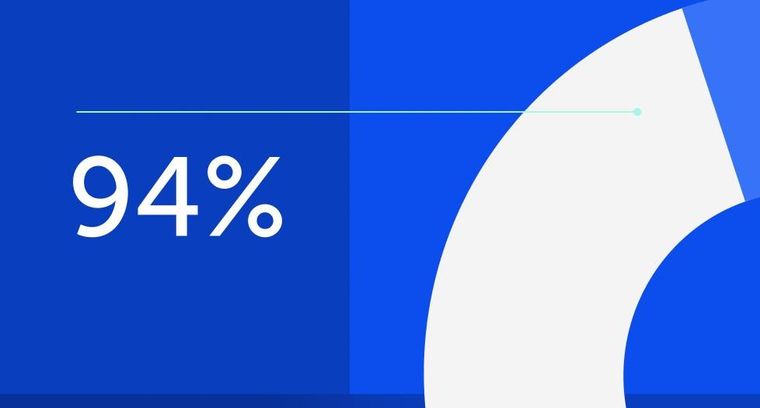
94% of researchers rate our articles as excellent or good
Learn more about the work of our research integrity team to safeguard the quality of each article we publish.
Find out more
REVIEW article
Front. Phys., 26 May 2021
Sec. Low-Temperature Plasma Physics
Volume 9 - 2021 | https://doi.org/10.3389/fphy.2021.677971
This article is part of the Research TopicLow-Temperature Plasma for Biomedical ApplicationsView all 10 articles
The efficiency of plasmas sources for the decontamination of heat-sensitive devices has been proven for more than 20 years, but commercial plasma-based sterilizers still have a narrow range of applications. This can be partially explained by difficulties to determine reliable bio-indicators and standardized microbiological test procedures required by industrial uses. In this paper, we examine the influence of environmental factors on the inactivation rate of microorganisms deposited on surfaces and treated by plasma sources. In addition, we present a literature review showing that several in-discharge and afterglow plasma sterilizers offer shorter treatment times than conventional low-temperature sterilizers to reduce the concentration of endospores on contaminated surfaces by 6-log. Finally we make a few recommendations for future plasma decontamination standards.
Low-temperature decontamination methods are increasingly used for the treatment of heat-sensitive materials in devices exposed to biohazards, such as endoscopes in hospitals [1], containers in the food industry [2], spacecraft components [3] or contaminated equipment in areas exposed to war acts [4]. Standard non-thermal sterilization methods suffer from limitations related to their toxicity, high cost, low material compatibility, and/or long sterilization cycles (several hours). An alternative approach consists in using atmospheric pressure nonequilibrium plasmas produced by electric discharges. The use of plasma sources for low-temperature decontamination has been widely studied for more than 20 years [5–7]. Various biocidal mechanisms of plasmas have been demonstrated, including cell lysis induced by charged species [8, 9], DNA inactivation by radiation from excited molecules and atoms [8, 10], and alteration of cell components by chemical products such as reactive oxygen and nitrogen species (RONS). This combination of inactivation mechanisms is attractive because a broad range of microorganisms can be treated, namely bacteria, viruses [11] including Sars-CoV2 [12], fungi [13, 14] and prions [15]. However, the intensities of the mentioned biocidal mechanisms strongly depend on the type of plasma source (discharge kind, gas flow, power,…), working distance between the plasma and the contaminated surface, and operating conditions (surface nature, humidity,…). Therefore, it is difficult to compare decontamination results using plasma sources because the inactivation results are highly dependent on the operating conditions. Comparing the results requires to define standard microbiological test conditions, and thus the major parameters that need to be controlled.
The present paper first aims to identify the influence of several environmental parameters that are not systematically mentioned in publications. Second, the decontamination efficiency of various plasma sources is assessed on the basis of the treatment time required to obtain a 6-log reduction of endospores, which are commonly used as bio-indicators (BI) to validate the efficiency of sterilizers. These results are classified according to the gas pressure and to the working distance between the plasma and the treated sample because the biocidal effects of plasmas are reduced as the working distance increases. In addition, the working distance is an important parameter for certain applications, particularly the decontamination of long tubes, where sensitive surfaces cannot be treated directly by the plasma. The observed trends are discussed and compared with inactivation results obtained against the same microorganisms using standard low-temperature sterilizers.
The paper is organized as follows. The first part introduces the definitions of the microbiological quantities used in the article, namely log-reduction, D-value, and bio-indicators. The second part shows the influence of various environmental factors on the inactivation of microorganisms treated by plasma sources or by chemicals. The third part compares and discusses literature results on the basis of the key environmental factors introduced in part 2.
The efficiency of decontamination methods is usually assessed by direct cell-counting methods that are based on the following procedure. First, a suspension of microorganisms is prepared, generally in sterile water. The concentration of the suspension is adjusted by subsequent dilution. Second, a fraction of the suspension is deposited onto two carriers and dried. In general, about ∼106 microorganisms are deposited on the carriers so as to follow ISO 11138 series and ISO 14161 standards. Third, one of the carriers is treated while the other is used as a control. Fourth, the microorganisms are extracted from the carriers and counted using a plate count method. To this end, the suspension of recovered microorganisms is diluted, spread on plates, and incubated. The number of Colony Forming Units (CFU) on the plate is finally counted. If N is the number of CFU after the decontamination treatment, and N0 the number of CFU on the control sample, the log-reduction factor (RF) is defined as follows:
For an initial load of 106 microorganisms on a surface, the maximum reduction factor measurable by direct cell counting is 6-log. On the basis of a statistical analysis, a reduction factor as low as 8-log can be determined by a fraction negative method [16].
