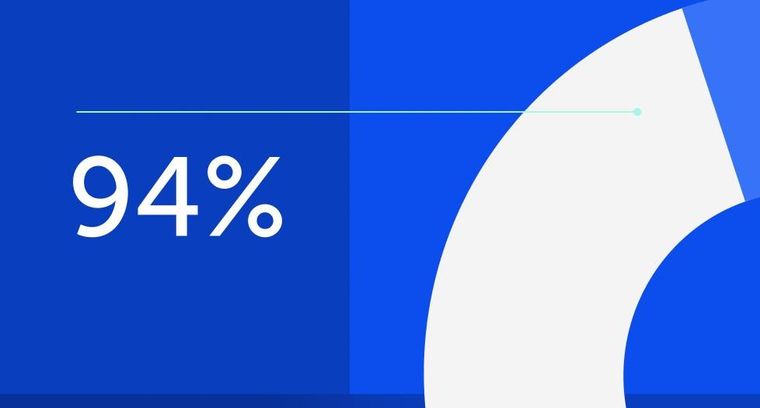
94% of researchers rate our articles as excellent or good
Learn more about the work of our research integrity team to safeguard the quality of each article we publish.
Find out more
REVIEW article
Front. Phys., 21 December 2021
Sec. Low-Temperature Plasma Physics
Volume 9 - 2021 | https://doi.org/10.3389/fphy.2021.786381
This article is part of the Research TopicProspects of Plasma Generated Species Interaction With Organic and Inorganic MaterialsView all 6 articles
Despite the high overall survival rates of severely injured military and civilian personnel requiring trauma and acute care, the challenges of treating infections and healing-resistant wounds have grown. Exposure to unknown environmental pathogens at the wound, including parasites and antibiotic resistant microorganisms, hinders timely and effective treatment using traditional techniques. Cold atmospheric pressure plasma (CAPP) provides a promising biophysical tool to address these issues by applying physically created modalities that cannot be circumvented by bioresistance to inactivate microorganisms and enhance wound healing. CAPPs generate charged particles and numerous reactive oxygen species (ROS) and reactive nitrogen species (RNS) that act on biological cells and tissues, often through plasma membrane interactions. This review fills a gap in the plasma medicine literature by specifically focusing on applying CAPPs for acute trauma, such as surgery, wound treatment, and disinfection. After briefly highlighting the areas of opportunity for improving acute trauma treatment and the fundamentals of CAPP generation, this review details emerging applications of CAPPs for enhanced wound healing, burn treatment, transdermal delivery, and surgical applications. We also discuss CAPP optimization through novel device design and synergistic combination with traditional treatment technologies to transition this biophysical technology to the battlefield and acute care settings.
Although the United States has achieved survival rates as high as 98% for casualties arriving alive at combat hospitals [1], up to 25% of battlefield deaths are potentially preventable [2, 3]. One remaining area of improvement involves focusing on the actions or inactions before a casualty reaches the combat hospital [1], which may be addressed by providing training to first responders that is comparable to physicians or nurses in fixed military hospitals during peacetime. They also do not have the resources at their disposal that would exist at the combat hospital or forward surgical team to adequately treat wounds and injuries that may ultimately result in deaths. Moreover, potential challenges with assuring air dominance to facilitate transport in future efforts make the ability to treat wounds in theater even more critical [4]. Also, in a future Pacific theater conflict, injured personnel may be exposed to various parasites that may require completely disparate treatments, motivating a single treatment method that may be efficient across a wide range of parasites.
One critical issue when ensuring the safety of injured personnel is treating wounds, both to enhance healing and prevent infection. Trauma to extremities accounted for >50% of injuries in Iraq and Afghanistan with infectious complications reported in >25% of those evacuated for trauma; osteomyelitis and deep-wound infections were reported in 9 and 27% of type III open-tibia fractures, respectively [5]. For instance, over 10 years of combat in Iraq and Afghanistan, 22 of 97 Gustilo–Anderson III tibial fractures documented in the United Kingdom were complicated by infection, with S. aureus the causative agent in 59% [6]. While the overall severity of injuries was not associated with infection, the degree of bone loss from the fracture was [6]. Acinetobacter baumannii-calcoaceticus was common in casualties injured in Iraq but was replaced by other extended spectrum beta-lactamase-producing Enterobacteriaceae and fungi in casualties from Afghanistan. Short courses of narrow-spectrum prophylactic antimicrobials and infection control were often used to control infection; debridement and irrigation were also often used [7]. Treatments for infection included surgery and antimicrobial therapy directed against expected and recovered pathogens [7].
Studied for the past two to three decades for their potential novel application for medical applications, particularly wound treatment and decontamination, cold atmospheric pressure plasmas (CAPPs) are becoming increasingly closer to clinical applications for emergency workers and the warfighter. A plasma is formed when a gas is exposed to a sufficiently strong applied voltage, or electric field, to cause electrical breakdown, or discharge formation [8]. During this discharge formation, CAPPs form reactive oxygen species (ROS), reactive nitrogen species (RNS), reactive oxygen nitrogen species (RONS), and charged particles that can influence biological systems [9]. The interaction of these CAPP-produced reactive species and charged particles with biological cells provides unique capabilities for inducing beneficial biophysical responses. For microorganisms, these reactive species may damage the membranes, resulting in loss of viability. In other cases, these particles may stimulate beneficial behavior, such as wound healing and cell growth.
A recent Perspective paper [10] provided a comprehensive update of the application of CAPPs in medicine. Our review does not attempt to provide such a broad overview of “plasma medicine,” but instead focuses on recent developments in medical technologies relevant to treating traumatic injuries and wounds or reducing bacterial loads for surgical applications. As such, we will briefly highlight the fundamental physics behind CAPP-based medical applications but refer the interested reader to more comprehensive reviews on medical and biological applications of CAPPs [9–29]. We note that other recent reviews have focused specifically on CAPP wound healing [12, 24, 26], dermatological applications [13, 15], and enhancing surgical implants [20]. The current review broadly examines recent studies for CAPP-based medical applications to highlight potential applications relevant to wound treatment, debridement, and medical procedures of relevance to first responders and surgeons, as well as to combat applications that may require rapid, flexible treatments.
This overview briefly focuses on the most critical aspects of CAPP delivery and mechanisms and supplements other more detailed reviews of the physics, chemistry, and application of CAPPs for medical applications [10–30]. As shown in Figure 1, CAPP delivery for biomedical application is divided into two categories: direct and indirect [11].
FIGURE 1. Example methods for delivering cold atmospheric pressure plasmas for biomedical applications by (A) direct application using a dielectric barrier discharge in argon driven by repetitive voltage pulses with nanosecond to microsecond duration or (B) indirect application using a micro-jet with helium as the operating gas to produce a cold plasma plume approximately 2.5 cm in length [11]. Laroussi M. Cold plasma in medicine and healthcare: the new frontier in low temperature plasma applications. Frontiers in Physics. 2020 Mar 20;8:74.; licensed under a Creative Commons Attribution (CC BY) 4.0 International license.
Direct treatment commonly uses a dielectric barrier discharge (DBD), as shown in Figure 1A. One may consider the DBD as two parallel plates with at least one plate covered with a dielectric material, such as glass or alumina. Biomedical applications often use the target, either the body for in vivo applications or a Petri dish for in vitro applications, as the ground electrode [30]. Original studies drove DBDs using sinusoidal voltages in the kHz range; however, subsequent studies found that nanosecond to microsecond pulses more efficiently enhanced discharge chemistry [11] and are now preferred for biomedical applications [31].
Indirect treatment separates the electrical breakdown event from the targeted specimen. Instead, the voltage, which may be pulsed or RF, is applied inside a holder containing flowing gas to cause breakdown, leading to reactive species and charged particle formation. This creates a plasma plume, as shown in Figure 1B, that may be targeted toward the specimen. Because the resulting plume may be very fine, they are commonly referred to as plasma jets, plasma pens, and plasma pencils. Plasma jets are valuable for applications requiring very targeted treatment, such as dental applications. Moreover, separating the high voltage from the specimen eliminates the risk of electrical shock or damage to target; however, a strong electric field is formed at the plume that can impact plasma plume propagation and the target [11].
One of the most commonly used indirect treatment systems is the kINPen. The kINPen plume is approximately 1 cm long with an operating frequency typically on the order of 1 MHz and a plasma dissipated power of approximately 1 MW. Tables 1–6 provide specific parameters for the experiments summarized later in this manuscript, including those using various versions of the kINPen. One of the critical features of any CAPP device is reactive species generation. Figure 2 shows a sample spectrum from a kINPen Sci operated in helium with nitrogen shielding gas [17] with data from [32].
TABLE 1. Summary of cold atmospheric pressure plasma (CAPP) applications for wound treatment, including plasma parameters, device setup, and key observation from each study.
TABLE 2. Summary of cold atmospheric pressure plasma (CAPP) applications for burn treatment, including plasma parameters, device setup, and key observation from each study.
TABLE 3. Summary of cold atmospheric pressure plasma (CAPP) applications for transdermal delivery, including plasma parameters, device setup, and key observation from each study.
TABLE 4. Summary of synergistic treatments using cold atmospheric pressure plasma (CAPP) and other modalities, including plasma parameters, device setup, and key observation from each study.
TABLE 5. Summary of surgical and internal applications of cold atmospheric pressure plasma (CAPP), including plasma parameters, device setup, and key observation from each study.
TABLE 6. Summary of system optimization of cold atmospheric pressure plasma (CAPP) systems, including plasma parameters, device setup, and key observation from each study.
FIGURE 2. A sample spectrum from a kINPen Sci operated in helium with nitrogen shielding gas [17] Reuter S, Von Woedtke T, Weltmann KD. The kINPen—a review on physics and chemistry of the atmospheric pressure plasma jet and its applications (2018) J Phys D: Appl Phys 51(23):233001. https://doi.org/10.1088/1361-6463/aab3ad; licensed under a Creative Commons Attribution (CC BY) 3.0 International license. The data is from Ref. [32].
Wound healing has been a key motivation for the medical applications of CAPPs. Table 1 summarizes recent studies examining CAPP applications for stimulating wound healing.
