- 1School of Physics, University of Bristol, Bristol, United Kingdom
- 2School of Chemistry, University of Bristol, Bristol, United Kingdom
- 3Institut Laue Langevin, Grenoble, France
- 4Mesa+ Institute for Nanotechnology, University of Twente, Enschede, Netherlands
- 5School of Chemical Engineering, UNSW Sydney, Kensington, NSW, Australia
Lipids have an important role in the complex lubrication of articulating joints, however changes in lipid phase behavior that occur owing to mechanical confinement are not well understood. Here, a surface force-type apparatus has been combined with neutron reflectometry to measure confinement-induced changes in the structure of lipids, the major surface-active component of the lubricant in articulating joints. The same incompressible state was accessed under low uniaxial stress (1 bar), irrespective of whether the lipids had started out unconfined above or below the Lα phase transition, and irrespective of whether they were fully or partially hydrated. In this incompressible state, the lipid component had thickened indicating extension and rearrangement of the lipid chains in response to the applied stress. The small amount of water remaining between each lipid bilayer was found to be similar for all chain lengths and starting phases. This represents the first structural evidence of the tightly bound water layer at the headgroups, which is required for hydration lubrication under load.
Introduction
In contrast to mechanical devices, which are mostly lubricated with oil, nature lubricates exclusively with water. In fact, water-based lubrication presents several advantages as water is nontoxic, abundant and an effective coolant, but water alone is a poor lubricant. It has been extensively shown that nature overcomes this with the addition of biological molecules, with the ability to modify surfaces to make them far more lubricating at slow speeds and under the high loads experienced in human joints [1, 2]. However, the mechanisms underlying biological aqueous lubrication in confined conditions are far from being well understood.
There have been a number of studies on the role of both lubricin and hyaluronic acid (HA) within the synovial fluid for lubrication [3, 4] and antifouling [5]. With increasing complexity, phospholipids have been included with the lubricin and HA in surface force measurements [6, 7]. A lowering of the friction coefficient was found when all components were included, with the phospholipids interpreted to be located on the outer surface of the lubricin-HA layer. It is still unclear exactly in what form the phospholipids within the synovial fluid exist, they could form vesicles [6, 8], alternatively some authors propose that a single surfactant like layer forms on each cartilage surface [6, 9] or they could form bilayers or multilayers on the surfaces. The Hills model focusses on the role of the phospholipids at the articular surface of cartilage, it describes phospholipids to be the major “solid” component of the lubricant in articulating joints [10–12], where the fluid component is water. The breakdown of this complex lubricating layer can contribute to wear of the cartilage and eventually osteoarthritis [13]. Thus, the structural study of these lipid constituents under confinement can offer insight into their role in lubrication.
Investigations into the force response of individual lipids under confinement is well established using the surface force apparatus (SFA) [14–17]. In particular, Orozco-Alcaraz and Kuhl [14] applied the technique to lipids while using silica as the substrate, to study symmetric DPPC-DPPC interactions and understand the influence of substrate charge on the bilayer interactions in confinement. Although the SFA provides a very sensitive measurement of forces in friction and confinement [18, 19], and can be used to infer the interactions between bilayers [15, 20], it is not possible to measure the structure simultaneously while the confinement is applied. Another confinement approach is pipette aspiration, which showed that the area of saturated lipids expands with applied confinement [21]. While the increase in area is measured directly, the full structure cannot be elucidated using the technique. Other pressure related approaches to confinement have also been used including osmotic pressure probed using small angle X-ray scattering [22, 23], and also through molecular dynamics simulations [24, 25]. X-ray and neutron reflectometry have proven especially powerful tools in the study of biomembranes in order to measure structure. For example, they can be used to determine phase behavior, layer spacing and volumes occupied by headgroups [26, 27]. These scattering approaches are particularly useful for the study of confinement [28]. Here, we utilize a new type of confinement apparatus [29–32] combined with in situ neutron reflectivity. It is important to mention that unlike a traditional SFA the samples are equilibrated at a small number of set pressures, with the distance between the confining surfaces, and the structure and hydration of the lipids, then determined by neutron reflectivity. In our experiments we study zwitterionic lipid stacks as a model for articulating cartilage surfaces under uniaxial confinement. Thus, direct measurement of the structure during confinement is undertaken.