The ISO 11138 series and ISO 14161 standards define sterility as a Sterility Assurance Level (SAL) of 10-6. The SAL denotes the probability that only one viable microorganism survives the treatment. Sterility cannot be verified in practice but can be extrapolated from the kinetics of inactivation of the most resistant microorganism, named the bio-indicator (BI). Table 1 lists some of the commonly used bio-indicators. It should also be noted that other standards, e.g. EN 13697, are used in industrial applications for disinfection purposes.
TABLE 1. Standard sterilization methods and microorganisms commonly used as bio-indicators for these methods, modified from [6].
Figure 1 shows the inactivation kinetics on a semi-logarithmic scale, and the methods used to analyze it. Direct cell counting methods are based on measurings the number of surviving microorganisms. Fraction negative methods, e.g. Stumbo-Murphy-Cochran method and Spearman-Karber method, are statistical techniques applied in the region of the inactivation curve where only a fraction of the treated items have no viable microorganisms after the treatment, i.e. the so-called quantal zone. The technique allows to demonstrate SAL up to 10–2 and to determine the D-value from a reduced range of the inactivation curve. Demonstrating lower SAL (SAL < 10–2) is difficult because of the large number of tests required, so extrapolation of the inactivation curve is necessary.
For sterilization methods such as steam heat, the inactivation kinetics follow an ideal exponential decay, and the inactivation curve can be easily extrapolated to determine the conditions for which sterility can be guaranteed. The slope of the semi-logarithmic line is termed the D-value and is widely used to assess the efficiency of a sterilization process. It corresponds to the time required to reduce the population of one type of microorganism by one order of magnitude.
In the case of non-thermal decontamination, a multi-step decay of microorganisms is regularly reported, e.g. [17–20]. The multi-step decay is generally attributed to a clumping of microorganisms that reduces the number of microorganisms attainable by the antimicrobial agents [5, 21] or to space-varying mechanisms of inactivation [16, 22] possibly related to an inhomogeneous distribution of the plasma agents across the test sample. Such multiphase decay prevents the determination of accurate D-values and thus does not allow plasma decontamination methods to reach the SAL of 10-6 required for sterilization. However, appropriate preparation and deposition of bioindicators may allow to observe a monophasic inactivation curve.
In this section, we review preparation methods and operating conditions that can significantly modify the inactivation results of plasma decontamination processes.
The initial suspension of microorganisms is generally prepared in sterile water. However, in realistic conditions, the bioburden is surrounded by organic and inorganic matter (soil). The soil can be well-removed by a preliminary cleaning using water (lowest bio-decontamination level) according to the guidelines of Rutala et al. [23]. However inorganic residues may still persist after cleaning. These residues can be simulated in microbiological treatments by adding salt or serum to the initial suspension of microorganisms. Such additives are known to strongly reduce the efficiency of most decontamination methods [24]. In particular, it has been shown that a high concentration of crystalline-type materials provides a greater protection to spores than serum with high protein content [23]. Using a surface microdischarge (SMD), Klämpfl et al. [25] observed by scanning electron microscopy (SEM) that Clostridium Difficile (NCTC 13366) endospores prepared with 0.03% BSA (bovine serum albumine), deposited and dried on stainless steel substrates, form clusters with surrounding saline structures. This barrier decreases the number of spores attainable by plasma species. As a result, the inactivation rate was lowered by 3-log when BSA was added to the suspension. Thus the inactivation results are highly sensitive to the method of preparation, which must be specified to make useful comparisons between decontamination systems.
Two main techniques are commonly used to deposit microorganisms on substrates : the spot and spray methods. The spot technique is more common and consists in placing a droplet of the prepared suspension of microbes on the inoculation surface. This method does not allow an accurate control of the local concentration of microorganisms, which readily form clusters and multilayered structures. In contrast, the spray method allows to deposit a monolayer of microorganisms onto the surface, thus ensuring a homogeneous and controlable surface concentration of microorganisms [26–28]. For this reason, the spray deposition method is interesting for standardized inoculation on substrates(29). Raguse et al. [29] compared the reduction factor for 5×107B. Subtilis spores deposited by spray and liquid spot methods on a glass substrate exposed to a low-pressure argon plasma. After 90 s of exposure, a 0.8-log reduction was measured on the substrate inoculated using the spot method while a 4.8-log-reduction was achieved using the spray method. The slower inactivation observed using the spot method can be attributed to the slower diffusion of germicidal agents inside the multi-layered cell structures. According to Shintani et al. [21], [30], the characteristic penetration depth of plasma species is about ∼10 nm, and ∼1 µm for hydrogen peroxide. Since, the dimension of a microbe is typically ∼1 µm, chemical sterilants readily diffuse deeply through multiple layers of cells while plasma species only access the first layer.
As a result, the method of microorganism deposition strongly influences the decontamination efficiency of non-penetrating surface agents such as plasma species, but also UV radiation [31]. However, Raguse et al. [29] showed that highly-penetrating agents such as X-rays were not influenced by the deposition technique.
The surface concentration of microorganisms is defined as the ratio of the initial microorganisms load to the inoculation area. Increasing the surface concentration of microorganisms enhances the formation of multi-layered structures preventing the diffusion of plasma species in the case of microorganisms deposited by a spot method [32]. Thus, decreasing the initial load of microorganisms or increasing the inoculation area may lead to higher inactivation rates. In Ref. [33], different dilutions of E. Coli on Agar were treated by surface microdischarges (SMD). A difference of 4-log in the initial load caused a difference of about 1 log in the inactivation rate after 10 s of treatment. A similar behaviour was observed in Ref. [34] with a corona discharge.