A recent study [33] demonstrated improved wound healing using a helium plasma jet based on a 6 kV pulse with a 1–2
Another study [34] examined the efficacy of treating wounds on Sprague-Dawley rats for 1 min using a helium plasma jet generated by a 17 kHz waveform with 1.33 kVrms, 10.7 mArms. The CAPP significantly decreased the wound area compared to the untreated control, increased wound contraction and re-epithelialization, and markedly increased the protein level of smooth muscle actin. The helium plasma jet did not alter collagen, which plays an important role in wound remodeling; however, it significantly increased the levels of TNF-
Another study examined the effectiveness of using a helium plasma jet with a 25 kHz, 5 kV waveform to accelerate pressure wound healing in a rat model [35], which is relevant to patients with spinal cord injuries or hospitalized patients undergoing orthopedic surgeries. The CAPP treated group exhibited accelerated pressure ulcer wound re-epithelialization, angiogenesis, and fibrosis compared to the untreated group. It also reduced the duration of the inflammation phase, while significantly accelerating wound healing and contraction. CAPP significantly decreased the wound area and increased wound closure rate compared to the untreated control at 7, 14, and 21 days following wounding. Further, the inflammation for the plasma treated wounds was significantly lower than the control for day 7 or later.
Arrays of plasma jets may be used to treat larger areas [36, 37]. One experiment assessed wound healing for 12 adult male Sprague-Dawley rates exposed to 20–40 s of daily plasma treatment from an array of helium plasma jets with typical waveforms of 20 kHz at 190–374 Vrms [36]. Seven days after wound formation, the wound area of the untreated control was 24 ± 5% of its initial value, while an identical wound treated twice daily for 20 s was 9 ± 2% of its initial area. The CAPP-treated wounds also exhibited uniform healing, which was attributed to the array encompassing a larger subsection of the wound than a single jet, increasing the production of long-lived excited species that have time to cover the full area. This device efficiently inactivated fungi (Candida albicans) and bacteria (Escherichia coli) both in vitro and in vivo [37]. Application to second-degree burns in rat models showed that CAPP induced lower levels of interleukin (IL)-1a and -6 expression than in the non-treated group and a higher level of IL-10, which is an anti-inflammatory cytokine. As observed in the earlier study [36], the size of the CAPP treated wounds was statistically smaller than the untreated wounds [37]. CAPP promoted denser and more uniform collagen arrangement than in untreated cases, upregulated anti-inflammatory genes, and decreased expression of inflammatory cytokines; this combined to promote re-epithelialization [37].
Applying 2 min daily treatments using an array of sixteen helium plasma jets held 1 mm from a lesion with an applied voltage of 1 kVpp at 5 MHz to six square wounds on the back of each of 6 sheep yielded complete wound healing [38]. Over a 5-week period, the untreated wounds exhibited a variable bacterial load with up to almost 1 × 106 CFU/ml, while CAPP dramatically reduced (significantly for 3 weeks after treatment and beyond) bacterial load in the wounds to 2.5 × 105 CFU/ml. The CAPP induced the Ki67 antigen, a protein typically considered as a proliferation marker, which confirmed in vivo stimulation of cell proliferation that accelerates wound closure, in agreement with other studies demonstrating CAPP-induced stimulation of fibroblast proliferation. CAPP also promoted vascularization 2 weeks after lesion induction; this most likely occurred due to CAPP generated ROS since moderate oxidative stress can enhance angiogenesis [39].
These examples clearly show the feasibility of CAPP for enhancing wound healing in a battlefield or field hospital environment. One could envision treating wounds with a CAPP immediately at the point of trauma to facilitate wound care, particularly in cases where extraction to a field hospital may be challenging due to the nature of the mission or lack of air superiority. Additionally, as injured personnel progress from the battlefield, CAPP treatment could continue to enhance recovery. While the devices noted above use plasma jets, one could also envision using DBDs, which may provide a larger treatment area [30]. An array of plasma jets could provide an advantage of facilitating contouring to a particular wound or surface geometry.
Diabetic wounds are often used for experimental systems because they are becoming increasingly prevalent in hospitals and are difficult to treat. While the ability of CAPPs to treat diabetic wounds may not seem directly relevant to the warfighter, it demonstrates the capability of the approach and the broader utility for the general population. It also provides a comparative study for treating chronic wounds in post-operative patients since diabetic wounds and ulcers may often be chronic conditions.
A 20 kHz, argon plasma jet effectively enhanced wound healing for rats with either Type 1 or Type 2 diabetes compared to normal rats [40]. The ratio of the current wound area to the initial wound area of all the plasma-treated normal and diabetic groups decreased by up to 30% compared to untreated groups during healing. CAPP had the most significant improvement on wound healing for the Type 2 rats between 4 d and 15 d after being wounded. Histological analysis revealed faster re-epithelialization, collagen deposition, less inflammation, and a more complete skin structure in the plasma-treated groups compared to untreated control. The plasma-treated wounds demonstrated more transforming growth factor beta (TGF-β) expression in the early stage (Day 7) of wound healing, but lower levels later. The overall results indicate that CAPP may increase the number of free radicals involved in cell signaling, which may affect some pathways and accelerate healing of both normal and diabetic wounds.
A study of 65 diabetic foot ulcer wounds from 45 patients showed that an argon plasma jet induced a statistically significant greater reduction in wound area compared to a placebo treatment [41]. Both CAPP and placebo reduced microbial load, although the changes were not statistically significant. The lack of statistical significance most likely occurs because all wounds received standard wound care procedures, such as systemic antibiotic treatment, if indicated, and regular wound debridement, which may mask the antimicrobial benefit of CAPP. While further studies would be required to explore the potential synergistic inactivation of these therapies with CAPP in reducing microbial loading, the results are encouraging for wound healing.
As described above, unlike DBDs, plasma jets can only treat a small surface unless they are arranged in an array. One study [42] compared the effectiveness of an atmospheric air surface DBD, a helium plasma jet, and an argon plasma jet on wound area reduction. The DBD applied a 0–30 kVpp (peak to peak), 11 kHz AC field 1 mm from the target to produce oxygen and nitrogen species. The helium and argon plasma jets used the same range of electric fields but generated the plasma inside a quartz tube with inner and outer diameters of 4 and 6 mm, respectively. The outer aluminum electrode was 10 mm from the tube nozzle and a 1 mm diameter needle was used as the ground. The dielectric of the DBD and the output of the plasma jet were placed 1 mm from 6 mm circular wounds and applied for 30 s for five consecutive days. From the second through seventh day after wounding, the helium plasma jet induced a statistically significant greater reduction in wound area reduction ratio than the DBD. Nine days after treatment, the helium and argon plasma jets had basically eliminated the wound, indicating that both plasma jets were more effective than the DBD. The challenge becomes comparing the “doses” delivered. While the voltage waveforms used to generate the plasmas were the same, the actual doses delivered to the target may differ. Species generation differed between CAPPs; the helium plasma jet generated oxygen, nitrogen, and helium species, while the argon plasma jet created argon plasma species and the DBD created oxygen, nitrogen, and helium species. Although the exact mechanism behind the interaction of the generated species and living tissues and microorganisms is complicated and remains an open topic of research, this study indicates that plasma jets with appropriate voltage waveforms and treatments times are comparable or superior to DBDs for certain wound treatments.
The efficacy of CAPP has also been examined for treating wounds on diabetic male mice using an 8.5 kV, 17 kHz helium plasma jet applied 2 cm from a wound for 90 s or 180 s [43]. After 14 days of treatment, CAPP induced statistically higher wound closure rates following both 90 and 180 s daily CAPP treatments. Compared to control, CAPP treatment caused statistically significant reductions in the protein expression of IL-6, tumor necrosis factor-α, inducible nitric oxide synthase, and superoxide dismutase in two CAPP groups and statistically significant increases in the protein expression of vascular endothelial growth factor and TGF-β. Moreover, CAPP induced no histological changes in normal skin, while suppressing inflammation, reducing oxidative stress, and enhancing angiogenesis.
A 2 min application of a plasma jet to MRSA and E. coli in vitro and to a wound model in genetic diabetic db/db mice was effective [44] using a 10 kVpp, 10 kHz voltage waveform with either helium, helium and 0.1% O2, or helium and 1% O2. The working gas of helium + 0.1% O2 produced the most reactive oxygen nitrogen species (RONS), which increased sterilization efficiency and oxygen supply to the extracellular matrix to stimulate blood vessel growth. The higher concentration of NO stimulated keratinocytes to secrete growth factors. Of particular note, wound closure was faster than untreated control for all CAPP conditions. All CAPP conditions also induced statistically significant increases in VEGF on day 7 and bFGF on days 7, 14, and 21 of treatment, indicating the stimulation of cell proliferation, vasculogenesis, and collagen synthesis, which are impaired in chronic diabetic wounds.
CAPP can also alter blood cell function, with gas selection and treatment duration providing tunability. One study examined the effects of different exposure times (5, 10, and 15 min) of a DBD on red blood cells (RBC) suspended in phosphate-buffered saline (PBS), platelet-rich plasma, and whole blood [45]. This DBD used air, argon, and nitrogen gases at 1.5 L/min with a 3 kVpp, ∼20 kHz waveform [45, 46]. RBCs treated for more than 10 min with an air DBD underwent more hemoloysis and alterations in deformability and aggregation than either N2 or Ar DBDs due to higher levels of hydrogen peroxide, hydroxyl oxidants, and other reactive oxygen/nitrogen species (RONS). Moreover, the air DBD induced longer blood coagulation times and smaller clot firmness than either argon or nitrogen DBDs, which induced no statistically significant difference from the untreated control. These results indicate that the gas selected for a given system may affect the interactions with blood cells, which can alter recovery.
Similarly, 30–120 s treatments of helium plasma jet using a 5 kV, 1–2
These results demonstrate the potential of using CAPP for facilitating platelet activation and aggregation to enhance wound healing. While the U. S. Army is funding the development of a hemostatic gel that flows deeply into penetrating wounds to stop bleeding [49], CAPP provides the possibility of simultaneously providing hemorrhage control, wound healing, and antimicrobial capability. The challenge is assessing the CAPP parameter space (e.g., delivery modality, voltage, PRF, and gas) to achieve these objectives.