Methods
Materials: The lipids used were a series of saturated phosphatidyl choline lipids with increasing hydrocarbon chain length; 1,2-dilauroyl-sn-glycero-3-phosphocholine (DLPC, 12:0, Tm = −2°C), 1,2-dimyristoyl-sn-glycero-3-phosphocholine (DMPC, 14:0, Tm = 24°C), 1,2-palmitoyl-snglycero-3-phosphocholine (DPPC, 16:0, Tm = 41°C), 1,2-distearoyl-sn-glycero-3-phosphocholine (DSPC, 18:0, Tm = 55°C) and one with an unsaturated chain 1,2-dioleoyl-sn-glycero-3-phosphocholine (DOPC, 18:1c9, Tm = −17°C). All lipids were purchased from Avanti Polar Lipids Inc. (United States) distributed by INstruchemie BV (Netherlands) and the transition temperatures from the LIPIDAT NIST standard reference database [33]. Chloroform (>99.9%, HPLC grade) and deuterium oxide were supplied by Sigma Aldrich (United Kingdom and France). Demineralised (Milli-Q) water with a resistivity of 18.2 MΩ cm was used in cleaning the silicon surfaces.
Sample preparation and experiment conditions: Samples were prepared by spin coating onto 75 mm diameter silicon blocks from a solution of 0.5% lipid in chloroform at 3000 RPM and annealed at 50°C in an oven for 1 h before use. The silicon blocks were secured in the confinement apparatus, Supplementary Figures S1 in Supporting Information (full details [29]) and mounted vertically on the D17 reflectometer [34]. D2O was used to hydrate the hydrogenous lipids. Two different hydration methods were used: vapor hydration was achieved by placing ∼2 ml of D2O inside the confinement cell but not where it would directly touch the sample; full hydration involved placing a few drops of D2O onto the surface of the sample before inflating the flexible membrane against it to create the confined geometry [35–37]. Owing to the vertical sample geometry of the D17 reflectometer, fully hydrated samples could not be measured without confinement applied, instead measurements commenced at 1 bar of confinement. The vapor hydrated samples reflectivity measurements were taken without mechanical confinement (0 bar of confinement) before the membrane was inflated and then measurements were taken with 1, 3 and 5 bar applied [35–38]. With each confining stress increase, reflectometry measurements were repeated until the sample had equilibrated. A wide range in scattering vector Q was achieved using three different reflection angles between 0.4 and 2.8°. The size of the beam footprint on the sample was kept constant at 2.5 cm2 × 2.5 cm2 by adjusting the pre-sample slit sizes. Further details of the confinement cell, Melinex properties and control measurements indicating the efficiency of the apparatus for expelling D2O can be found reproduced in the Supporting Information (Supplementary Figures S2) and in our previous publication [29].
Model fitting: A layer model was used to fit the neutron reflectometry data with the main repeat unit of a water layer and a lipid layer. A parameter, Nk, was included to estimate the variation in the number of bilayers in the lipid stack was used. The full details of the model, how the final model was developed and the parameters used are provided in the supporting information.
Results
It has been shown that zwitterionic phosphatidylcholine (PC) lipids (>60% of all lipids) are a significant surface active (∼13%) constituent of synovial fluid [5, 23], forming a stack of approximately 3–7 bilayers at the cartilage surface [39]. We model these surface lipids using stacks of lipid bilayers with PC head groups and a range of saturated chain lengths from dilauryl (12:0) to distearyl (18:0) chains; the lipids are spin coated onto silicon substrates. These layers were hydrated within the confinement sample environment using D2O vapor and the neutron reflection measurements made using the D17 reflectometer (ILL, Grenoble) [34] uniaxial stress increasing from 1 to 5 bar (see [29], Supplementary Figures S1, Supporting Information and below for further experimental details).
Figure 1A shows the change in repeat spacing of the lipid bilayer stack (defined on the schematic in Figure 2), calculated from the Q position of the Bragg peak as an initial indication of the trends, as each sample was confined (data shown in Supporting Information, Supplementary Figures S4–S5). The 0 bar repeat distances were measured in situ prior to confinement at ∼98% humidity. The values are similar but predictably slightly lower than literature values for fully hydrated stacks [40–42]. In most cases, the lipid stacks follow the trend of reducing their repeat spacing in response to confinement, as expected due to a loss of water from between the bilayers. However, for the DPPC and DSPC samples heated to above their phase transition and hence in the Lα phase, the opposite trend was observed and their repeat spacing in fact increased significantly on confinement. Further, in both cases repeat distances converge towards the same values on confinement as they each had for the lower temperature despite the different starting phases.
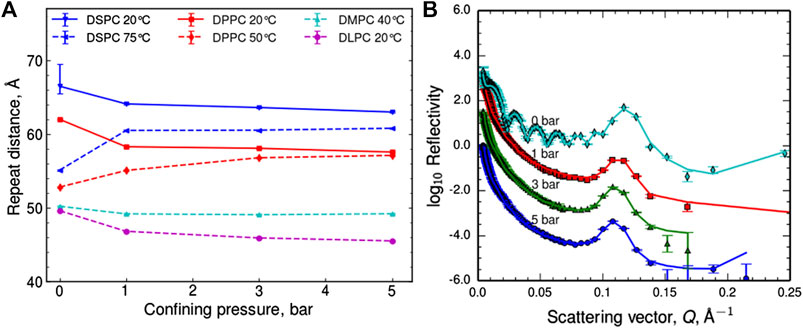
FIGURE 1. (A) Trends of the repeat distances in the lipid bilayer stacks, hydrated with D2O vapor taken from the positions of the Bragg peaks. Lines are to guide the eye only, with solid lines for temperatures below the expected phase transition to Lα and dashed lines for those above. (B) Neutron reflectometry profiles for DPPC hydrated with D2O vapor at 50°C under different applied uniaxial stresses, off-set for clarity, the lines represent model fits to the data.