Variations in the strains of a single microorganism species are referenced in collections –e.g. American Type Culture Collection (ATCC), National Collection of Type Culture (NCTC). The susceptibility of different strains of E. Coli (ATCC 25922 and NCTC 12900) to air DBD exposure was studied in Ref. [35]. After 30 sec of air plasma treatment, it was shown that the strain ATCC 25922 was reduced by 3.4 log whereas the strain NCTC 12900 (corresponding to collection n° ATCC 700728) was only reduced by 1.8 log. The different efficiencies are due to intrinsinc DNA variations within the same type of microorganism. It is important to note also that the name of microorganisms is subject to adjustments. Table 2 gives the correspondance of DSM (German Collection of Microorganisms and Cell Cultures) collection numbers with other collection numbers of some bacillus strains of bacteria, as well as previous designations that may also be encountered. It should also be noted that microorganisms available from culture collections are typically less resistant than their real-world counterparts, because the latter have been subjected to environmental stresses and have evolved to adapt over countless generations.
TABLE 2. Different names of some bacillus strains of bacteria (after [63])
High relative humidity (RH) is known to cause the swelling of endospores [36], which enhances the efficiency of many decontamination methods –e.g. EtO and formaldehyde [37] – because of the higher water content in the spore core allowing the formation of aggressive chemicals, such as OH, inside the spore. In Ref. [37], the moisture content (hydration) of B. Subtilis was measured for different RH. It was shown that the moisture content slightly increases from 0 to 20% when the RH is varied from 0 to 75%, and strongly increases at higher RH (from 20 to 70% between 75 and 95% RH). At RH > 75 %, the strong increase of the water content causes spore swelling.
The relative humidity (RH) also affects the gas-phase plasma chemistry [36]. Figure 2 shows the influence of the RH on the spore moisture content and the log-reduction of spores treated by atmospheric cold plasma (ACP) [38] and dielectric barrier discharge (DBD) [39]. The results indicate that a higher RH is always correlated with higher sporicidal activity, probably because of the formation of water-related biocides, such as OH, H2O2, HNO2, HNO3. Similar conclusions were obtained in Ref. [26]
FIGURE 2. Effect of air relative humidity on (A) the moisture content of B. Subtilis in contact with air at 25°C estimated from water desorption of wet cells and water adsorption of dessicated cells [37], and (B) the inactivation of B. Atrophaeus endospore by direct (∆) and post-discharge (□) DBD treatment [38], and surface DBD (SDBD) after exposures of 150 s (□) and 450 s (∆), [39].
The effect of the material and the structure of the treated surface on the antimicrobial agent efficacy is complex. Sigwarth et al. [40] studied the reduction of G. Stearothermophilus (ATCC 7953) endospores deposited on various surface materials after exposure to hydrogen peroxide. Up to a 3-log difference in the reduction was measured on different materials, but the authors saw no clear correlation between the material properties and the shift in the resistance of endospores.
Figure 3 shows the inactivation of endospores B. Anthracis, B. Subtilis and G. Stearothermophilus on different substrates exposed to formaldehyde and hydrogen peroxide [41, 42]. With formaldehyde, the inactivation results are fairly similar for all materials. In contrast, for hydrogen peroxide exposure, the inactivation results strongly depend on the substrate material as shown in Ref. [40]. These results suggest that endospores are less likely inactivated by H2O2 when they are deposited on porous materials. According to the authors, a possible penetration of spores inside porous substrates precludes interaction of H2O2 with a significant fraction of spores because of the comparatively small penetration depth of H2O2 [23].
FIGURE 3. Inactivation of B. Anthracis (black), B. Subtilis (red) and G. Stearothermophilus (blue) endospores dried on various surface materials and exposed to hydrogen peroxide H2O2(top graph) and gaseous formaldehyde CH2O (bottom), adapted from [41, 42].
The effect of material permeability can be further understood from the work of Mahfoudh et al. [36] who studied the effect of dry gaseous ozone on the inactivation of endospores deposited on different polymeric surfaces. Figure 4 shows the results obtained by exposing the samples contaminated by B. Atrophaeus to 4000 ppm of dry ozone for 1 hour. The log-reductions are shown together with the permeability coefficients of the materials studied (values taken from GoodFellow). The results show a correlation between the permeability of the surface material and the achieved inactivation rate with a maximum 4.6-log reduction obtained on a highly permeable silicone surface. Similar results were obtained in decontamination using ethylene oxide (EtO) in Ref. [37].
FIGURE 4. Effect of exposure to dry gaseous ozone (4000 ppm, 60 min, RH < 2 %) on the inactivation of B. Atrophaeus endospores dried on pyrex glass and polymeric surfaces (top, modified from [36]), and permeability coefficients (units: 10-13 cm3.cm.cm-2.s-1.Pa-1) of treated materials (bottom, data taken from GoodFellow).
In direct plasma decontamination, additional interactions between the plasma and the surfaces increase the influence of the surface material. Interactions between the surface material and the gas phase species were observed by Levif et al. [43]. Operating at reduced pressure, the authors demonstrated that B. Atrophaeus spores deposited on polystyrene petri dishes were more resistant than on a glass surface, because of the interaction between the plasma and the surface material. As a result, treating microorganisms on porous surfaces significantly increases the inactivation rate. A possible reason for this is the penetration of spores inside the material, thus preventing clumping and shielding of microorganisms at the surface. In the case of direct plasma treatment, interaction of the plasma with the surface material influences the gas phase chemistry and thus the inactivation mechanisms.
