D, Zheng H, Pardo V, et al. Large Orbital Polarization in a Metallic Square-Planar Nickelate. Nat Phys (2017) 13:864–9. doi:10.1038/nphys4149
39. Botana AS, Pardo V, Norman MR. Electron Doped Layered Nickelates: Spanning the Phase Diagram of the Cuprates. Phys Rev Mater (2017) 1:021801. doi:10.1103/physrevmaterials.1.021801
41. Kawai M, Inoue S, Mizumaki M, Kawamura N, Ichikawa N, Shimakawa Y. Reversible Changes of Epitaxial Thin Films from Perovskite LaNiO3 to Infinite-Layer Structure LaNiO2. Appl Phys Lett (2009) 94:082102. doi:10.1063/1.3078276
42. Kawai M, Matsumoto K, Ichikawa N, Mizumaki M, Sakata O, Kawamura N, et al. Orientation Change of an Infinite-Layer Structure LaNiO2 Epitaxial Thin Film by Annealing with CaH2. Cryst Growth Des (2010) 10:2044–6. doi:10.1021/cg100178y
43. Kaneko D, Yamagishi K, Tsukada A, Manabe T, Naito M. Synthesis of Infinite-Layer LaNiO2 Films by Metal Organic Decomposition. Physica C: Supercond (2009) 469:936–9. doi:10.1016/j.physc.2009.05.104
44. Ikeda A, Krockenberger Y, Irie H, Naito M, Yamamoto H. Direct Observation of Infinite NiO2planes in LaNiO2films. Appl Phys Express (2016) 9:061101. doi:10.7567/apex.9.061101
45. Ikeda A, Manabe T, Naito M. Comparison of Reduction Agents in the Synthesis of Infinite-Layer LaNiO2 Films. Physica C: Superconductivity its Appl (2014) 506:83–6. doi:10.1016/j.physc.2014.09.002
46. Lee K, Goodge BH, Li D, Osada M, Wang BY, Cui Y, et al. Aspects of the Synthesis of Thin Film Superconducting Infinite-Layer Nickelates. APL Mater (2020) 8:041107. doi:10.1063/5.0005103
47. Lin H, Gawryluk DJ, Klein YM, Huangfu S, Pomjakushina E, Rohr FV, et al. ,arXiv:2104.14324 (2021).
48. Lu H, Rossi M, Nag A, Osada M, Li DF, Lee K, et al. Magnetic Excitations in Infinite-Layer Nickelates. Science (2021) 373:213–6. doi:10.1126/science.abd7726
49. Lin JQ, Arribi PV, Fabbris G, Botana AS, Meyers D, Miao H, et al. Strong Superexchange in a d^{9-δ} Nickelate Revealed by Resonant Inelastic X-Ray Scattering. Phys Rev Lett (2021) 126:087001. doi:10.1103/physrevlett.126.087001
50. Choi M-Y, Lee K-W, Pickett WE. Role of 4 F States in Infinite-Layer NdNiO2. Phys Rev B (2020) 101:020503. doi:10.1103/physrevb.101.020503
51. Hepting M, Li D, Jia CJ, Lu H, Paris E, Tseng Y, et al. Electronic Structure of the Parent Compound of Superconducting Infinite-Layer Nickelates. Nat Mater (2020) 19:381–5. doi:10.1038/s41563-019-0585-z
52. LaBollita H, Botana AS. Tuning the Van Hove Singularities inAV3Sb5(A=K,Rb,Cs)via Pressure and Doping. Phys Rev B (2021) 104:035148. doi:10.1103/physrevb.104.205129
53. Goodge BH, Li D, Lee K, Osada M, Wang BY, Sawatzky GA, et al. Doping Evolution of the Mott-Hubbard Landscape in Infinite-Layer Nickelates. Proc Natl Acad Sci USA (2021) 118:e2007683118. doi:10.1073/pnas.2007683118
54. Hepting M, Green RJ, Zhong Z, Bluschke M, Suyolcu YE, Macke S, et al. Complex Magnetic Order in Nickelate Slabs. Nat Phys (2019) 14 (11):1097–1102. doi:10.1038/s41567-018-0218-5