Plasma medicine provides a means to induce biophysical phenomena that cannot be achieved in conventional ways. As this technology approaches the clinic, commercial devices are becoming more prevalent, motivating studies of their efficacy and safety. A recent study tested the efficacy and safety of a commercial DBD system (Plasmacure) by performing in vitro bactericidal efficacy and safety experiments and in vivo bactericidal experiments [50]. A 2 min treatment of Staphylococcus aureus in collagen-elastin matrices resulted in a 4-log10 reduction with a lower reduction on dermal samples. CAPP treatments up to 2 min did not affect cellular activity or DNA integrity of human dermal samples. However, repeated daily 2 min CAPP treatments induced a statistically insignificant reduction in cellular activity of dermal samples to 80% after 2–4 days, indicating that CAPP treatments do not adversely alter skin function. For the in vivo studies, treated volunteers reported moderate pain scores (numerical rating scale of 3.3 on a scale of 10) but considered the procedure acceptable. CAPP treatment temporarily increased local skin temperature (≈3.4°C) and erythema, which decreased with decreasing plasma power. Applying CAPP to intact skin artificially contaminated with Pseudomonas aeruginosa induced reasonable inactivation (2.9 log10) of bacterial load in 14/15 volunteers. This value provides an upper bound on the expected effectiveness since the blood, exudate, and cellular limit would shield microorganisms from the CAPP in the more complicated wound environment.
Another study evaluated the antibacterial efficacy of the kINPen® MED argon plasma jet on Staphylococcus aureus in suspension and in biofilm [51]. CAPP treatments of 0–240 s followed by 15- or 120-min incubation induced a slight reduction in bacterial count under planktonic conditions, but had no effect on biofilms. Indirect CAPP treatment of keratinocytes significantly decreased metabolic activity. Adding catalase reversed these effects, indicating a dominant role of CAPP-generated hydrogen peroxide. Thus, while the kINPen® MED argon plasma jet may not be an effective antibacterial therapy for wounds alone, it may be useful when combined with other therapies.
Fibroblasts play a key role in wound healing. Because of the ability of the reactive species generated by plasmas to stimulate various cells, a recent study examined the efficacy of various plasma jets for promoting fibroblast proliferation [52]. A detailed analysis using a 15 kVpp, 60 Hz waveform with various ratios of N2 to N2+O2 showed that a 15 s treatment with N2/(N2+O2) = 70% maximized fibroblast differentiation. These results demonstrated that NO• dose correlates strongly with fibroblast proliferation.
In vitro studies of primary dermal fibroblasts isolated from murine skin and in vivo studies in a murine wound model demonstrated that applying a kINPen (neoplas MED) jet changed the phosphorylation of signaling molecules and increased tissue oxygenation and skin perfusion during ROS-driven wound healing [53]. The CAPP also affected cell surface adhesion receptors, structural proteins, and the transcription of genes associated with various differentiation markers, while enhancing oxygenation and tissue-hemoglobin perfusion of the superficial skin layer. These chemical effects suggest that adjusting the dominant species of the CAPP gas, which plays a major role in the wound healing process, may enable further device optimization and tunability. Of particular note, higher expression of many extracellular matrix and cytoskeletal genes suggests that ROS may alter the signaling of Nrf2, which controls intracellular and extracellular redox signals, and HSF1, which binds many non-heat shock proteins that are essential in cell growth.
To further characterize potential beneficial or deleterious effects of CAPP on the skin, another study examined a 13.5 kV, 300 Hz, 0–300 s atmospheric pressure air DBDs on keratinocytes [54], the primary cell type in the epidermis. Even the longest treatment time considered did not reduce cell viability; however, increasing treatment time did affect migration/proliferation, resulting in a delay of gap closure in migration assays. Moreover, the longer treatment times caused a pro-differentiation effect in the mRNA expression of involucrin and Ki67 that resembled that induced by adding an amount of H2O2 equivalent to that found after the 300 s CAPP treatment. These results suggest that using CAPP to treat chronic wounds could provide a tunable method for inactivating microorganisms, improving microcirculation, and compensating for missing H2O2, which together promote keratinocyte migration and proliferation to initiate angiogenesis, tissue regeneration, and wound closure.
These studies demonstrate the safety of applying standard CAPP devices and parameters. As we shall discuss later, actual devices may require different fill gases and voltages. While safety studies would be required, we conjecture that they should provide similar levels of safety.
CAPPs can also applied to burn wounds with Table 2 summarizing recent studies. A helium jet generated using a 50 Hz waveform at 24 kV for in vivo studies, and 32 kV for in vitro and ex vivo studies, showed enhanced burn wound neovascularization using a mouse model of a third-degree burn wound [55]. CAPP significantly increased the in vivo production of endothelial NO synthase (eNOS), which catalyzes NO synthesis in endothelial cells, and significantly increased the expression of pro-angiogenic PDGFRβ and CD31 markers in mouse wounds. This study also showed that CAPP induced eNOS phosphorylation and activation, which increased the levels of endogenous NO in endothelial cells. This further stimulated VEGFA/VEGFR2 signaling, which further stimulated in vitro angiogenesis, although VEGF levels in CAPP-treated burn wounds were unchanged. This study showed that CAPP effectively increased microvessel formation both in vivo and in vitro.
Applying a 50/60 Hz argon plasma jet with a 5.0 standard liter/minute (slpm) flow rate for 2 min daily for 7 days after inducing burn wounds in Sprague-Dawley rats significantly decreased the expression of proinflammatory cytokines and increased the expression of anti-inflammatory cytokines, which is important since the excessive inflammation often causes poor wound healing and appropriate control of cytokines for organizing the ECM is critical [56]. Specifically, plasma-produced ROS altered the expression of genes involved in wound healing, including TNFα, IL-1β, TGF-β, IL-10, IL-6, iNOS, VEGF, PDGFB, FGF-2, KGF-1, and EGF. Moreover, CAPP also increased the expression of CD68, α-SMA, Fibronectin, and tenascin-C and collagen synthesis. Thus, this study demonstrated the feasibility of using CAPP to treat burn wounds.
CAPP have also been used to effectively treat burns in 44 Wistar Kyoto rats with 11 rats untreated, 11 rats treated with CAPP, 11 rats treated with antiseptic, and 11 rats treated with levomecol [57]. Treating wounds with CAPP leads to final healing after 18 days, compared to 24 days with levomecol, 28 days with antiseptics, and 35 days with no treatment. Similarly, the frequency of infectious complications was significantly lower for CAPP treated burns (9%) compared to levomecol (18.2%), antiseptics (27.2%), or untreated burns (54.5%). The area of the resulting scar after wound healing for the CAPP treated burns was also statistically lower (4 cm2) than levomecol (7 cm2), antiseptic (9 cm2), or untreated burns (12 cm2). The authors stated that CAPP minimized infection by generating a 50–70 nm nanofilm from the coagulated protein layer of the wound exudate and cell fragments that prevented microorganism penetration into the wound.
These studies demonstrate that CAPP can minimize infection and maximize recovery of wounds from burns. This would be an important consideration for treatments in the battlefield at the point of injury.
The interaction of CAPP induced species and electric fields may also enhance transdermal delivery. One study applied 10, 30, and 60 s treatments with argon and helium plasma jets with ∼7 kVpp and ∼45 kHz AC waveforms 4 mm from porcine skin or keratinocytes [58]. Argon plasma irradiation for 60 s resulted in 2.5-fold higher transdermal absorption of aniline blue dye on porcine skin compared to the untreated control. In addition, treating keratinocytes with CAPP for 60 min induced a statistically significant increase in high-molecular-weight molecule (70 and 150 kDa) transmission, demonstrating the possibility of enhanced CAPP induced drug delivery to keratinocytes.
Applying DBDs to the skin requires specifically characterizing and understanding the effects of the CAPP on the skin surface [59]. It is well known that directly applying electric pulses to skin can permeabilize the stratum corneum through electroporation [60], or the formation of pores in the cell membrane that arises due to an applied electric pulse sufficiently charging the cell membrane above a threshold value, typically on the order of 250 mV to 1 V [61]. Since a DBD entails applying an electric field to the skin, electroporation of the stratum corneum may be a potential contributory phenomenological factor. A recent study examined the permeabilization of the stratum corneum ex vivo under exposure to low power
Combining a plasma arc generated by a 1 kVpp, 40 kHz waveform with 20, 40, and 60% duty cycle with electroporation enhanced adsorption rate by 418% in female patient skin as measured by Raman spectroscopy [67]. The concept is that the electric field generated by the waveform applied to the tissue facilitates electroporation in synergy with a fine hole that the arc plasma generates in the stratum corneum to enhance transdermal delivery.
While one would not normally think of CAPP as the first approach for enhancing transdermal or drug delivery, this could provide an additional benefit in treating battlefield trauma. For instance, CAPP could be combined with an appropriate antibiotic and/or wound healing agent to synergistically improve CAPP performance. We discuss some more specific details of synergistic approaches in Section 6.
While CAPP is effective for many applications, any modality eventually encounters limits to effectiveness. One common method to enhance a modality’s effectiveness is to combine it with other modalities that may have complementary mechanisms of action. For instance, pulsed electric fields (PEFs) are commonly used for microorganism inactivation with the primary mechanism of inactivation involving the permeabilization of the outer cell membrane or cell wall [68]. Combining PEFs with other inactivation mechanisms, such as antibiotics, ultrasound, and temperature, can enhance inactivation efficacy, sometimes beyond that anticipated by simply adding the individual efficacies [67]. Similarly, CAPPs may be combined with other approaches to enhance their effectiveness, as summarized in Table 4.
One potential synergistic therapy involves combining CAPP with natural products. For instance, vitamin C dramatically enhanced the efficacy of CAPP against 48 h mature bacterial biofilms of Staphylococcus epidermidis, Escherichia coli, and Pseudomonas aeruginosa [69]. Vitamin C is a common food additive that can generate ROS and kill some bacterial species [70]. Thus, vitamin C and CAPP share primary mechanisms of action for microorganism inactivation that may contribute synergistically. Pre-treating the biofilm with vitamin C for 15 min prior to CAPP treatment using a kINPen 11 (neoplas control GmbH, Greifswald, Germany) plasma jet with a 1 MHz, 2–3 kV waveform reduced the viability compared to CAPP treatment alone from 10 to 2% in E. coli biofilm, 50–11% in P. aeruginosa biofilm, and 61–18% in S. epidermidis biofilm. Although combining vitamin C with CAPP does not improve microorganism inactivation, it does reduce CAPP treatment time, making CAPP treatment more practical under more conditions.