In several demonstrations of plasma treatments of spores, the D-values could not be determined. In these cases, the inactivation curve cannot be extrapolated and the sterility cannot be guaranteed according to the sterility assurance level criteria (SAL of 10–6). Rather than speaking of “sterilization”, von Woedtke et al. [16] proposed the microbiological safety qualification “proof of antimicrobial efficiency on the highest experimentally accessible level” as the highest possible qualification for plasma decontamination. Here we report the experimental results for which a 6-log reduction of bacterial spores (most common BIs) dried on surfaces was demonstrated according to the von Woedtke definition. The time required to ensure a 6-log reduction of BIs is noted T6log.
Figure 5 shows an overview of spore inactivation results on dried surfaces by atmospheric and reduced pressure plasmas, as a function of the distance between the plasma source and the sample. Figure 5 also shows typical T6log values for standard decontamination methods. We only plot the experimental results for which a 6-log reduction of spores was measured or extrapolated from a single D-value. The experimental conditions of the reported results are detailed in Supplementary material (Supplementary table S1, S2) and in the cited references. An additional table (Supplementary table S3) provides a review of the experimental results that could not be included in Figure 5.
FIGURE 5. Exposure time T6log required to achieve at least a 6-log reduction of various types of spores on dried surface using different methods. (A) direct plasma exposure with DBD (Mur. [26, 49], Ven. [44], Aki. [45], Pat [38]) microwave plasma (Half. [27], Sta. [50], Nag [51]) (B) post-discharge exposure as a function of the distance between the plasma and the treated sample using SMD (□, Klä. [25, 52], Shim [53]), RF-APPJ (∆, Lim [54], Herr [55]) and microwave plasma (○, Sch. [56], Moi [57]). (C) standard decontamination methods including ethylene oxide (EtO, Shin [58]), Sterrad systems (Rut. [59], Alf [24]), hydrogen peroxide (H2O2, Klä. [25], Rog [41]), dry heat (Kem [60]) and formaldehyde (Rog [42]). The colors of the bars and symbols refer to the type of spores treated. Experimental conditions are detailed in Supplementary material and in the mentioned references. The asterisk indicates that the technique requires an additional aeration time (typically 15 h) in order to remove toxic residuals.
Figure 5A. shows that two types of plasma sources demonstrated a 6-log reduction of endospores on dried surfaces in the plasma phase: microwave (MW) sources operating at reduced pressure, and dielectric barrier discharges (DBD) operating at atmospheric pressure. The lowest T6-log value was reported by Muranyi et al. on B. Pumilis, B. Atrophaeus and A. Niger spores using a cascaded-DBD (CDBD) in atmospheric pressure air. The reported T6-log is about 10 seconds whereas other DBDs required about 100 seconds [38, 44, 45]. This difference can be partially explained by the fact that Muranyi et al. sprayed the initial microbial load of 106 CFU on a dried polyethylene terephthalate (PET) sample of 16 cm2, so that a homogeneous concentration of spores of about 6×104 spores/cm2 was obtained. In the other reports, the concentration was typically 106 spores/cm2, thus increasing the risk of formation of multiple layers of spores, and preventing the contact of plasma agents with the spores. This example shows that standardized microbiological test procedures are needed to better compare the efficiencies of different plasma sources.
In the post-discharge (see Figure 5B), surface micro-discharges (SMD), radiofrequency atmospheric pressure plasma jets (RF-APPJ) and reduced pressure MW plasma sources were also able to reach 6-log reduction. The T6-log values range from about 20 seconds at a few millimeters from the discharge using RF-APPJ to several thousands of seconds at 1 meter using MW plasmas. As expected, increasing the distance from the plasma source lowers the dose of biocidal agents, but provides lower material degradation and higher treatment area. This configuration is promising for the decontamination of long, small diameter tubes such as the lumens of endoscopes [19, 46], for which the diffusion of the sterilant along the whole length of the lumen is still a major concern [24], particularly in the presence of residual inorganic and organic soil [47, 48].
All reported results showed that a 6-log reduction can be achieved in less than a few thousands of seconds, which is comparable with the typical performances of standard low temperature sterilizers (see Figure 5C).
Plasma decontamination has various advantages compared to other methods including:
i/ compatibility with heat-sensitive devices,
ii/ ability to penetrate into narrow devices (e.g. endoscopes),
iii/ absence of toxic waste,
iv/ no shelf life of the sterilization products
v/ onsite operational characteristic.
To date, however, the role of plasma is minor in commercial sterilizing processes. For instance, the efficiency of hydrogen peroxide plasma(e.g. Sterrad sterilization system from Johnson & Johnson) is mainly due to hydrogen peroxide exposure, while the plasma phase is employed to eliminate toxic residues[61]. Nonetheless one application of low-pressure plasma has been clearly identified recently for packaging sterilization in the pharmaceutical industry [62]. Its efficiency against endospores typically used as bio-indicators shows that plasma is a promising candidate as a sterilization method. Yet, multiphase inactivation curves indicate that the biocidal agents are not distributed uniformly (low penetration depth and inhomogeneous plasma) thus preventing efficient contact with the microorganisms and making it difficult to compare with literature results. In order to avoid this, standardized microbiological test procedures are needed, and the following recommendation can be made. First a monolayer of spores should be used to obtain reproducible results. To this end, the concentration of spores should be low and the spray deposition method should be employed in order to prevent the formation of clusters. Second, a small inoculation area should be used to ensure that the distribution of plasma agents is homogeneous across the test sample. Third, non-porous and inert substrate material (e.g. glass holder) should be used, except if a specific application is considered (e.g. decontamination of polymer surfaces). In particular, we expect that a high permeability of the substrate allows diffusion of spores inside the material, thus preventing homogeneous exposure of the spores to the plasma, and increasing the risk of incomplete collection of the spores before counting.