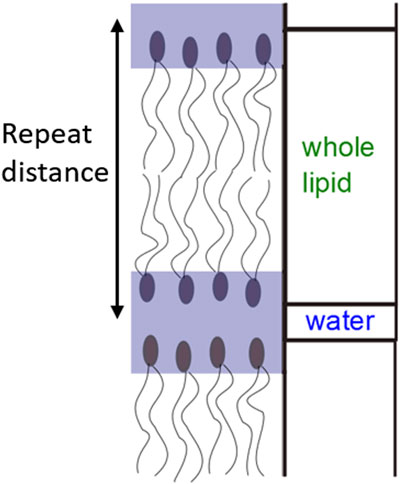
FIGURE 2. Schematic depicting the repeat distance and how the layer model for neutron reflectivity fitting was defined.
To extract further information from the neutron reflectivity curves, a layer model was used to fit the data. In the model chosen the lipid head and tail regions were combined into a layer with the water in between the lipids considered as a second layer so the repeat unit was one lipid bilayer and one water layer (Figure 2). Each lipid bilayer was defined by the same three parameters; thickness, neutron scattering length density (SLD) and roughness and the same three parameters were defined for the water layer between the lipid head groups. The outermost roughness and the variation in the exact number of bilayers across the surface of the sample were also taken into account during fitting (see also the schematic in Supporting Information, Supplementary Figures S3). Figure 1B shows this model fit to the DPPC at 50°C data set, full details of the model and parameters used to fit the data are given in the Supporting Information along with the complete data sets with corresponding fits (Supplementary Tables S1–S5, Supplementary Figures S4–S7).
The fitted thickness of these two layers as a function of confinement pressure can be seen for all of the samples in Figures 3A,B. This separation of the contributions to the overall repeat distance confirms that the water layer spacing is consistently reduced to 6 ± 2 Å after application of 1 bar confining pressure, independent of the lipid chain length or phase. This is consistent with a single solvation shell of water molecules closely associated with each PC head group [43], where 2.7 Å is the approximate diameter of a single water molecule [44] and also to the approach of protein solution scattering where proteins are modeled with a 3 Å bound water layer [45]. To ensure this phenomenon was not a function of hydrating the membranes using a humid atmosphere rather than bulk water, measurements on some lipid samples were repeated but hydrating with bulk water rather than water vapor (Supporting Information, Supplementary Figures S6). The water layer thickness also decreased to 6 ± 2 Å under 1 bar of confining pressure with no further loss of water at higher pressures, confirming that this was not due to limitations in the amount of available hydrating solvent.
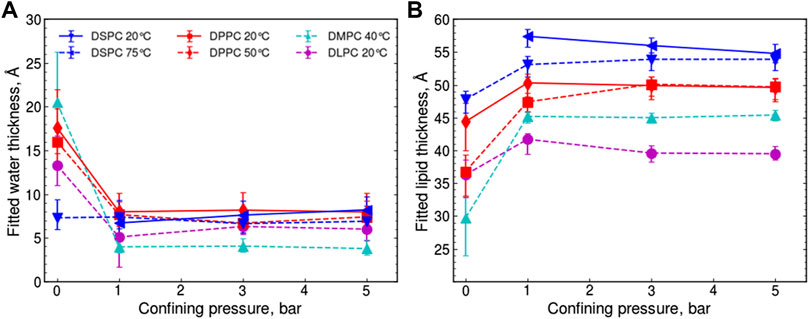
FIGURE 3. Trends of the fitted (A) water layer thickness and (B) lipid layer thickness in the lipid bilayer stacks, hydrated with D2O vapor, taken from the best model fits. Lines are to guide the eye only and the symbols define data for the same samples in both (A) and (B). Uncertainties are derived from fitting the data with two alternative scattering length densities for the D2O vapor which would not change within a series of confining pressures, meaning that the confidence in the relative positions of the points is greater than the error bars shown, this is discussed further in the Supporting Information.