Combining allogenic mesenchymal stem cells (MSCs) with a helium plasma jet with 1.75 slpm and a voltage waveform at 5 MHz improved wound skin regeneration [71, 72]. The self-renewal, multi-lineage differentiation, and secretion of various bioactive molecules that facilitate wound healing make MSCs ideal for treating skin wounds [73]. Treating experimental wounds in sheep with CAPP and MSCs resulted in slower, but more efficient, wound healing than treating individually with either modality or not providing treatment [71]. Specifically, the combined treatment improved the correct development of skin appendages and structural proteins of the dermis. Thus, CAPP may have influenced the MSC’s paracrine activity during initial healing by modulating inflammation and increasing cell proliferation [71]. The anti-fibrotic effects of the MSCs due to their paracrine activity may have counteracted the wound contraction and myofibroblast differentiation induced by CAPP, which would promote the formation and maturation of the granulation tissue.
Not all combinations are beneficial; for example, enhanced wound healing through ROS generation by CAPP may interfere with antioxidants in natural products, resulting in an overall cancellation of the healing enhancement. One study examined the efficacy of applying Binahong leaf extract followed by CAPP for enhanced acute wound healing [74]. The specific argon plasma jet used a 9.58 kVpp, 18.32 MHz waveform [75]. The active components Binahong leaf extract, such as flavonoids and saponins, have anti-inflammatory, antioxidant, and antibacterial properties [76] with flavonoids destroying bacterial cell walls and saponins serving as antiseptics that prevent microorganism growth [77]. While applying the CAPP improved wound healing, applying the Binahong leaf extract before the CAPP impeded this enhancement [74]. The authors observed similar behavior when combining CAPP with Daun sirih leaves [75]. For Daun sirih, they hypothesized that the ethanol solvent may impede CAPP-induced acceleration of acute wound healing [75]. For Binahong leaf extract, they hypothesized that the antioxidant abilities of the Binahong leaf extract hindered the effectiveness of CAPPs to enhance wound healing by counteracting CAPP-induced oxidant formation [74].
Thus, depending on the specific application, CAPP may be combined with other therapies for synergistic benefit in the battlefield. This would certainly be a potential area of interesting study, particularly for combination with natural products or chemicals that may already be readily available to the warfighter. This could be an area of further study to develop ways to enhance CAPP effectiveness as devices are made field ready.
In addition to surface and external applications, CAPP are increasingly used for internal applications due to their demonstrated antimicrobial and coagulation effectiveness. For instance, many surgeries may benefit from a technique that can hasten wound healing and minimize infection while introducing minimal side effects. Moreover, implants may also be susceptible to infections and CAPP provide a means to minimize microbial growth on these materials. Other internal applications, such as stimulating osteoblasts to promote bone healing and neurological applications, are becoming of increased interest. Table 5 summarizes recent applications in these areas.
A recent surgically related example considered applying a 25 kV, 5 kHz air DBD with a discharge power density of 2 W/cm2 for 90–100 s treatments of rabbits following splenectomy [78]. CAPP treatments of 90–100 s effectively coagulated spleen injuries in rabbits. Although minimal damage to the spleen parenchyma occurred in the first few minutes of treatment, the spleen later regenerated with a connective tissue scar. The preserved part of the spleen exhibited complete structural and functional restoration on the 180th day after treatment. While clinical studies would be required before transitioning to the warfighter, this preliminary study demonstrates the potential for applying CAPP to treating internal injuries, which could be critical at the battlefield and a field hospital.
Minimizing bacterial load on surgical sutures is another important consideration for minimizing potential post-operative infections. As a first step, a recent study applied a DBD plasma with a 4.0–5.5 kV, 1.0–5.0 kHz waveform to surgical sutures for 0–360 s [79]. For treatments with DBD alone, the decontamination efficacy of the surgical sutures increased with increasing exposure times from 60 to 360 s. Using a phosphate buffered solution to remove dead cells and permit CAPP interaction with cells in the deeper layers of bacterial biofilm enhanced CAPP inactivation efficacy. As a result, the lethal effect was most often achieved after 300 s regardless of the type of suture and biofilm. Compared to disinfectants, CAPP efficiently decontaminated polymeric surgical suture surfaces for most tested cases, although future studies are required to assess potential CAPP-induced structural modifications of the surgical sutures. This could be a valuable addition in battlefield or surgical hospital application to mitigate potential infections that may arise after surgeries.
Ensuring the effectiveness and preventing infections due to implants is clearly a concern for surgeries following traumatic injuries. CAPP may provide a means to help minimize bacterial load on the implant and stimulate subsequent recovery by stimulating osteoblast differentiation and proliferation at the area of the implant. A recent study compared the efficacy of argon, nitrogen, and 90% argon/10% nitrogen plasma jets with a 500 V, 13.56 MHz AC waveform [80, 81] on polyetheretherketone, a thermoplastic polymer with numerous advantages for dental implants. All CAPP treatments increased hydrophilicity, osteogenic activity, and antibacterial efficiency compared to untreated control, with N2 inducing the best osteogenic activity and hydrophilicity, and Ar/N2 having the strongest antibacterial efficiency of S. aureus and S. mutans.
Another study assessed the efficacy of CAPP for treating stainless steel implants in vitro and in vivo in rabbits [82] using a surface DBD with a high voltage of 7 kV, an 800 ms pulse duration, and ∼3 Hz PRF [83]. CAPP sterilization was as effective as steam sterilization of P multocida–contaminated stainless-steel implants in the rabbits with no important adverse tissue effects. Compared to a steam-autoclave, the plasma device would provide compactness and portability that may facilitate surgical equipment or instrumentation sterilization for ambulatory practice, such as on a farm for veterinarians or in a battlefield or ambulance for a medical doctor or an emergency medical technician/corpsman. Future studies could explore the efficacy of CAPP treatment of implants on various microorganisms, or on osteoblasts, as mentioned above, which could be critical for treating casualties to avoid infections after implants.
A promising implant abutment material because of its biocompatibility and esthetics [84, 85], zirconia is considered a suitable substitute for titanium for oral implants [86]. Treating zirconia with a helium plasma jet with a 13.5 slpm flow rate and a 2.85 kV, 17.0 kHz waveform for 60 s did not alter its morphology [87]. Using ROS staining and a reactive oxygen scavenger demonstrated that the 60 s treatment induced sufficient ROS to simultaneously exceed the threshold for inactivating S. mutans, while remaining within the limits for human fibroblasts. Combined with a prior study that demonstrated that this CAPP could enhance human gingival fibroblast growth without adverse effects, these results suggest the potential for CAPP treatment as a valuable tool for preparing zirconia for dental implants. While this study was focused on dental applications, the approach demonstrates the feasibility for applying CAPP to any materials used for implants, which could be valuable for general implants for wounded servicemembers.
Osteomyelitis, or infection of the bone, may occur due to many factors, most notably trauma, most commonly in long-bones, vertebrae, or feet [88], but also in dental infections and trauma [89], general wounds [90], and bone surgery and joint replacement [90]. From 2003–2004, Brooke Army Medical Center (BAMC) in San Antonio, Texas encountered 23 wounded patients with multidrug resistant Acinetobacter osteomyelitis, compared to only 2 active-duty soldiers out of 326 admitted to the facility in the 14 months preceding the study period [91]. Despite the significance of this issue, there remains no consensus treatment, although surgical intervention is often chosen [91]. Treating 7 mm diameter of bone with a thickness of 1, 2, 3, or 4 mm using the kINPen ®MED argon plasma jet at a flow rate of 5 L/min induced ∼1.2–2.3 log10 reduction in Staphylococcus aureus (selected due to its prevalence in osteomyelitis in the jawbone, although it is more broadly the most common microorganism responsible for osteomyelitis [91]) placed on the bone [89]. Of particular note, CAPP effectively inactivated S. aureus, on both the treated and untreated side of the bone for thickness up to 4 mm, suggesting the potential utility for treating both surfaces and the lower cancellous area of the bone. CAPP often have difficulty penetrating liquids or watery films [93], which may hinder its application in many practical wound healing scenarios, such as those involving bleeding; Ref. [89] observes similar behavior with regions of higher bone density. An opportunity for broadening the applicability of CAPP to the warfighter would be to perform a similar experiment with Acinetobacter, which arose in the BAMC case mentioned above. While some studies have examined CAPP for Acinetobacter inactivation [94–96], none have examined the more relevant bone environment, as done for S. aureus.
Another study [97] applied the Plasma ONE MEDICAL device [98] to alter gene expression and protein regulation that are critically involved in wound healing in human osteoblast-like MG63 osteosarcoma cells. The standard ONE MEDICAL device provided 3–18 kV electric pulses with 2.5–10 μs duration and PRF of 420–1220 Hz. Although not reported here, a prior study by this group examining CAPPs for periodontal wound healing used distances of 1 or 2 cm [99]. CAPP enhanced mRNA of interleukin (IL)-1
CAPP may hold promise for neurological treatments [100]. A recent study applied a DBD using 29 kV, 20 ns pulses with 2 ns rise-times and 1–15 Hz PRF to accelerate neurite regeneration and astrocyte regrowth following mechanical trauma in vitro. Astrocyte cultures required an exposure of 20–90 mJ to accelerate re-growth up to 3 days post-injury, while lower treatment intensities (
An ongoing debate in the plasma medicine community concerns the relative advantages of indirect treatment using plasma jets and direct treatments using DBDs. DBDs combine direct electric field application with charged particle and reactive species generation. Electric fields of sufficient intensity and duration can permeabilize the cell membranes of targeted cells by electroporation. This process alone, or synergistically with other modalities such as heating, drugs, ultrasound, or plasmas, can inactivate microorganisms [68]. Although a treated subject does not typically feel discomfort for short exposure times (and, even then, may simply start to feel the heating of the skin), applying high voltage directly to the tissue may increase safety concerns and complicate regulatory authorization. In the United States, any new therapeutic device for civilian patients requires approval by the Food and Drug Administration; military applications would require approval by separate officials and may be the first step for moving this technology toward the clinic in the United States. By separating the high voltage that creates the plasma from the target, plasma jets mitigate the high voltage safety concern. Moreover, plasma jets provide additional flexibility in maneuverability since they “propel” the plasma to the target rather than requiring positioning a large electrode near the target. This may facilitate applications in confined areas in the body, such as dental applications, which require finely controlled application of a maneuverable exposure [103]. Table 6 summarizes recent studies relevant to device optimization.