The literature review indicates that the relative humidity (RH) increases the sporicidal activity of the plasma phase through the swelling of spores. We suggest that RH should be considered as a parameter to optimize the process, keeping in mind that high RH may be detrimental for the treatment of moisture-sensitive devices such as electronics.
We gave a summary of plasma inactivation results for which a 6-log reduction of endospores dried on surfaces was achieved. We found that several types of plasma sources operating in various gases and pressures can achieve a 6-log reduction in less than one minute, which is comparable with the processing time of standard low-temperature sterilization methods. From the literature review, we could not identify a single relevant bioindicator for plasma sterilization, because this depends on the dominant biocidal mechanism, which is specific to each plasma source.
In conclusion, plasma sources have demonstrated to be competitive sterilizing techniques. A number of biocidal plasma mechanisms are now well-known, but inadequate preparation and control of the environmental conditions does not allow a fair comparison of the biocidal efficiencies of different plasma sources. We recommend that future studies carefully consider the preparation and deposition of bioindicators in order to accurately determine the processing time required for sterility, and thus provide a means to rigorously compare the efficiency of different plasma devices. In summary, we recommend using:
• monolayer of spores for reproducibility
• spray deposition method to prevent the formation of clusters
• inoculation area smaller than size of the plasma to ensure homogeneous treatment
• inert standard deposition material with low permeability to prevent the diffusion of spores inside the material (good candidate substrates could be glass holder)
AS, GDS, COL, have contributed equally to this manuscript.
This research was supported by DGA grant DESDEMONA (RAPID contract EJ nr. 2101 241 872).
The authors declare that the research was conducted in the absence of any commercial or financial relationships that could be construed as a potential conflict of interest.
The Supplementary Material for this article can be found online at: https://www.frontiersin.org/articles/10.3389/fphy.2021.677971/full#supplementary-material
1. Mikhail M, Young T. Sterilization of Flexible Endoscopes. Decontamination in Hospitals and Healthcare. Elsevier (2019). p. 519–29.
2. Kumar P, Han JH. Packaging Materials for Non-thermal Processing of Food and Beverages. Emerging Food Packaging Technologies. Elsevier (2012). p. 323–34. doi:10.1533/9780857095664.3.323
3. Cortesão M, de Haas A, Unterbusch R, Fujimori A, Schütze T, Meyer V, et al. Aspergillus niger Spores Are Highly Resistant to Space Radiation. Front Microbiol (2020) 11:1–12. doi:10.3389/fmicb.2020.00560
4. Rastogi VK, Wallace L. Handbook on Biological Warfare Preparedness. Elsevier (2019). p. 195–208.Environmental Sampling and Bio-Decontamination-Recent Progress, Challenges, and Future Direction.
5. Moisan M, Barbeau J, Moreau S, Pelletier J, Tabrizian M, Yahia LH. Low-temperature Sterilization Using Gas Plasmas: a Review of the Experiments and an Analysis of the Inactivation Mechanisms. Int J Pharmaceutics (2001) 226:1–21. doi:10.1016/s0378-5173(01)00752-9
6. Ehlbeck J, Schnabel U, Polak M, Winter J, Von Woedtke T, Brandenburg R, et al. Low Temperature Atmospheric Pressure Plasma Sources for Microbial Decontamination. J Phys D Appl Phys (2011) 44(1):013002. doi:10.1088/0022-3727/44/1/013002
7. Moreau M, Orange N, Feuilloley MGJ. Non-thermal Plasma Technologies: New Tools for Bio-Decontamination. Biotechnol Adv (2008) 26:610–7. doi:10.1016/j.biotechadv.2008.08.001
8. Laroussi M. Low Temperature Plasma-Based Sterilization: Overview and State-Of-The-Art. Plasma Process Polym (2005) 2:391–400. doi:10.1002/ppap.200400078
9. Lunov O, Zablotskii V, Churpita O, Jäger A, Polívka L, Syková E, et al. The Interplay between Biological and Physical Scenarios of Bacterial Death Induced by Non-thermal Plasma. Biomaterials (2016) 82:71–83. doi:10.1016/j.biomaterials.2015.12.027
10. Rossi F, De Mitri R, Bobin S, Eloy R. Plasma Sterilisation: Mechanisms Overview and Influence of Discharge Parameters. Plasma Processes and Polymers. Wiley VCH (2005). p. 319–31. doi:10.1002/3527605584.ch24Plasma Sterilisation: Mechanisms Overview and Influence of Discharge Parameters
