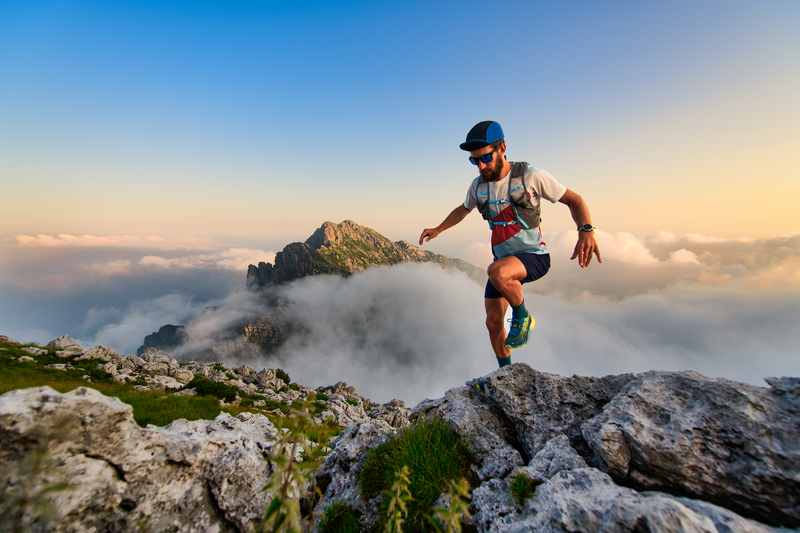
95% of researchers rate our articles as excellent or good
Learn more about the work of our research integrity team to safeguard the quality of each article we publish.
Find out more
ORIGINAL RESEARCH article
Front. Phys. , 12 April 2021
Sec. Optics and Photonics
Volume 9 - 2021 | https://doi.org/10.3389/fphy.2021.634324
This article is part of the Research Topic Modern Tools for Time-Resolved Luminescence Biosensing and Imaging View all 15 articles
Pollution by heavy metals represents a significant environmental burden. We employed confocal microscopy with spectral detection and fluorescence lifetime imaging (FLIM) to evaluate the effect of nanoparticles (NPs) from various metals (Zinc, Nickel, Cobalt, Copper) on endogenous fluorescence of Fontinalis antipyretica moss. Short term (3–5 day) exposure to NPs, designed and fabricated by direct synthesis using femtosecond laser ablation in water, was studied. The green flavonoid and/or lignin endogenous fluorescence peaking between 500 and 560 nm was found to be increased by Zn and significantly reduced by Cu. The overall red chlorophyll fluorescence intensity with a maximum of 680 nm remained largely unchanged after exposure to Ni and Zn, but was decreased in the presence of Co and completely abolished by Cu. All NPs but Zn induced changes in the fluorescence lifetimes, demonstrating increased sensitivity of this parameter to environmental pollution. Gathered data indicate fast responsiveness of the endogenous fluorescence in the Fontinalis antipyretica moss to the presence of heavy metals that can thus potentially serve as a biosensing tool for monitoring environmental pollution in the moss natural environment.
In the last decades, the environment has been severely polluted by heavy metals. Despite the fact that some heavy metals are essential trace elements for the functioning of living organisms, most can be toxic due to formation of complex compounds within the cells [1]. Excessive levels of metals such as Co, Cu, or Zn, induced by industrial developments, thus often lead to detrimental effects on living cells and organisms [2]. Heavy metals induce lipid peroxidation and DNA damage leading to several diseases, namely various types of cancer [3]. Other potential harmful effects include metabolic disorders, lung inflammation, hepatic and/or heart diseases, as well as nervous system problems and foetal as well as birth defects [4, 5]. Mosses, together with algae and higher plants, are highly sensitive to environmental pollution including that of heavy metals that they are also capable to accumulate [6]. The decrease in the photosynthetic capacity, inhibition of growth, as well as reduced biosynthesis of chlorophylls and/or carotenoids often accompanies metal toxicity [7, 8]. In the past, diverse investigations proved the suitability of Fontinalis antipyretica for heavy metal biomonitoring in natural habitats [9, 10]. Previous research has demonstrated that aquatic moss Fontinalis antipyretica can be used as an indicator of heavy metal toxicity [11, 12]. For that reason, we opted for the Fontinalis antipyretica moss and evaluated its responsiveness to heavy metals using spectrally- and time-resolved monitoring of their endogenous fluorescence.