In response to confinement by 1 bar, the thickness of the lipid bilayers (Figure 3B) increased for each lipid, independent of the tail length or starting phase, compensating (more or less) for the shrinking water layer. The same behavior was also seen for the fully hydrated samples (Supporting Information, Supplementary Figures S6). Further, the lipid bilayer thicknesses from the different starting phases converge at 3 bar for DPPC and 5 bar for DSPC suggesting that the same, incompressible, lipid state is accessed under confinement, irrespective of the starting phase. Thus, a direct correlation is observed between the amount of stress required to reach the incompressible confined state and the chain length of the lipid tails. It is possible that the response to confinement arises from the dehydration of the region between lipid bilayers, reducing the cross-sectional area per lipid headgroup and allowing closer packing of the lipid tail region resulting in a thickening, consistent with similar results seen for DLPC in X-ray diffraction studies [40].
Finally, while saturated PC lipids are the most prevalent constituent on the cartilage surface, a significant amount are also unsaturated, of which DOPC is present in the highest quantities [38, 46]. Therefore, for completion, these confinement experiments were repeated with stacks of DOPC bilayers hydrated with both vapor and bulk water. The analyzed data (Supporting Information, Supplementary Figures S5, S6) show the same features as in the saturated cases, despite the larger equilibrium area per molecule and relative disorder from the unsaturated double bonds on the lipids. The water spacing between bilayers reduces to ∼6 Å, but the water was not expelled entirely, and the thickness of the lipid region swells correspondingly under confinement, both reaching a plateau by 3 bar of applied uniaxial stress. The ratio between the fitted water and lipid layer thicknesses (Supporting Information, Supplementary Figures S8) also shows a similar trend for all of the vapor hydrated lipids except for the DMPC, which starts from a more hydrated state, and the DSPC at 75°C, which starts from a less hydrated state which may be attributable to its denser tail region. However it is interesting that the saturation of the lipid tail does not cause a dramatically different behavior.
Discussion
Here, we described the first direct structural study of lipid bilayers under confinement, providing an excellent model system for understanding the lipid lubricating properties of articulating cartilage. We propose that the low friction experienced between layers of stacked lipids as they slide past each other is due to the hydration lubrication mechanism, where a layer of water remains closely associated with a zwitterionic lipid head groups even at high pressures [47–51], in accordance with the Hills model [11, 12]. It should be noted that in this study the applied pressures were limited by our experimental setup to a maximum of 5 bar. Although a wide range of pressures occur at different points within a joint under various conditions starting from low pressures in the fluid [52, 53], like those probed here, and increasing to very high contact pressures (up to 200 bar) that can occur at contact points, locally within mammalian joints [54, 55]. In future experiments we hope to increase pressures to a range in line with the higher pressures that occur in vivo and particularly in diseased joints.
The results presented here show the first structural evidence to support our hypothesis, where a spacing approximately equivalent to one hydration layer of water molecules per lipid head group is maintained even at higher applied uniaxial stresses. SFA studies indicate that the lubrication behavior and frictional forces are maintained across a wide range of pressures [47, 48], and therefore the structures we measured at low levels of confinement remain comparable to those experienced locally at the cartilage surface [55].
Further, we have shown that the same lipid state corresponding to the lipid layer thickening is accessed under confinement, independent of the lipid tail saturation or starting lipid state. The thickening warrants further investigation but suggests a repacking of the lipid tail region due to the reduced cross-sectional area per lipid when the headgroups are partially dehydrated (as proposed in the illustration in Figure 4). The structure may be similar or identical to the sub gel phase [56, 57]. The results also offer an insight into the high mechanical robustness of the lipid bilayers. The results suggest that it is the access to a closer packed phase, with thicker layers for all lipids when confined, that increases the bilayer robustness. This lipid response is expected to improve their resistance to the high shear and compression forces experienced in human joints while still maintaining their hydrated lubricating properties. The water expelled during confinement may also play a role in the lubrication, synergistically with the transfer of fluid between the cartilage and synovial fluid which is believed to occur during joint lubrication [58]. Understanding of the hydration and structural changes of the PC lipids under uniaxial stress is the first step toward fully understanding how their structure is changed in dynamic, lubricating joints and how the act to improve the lubricating properties of synovial fluid. The composition of the synovial fluid changes with diseases such as osteoarthritis and DPPC has also been shown to reduce the friction coefficient and therefore is a prospective treatment for damaged joints [59, 60].
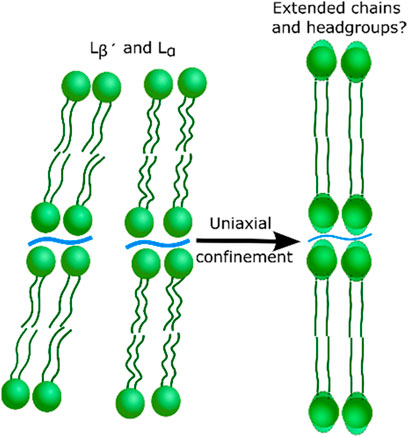
FIGURE 4. Cartoon to illustrate the possible re-ordering of the lipid bilayers indicated by the confinement of lipid stacks.