11. Filipić A, Gutierrez-Aguirre I, Primc G, Mozetič M, Dobnik D. Cold Plasma, a New Hope in the Field of Virus Inactivation. Trends Biotechnol (2020) 38(11):1278–91. doi:10.1016/j.tibtech.2020.04.003
12. Chen Z, Garcia G, Arumugaswami V, Wirz RE. Cold Atmospheric Plasma for SARS-CoV-2 Inactivation. Phys Fluids (2020) 32:111702. doi:10.1063/5.0031332
13. Misra NN, Yadav B, Roopesh MS, Jo C. Cold Plasma for Effective Fungal and Mycotoxin Control in Foods: Mechanisms, Inactivation Effects, and Applications. Compr Rev Food Sci Food Saf (2019) 18:106–20. doi:10.1111/1541-4337.12398
14. Hojnik N, Modic M, Ni Y, Filipič G, Cvelbar U, Walsh JL. Effective Fungal Spore Inactivation with an Environmentally Friendly Approach Based on Atmospheric Pressure Air Plasma. Environ Sci Technol (2019) 53:1893–904. doi:10.1021/acs.est.8b05386
15. Elmoualij B, Thellin O, Gofflot S, Heinen E, Levif P, Séguin J, et al. Decontamination of Prions by the Flowing Afterglow of a Reduced-Pressure N2–O2 Cold-Plasma. Plasma Process. Polym (2012) 9:612–8. doi:10.1002/ppap.201100194
16. von Woedtke T, Kramer A, Weltmann K-D. Plasma Sterilization: What Are the Conditions to Meet This Claim?. Plasma Process Polym (2008) 5(6):534–9. doi:10.1002/ppap.200800013
17. Moisan M, Levif P, Séguin J, Barbeau J. Sterilization/disinfection Using Reduced-Pressure Plasmas: Some Differences between Direct Exposure of Bacterial Spores to a Discharge and Their Exposure to a Flowing Afterglow. J Phys D Appl Phys (2014) 47(28). doi:10.1088/0022-3727/47/28/285404
18. Boudam MK, Moisan M, Saoudi B, Popovici C, Gherardi N, Massines F. Bacterial Spore Inactivation by Atmospheric-Pressure Plasmas in the Presence or Absence of UV Photons as Obtained with the Same Gas Mixture. J Phys D: Appl Phys (2006) 39(16):3494–507. doi:10.1088/0022-3727/39/16/s07
19. Pointu A-M, Ricard A, Odic E, Ganciu M. Nitrogen Atmospheric Pressure post Discharges for Surface Biological Decontamination inside Small Diameter Tubes. Plasma Process Polym (2008) 5(6):559–68. doi:10.1002/ppap.200800016
20. Becker K, Koutsospyros A, Yin SM, Christodoulatos C, Abramzon N, Joaquin JC, et al. Plasma Physics and Controlled Fusion (2005). Environmental and Biological Applications of Microplasmas.
21. Shintani H. Current Mistaken Interpretation of Microbiological Data on Gas Plasma Sterilization. Pharm Regul Aff Open Access (2015) 04(02). doi:10.4172/2167-7689.1000138
22. Kogelheide F, Voigt F, Hillebrand B, Moeller R, Fuchs F, Gibson AR, et al. The Role of Humidity and UV-C Emission in the Inactivation of B. Subtilis Spores during Atmospheric-Pressure Dielectric Barrier Discharge Treatment. J Phys D Appl Phys (2020) 53:295201. doi:10.1088/1361-6463/ab77cc
23. Rutala WA, Weber DJ. Guideline for Disinfection and Sterilization in Guideline for Disinfection and Sterilization in Healthcare Facilities (2008).
24. Alfa MJ, Degagne P, Olson N, Puchalski T, Degagne P, Olson N, et al. Comparison of Ion Plasma, Vaporized Hydrogen Peroxide, and 100% Ethylene Oxide Sterilizers to the 12/88 Ethylene Oxide Gas Sterilizer. Infect Control Hosp Epidemiol (1996) 17(2):92–100. doi:10.1086/647252
25. Klämpfl TG, Shimizu T, Koch S, Balden M, Gemein S, Li Y-F, et al. Decontamination of Nosocomial Bacteria IncludingClostridium difficileSpores on Dry Inanimate Surface by Cold Atmospheric Plasma. Plasma Process Polym (2014) 11(10):974–84. doi:10.1002/ppap.201400080
26. Muranyi P, Wunderlich J, Heise M. Sterilization Efficiency of a Cascaded Dielectric Barrier Discharge. J Appl Microbiol (2007) 103(5):1535–44. doi:10.1111/j.1365-2672.2007.03385.x
27. Halfmann H, Bibinov N, Wunderlich J, Awakowicz P. A Double Inductively Coupled Plasma for Sterilization of Medical Devices. J Phys D: Appl Phys (2007) 40(14):4145–54. doi:10.1088/0022-3727/40/14/008
28. Heise M, Neff W, Franken O, Muranyi P, Wunderlich J. Sterilization of Polymer Foils with Dielectric Barrier Discharges at Atmospheric Pressure. Plasmas Polym (2004) 9(1):23–33. doi:10.1023/b:papo.0000039814.70172.c0
29. Raguse M, Fiebrandt M, Stapelmann K, Madela K, Laue M, Lackmann J-W, et al. Improvement of Biological Indicators by Uniformly Distributing Bacillus Subtilis Spores in Monolayers to Evaluate Enhanced Spore Decontamination Technologies. Appl Environ Microbiol (2016) 82(7):2031–8. doi:10.1128/aem.03934-15
30. Shintani H, Shimizu N, Y I, Imanishi T, Tamazawa K, Taniguchi A, et al. Inactivation of Microorganisms and Endotoxins by Low Temperature Nitrogen Gas Plasma Exposure. Biocontrol Sci (2007) 12(4):131–43. doi:10.4265/bio.12.131