One interesting point when considering jets, or arrays of jets, is the importance of the gas flow rate [104]. A recent study constructed a 4 × 4 helium plasma-jet-array device with a sinusoidal voltage waveform of 12 kVpp and a frequency of 19.5 kHz. Exposing a KI-starch reagent to the helium plasma jet showed that shape of the active substances changed when the gas-flow rate was reduced below 8 slm. Furthermore, using a gas-flow rate of 5 slm increased the inactivation range of E. coli at 90 s and S. aureus at 180 s to close to twice the array area. Because the molecular weight of helium (4 g/mol) was lighter than air (29 g/mol), using too high a gas flow rate could cause the active substances to be blown away without contacting the medium containing KI-starch reagent.
Skin diseases can also be significant issues, so a recent study developed a nonthermal multi-needle DBD jet capable of treating large area lesions [105]. The study specifically considered helium, argon, air, and nitrogen plasmas with 25 kHz, 2.73 kVrms, 2.78 kVrms, 3.15 kVrms, and 3.22 kVrms waveforms yielding 18 W, 22.9 W, 31.3 W, and 33.7 W discharges, respectively. The multi-needle structures allowed stable application of the jet over a wide area. This is critical for treating skin areas, such as psoriasis, which was selected as a representative skin disease. Helium and argon plasmas both generate ROS, which are valuable for treating psoriasis. In vitro studies showed that the argon plasma jet inhibited the activation of STAT 3, which is a key contributor in the development and pathogenesis of psoriasis and psoriatic-like inflammatory conditions, in vitro [106], while in vivo studies demonstrated amelioration of psoriasis.
One critical aspect of all these treatments and applications is the reactive species generated by the CAPP. A recent study examined an argon plasma jet without and with water vapor using standard AC waveforms of 25 kHz, 7.5 kVpp (5.03 W) and 25 kHz, 11.1 kVpp (25.47 W), respectively [107]. Optical emission spectroscopy and liquid acidification indicate that adding small amounts of nitrogen and oxygen to the argon plasma jet enhance the effectiveness of generating the desired reactive species for biomedical applications. Whether the electric field is applied to the target (direct) or not (indirect) also plays an important role in the species generation and CAPP interactions. The efficacy of acidification is more prominent for indirect treatment, suggesting that ambient air introduces some mechanisms that may be unclear.
One of the practical challenges with applying CAPPs in a battlefield or trauma setting is providing the necessary power to achieve high voltages. A recent study designed and tested a prototype CAPP system based on a 12 V rechargeable battery so that it did not require connection to an electrical power outlet [108]. This portable system used a 2.5–12 kVpp, 20–40 kHz voltage waveform to generate an air DBD to inactivate planktonic and biofilm versions of E. coli and S. aureus. Applying the DBD for 30 s or longer induced greater than 5-log10 reduction in both E. coli and S. aureus in planktonic form. In biofilm form, a 30 s treatment reduced E. coli and S. aureus viability to 45.4 and 48.9%, respectively; extending treatment time to 120 s reduced these viabilities to 3.9 and 14.1%, respectively. Future work may further optimize device portability or extend to in vivo testing.
This review has summarized recent applications of CAPP for biomedical applications, focusing on wound treatment, burn wound treatment, transdermal delivery, surgical treatments, synergistic treatments, and device optimization. It fills a critical gap between typical reviews, which are broad and describe general applications, by providing a comprehensive summary of developments in plasma medicine over the past 5 years, with a focus on trauma and acute care, as CAPP moves to clinical applications. One major goal of this review is to demonstrate that medical applications of CAPP have advanced to the point that plasma medicine is ready for more serious consideration in a clinical setting, particularly for emergent or critical care.
Several commercial devices, most notably the KinPen, have been developed and used across research groups, demonstrating the consistency of behavior and effects that are critical for widespread adoption of CAPPs in medical settings. While wound healing dominates much of plasma medicine, other applications, such as surgical and implant applications, that may be more niche at present could grow in importance. In both military and civilian emergency medicine, the wounded are more likely to survive initial trauma due to improved technology (e.g. safer vehicles or more consistent use of personal protective equipment), making them more susceptible to subsequent medical issues, such as infections. While CAPP clearly has near term application to wound therapy, it also shows promise in dealing with battlefield trauma through applications to surgical sterilization of sutures to minimize infections and sterilization of implants for minimizing infection and stimulating osteoblast growth.
The form of a field deployable device will come down to design tradeoffs, which we will briefly describe. One major challenge with adopting CAPP technology, particularly in the battlefield or for first responders, has been device portability. Many plasma devices are relatively large (e.g. tabletop) and require a fair amount of power, necessitating their connection to wall power. Recent development of a battery-operated plasma device [108] demonstrates the feasibility of designing truly portable devices. This will become increasingly important in the future battlefield, where air superiority may no longer be guaranteed, and the wounded must be treated at the scene or during transport. Ref. [108] demonstrates the feasibility to constructing a DBD that works in atmospheric pressure air, which provides the greatest flexibility from an operational perspective since the device neither needs separate fill gas or a power outlet. The real question comes down to whether the DBD is the best form factor in the battlefield. On the one hand, it provides great value for treating large wounds since it uses a large electrode; on the other hand, the distance between the electrode and the injured personnel is critical for obtaining the appropriate breakdown condition and it may be difficult to have someone who is severely wounded stay sufficiently still. Moreover, some injuries may require the flexibility of delivery that a plasma jet provides. While plasma jets often use fill gases, the Tables demonstrate cases where plasma jets use air. Thus, while commercial devices are pursuing various fill gases, such as the kINPen’s use of argon or helium, we recommend studies comparing the efficacy and safety of battery powered, air plasmas jets and battery powered DBDs in animal studies replicating appropriate wounds to assess the tradeoffs of these designs. One possibility could be to make a commercial device (e.g. the kINPen) battery powered. Moreover, because some of these considerations (most notably the air plasma jet) are not standard conditions compared to the rest of the literature, future studies are also required to optimize the plasma parameters (most notably voltage, frequency, and treatment time). As the applications move into field hospitals, where power and space limitations are less critical, standard commercial options could be pursued. Also, because wound size would vary between patients, jet devices should also be adaptable to allow easy incorporation of multiple jets. Another issue that we have not seen addressed in the literature is device ruggedization. While a device may be able to sit on a cart or table in a standard hospital environment, the battlefield environment would require a device that can be carried in a pack and would certain be exposed to rough handling. A device for the battlefield would need to be sufficiently ruggedized to ensure that it continued to operate within appropriate specifications. Aside from mechanical ruggedization, it would also need to operate under various ambient temperature and humidity conditions, which can alter plasma performance [109].
In conclusion, CAPP provide a flexible means of addressing numerous surgical and emergent care applications. Device design and optimization have progressed to the point where efficacy, safety, and portability have been demonstrated. The next steps include developing and testing portable, battery-powered devices for addressing injuries at the point of injury for ambulances and trauma in the battlefield and continued system development to address larger wounds and regenerative medicine.
AG conceived the idea for the article, performed the literature search and data analysis, and drafted the manuscript. TM provided important information on background and critically revised the manuscript. All authors read and approved the final manuscript.
This effort was supported by the Naval Force Health Protection Program of Dr. Timothy Bentley, Code 342, Office of Naval Research. The publication fees were supported by the Office of Naval Research (Grant No. N00014-21-1-2055).
The authors declare that the research was conducted in the absence of any commercial or financial relationships that could be construed as a potential conflict of interest.
All claims expressed in this article are solely those of the authors and do not necessarily represent those of their affiliated organizations, or those of the publisher, the editors and the reviewers. Any product that may be evaluated in this article, or claim that may be made by its manufacturer, is not guaranteed or endorsed by the publisher.