To conclude, we present the first measurements of the structures of lipid bilayers with a simultaneously applied uniaxial stress. The structural properties have been observed directly using neutron reflectometry, thus demonstrating that the apparatus used can provide extensive insight into the structural behavior of such biological systems. Specifically, in this study we have provided direct support for the mechanism of hydration lubrication previously hypothesized on the basis of indirect measurements.
Data Availability Statement
The original contributions presented in the study are included in the article/Supplementary Material and as raw data from the ILL DOIs referenced 35–37, further inquiries can be directed to the corresponding author.
Author Contributions
LM: Conceptualization, Data curation, Investigation, Methodology, Original draft. SA: Investigation, Review and; editing. RB: Conceptualization, Investigation, Methodology, Review and; editing. WV: Conceptualization, Data curation, Investigation, Methodology, Review and; editing. SP: Conceptualization, Investigation, Methodology, Review and; editing, Supervision, Funding acquisition. RR: Conceptualization, Investigation, Project administration, Methodology, Supervision, Review and; editing, Funding acquisition.
Conflict of Interest
The authors declare that the research was conducted in the absence of any commercial or financial relationships that could be construed as a potential conflict of interest.
Publisher’s Note
All claims expressed in this article are solely those of the authors and do not necessarily represent those of their affiliated organizations, or those of the publisher, the editors and the reviewers. Any product that may be evaluated in this article, or claim that may be made by its manufacturer, is not guaranteed or endorsed by the publisher.
Acknowledgments
The authors gratefully acknowledge the superb work of Charlie Murray, from the Chemistry workshop and Richard Exley, Adrian Crimp, Tim Newbury and Gideon Hugo from the Physics workshop at the University of Bristol who created our sample environment. In addition, we would also like to extend our thanks to ILL, Grenoble, France, for allocating beam time for these experiments 9-13-375, 9-13-454 and 9-13-496,35–37 as well as the funding provided by EPSRC under Grant EP/H0148611. The authors acknowledge the TU Wien Bibliothek for financial support through its Open Access Funding Program.
Supplementary Material
The Supplementary Material for this article can be found online at: https://www.frontiersin.org/articles/10.3389/fphy.2021.703472/full#supplementary-material
References
1. Spencer ND. Aqueous Lubrication: Natural and Biomimetic Approaches. Singapore: World Scientific Publishing Co Pte Ltd. (2014). doi:10.1142/7818
2. Urbakh M, Klafter J, Gourdon D, Israelachvili J. The Nonlinear Nature of Friction. Nature (2004) 430(6999):525–8. doi:10.1038/nature02750
3. Chang DP, Abu-Lail NI, Coles JM, Guilak F, Jay GD, Zauscher S. Friction Force Microscopy of Lubricin and Hyaluronic Acid between Hydrophobic and Hydrophilic Surfaces. Soft Matter (2009) 5(18):3438–45. doi:10.1039/b907155e
4. Jay GD, Waller KA. The Biology of Lubricin: Near Frictionless Joint Motion. Matrix Biol (2014) 39:17–24. doi:10.1016/j.matbio.2014.08.008
5. Ye H, Han M, Huang R, Schmidt TA, Qi W, He Z, et al. Interactions between Lubricin and Hyaluronic Acid Synergistically Enhance Antiadhesive Properties. ACS Appl Mater Inter (2019) 11(20):18090–102. doi:10.1021/acsami.9b01493
6. Seror J, Zhu L, Goldberg R, Day AJ, Klein J. Supramolecular Synergy in the Boundary Lubrication of Synovial Joints. Nat Commun (2015) 6. doi:10.1038/ncomms7497
7. Yu J, Banquy X, Greene GW, Lowrey DD, Israelachvili JN. The Boundary Lubrication of Chemically Grafted and Cross-Linked Hyaluronic Acid in Phosphate Buffered Saline and Lipid Solutions Measured by the Surface Forces Apparatus. Langmuir (2012) 28(4):2244–50. doi:10.1021/la203851w
8. Schroeder A, Verberne G, Merkher Y, Diminsky D, Maroudas A, Halperin G, et al. Surface Active Phospholipids as Cartilage Lubricants. In: Proceedings of the ASME 2008 9th Biennial Conference on Engineering Systems Design and Analysis; 2008 Jul 7–9; Haifa, Israel. Vol. 3, Design; Tribology; Education. ASME (2008). p. 549–53. doi:10.1115/ESDA2008-59523