31. Coohill TP, Sagripanti J-L. Overview of the Inactivation by 254 Nm Ultraviolet Radiation of Bacteria with Particular Relevance to Biodefense. Photochem Photobiol (2008) 84:1084–90. doi:10.1111/j.1751-1097.2008.00387.x
32. Fernández A, Shearer N, Wilson DR, Thompson A. Effect of Microbial Loading on the Efficiency of Cold Atmospheric Gas Plasma Inactivation of Salmonella enterica Serovar Typhimurium. Int J Food Microbiol (2012) 152(3):175–80. doi:10.1016/j.ijfoodmicro.2011.02.038
33. Li Y-F, Shimizu T, Zimmermann JL, Morfill GE. Cold Atmospheric Plasma for Surface Disinfection. Plasma Process. Polym (2012) 9(6):585–9. doi:10.1002/ppap.201100090
34. Dobrynin D, Friedman G, Fridman A, Starikovskiy A. Inactivation of Bacteria Using Dc corona Discharge: Role of Ions and Humidity. New J Phys (2011) 13:103033. doi:10.1088/1367-2630/13/10/103033
35. Lu H, Patil S, Keener KM, Cullen PJ, Bourke P. Bacterial Inactivation by High-Voltage Atmospheric Cold Plasma: Influence of Process Parameters and Effects on Cell Leakage and DNA. J Appl Microbiol (2014) 116(4):784–94. doi:10.1111/jam.12426
36. Mahfoudh A, Moisan M, Séguin J, Barbeau J, Kabouzi Y, Kéroack D. Inactivation of Vegetative and Sporulated Bacteria by Dry Gaseous Ozone. Ozone: Sci Eng (2010) 32(3):180–98. doi:10.1080/01919511003791971
37. Gilbert GL, Gambill VM, Spiner DR, Hoffman RK, Phillips CR, Gambill M. Effect of Moisture on Ethylene Oxide Sterilization. Vol. 12, Applied Microbiology (1964).
38. Patil S, Moiseev T, Misra NN, Cullen PJ, Mosnier JP, Keener KM, et al. Influence of High Voltage Atmospheric Cold Plasma Process Parameters and Role of Relative Humidity on Inactivation of Bacillus Atrophaeus Spores inside a Sealed Package. J Hosp Infect (2014) 88(3):162–9. doi:10.1016/j.jhin.2014.08.009
39. Hähnel M, Von Woedtke T, Weltmann K-D. Influence of the Air Humidity on the Reduction ofBacillusSpores in a Defined Environment at Atmospheric Pressure Using a Dielectric Barrier Surface Discharge. Plasma Process Polym (2010) 7:244–9. doi:10.1002/ppap.200900076
40. Sigwarth V, Stärk A. Effect of Carrier Materials on the Resistance of Spores of Bacillus Stearothermophilus to Gaseous Hydrogen Peroxide. PDA J Pharm Sci Technol (2003) 57(1):3–11.
41. Rogers JV, Sabourin CLK, Choi YW, Richter WR, Rudnicki DC, Riggs KB, et al. Decontamination Assessment of Bacillus Anthracis, Bacillus Subtilis, and Geobacillus Stearothermophilus Spores on Indoor Surfaces Using a Hydrogen Peroxide Gas Generator. J Appl Microbiol (2005) 99(4):739–48. doi:10.1111/j.1365-2672.2005.02686.x
42. Rogers JV, Choi YW, Richter WR, Rudnicki DC, Joseph DW, Sabourin CLK, et al. Formaldehyde Gas Inactivation ofBacillus Anthracis,Bacillus Subtilis, andGeobacillus Stearothermophilusspores on Indoor Surface Materials. J Appl Microbiol (2007) 103(4):1104–12. doi:10.1111/j.1365-2672.2007.03332.x
43. Levif P, Séguin J, Moisan M, Soum-Glaude A, Barbeau J. Packaging Materials for Plasma Sterilization with the Flowing Afterglow of an N2-O2 Discharge: Damage Assessment and Inactivation Efficiency of Enclosed Bacterial Spores. J Phys D Appl Phys (2011) 44(40). doi:10.1088/0022-3727/44/40/405201
44. Venezia RA, Orrico M, Houston E, Yin S-M, Naumova YY. Lethal Activity of Nonthermal Plasma Sterilization against Microorganisms. Infect Control Hosp Epidemiol (2008) 29(5):430–6. doi:10.1086/588003
45. Akitsu T, Ohkawa H, Tsuji M, Kimura H, Kogoma M. Plasma Sterilization Using Glow Discharge at Atmospheric Pressure. Surf Coat Tech (2005) 193:29–34. doi:10.1016/j.surfcoat.2004.07.042
46. Bhatt S, Mehta P, Chen C, Schneider CL, White LN, Chen HL, et al. Efficacy of Low-Temperature Plasma-Activated Gas Disinfection against Biofilm on Contaminated GI Endoscope Channels. Gastrointest Endosc (2018) 89(1):105–14. doi:10.1016/j.gie.2018.08.009
47. Alfa MJ, DeGagne P, Olson N, Hizon R. Comparison of Liquid Chemical Sterilization with Peracetic Acid and Ethylene Oxide Sterilization for Long Narrow Lumens. Am J Infect Control (1998) 26:469–77. doi:10.1016/s0196-6553(98)70018-5
48. Humphries RM, McDonnell G. Superbugs on Duodenoscopes: the Challenge of Cleaning and Disinfection of Reusable Devices. J Clin Microbiol (2015) 53:3118–25. doi:10.1128/jcm.01394-15
49. Muranyi P, Wunderlich J, Heise M. Influence of Relative Gas Humidity on the Inactivation Efficiency of a Low Temperature Gas Plasma. J Appl Microbiol (2008) 104(6):1659–66. doi:10.1111/j.1365-2672.2007.03691.x