1. Mabry RL, DeLorenzo R. Challenges to Improving Combat Casualty Survival on the Battlefield. Mil Med (2014) 179(5):477–82. doi:10.7205/MILMED-D-13-00417
2. Eastridge BJ, Mabry RL, Seguin P, Cantrell J, Tops T, Uribe P, et al. Death on the Battlefield (2001-2011). J Trauma Acute Care Surg (2012) 73(6 Suppl. 5):S431–S437. doi:10.1097/TA.0b013e3182755dcc
3. Kelly JF, Ritenour AE, McLaughlin DF, Bagg KA, Apodaca AN, Mallak CT, et al. Injury Severity and Causes of Death from Operation Iraqi Freedom and Operation Enduring Freedom: 2003-2004 versus 2006. J Trauma (2008) 64(2 Suppl. l):S21–S27. discussion S26-27. doi:10.1097/TA.0b013e318160b9fb
4. Johnson J. Washington's Perceptions and Misperceptions of Beijing's Anti-access Area-Denial (A2-AD) 'strategy': Implications for Military Escalation Control and Strategic Stability. Pac Rev (2017) 30(3):271–88. doi:10.1080/09512748.2016.1239129
5. Yun HC, Murray CK, Nelson KJ, Bosse MJ. Infection after Orthopaedic Trauma: Prevention and Treatment. J Orthop Trauma (2016) 30:S21–S26. doi:10.1097/BOT.0000000000000667
6. Penn-Barwell JG, Bennett PM, Mortiboy DE, Fries CA, Groom AFG, Sargeant ID. Factors Influencing Infection in 10 Years of Battlefield Open Tibia Fractures. Strateg Trauma Limb Reconstr (2016) 11(1):13–8. doi:10.1007/s11751-016-0250-x
7. Yun HC, Blyth DM, Murray CK. Infectious Complications after Battlefield Injuries: Epidemiology, Prevention, and Treatment. Curr Trauma Rep (2017) 3(4):315–23. doi:10.1007/s40719-017-0102-2
8. Garner AL, Loveless AM, Dahal JN, Venkattraman A. A Tutorial on Theoretical and Computational Techniques for Gas Breakdown in Microscale Gaps. IEEE Trans Plasma Sci (2020) 48(4):808–24. doi:10.1109/TPS.2020.2979707
9. von Woedtke T, Schmidt A, Bekeschus S, Wende K, Weltmann K-D. Plasma Medicine: A Field of Applied Redox Biology. In Vivo (2019) 33(4):1011–26. doi:10.21873/invivo.11570
10. von Woedtke T, Emmert S, Metelmann H-R, Rupf S, Weltmann K-D. Perspectives on Cold Atmospheric Plasma (CAP) Applications in Medicine. Phys Plasmas (2020) 27(7):070601. doi:10.1063/5.0008093
11. Laroussi M. Cold Plasma in Medicine and Healthcare: the New Frontier in Low Temperature Plasma Applications. Front Phys (2020) 8:74. 2020 Mar 20. doi:10.3389/fphy.2020.00074
12. Shimizu T. Wound Treatment by Low-Temperature Atmospheric Plasmas and Issues in Plasma Engineering for Plasma Medicine. Jpn J Appl Phys (2020) 59(12):120501. doi:10.35848/1347-4065/abc3a0
13. Liu D, Zhang Y, Xu M, Chen H, Lu X, Ostrikov K. Cold Atmospheric Pressure Plasmas in Dermatology: Sources, Reactive Agents, and Therapeutic Effects. Plasma Process Polym (2020) 17(4):1900218. doi:10.1002/ppap.201900218
14. Laroussi M. Plasma Medicine: a Brief Introduction. Plasma (2018) 1(1):47–60. doi:10.3390/plasma1010005
15. Bernhardt T, Semmler ML, Schäfer M, Bekeschus S, Emmert S, Boeckmann L. Plasma Medicine: Applications of Cold Atmospheric Pressure Plasma in Dermatology. Oxidative Med Cell Longevity (2019) 2019:1–10. doi:10.1155/2019/3873928
16. Graves DB. Mechanisms of Plasma Medicine: Coupling Plasma Physics, Biochemistry, and Biology. IEEE Trans Radiat Plasma Med Sci (2017) 1(4):281–92. doi:10.1109/TRPMS.2017.2710880
17. Reuter S, Von Woedtke T, Weltmann K-D. The kINPen-A Review on Physics and Chemistry of the Atmospheric Pressure Plasma Jet and its Applications. J Phys D: Appl Phys (2018) 51(23):233001. doi:10.1088/1361-6463/aab3ad
18. Laroussi M, Lu X, Keidar M. Perspective: The Physics, Diagnostics, and Applications of Atmospheric Pressure Low Temperature Plasma Sources Used in Plasma Medicine. J Appl Phys (2017) 122(2):020901. doi:10.1063/1.4993710
19. Boehm D, Bourke P. Safety Implications of Plasma-Induced Effects in Living Cells - a Review of In Vitro and In Vivo Findings. Biol Chem (2018) 400(1):3–17. doi:10.1515/hsz-2018-0222
20. Tan F, Fang Y, Zhu L, Al-Rubeai M. Cold Atmospheric Plasma as an Interface Biotechnology for Enhancing Surgical Implants. Crit Rev Biotechnol (2021) 41(3):425–40. doi:10.1080/07388551.2020.1853671
21. Khlyustova A, Labay C, Machala Z, Ginebra M-P, Canal C. Important Parameters in Plasma Jets for the Production of RONS in Liquids for Plasma Medicine: A Brief Review. Front Chem Sci Eng (2019) 13(2):238–52. doi:10.1007/s11705-019-1801-8
22. Tanaka H, Ishikawa K, Mizuno M, Toyokuni S, Kajiyama H, Kikkawa F, et al. State of the Art in Medical Applications Using Non-thermal Atmospheric Pressure Plasma. Rev Mod Plasma Phys (2017) 1(1):1–89. doi:10.1007/s41614-017-0004-3
23. Šimončicová J, Kryštofová S, Medvecká V, Ďurišová K, Kaliňáková B. Technical Applications of Plasma Treatments: Current State and Perspectives. Appl Microbiol Biotechnol (2019) 103(13):5117–29. doi:10.1007/s00253-019-09877-x
24. Assadian O, Ousey KJ, Daeschlein G, Kramer A, Parker C, Tanner J, et al. Effects and Safety of Atmospheric Low‐temperature Plasma on Bacterial Reduction in Chronic Wounds and Wound Size Reduction: A Systematic Review and Meta‐analysis. Int Wound J (2019) 16(1):103–11. doi:10.1111/iwj.12999
25. Babaeva NY, Naidis GV. Modeling of Plasmas for Biomedicine. Trends Biotechnol (2018) 36(6):603–14. doi:10.1016/j.tibtech.2017.06.017
26. Shimizu T, Ikehara Y. Benefits of Applying Low-Temperature Plasma Treatment to Wound Care and Hemostasis from the Viewpoints of Physics and Pathology. J Phys D: Appl Phys (2017) 50(50):503001. doi:10.1088/1361-6463/aa945e
27. Braný D, Dvorská D, Halašová E, Škovierová H. Cold Atmospheric Plasma: A Powerful Tool for Modern Medicine. Ijms (2020) 21(8):2932. doi:10.3390/ijms21082932
28. Petlin DG, Tverdokhlebov SI, Anissimov YG. Plasma Treatment as an Efficient Tool for Controlled Drug Release from Polymeric Materials: a Review. J Controlled Release (2017) 266:57–74. doi:10.1016/j.jconrel.2017.09.023
29. Bekeschus S, Favia P, Robert E, von Woedtke T. White Paper on Plasma for Medicine and hygiene: Future in Plasma Health Sciences. Plasma Process Polym (2019) 16:1800033. doi:10.1002/ppap.201800033
30. Fridman G, Friedman G, Gutsol A, Shekhter AB, Vasilets VN, Fridman A. Applied Plasma Medicine. Plasma Process Polym (2008) 5(6):503–33. doi:10.1002/ppap.200700154
31. Ayan H, Fridman G, Gutsol AF, Vasilets VN, Fridman A, Friedman G. Nanosecond-pulsed Uniform Dielectric-Barrier Discharge. IEEE Trans Plasma Sci (2008) 36(2):504–8. doi:10.1109/TPS.2008.917947
32. Schmidt-Bleker A, Winter J, Bösel A, Reuter S, Weltmann K-D. On the Plasma Chemistry of a Cold Atmospheric Argon Plasma Jet with Shielding Gas Device. Plasma Sourc Sci. Technol. (2015) 25(1):015005. doi:10.1088/0963-0252/25/1/015005
33. Wang X-F, Fang Q-Q, Jia B, Hu Y-Y, Wang Z-C, Yan K-p., et al. Potential Effect of Non-thermal Plasma for the Inhibition of Scar Formation: a Preliminary Report. Sci Rep (2020) 10(1):1064. doi:10.1038/s41598-020-57703-6
34. Zhang J-P, Guo L, Chen Q-L, Zhang K-Y, Wang T, An G-Z, et al. Effects and Mechanisms of Cold Atmospheric Plasma on Skin Wound Healing of Rats. Contrib Plasma Phys (2019) 59(1):92–101. doi:10.1002/ctpp.201800025
35. Chatraie M, Torkaman G, Khani M, SalehiShokri HB, Shokri B. In Vivo study of Non-invasive Effects of Non-thermal Plasma in Pressure Ulcer Treatment. Sci Rep (2018) 8(1):5621. doi:10.1038/s41598-018-24049-z
36. Park CH, Lee JS, Kim JH, Kim D-K, Lee OJ, Ju HW, et al. Wound Healing with Nonthermal Microplasma Jets Generated in Arrays of Hourglass Microcavity Devices. J Phys D: Appl Phys (2014) 47(43):435402. doi:10.1088/0022-3727/47/43/435402
37. Lee OJ, Ju HW, Khang G, Sun PP, Rivera J, Cho JH, et al. An Experimental Burn Wound-Healing Study of Non-thermal Atmospheric Pressure Microplasma Jet Arrays. J Tissue Eng Regen Med (2016) 10(4):348–57. doi:10.1002/term.2074
38. Martines E, Brun P, Cavazzana R, Cordaro L, Zuin M, Martinello T, et al. Wound Healing Improvement in Large Animals Using an Indirect Helium Plasma Treatment. Clin Plasma Med (2020) 17-18:100095. doi:10.1016/j.cpme.2020.100095
39. Kim Y-W, Byzova TV. Oxidative Stress in Angiogenesis and Vascular Disease. Blood (2014) 123:625–31. doi:10.1182/blood-2013-09-512749
40. Cheng K-Y, Lin Z-H, Cheng Y-P, Chiu H-Y, Yeh N-L, Wu T-K, et al. Wound Healing in Streptozotocin-Induced Diabetic Rats Using Atmospheric-Pressure Argon Plasma Jet. Sci Rep (2018) 8(1):12214. doi:10.1038/s41598-018-30597-1
41. Stratmann B, Costea T-C, Nolte C, Hiller J, Schmidt J, Reindel J, et al. Effect of Cold Atmospheric Plasma Therapy vs Standard Therapy Placebo on Wound Healing in Patients with Diabetic Foot Ulcers. JAMA Netw Open (2020) 3(7):e2010411. doi:10.1001/jamanetworkopen.2020.10411
42. Shahbazi Rad Z, Abbasi Davani F. Measurements of the Electrical Parameters and Wound Area for Investigation on the Effect of Different Non-thermal Atmospheric Pressure Plasma Sources on Wound Healing Time. Measurement (2020) 155:107545. doi:10.1016/j.measurement.2020.107545
43. He R, Li Q, Shen W, Wang T, Lu H, Lu J, et al. The Efficacy and Safety of Cold Atmospheric Plasma as a Novel Therapy for Diabetic Wound In Vitro and In Vivo. Int Wound J (2020) 17(3):851–63. doi:10.1111/iwj.13341
44. Pan S, Zhang S, Chen H. Low Temperature Plasma Promotes the Healing of Chronic Wounds in Diabetic Mice. J Phys D: Appl Phys (2020) 53(18):185205. doi:10.1088/1361-6463/ab7514
45. Kim J, Ghimire B, Lim S, Choi EH, Park H-K, Kaushik NK. Coagulation, Deformability, and Aggregation of RBCs and Platelets Following Exposure to Dielectric Barrier Discharge Plasma with the Use of Different Feeding Gases. J Phys D: Appl Phys (2019) 52(15):155202. doi:10.1088/1361-6463/ab0198
46. Kim J, Kim JH, Chang B, Choi EH, Park H-K. Hemorheological Alterations of Red Blood Cells Induced by Non-thermal Dielectric Barrier Discharge Plasma. Appl Phys Lett (2016) 109(19):193701. doi:10.1063/1.4967451
47. Jia B, Liu J, Yin S, Liu Z, Zheng S, Yan K. Low Temperature Plasma Treatment of Rat Blood Is Accompanied by Platelet Aggregation. Plasma Chem Plasma Process (2021) 41:955–72. doi:10.1007/s11090-021-10176-5
48. Neculaes B, Garner AL, Klopman S, Morton CA, Torres AS. A Multi-Donor Ex Vivo Platelet Activation and Growth Factor Release Study Using Electric Pulses with Durations up to 100 Microseconds. IEEE Access (2021) 9:31340–9. doi:10.1109/ACCESS.2021.3060443
49.Stopping bleeding saves lives on the battlefield (IMAGE) (2021) Stopping Bleeding Saves Lives on the Battlefield (IMAGE) Available at: https://www.army.mil/article/245725/stopping_bleeding_saves_lives_on_the_battlefield, Accessed 21 November 2021.