The choice of the appropriate detection method for environmental monitoring of the presence of heavy metals in aqueous solution is broadly important. Among available methods, fluorescence emission spectroscopy is an attractive approach because of its non-invasiveness, easy-to-use operation and the widespread availability of equipment for analysis. We employed both spectrally- and time-resolved fluorescence monitoring. Time-resolved imaging presents an advanced tool to monitor changes of the endogenous fluorophores to their environment directly in living cells [13, 14]. Chlorophyll a is a well-established fluorescing pigment employed in photosynthesis [15]. In addition to the chlorophyll fluorescence, NADPH dependent blue-green fluorescence (BGF) was also described in isolated chloroplasts [16, 17], while most of the green fluorescence in the intercellular space was associated with lignins of the intermediate lamella [18]. We previously demonstrated a sensitivity of endogenous fluorescence to various environmental stressors employing the spectrally and time-resolved microscopy in algae [19, 20]. In this contribution, we focus on endogenous fluorescence, derived primarily from chlorophylls, but also from flavonoids and/or lignins, studied by spectrally- and time-resolved imaging in moss.
To study the effect of heavy metals, we have chosen heavy metal NPs produced by femtosecond laser ablation, as previously described [21]. NPs possess particular properties related to their small size, as well as composition. Consequently, they offer a large number of applications [22–24]. Their interaction with living organisms was broadly investigated. CuO, as well as ZnO NPs were shown to have antimicrobial properties [25–27]. Ni and Co NPs were also demonstrated to induce stress in the microalgae [28, 29]. Under heavy metals exposure, the growth and the chemical composition of several substances, including chlorophylls, have been reduced within the first 48 h [30]. We also noted the capacity of heavy metal NPs to reduce the chlorophyll fluorescence after 10–14 days of exposure in algae [31].
In this contribution, we focus on the effect of heavy metals on endogenous fluorescence in the Fontinalis antipyretica moss. Water is an exceptionally essential source for the presence of life on the Earth. The water quality has been seriously affected by human activities. Heavy metals enter into the aquatic system through the agricultural runoff and industrial discharges [32]. Previously, we have demonstrated the sensitivity of marine algae Dunalliela tertiolecta to laboratory-induced stress with heavy metal cadmium [19, 33]. The study showed an appearance of the stress-related light-harvesting complex (LHC), as well as changes at the endogenous fluorescence levels. We also demonstrated that endogenous fluorescence can potentially serve as a biosensing tool for monitoring environmental pollution [34]. Our aim in this work is to evaluate non-invasively the short term (in terms of days) exposure of Fontinalis antipyretica moss to chosen heavy metal NPs (Zn, Ni, Co, Cu), employing spectrally- and time-resolved studies of their endogenous fluorescence.
Water bryophyte green moss species Fontinalis antipyretica was gathered commercially from aquashop (Aquasymbioza, Bratislava, Slovakia) and grown under ambient light. For the measurements, green moss fyloides were cut and placed on a microscope slide without further modification.
NPs of the size in the range 10–100 nm were prepared by laser ablation of metal surfaces, as described previously [21, 31]. In brief, discs from pure metals were covered by deionised water and we have employed ultrafast spirit ytterbium amplified laser (HighQ, SpectraPhysics, Newport, 300 fs pulse width, 1,040 nm wavelength; 4 W mean power operated at 100 kHz) that was focused at the metal disc surface by Plan N 10×/0.25 UIS2 objective (Olympus). The laser beam movement over the surface was done by motorized XYZ micropositioner (Walter Uhl Technische Mikroskopie GmbH & Co.) via a joystick interface.
Substrates for the fabrication of NPs included following metal discs of pure elements (Goodfellow Cambridge Ltd.):
The size of the NPs, determined by Scanning Electron Microscopy [21] reached under 20 nm for Co and Cu, between 10 and 20 nm for Zn (often aggregating into chunks up to 80 nm) and between 20 and 80 nm for Ni.
NPs were prepared in distilled water and 20 µl of the solution with NPs was added without additional modification to Fontinalis antipyretica moss in 0.5 ml of pure water. In the control sample, the equivalent of deionized water was added. NPs were maintained with the moss leaves during 3–5 days before use. All procedures complied with the Institutional rules and guidelines of the International Laser Center in Bratislava.