9. Hills BA. Oligolamellar Lubrication of Joints by Surface Active Phospholipid. J Rheumatol (1989) 16(1):82–91.
10. Hills BA, Monds MK. Deficiency of Lubricating Surfactant Lining the Articular Surfaces of Replaced Hips and Knees. Rheumatology (1998) 37(2):143–7. doi:10.1093/rheumatology/37.2.143
11. Hills BA. Boundary Lubrication In Vivo. Proc Inst Mech Eng H (2000) 214:83–94. doi:10.1243/0954411001535264
12. Schwarz IM, Hills BA. Surface-Active Phospholipid as the Lubricating Component of Lubricin. Rheumatology (1998) 37(1):21–6. doi:10.1093/rheumatology/37.1.21
13. Hills BA. Surface-active Phospholipid: a Pandora's Box of Clinical Applications. Part II. Barrier and Lubricating Properties. Intern Med J (2002) 32(5–6):242–51. doi:10.1046/j.1445-5994.2002.00201.x
14. Orozco-Alcaraz R, Kuhl TL. Interaction Forces between DPPC Bilayers on Glass. Langmuir (2013) 29(1):337–43. doi:10.1021/la3039329
15. Helm CA, Israelachvili JN, McGuiggan PM. Role of Hydrophobic Forces in Bilayer Adhesion and Fusion. Biochemistry (1992) 31(6):1794–805. doi:10.1021/bi00121a030
16. Marra J, Israelachvili J. Direct Measurements of Forces between Phosphatidylcholine and Phosphatidylethanolamine Bilayers in Aqueous Electrolyte Solutions. Biochemistry (1985) 24(17):4608–18. doi:10.1021/bi00338a020
17. Bilotto P, Lengauer M, Andersson J, Ramach U, Mears LLE, Valtiner M. Interaction Profiles and Stability of Rigid and Polymer-Tethered Lipid Bilayer Models at Highly Charged and Highly Adhesive Contacts. Langmuir (2019) 35(48):15552–63. doi:10.1021/acs.langmuir.9b01942
18. Israelachvili J, Min Y, Akbulut M, Alig A, Carver G, Greene W, et al. Recent Advances in the Surface Forces Apparatus (SFA) Technique. Rep Prog. Phys. (2010) 73(3). doi:10.1088/0034-4885/73/3/036601
19. Schwenzfeier KA, Erbe A, Bilotto P, Lengauer M, Merola C, Cheng HW, et al. Optimizing Multiple Beam Interferometry in the Surface Forces Apparatus: Novel Optics, Reflection Mode Modeling, Metal Layer Thicknesses, Birefringence, and Rotation of Anisotropic Layers. Rev Sci Instrum (2019) 90(4). doi:10.1063/1.5085210
20. Briscoe WH. Aqueous Boundary Lubrication: Molecular Mechanisms, Design Strategy, and Terra Incognita. Curr Opin Colloid Interf Sci (2017) 27:1–8. doi:10.1016/j.cocis.2016.09.002
21. Rawicz W, Olbrich KC, McIntosh T, Needham D, Evans E. Effect of Chain Length and Unsaturation on Elasticity of Lipid Bilayers. Biophysical J (2000) 79(1):328–39. doi:10.1016/s0006-3495(00)76295-3
22. Leite Rubim R, Gerbelli BB, Bougis K, Pinto de Oliveira CL, Navailles L, Nallet F, et al. Water Activity in Lamellar Stacks of Lipid Bilayers: “Hydration Forces” Revisited. Eur Phys J E (2016) 39(1):1–11. doi:10.1140/epje/i2016-16003-0
23. Bougis K, Leite Rubim R, Ziane N, Peyencet J, Bentaleb A, Février A, et al. Stabilising Lamellar Stacks of Lipid Bilayers with Soft Confinement and Steric Effects. Eur Phys J E (2015) 38(7). doi:10.1140/epje/i2015-15078-3
24. Kanduč M, Schneck E, Netz RR. Hydration Interaction between Phospholipid Membranes: Insight into Different Measurement Ensembles from Atomistic Molecular Dynamics Simulations. Langmuir (2013) 29(29):9126–37. doi:10.1021/la401147b