50. Boekema B, Stoop M, Vlig M, van Liempt J, Sobota A, Ulrich M, et al. Antibacterial and Safety Tests of a Flexible Cold Atmospheric Plasma Device for the Stimulation of Wound Healing. Appl Microbiol Biotechnol (2021) 105:2057–70. doi:10.1007/s00253-021-11166-5
51. Plattfaut I, Besser M, Severing A-L, Stürmer EK, Opländer C. Plasma Medicine and Wound Management: Evaluation of the Antibacterial Efficacy of a Medically Certified Cold Atmospheric Argon Plasma Jet. Int J Antimicrob Agents (2021) 57:106319. doi:10.1016/j.ijantimicag.2021.106319
52. Hori Y, Iwata N, Gamaleev V, Oh JS, Murata T, Hori M, et al. Identification of Key Neutral Species in Atmospheric‐pressure Plasma for Promoting Proliferation of Fibroblast Cells. Plasma Process Polym (2021) 18:e2000225. doi:10.1002/ppap.2020002210.1002/ppap.202000225
53. Schmidt A, Liebelt G, Nießner F, von Woedtke T, Bekeschus S. Gas Plasma-Spurred Wound Healing Is Accompanied by Regulation of Focal Adhesion, Matrix Remodeling, and Tissue Oxygenation. Redox Biol (2021) 38:101809. doi:10.1016/j.redox.2020.101809
54. Balzer J, Demir E, Kogelheide F, Fuchs PC, Stapelmann K, Opländer C. Cold Atmospheric Plasma (CAP) Differently Affects Migration and Differentiation of Keratinocytes via Hydrogen Peroxide and Nitric Oxide-Related Products. Clin Plasma Med (2019) 13:1–8. doi:10.1016/j.cpme.2018.11.001
55. Duchesne C, Banzet S, Lataillade JJ, Rousseau A, Frescaline N. Cold Atmospheric Plasma Modulates Endothelial Nitric Oxide Synthase Signalling and Enhances Burn Wound Neovascularisation. J Pathol (2019) 249(3):368–80. doi:10.1002/path.5323
56. Lee Y, Ricky S, Lim TH, Kim H, Lee EJ, Song Y, et al. An Atmospheric Plasma Jet Induces Expression of Wound Healing Genes in Progressive Burn Wounds in a Comb Burn Rat Model: a Pilot Study. J Burn Care Res (2021) 22:irab005. doi:10.1093/jbcr/irab005
57. Zinovev E, Tsygan V, Asadulaev M, Borisov O, Lopatin I, Lukianov S, et al. Possibilities of Application of Low- Temperature Air Pressure in the Treatment of Burn Wounds. Plasma Med (2018) 8(3):217–23. doi:10.1615/PlasmaMed.2018028250
58. Lee S, Choi J, Kim J, Jang Y, Lim TH. Atmospheric Pressure Plasma Irradiation Facilitates Transdermal Permeability of Aniline Blue on Porcine Skin and the Cellular Permeability of Keratinocytes with the Production of Nitric Oxide. Appl Sci (2021) 11(5):2390. doi:10.3390/app11052390
59. Gelker M, Mrotzek J, Ichter A, Müller-Goymann CC, Viöl W. Influence of Pulse Characteristics and Power Density on Stratum Corneum Permeabilization by Dielectric Barrier Discharge. Biochim Biophys Acta (Bba) - Gen Subjects (2019) 1863(10):1513–23. doi:10.1016/j.bbagen.2019.05.014
60. Dermol-Černe J, Pirc E, Miklavčič D. Mechanistic View of Skin Electroporation - Models and Dosimetry for Successful Applications: an Expert Review. Expert Opin Drug Deliv (2020) 17(5):689–704. doi:10.1080/17425247.2020.1745772
61. Teissié J, Rols MP. An Experimental Evaluation of the Critical Potential Difference Inducing Cell Membrane Electropermeabilization. Biophysical J (1993) 65:409–13. doi:10.1016/S0006-3495(93)81052-X
62. Schoenbach KH, Joshi RP, Kolb JF, Nianyong Chen N, Stacey M, Blackmore PF, et al. Ultrashort Electrical Pulses Open a New Gateway into Biological Cells. Proc IEEE (2004) 92(7):1122–37. doi:10.1109/JPROC.2004.829009
63. Pakhomov AG, Shevin R, White JA, Kolb JF, Pakhomova ON, Joshi RP, et al. Membrane Permeabilization and Cell Damage by Ultrashort Electric Field Shocks. Arch Biochem Biophys (2007) 465(1):109–18. doi:10.1016/j.abb.2007.05.003
64. Pakhomov AG, Bowman AM, Ibey BL, Andre FM, Pakhomova ON, Schoenbach KH. Lipid Nanopores Can Form a Stable, Ion Channel-like Conduction Pathway in Cell Membrane. Biochem Biophysical Res Commun (2009) 385(2):181–6. doi:10.1016/j.bbrc.2009.05.035
65. Pakhomov AG, Gianulis E, Vernier PT, Semenov I, Xiao S, Pakhomova ON. Multiple Nanosecond Electric Pulses Increase the Number but Not the Size of Long-Lived Nanopores in the Cell Membrane. Biochim Biophys Acta (Bba) - Biomembranes (2015) 1848(4):958–66. doi:10.1016/j.bbamem.2014.12.026
66. Nesin OM, Pakhomova ON, Xiao S, Pakhomov AG. Manipulation of Cell Volume and Membrane Pore Comparison Following Single Cell Permeabilization with 60- and 600-ns Electric Pulses. Biochim Biophys Acta (Bba) - Biomembranes (2011) 1808(3):792–801. doi:10.1016/j.bbamem.2010.12.012
67. Kim E-S, Enkhzaya G, Hwang H-S, Han J-H, Kim C-S, Shin J-W, et al. Highly Efficient Transfection Effect of Transdermal Drug Delivery via Skin by Hybrid Bipolar Arc Plasma Stimulation and Dual Pulse Electroporation Technique. IEEE Access (2021) 9:24071–8. doi:10.1109/ACCESS.2021.3056723
68. Garner AL. Pulsed Electric Field Inactivation of Microorganisms: from Fundamental Biophysics to Synergistic Treatments. Appl Microbiol Biotechnol (2019) 103(19):7917–29. doi:10.1007/s00253-019-10067-y
69. Helgadóttir S, Pandit S, Mokkapati VRSS, Westerlund F, Apell P, Mijakovic I. Vitamin C Pretreatment Enhances the Antibacterial Effect of Cold Atmospheric Plasma. Front Cel Infect. Microbiol. (2017) 7:43. doi:10.3389/fcimb.2017.00043
70. Vilchèze C, Hartman T, Weinrick B, Jacobs WR. Mycobacterium tuberculosis Is Extraordinarily Sensitive to Killing by a Vitamin C-Induced Fenton Reaction. Nat Commun (2013) 4(1):1881. doi:10.1038/ncomms2898
71. Melotti L, Martinello T, Perazzi A, Martines E, Zuin M, Modenese D, et al. Could Cold Plasma Act Synergistically with Allogeneic Mesenchymal Stem Cells to Improve Wound Skin Regeneration in a Large Size Animal Model? Res Vet Sci (2021) 136:97–110. doi:10.1016/j.rvsc.2021.01.019
72. Martines E, Zuin M, Cavazzana R, Gazza E, Serianni G, Spagnolo S, et al. A Novel Plasma Source for Sterilization of Living Tissues. New J Phys (2009) 11(11):115014. doi:10.1088/1367-2630/11/11/115014
73. Cerqueira MT, Pirraco RP, Marques AP. Stem Cells in Skin Wound Healing: Are We There yet? Adv Wound Care (2016) 5(4):164–75. 2016 Apr 1. doi:10.1089/wound.2014.0607
74. Darmawati S, Nasruddin N, Kurniasiwi P, Mukaromah AH, Iswara A, Putri GSA, et al. Plasma Jet Effectiveness Alteration in Acute Wound Healing by Binahong (Anredera Cordifolia) Extract. Plasma Med (2020) 10(4):259–71. doi:10.1615/PlasmaMed.2021037264
75. Rahayu HSE, Nasruddin N, Nurani LH, Darmawati S, Rohmani A, Lutfiyati H, et al. Ethanolic Extract of the Natural Product of Daun Sirih (Piper Betle) Leaves May Impede the Effectiveness of the Plasma Jet Contact Style for Acute Wounds. Clin Plasma Med (2019) 15:100090. doi:10.1016/j.cpme.2019.100090
76. Lestari D, Sukandar Y, Fidrianny I. Anredera Cordifolia Leaves Extract as Anti-hyperlipidemia and Endothelial Fat Content Reducer in Male Wistar Rat. Int J Pharm Clin Res (2015) 7(6):435–9. doi:10.25258/ijpcr.v7i6.11
77. Yuniarti WM, Lukiswanto BS. Effects of Herbal Ointment Containing the Leaf Extracts of Madeira Vine (Anredera Cordifolia (Ten.) Steenis) for Burn Wound Healing Process on Albino Rats. Vet World (2017) 10(7):808–13. doi:10.14202/vetworld.2017.808-813
78. Aleinik A, Baikov A, Shevtsova N, Semichev E, Bushlanov P, Turgunova N. Application of Cold Plasma for Performing a Typical Resection of the Spleen. Biomed Phys Eng Express (2018) 4(5):055026. doi:10.1088/2057-1976/aadb2d
79. Czapka T, Maliszewska I, Winkler A. Decontamination of Polymeric Surgical Sutures Covered with Bacterial Biofilms Using Nonthermal Plasma. Plasma Chem Plasma Process (2021) 41(1):227–43. doi:10.1007/s11090-020-10142-7
80. Liu C, Bai J, Wang Y, Chen L, Wang D, Ni S, et al. The Effects of Three Cold Plasma Treatments on the Osteogenic Activity and Antibacterial Property of PEEK. Dental Mater (2021) 37(1):81–93. doi:10.1016/j.dental.2020.10.007
81. Xue C, Gao F, Wen D-Q, Wang Y-N. Experimental Investigation of the Electron Impact Excitation Behavior in Pulse-Modulated Radio Frequency Ar/O 2 Inductively Coupled Plasma. J Appl Phys (2019) 125(2):023303. doi:10.1063/1.5079585