Confocal autofluorescence images were gathered using the laser scanning confocal microscope Axiovert 200 LSM 510 Meta (Carl Zeiss, Germany) equipped with objective C-Apochromat 40×, 1.2 W Corr. Moss leaves were excited with the 450 nm laser (Kvant, Slovakia), fluorescence was detected using two photomultipliers and 16 channel META detector. Channel 1 was recorded with BP 500–550 IR filter and channel 2 using BP 650–710 IR filter. Spectrally-resolved blue/green images were recorded between 477 and 638 nm with 11 nm step using 472–643 filter. Spectrally-resolved red images were gathered between 638 and 713 nm with 11 nm step using 632–718 filter.
FLIM images were recorded using time-correlated single-photon counting (TCSPC) technique equipped with a 475 nm picosecond pulsed laser diode (BDL-475, Becker&Hickl, Germany) [14]. The laser beam was reflected to the sample through an epifluorescence path of the Axiovert 200 LSM 510 Meta (Zeiss, Germany) inverted microscope and LP 500 nm filter was used to separate emitted fluorescence from laser excitation. HPM 100–40 photomultiplier and SPC-830 TCSPC board were used for detection of time-resolved signals (both Becker&Hickl, Germany).
Confocal images were analyzed by ZEN 2011 software (Zeiss, Germany). FLIM images were analyzed using proprietary software packages SPCImage (Becker&Hickl, Germany). In the data fitting procedure, the synthetic IRF function produced by SPCImage software (Becker Hickl, Germany) was employed as an instrument response function. Statistical comparison was done by Origin 6.0 Professional using one-way Anova, with p < 0.05 considered as significant.
Our aim was to examine changes in the spectrally- and time-resolved endogenous fluorescence of the green moss water bryophyte species Fontinalis antipyretica following the short-term (3–5 days) exposure to diverse heavy metal NPs.
The endogenous fluorescence of the moss was evaluated in the presence of NPs at two emission windows: first in the green spectral region, 500–550 nm, and second in the red spectral region, 650–710 nm in response to excitation at 450 nm (Figure 1A green and red windows). The two different channels were chosen because the red chlorophyll fluorescence was several times higher than the green one. The intensity in the red spectral region was comprised within the cell chloroplasts (Figure 1B red “balls”), as expected for the contribution of chlorophylls [15]. The green fluorescence was mainly situated in the intercellular space (Figure 1B green “fibres”) and, in part, also recorded intracellularly. The green fluorescence is most likely related to the fluorescence of intracellular flavins and flavonoids when recorded inside the cells and/or lignins when measured as green “fibres” in the intercellular environment [18].
FIGURE 1. Fluorescence microscopy images of endogenous fluorescence of Fontinalis antipyretica in control conditions and in the presence of heavy metal NPs. (A) Images recorded by laser scanning confocal microscopy in control conditions, excitation 450 nm. Green fluorescence channel 1) emission 550–550 nm, red fluorescence channel 2) emission 650–710 nm, grey channel 3) transmission image, mixed channel 4) overlay of channels 1–3. (B) Amplified section of the overlaid green and red fluorescence image, (C) the overlay of all channels in the presence of the tested heavy metal NPs (Zn, Ni, Co, and Cu); scale 20 μm.
In the presence of heavy metal NPs, we have observed changes in the endogenous fluorescence in the moss (Figure 1C). Zn induced a clear rise in the green fluorescence in the intercellular space, while Cu attenuated both the green and the red fluorescence of the moss. Next, to evaluate the spectral characteristics of the fluorescence that was affected by heavy metals, we have therefore employed the spectrally-resolved fluorescence. Spectral changes in the endogenous fluorescence of the moss were evaluated by spectrally-resolved images taken in the blue/green spectral region, using an 11 nm step between 477 and 638 nm (Figure 2A). In these conditions, endogenous fluorescence in control conditions was recordable between 520 and 560 nm (Figure 2B). Using this protocol in control conditions, the fluorescence peaked at 540 nm. When recorded at this wavelength, we noted a significant rise in the presence of Zn (Figure 2C). On the other hand, Cu induced a significant decrease in this fluorescence, while Ni and Co had a tendency to reduce it, but this effect did not reach significance. At Figure 2D, representative images in the presence of NPs are shown. Aggregation was proposed to play role in the modification of the lignin fluorescence [35] and we advance that it can also play a role in this action.