25. Kanduč M, Schlaich A, Vries AHD, Jouhet J, Mare E, Deme B, et al. Tight Cohesion between Glycolipid Water – Headgroup Interactions. Nat Commun (2017) 8. doi:10.1038/ncomms14899
26. Domenici F, Castellano C, Congiu a., Pompeo G, Felici R. Ordering and Lyotropic Behavior of a Silicon-Supported Cationic and Neutral Lipid System Studied by Neutron Reflectivity. Appl Phys Lett (2008) 92(19):193901. doi:10.1063/1.2917807
27. Junghans A, Watkins EB, Barker RD, Singh S, Waltman MJ, Smith HL, et al. Analysis of Biosurfaces by Neutron Reflectometry: From Simple to Complex Interfaces. Biointerphases (2015) 10(1):019014. doi:10.1116/1.4914948
28. Wei Z, Prescott SW. Scattering Approaches to Probing Surface Layers under Confinement. Curr Opin Colloid Interf Sci (2015) 20(4):253–60. doi:10.1016/j.cocis.2015.09.001
29. de Vos WM, Mears LLE, Richardson RM, Cosgrove T, Dalgliesh RM, Prescott SW. Measuring the Structure of Thin Soft Matter Films under Confinement: A Surface-Force Type Apparatus for Neutron Reflection, Based on a Flexible Membrane Approach. Rev Scientific Instr (2012) 83(11):113903. doi:10.1063/1.4767238
30. de Vos WM, Mears LLE, Richardson RM, Cosgrove T, Barker R, Prescott SW. Nonuniform Hydration and Odd-Even Effects in Polyelectrolyte Multilayers under a Confining Pressure. Macromolecules (2013) 46:1027–34. doi:10.1021/ma3021773
31. Abbott SB, de Vos WM, Mears LLE, Barker R, Richardson RM, Prescott SW. Hydration of Odd-Even Terminated Polyelectrolyte Multilayers under Mechanical Confinement. Macromolecules (2014) 47:3263–73. doi:10.1021/ma500557m
32. Abbott SB, de Vos WM, Mears LLE, Cattoz B, Skoda MWA, Barker R, et al. Is Osmotic Pressure Relevant in the Mechanical Confinement of a Polymer Brush? Macromolecules (2015) 48(7):2224–34. doi:10.1021/ma502246r
33. Silvius JR. Thermotropic Phase Transitions of Pure Lipids in Model Membranes and Their Modifications by Membrane Proteins In: Lipid Protein Interactions. New York: John Wiley & Sons (1982).
34. Cubitt R, Fragneto G. D17: the New Reflectometer at the ILL. Appl Phys A: Mater Sci Process (2002) 74:S329–S331. doi:10.1007/s003390201611
35. De Vos W, Abbott S, Barker R, Cosgrove T, Mears L, Prescott S, et al. Confinement Induced Phase Transitions in Lipid Bi-layers. Grenoble: Institut Laue-Langevin (ILL) (2012). doi:10.5291/ILL-DATA.9-13-454
36. Prescott S, Abbott S, Barker R, Cosgrove T, De Vos W, Mears L, et al. Detailed Hydration and Temperature Study of Phosphocholine Lipids. Grenoble: Institut Laue-Langevin (ILL) (2013). doi:10.5291/ILL-DATA.9-13-518
37. Richardson RM, Abbott S, Barker R, Cosgrove T, De Vos W, Mears L, et al. Confinement Effects on Lipids with Charged Headgroups. Grenoble: Institut Laue-Langevin (ILL) (2013). doi:10.5291/ILL-DATA.9-13-496
38. Kosinska MK, Ludwig TE, Liebisch G, Zhang R, Siebert HC, Wilhelm J, et al. Articular Joint Lubricants during Osteoarthritis and Rheumatoid Arthritis Display Altered Levels and Molecular Species. PLoS One (2015) 10(5):1–18. doi:10.1371/journal.pone.0125192
39. Sarma AV, Powell GL, LaBerge M. Phospholipid Composition of Articular Cartilage Boundary Lubricant. J Orthop Res (2001) 19(4):671–6. doi:10.1016/s0736-0266(00)00064-4