82. Avellar HK, Williams MR, Brandão J, Narayanan S, Ramachandran A, Holbrook TC, et al. Safety and Efficacy of Cold Atmospheric Plasma for the Sterilization of a Pasteurella Multocida-Contaminated Subcutaneously Implanted Foreign Body in Rabbits. Am J Vet Res (2021) 82(2):118–24. doi:10.2460/ajvr.82.2.118
83. Timmons C, Pai K, Jacob J, Zhang G, Ma LM. Inactivation of Salmonella enterica, Shiga Toxin-Producing Escherichia coli, and Listeria Monocytogenes by a Novel Surface Discharge Cold Plasma Design. Food Control (2018) 84:455–62. doi:10.1016/j.foodcont.2017.09.007
84. Blatz MB, Bergler M, Holst S, Block MS. Zirconia Abutments for Single-Tooth Implants-Rationale and Clinical Guidelines. J Oral Maxillofac Surg (2009) 67:74–81. doi:10.1016/j.joms.2009.07.011
85. Pozzobon JL, Pereira GKR, Wandscher VF, Dorneles LS, Valandro LF. Mechanical Behavior of Yttria-Stabilized Tetragonal Zirconia Polycrystalline Ceramic after Different Zirconia Surface Treatments. Mater Sci Eng C (2017) 77:828–35. doi:10.1016/j.msec.2017.03.299
86. Sivaraman K, Chopra A, Narayan AI, Balakrishnan D. Is Zirconia a Viable Alternative to Titanium for Oral Implant? A Critical Review. J Prosthodontic Res (2018) 62:121–33. doi:10.1016/j.jpor.2017.07.003
87. Yang Y, Zheng M, Jia Y-N, Li J, Li H-P, Tan J-G. Time-dependent Reactive Oxygen Species Inhibit Streptococcus Mutans Growth on Zirconia after a Helium Cold Atmospheric Plasma Treatment. Mater Sci Eng C (2021) 120:111633. doi:10.1016/j.msec.2020.111633
88. Sia IG, Berbari EF. Osteomyelitis. Best Pract Res Clin Rheumatol (2006) 20(6):1065–81. doi:10.1016/j.berh.2006.08.014
89. Kniha K, Möhlhenrich SC, Bock A, Ayoub N, Modabber A, Hölzle F, et al. Evaluation of the Bactericidal Effect of Cold Atmospheric Pressure Plasma on Contaminated Human Bone: an In Vitro Study. Br J Oral Maxillofac Surg (2020) 58:329–33. doi:10.1016/j.bjoms.2020.01.003
90. Davis KA, Moran KA, McAllister CK, Gray PJ. Multidrug-resistant Acinetobacter Extremity Infections in Soldiers. Emerg Infect Dis (2005) 11(8):1218–24. doi:10.3201/1108.050103
91. Lew DP, Waldvogel FA. Osteomyelitis. The Lancet (2004) 364(9431):369–79. doi:10.1016/S0140-6736(04)16727-5
92. Hauer L, Jambura J, Hrusak D, Chalupova M, Posta P, Rusnak S, et al. Surgical Therapy for Medication-Related Osteonecrosis of the Jaw in Osteoporotic Patients Treated with Antiresorptive Agents. Biomed Pap (2020) 164(1):100–7. doi:10.5507/bp.2018.081
93. Hafner S, Ehrenfeld M, Neumann A-C, Wieser A. Comparison of the Bactericidal Effect of Cold Atmospheric Pressure Plasma (CAPP), Antimicrobial Photodynamic Therapy (aPDT), and Polihexanide (PHX) in a Novel Wet Surface Model to Mimic Oral Cavity Application. J Craniomaxillofac Surg (2018) 46:2197–202. doi:10.1016/j.jcms.2018.09.006
94. Lis KA, Kehrenberg C, Boulaaba A, von Köckritz-Blickwede M, Binder S, Li Y, et al. Inactivation of Multidrug-Resistant Pathogens and Yersinia Enterocolitica with Cold Atmospheric-Pressure Plasma on Stainless-Steel Surfaces. Int J Antimicrob Agents (2018) 52(6):811–8. doi:10.1016/j.ijantimicag.2018.08.023
95. Ruan Z, Guo Y, Gao J, Yang C, Lan Y, Shen J, et al. Control of Multidrug-Resistant planktonicAcinetobacter Baumannii: Biocidal Efficacy Study by Atmospheric-Pressure Air Plasma. Plasma Sci Technol (2018) 20(6):065513. doi:10.1088/2058-6272/aab302
96. Svarnas P, Spiliopoulou A, Koutsoukos P, Gazeli K, Anastassiou E. Acinetobacter Baumannii Deactivation by Means of DBD-Based Helium Plasma Jet. Plasma (2019) 2(2):77–90. doi:10.3390/plasma2020008
97. Eggers B, Marciniak J, Memmert S, Kramer FJ, Deschner J, Nokhbehsaim M. The Beneficial Effect of Cold Atmospheric Plasma on Parameters of Molecules and Cell Function Involved in Wound Healing in Human Osteoblast-like Cells In Vitro. Odontology (2020) 108:607–16. doi:10.1007/s10266-020-00487-y
98.plasmamedicalsystems (2021) Plasmamedicalsystems Available at: http://www.plasmamedicalsystems.com/2/dental/technical-details/, Accessed 11 September 2021.
99. Kleineidam B, Nokhbehsaim M, Deschner J, Wahl G. Effect of Cold Plasma on Periodontal Wound Healing-An In Vitro Study. Clin Oral Invest (2019) 23(4):1941–50. doi:10.1007/s00784-018-2643-3
100. Katiyar KS, Lin A, Fridman A, Keating CE, Cullen DK, Miller V. Non-thermal Plasma Accelerates Astrocyte Regrowth and Neurite Regeneration Following Physical Trauma In Vitro. Appl Sci (2019) 9(18):3747. doi:10.3390/app9183747
101. Dagro AM, Wilkerson JW, Thomas TP, Kalinosky BT, Payne JA. Computational Modeling Investigation of Pulsed High Peak Power Microwaves and the Potential for Traumatic Brain Injury. Sci Adv (2021) 7(44):eabd8405. doi:10.1126/sciadv.abd8405
102. Tian M, Qi M, Liu Z, Xu D, Chen H, Kong MG. Cold Atmospheric Plasma Elicits Neuroprotection against Glutamate Excitotoxicity by Activating Cellular Antioxidant Defense. Plasma Chem Plasma Process (2021) 41:945–54. doi:10.1007/s11090-021-10172-9
103. Jiang C, Chen M-T, Gorur A, Schaudinn C, Jaramillo DE, Costerton JW, et al. Nanosecond Pulsed Plasma Dental Probe. Plasma Process Polym (2009) 6(8):479–83. doi:10.1002/ppap.200800133
104. Zhu H, Guo L, Li M, Jiang Y, Li H. Comparison of Spatial Distribution of Active Substances and Sterilization Range Generated by Array of Printed-Circuit-Board Plasma Jets. Vacuum (2021) 184:109982. doi:10.1016/j.vacuum.2020.109982
105. Ma S, Lee M-H, Kang SU, Lee YS, Kim C-H, Kim K. Development of an Atmospheric Nonthermal Multineedle Dielectric Barrier Discharge Jet for Large Area Treatment of Skin Diseases. Curr Appl Phys (2021) 24:24–31. doi:10.1016/j.cap.2021.02.003
106. Calautti E, Avalle L, Poli V. Psoriasis: a STAT3-Centric View. Ijms (2018) 19(1):171. doi:10.3390/ijms19010171
107. Lin YT. Enhancement of Selected Species in Nonthermal Atmospheric Pressure Plasma: Implications on Wound Healing Effects. IEEE Trans Plasma Sci (2019) 47(2):1134–44. doi:10.1109/TPS.2018.2867495
108. Usta YH, Çukur E, Yıldırım Ç, Ercan UK. Design of a Portable, Battery-Powered Non-thermal Atmospheric Plasma Device and Characterization of its Antibacterial Efficacies. J Electrostatics (2019) 99:1–8. doi:10.1016/j.elstat.2019.03.002
Keywords: plasma medicine, reactive species, wound healing, microorganism inactivation, dielectric barrier discharge, plasma jets
Citation: Garner AL and Mehlhorn TA (2021) A Review of Cold Atmospheric Pressure Plasmas for Trauma and Acute Care. Front. Phys. 9:786381. doi: 10.3389/fphy.2021.786381
Received: 30 September 2021; Accepted: 30 November 2021;
Published: 21 December 2021.
Edited by:
Kenji Ishikawa, Nagoya University, JapanReviewed by:
Nigel John Mason, University of Kent, United KingdomCopyright © 2021 Garner and Mehlhorn. This is an open-access article distributed under the terms of the Creative Commons Attribution License (CC BY). The use, distribution or reproduction in other forums is permitted, provided the original author(s) and the copyright owner(s) are credited and that the original publication in this journal is cited, in accordance with accepted academic practice. No use, distribution or reproduction is permitted which does not comply with these terms.
*Correspondence: Allen L. Garner, YWxnYXJuZXJAcHVyZHVlLmVkdQ==
Disclaimer: All claims expressed in this article are solely those of the authors and do not necessarily represent those of their affiliated organizations, or those of the publisher, the editors and the reviewers. Any product that may be evaluated in this article or claim that may be made by its manufacturer is not guaranteed or endorsed by the publisher.
Research integrity at Frontiers
Learn more about the work of our research integrity team to safeguard the quality of each article we publish.