FIGURE 2. Spectrally-resolved images of Fontinalis antipyretica blue/green fluorescence. (A) An original lambda-coded image in control conditions taken with an 11 nm step between 477 and 638 nm, excitation 450 nm. (B) Comparison of the recorded spectra in the presence of individual NPs (n = 28 in Ctrl, n = 20 in Zn, n = 30 in Ni, n = 28 in Co, n = 37 in Cu). (C) Statistical comparison at 540 nm, *p < 0.05 with one-way Anova. (D) Original recordings in the presence of Zn, Ni, Co and Cu NPs, scale 20 μm.
We have also recorded a rise in the blue signal at 477–488 nm in the presence of all but Zn NPs (Figure 2D, blue colour). This signal was mostly lacking in control conditions and, as we already reported previously [31], is most likely related to the reflection on the metal particles. This fluorescence was mainly situated in the intercellular space and its lack in the presence of Zn can thus be related to the rise in the stronger green fluorescence in this condition.
Red fluorescence of the moss represents the typical chlorophyll fluorescence [15]. When evaluated using spectrally-resolved confocal imaging, the fluorescence was taken in the red spectral region between 638 and 713 nm with an 11 nm step (see Figure 3A for the original recording in control conditions, with spectra at Figure 3B). In these conditions, the moss fluorescence in the chloroplasts peaked at 680 nm, as expected for the chlorophylls. At this emission wavelength, no change in the fluorescence was recorded in the presence of Zn (Figure 3C, with the original recording at Figure 3D). On the other hand, Co and Cu significantly reduced the red fluorescence at 680 nm (Figures 3C,D). Ni tends to decrease the chlorophyll signal, but this effect did not reach significance. The gathered result uncovered different sensitivities of the chlorophyll fluorescence to NPs.
FIGURE 3. Spectrally-resolved confocal microscopy images of Fontinalis antipyretica red fluorescence. (A) An original lambda-coded image in control conditions taken with an 11 nm step between 638 and 713 nm, excitation 450 nm. (B) Comparison of the recorded spectra in the presence of individual NPs (n = 42 in Ctrl, n = 28 in Zn, n = 26 in Ni, n = 36 in Co, n = 31 in Cu). (C) Statistical comparison at 680 nm, *p < 0.05 with one-way Anova. (D) Spectrally-resolved confocal microscopy imaging of the red fluorescence in the presence of Zn, Ni, Co, and Cu NPs, scale 20 μm.
In order to better understand the observed reduction in the chlorophyll fluorescence in the presence of NPs, we have employed FLIM. Time-resolved fluorescence was recorded by TCSPC following excitation at 475 nm, using LP 500 nm emission filter (Figure 4). In such settings, most of the recorded fluorescence is situated intracellularly and is derived from chloroplasts inside Fontinalis anripyretica cells (seeFigure 4A for the original recording of the FLIM image in control conditions). Fluorescence decays (see example of the decay curve in control conditions at Supplementary Figure S1) presented the best χ2 (under 1.2–1.6) for a 3-exponential decomposition (see lifetime values with corresponding amplitudes at Supplementary Table S1). At the same time, most of the fluorescence decayed at tau1. Such fluorescence lifetime situated between 200 and 500 ps (Figure 4B) which corresponds well to the chlorophyll fluorescence [20, 36]. In addition to fluorescence lifetime histograms (Figure 4B), we therefore also evaluated amplitudes related to the fixed lifetime of 100 ps (close to low lifetime values recorded in conditions such as Cu) and 350 ps (recorded in most conditions, including the control ones). In control conditions, the a1/a2 ratio for these fixed lifetimes was 20/73% with a small (7%) residual third component (Figure 4C).
FIGURE 4. FLIM images of Fontinalis antipyretica moss. (A) An original FLIM image in control conditions (CTRL) following excitation at 475 nm, using LP 500 nm emission filter. Lifetime distribution showed in the range 200–500 ps; (B) histograms of fluorescence lifetimes in the recorded conditions illustrated between 50 and 500 ps. (C) Amplitudes gathered by a 3-exponential decay for fixed lifetimes of 100 ps (a1, component 1) and 350 ps (a2, component 2), with residual third component a3 (n = 6 in Ctrl, n = 5 in Zn, n = 7 in Ni, n = 6 in Co, n = 4 in Cu). (D) Representative FLIM images in the presence of Zn, Ni, Co and Cu NPs, scale 200–500 ps.