40. Chen F, Hung W. Structural Changes of Lipid Membrane Induced by Dehydration. Chin J Phys (1996) 34(6):1363–72.
41. Katsaras J. Highly Aligned Lipid Membrane Systems in the Physiologically Relevant “Excess Water” Condition. Biophysical J (1997) 73(6):2924–9. doi:10.1016/s0006-3495(97)78320-6
42. Cavalcanti LP, Haas H, Bordallo HN, Konovalov O, Gutberlet T, Fragneto G. In Situ X-Ray and Neutron Diffraction Study of Lipid Membrane Swelling. Eur Phys J Spec Top (2007) 141(1):217–21. doi:10.1140/epjst/e2007-00043-9
43. Perera L, Essmann U, Berkowitz ML. Role of Water in the Hydration Force Acting between Lipid Bilayers. Langmuir (1996) 12(11):2625–9. doi:10.1021/la9515534
44. Zhang Y, Xu Z. Atomic Radii of Noble Gas Elements in Condensed Phases. Am Mineral (1995) 80(7–8):670–5. doi:10.2138/am-1995-7-803
45. Svergun D, Barberato C, Koch MHJ. CRYSOL- a Program to Evaluate X-ray Solution Scattering of Biological Macromolecules from Atomic Coordinates. J Appl Cryst (1995) 28(6):768–73. doi:10.1107/s0021889895007047
46. Cleland KA, James MJ, Neumann MA, Gibson RA, Cleland LG. Differences in Fatty Acid Composition of Immature and Mature Articular Cartilage in Humans and Sheep. Lipids (1996) 30(10):949–53. doi:10.1007/BF02537487
47. Goldberg R, Schroeder A, Silbert G, Turjeman K, Barenholz Y, Klein J. Boundary Lubricants with Exceptionally Low Friction Coefficients Based on 2D Close-Packed Phosphatidylcholine Liposomes. Adv Mater (2011) 23(31):3517–21. doi:10.1002/adma.201101053
48. Goldberg R, Schroeder A, Barenholz Y, Klein J. Interactions between Adsorbed Hydrogenated Soy Phosphatidylcholine (HSPC) Vesicles at Physiologically High Pressures and Salt Concentrations. Biophysical J (2011) 100(10):2403–11. doi:10.1016/j.bpj.2011.03.061
49. Raviv U, Klein J. Fluidity of Bound Hydration Layers. Science (80) (2002) 297:1540–3. doi:10.1126/science.1074481
50. Trunfio-Sfarghiu AM, Berthier Y, Meurisse MH, Rieu JP. Role of Nanomechanical Properties in the Tribological Performance of Phospholipid Biomimetic Surfaces. Langmuir (2008) 24(16):8765–71. doi:10.1021/la8005234
51. Dekkiche F, Corneci MC, Trunfio-Sfarghiu AM, Munteanu B, Berthier Y, Kaabar W, et al. Stability and Tribological Performances of Fluid Phospholipid Bilayers: Effect of Buffer and Ions. Colloids Surf B: Biointerfaces (2010) 80(2):232–9. doi:10.1016/j.colsurfb.2010.06.012
52. Gu KB, Li LP. A Human Knee Joint Model Considering Fluid Pressure and Fiber Orientation in Cartilages and Menisci. Med Eng Phys (2011) 33:497–503. doi:10.1016/j.medengphy.2010.12.001
54. Yoshida H, Faust A, Wilckens J, Kitagawa M, Fetto J, Chao EY-S. Three-Dimensional Dynamic Hip Contact Area and Pressure Distribution during Activities of Daily Living. J Biomech (2006) 39:1996–2004. doi:10.1016/j.jbiomech.2005.06.026
55. Hodge WA, Fijan RS, Carlson KL, Burgess RG, Harris WH, Mann RW. Contact Pressures in the Human Hip Joint Measured In Vivo. Proc Natl Acad Sci (1986) 83(9):2879–83. doi:10.1073/pnas.83.9.2879
56. McIntosh TJ, Simon SA. Contributions of Hydration and Steric (Entropic) Pressures to the Interactions between Phosphatidylcholine Bilayers: Experiments with the Subgel Phase. Biochemistry (1993) 32(32):8374–84. doi:10.1021/bi00083a042
57. Kranenburg M, Smit B. Phase Behavior of Model Lipid Bilayers†. J Phys Chem B (2005) 109(14):6553–63. doi:10.1021/jp0457646
58. Ermakov S, Beletskii A, Eismont O, Nikolaev V. Liquid Crystals in Biotribology. Switzerland: Springer (2016). doi:10.1007/978-3-319-20349-2
59. Ozturk HE, Stoffel KK, Jones CF, Stachowiak GW. The Effect of Surface-Active Phospholipids on the Lubrication of Osteoarthritic Sheep Knee Joints: Friction. Tribology Lett (2004) 16(4):283–9. doi:10.1023/b:tril.0000015204.41674.d3
Keywords: phosphatidylcholine, neutron reflectometry, compression, dehydration, phase change, lubrication
Citation: Mears LLE, Abbott SB, Barker RD, de Vos WM, Prescott SW and Richardson RM (2021) Structural Evidence for a Reinforcing Response and Retention of Hydration During Confinement of Cartilage Lipids. Front. Phys. 9:703472. doi: 10.3389/fphy.2021.703472
Received: 06 May 2021; Accepted: 22 July 2021;
Published: 03 August 2021.
Edited by:
Xuejin Li, Zhejiang University, ChinaReviewed by:
Nir Kampf, Weizmann Institute of Science, IsraelSobhan Sen, Jawaharlal Nehru University, India
Copyright © 2021 Mears, Abbott, Barker, de Vos, Prescott and Richardson. This is an open-access article distributed under the terms of the Creative Commons Attribution License (CC BY). The use, distribution or reproduction in other forums is permitted, provided the original author(s) and the copyright owner(s) are credited and that the original publication in this journal is cited, in accordance with accepted academic practice. No use, distribution or reproduction is permitted which does not comply with these terms.
*Correspondence: Laura L. E. Mears, bWVhcnNAaWFwLnR1d2llbi5hYy5hdA==
†Present addresses: Laura L. E. Mears, Institute for Applied Physics, Vienna University of Technology, Vienna, AustriaRobert D. Barker, School of Physical Sciences, University of Kent, Canterbury, United Kingdom