50. Stapelmann K, Fiebrandt M, Raguse M, Awakowicz P, Reitz G, Moeller R. Utilization of Low-Pressure Plasma to Inactivate Bacterial Spores on Stainless Steel Screws. Astrobiology (2013) 13(7):597–606. doi:10.1089/ast.2012.0949
51. Nagatsu M, Zhao Y, Motrescu I, Mizutani R, Fujioka Y, Ogino A. Sterilization Method for Medical Container Using Microwave-Excited Volume-Wave Plasma. Plasma Process. Polym (2012) 9(6):590–6. doi:10.1002/ppap.201100111
52. Klämpfl TG, Isbary G, Shimizu T, Li Y-F, Zimmermann JL, Stolz W, et al. Cold Atmospheric Air Plasma Sterilization against Spores and Other Microorganisms of Clinical Interest. Appl Environ Microbiol (2012) 78(15):5077–82. doi:10.1128/aem.00583-12
53. Shimizu S, Barczyk S, Rettberg P, Shimizu T, Klaempfl T, Zimmermann JL, et al. Cold Atmospheric Plasma - A New Technology for Spacecraft Component Decontamination. Planet Space Sci (2014) 90:60–71. doi:10.1016/j.pss.2013.10.008
54. Lim JP, Uhm HS, Li SZ. Influence of Oxygen in Atmospheric-Pressure Argon Plasma Jet on Sterilization of Bacillus Atrophaeous Spores. Phys Plasmas (2007) 14(9). doi:10.1063/1.2773705
55. Herrmann HW, Henins I, Park J, Selwyn GS. Decontamination of Chemical and Biological Warfare (CBW) Agents Using an Atmospheric Pressure Plasma Jet (APPJ). Phys Plasmas (1999) 6(5):2284–9. doi:10.1063/1.873480
56. Schnabel U, Andrasch M, Weltmann K-D, Ehlbeck J. Inactivation of Vegetative Microorganisms andBacillus atrophaeusEndospores by Reactive Nitrogen Species (RNS). Plasma Process Polym (2014) 11(2):110–6. doi:10.1002/ppap.201300072
57. Moisan M, Boudam K, Carignan D, Kéroack D, Levif P, Barbeau J, et al. Sterilization/disinfection of Medical Devices Using Plasma: the Flowing Afterglow of the Reduced-Pressure N2-O2discharge as the Inactivating Medium. Eur Phys J Appl Phys (2013) 63:10001. doi:10.1051/epjap/2013120510
58. Shintani H. Ethylene Oxide Gas Sterilization of Medical Devices. Biocontrol Sci (2017) 22(1):1–16. doi:10.4265/bio.22.1
59. Rutala WA, Gergen MF, Weber DJ. Comparative Evaluation of the Sporicidal Activity of New Low-Temperature Sterilization Technologies: Ethylene Oxide, 2 Plasma Sterilization Systems, and Liquid Peracetic Acid. Am J Infect Control (1998) 26(4):393–8. doi:10.1016/s0196-6553(98)70034-3
60. Kempf MJ, Schubert WW, Beaudet RA. Determination of Lethality Rate Constants and D-Values forBacillus Atrophaeus(ATCC 9372) Spores Exposed to Dry Heat from 115°C to 170°C. Astrobiology (2008) 8(6):1169–82. doi:10.1089/ast.2007.0208
61. Krebs MC, Bécasse P, Verjat D, Darbord JC. Gas-plasma Sterilization: Relative Efficacy of the Hydrogen Peroxide Phase Compared with that of the Plasma Phase. Int J Pharmaceutics (1998) 160(1):75–81. doi:10.1016/s0378-5173(97)00296-2
62. Denis B, Steves S, Semmler E, Bibinov N, Novak W, Awakowicz P. Plasma Sterilization of Pharmaceutical Products: From Basics to Production. Plasma Process. Polym (2012) 9(6):619–29. doi:10.1002/ppap.201100211
Keywords: plasma, endospores, decontamination, sterilization, non-thermal
Citation: Salmon A, Stancu GD and Laux CO (2021) Decontamination Of Endospores By Plasma Sources On Dried Surfaces: A Review Of Key Parameters And Inactivation Results. Front. Phys. 9:677971. doi: 10.3389/fphy.2021.677971
Received: 08 March 2021; Accepted: 10 May 2021;
Published: 26 May 2021.
Edited by:
Mounir Laroussi, Old Dominion University, United StatesReviewed by:
James L Walsh, University of Liverpool, United KingdomCopyright © 2021 Salmon, Stancu and Laux. This is an open-access article distributed under the terms of the Creative Commons Attribution License (CC BY). The use, distribution or reproduction in other forums is permitted, provided the original author(s) and the copyright owner(s) are credited and that the original publication in this journal is cited, in accordance with accepted academic practice. No use, distribution or reproduction is permitted which does not comply with these terms.
*Correspondence: A Salmon, YXJ0aHVyLnNhbG1vbkBjZW50cmFsaWVucy5uZXQ=
Disclaimer: All claims expressed in this article are solely those of the authors and do not necessarily represent those of their affiliated organizations, or those of the publisher, the editors and the reviewers. Any product that may be evaluated in this article or claim that may be made by its manufacturer is not guaranteed or endorsed by the publisher.
Research integrity at Frontiers
Learn more about the work of our research integrity team to safeguard the quality of each article we publish.