55. Jiang M, Berciu M, Sawatzky GA. Critical Nature of the Ni Spin State in Doped NdNiO2. Phys Rev Lett (2020) 124:207004. doi:10.1103/physrevlett.124.207004
56. Karp J, Hampel A, Zingl M, Botana AS, Park H, Norman MR, et al. Comparative many-body Study of Pr4Ni3O8 and NdNiO2. Phys Rev B (2020) 102:245130. doi:10.1103/physrevb.102.245130
58. Wang B-X, Rosenkranz S, Rui X, Zhang J, Ye F, Zheng H, et al. Antiferromagnetic Defect Structure in LaNi O3–δ Single Crystals. Phys Rev Mat (2018) 2:064404. doi:10.1103/physrevmaterials.2.064404
59. Puphal P, Wu YM, Fürsich K, Lee H, Pakdaman M, Bruin J, et al. Topotactic Transformation of Single Crystals: From Perovskite to Infinite-Layer Nickelates. Sci Adv (2021). 7:eabl8091.
60. Hossain MA, Mottershead JDF, Fournier D, Bostwick A, McChesney JL, Rotenberg E, et al. In Situ doping Control of the Surface of High-Temperature Superconductors. Nat Phys (2008) 4:527–31. doi:10.1038/nphys998
61. Kim YK, Sung NH, Denlinger JD, Kim BJ. Observation of a D-Wave gap in Electron-Doped Sr2IrO4. Nat Phys (2016) 12:37–41. doi:10.1038/nphys3503
62. Dey K, Hergett W, Telang P, Abdel-Hafiez MM, Klingeler R. Magnetic Properties of High-Pressure Optical Floating-Zone Grown LaNiO3 Single Crystals. J Cryst Growth (2019) 524:125157. doi:10.1016/j.jcrysgro.2019.125157
63. Guo H, Li ZW, Zhao L, Hu Z, Chang CF, Kuo CY, et al. Antiferromagnetic Correlations in the Metallic Strongly Correlated Transition Metal Oxide LaNiO3. Nat Commun (2017) 9:43. doi:10.1038/s41467-017-02524-x
64. Zhang J, Zheng H, Ren Y, Mitchell JF. High-Pressure Floating-Zone Growth of Perovskite Nickelate LaNiO3 Single Crystals. Cryst Growth Des (2017) 17:2730–5. doi:10.1021/acs.cgd.7b00205
65. Ardizzone I, Teyssier J, Crassee I, Kuzmenko AB, Mazzone DG, Gawryluk DJ, et al. Raman Spectroscopic Evidence for Multiferroicity in Rare Earth Nickelate Single Crystals. Phys Rev Res (2021) 3:033007. doi:10.1103/physrevresearch.3.033007
Keywords: superconductivity, high‐Tc superconductors, crystal growth, thin film, metal insulator transition, stripes
Citation: Mitchell JF (2021) A Nickelate Renaissance. Front. Phys. 9:813483. doi: 10.3389/fphy.2021.813483
Received: 11 November 2021; Accepted: 24 November 2021;
Published: 23 December 2021.
Edited by:
Matthias Hepting, Max Planck Institute for Solid State Research, GermanyReviewed by:
Massimiliano Polichetti, University of Salerno, ItalyCopyright © 2021 Mitchell. This is an open-access article distributed under the terms of the Creative Commons Attribution License (CC BY). The use, distribution or reproduction in other forums is permitted, provided the original author(s) and the copyright owner(s) are credited and that the original publication in this journal is cited, in accordance with accepted academic practice. No use, distribution or reproduction is permitted which does not comply with these terms.
*Correspondence: J. F. Mitchell, bWl0Y2hlbGxAYW5sLmdvdg==