When NPs were applied to Fontinalis anripyretica cells, statistical comparison of the recorded lifetime histograms (Figure 4B) revealed that, when compared to control conditions, Zn induced little or no change in the lifetime distribution for this fluorescence (Figures 4B,D), with the a1/a2 unaffected (23/68%, Figure 4C). On the other hand, in the presence of Co, the a1/a2 ratio reached close to parity (50/45%). Interestingly, Ni that was unable to induce a significant change in the overall fluorescence reversed the a1/a2 ratio to 68/28%. Such reverse of the a1/a2 ratio was even more pronounced in the presence of Cu, where it reached 89/10% (Figure 4C). This effect of Cu was also observable at the lifetime histograms (Figure 4B): as the Cu shortened the fluorescence lifetimes, an appearance of a very short lifetime around 150 ps was noted. Observed effects of NPs on the chlorophyll fluorescence lifetimes indicate that the recorded decrease in the chlorophyll fluorescence in the presence of NPs is most likely related to the change in the molecular environment. These results also suggest higher sensitivity of the fluorescence lifetimes than that of the fluorescence intensity to environmental stress, making this parameter a potential biosensing tool for rapid responsiveness of the moss to the heavy metal presence.
Data gathered in this contribution demonstrate that the short-term exposure for 3–5 days of the Fontinalis anripyretica moss to NPs produced from various metal targets using fs laser ablation [21] induced changes in its endogenous fluorescence. More precisely, we observed an increase of the green fluorescence in the spectral region 500–560 nm by the Zn and its decrease by the Cu NPs. On the other hand, the red chlorophyll fluorescence was reduced by the Co and Cu NPs and this effect was accompanied by changes in the fluorescence lifetimes induced by all but Zn NPs.
Heavy metal pollution is one of the most sensitive environmental issues due to production and a discharge into the environment of wastes containing different heavy metals by various industries. The presence of heavy metals in water and wastewater is thus increasing [2, 6]. An inhibited biosynthesis of chlorophylls, together with the decrease of growth-limiting photosynthesis and respiration [7, 8, 30] belong to the most frequent symptoms of the metal toxicity. In the present study, we evaluate the sensitivity of endogenous fluorescence to short-term exposure of heavy metal NPs in water bryophyte species Fontinalis antipyretica moss. This green moss is naturally occurring in sweet water lakes and is thus a good choice for the study of environmental pollution in water environments [37, 38]. It occurs on submerged rocks and wood in flowing or standing waters, including sweet water lakes, where it forms refuge for fish eggs. This moss was found to have good accumulation properties for metals when used in active biomonitoring in the river environment [11]. In addition, the Fontinalis antipyretica moss presents high sensitivity of chlorophyll fluorescence parameters to environmental changes [38] and can adsorb some of the heavy metals (Cd, Zn) [39].
Endogenous fluorescence was evaluated in two spectral regions: the green (peaking between 540 and 560 nm) and the red (peaking at 680 nm). Previously, the NADPH dependent blue-green fluorescence (BGF) was described in isolated chloroplasts [16, 17]. In our experiments, green/yellow fluorescence stimulated by excitation at 450 nm was most pronounced between 510 and 560 nm and this fluorescence inside the cells is most likely derived from NADPH-related flavins and flavonoids. In addition to the blue-green fluorescence inside the cells, most of the green fluorescence was observed in the intercellular space. Such green fluorescence can be derived from lignins of the intermediate lamella [18]. This fluorescence was clearly increased in the presence of Zn NPs and completely abolished in the presence of the Cu NPs. Metal oxide NPs of ZnO are known to have antimicrobial properties associated with an increase in the ROS production [26]. At the same time, aggregation-induced conjugation from phenylpropane units was believed to be the main source of the visible emission of lignin and different phenylpropane aggregates consequently forming the multi-fluorophore system in lignin micelle [40]. Lignin aggregation fluorescence behavior has great potential in its microstructure analysis and a case study of pH/ionic strength-induced solution behaviour analysis was presented [40]. We propose that such an oxidative-stress related aggregation-dependent mechanism can play role in the observed effect of the rise in the intercellular space green fluorescence by Zn and its decrease by Cu in the Fontinalis antipyretica moss.
Most of the endogenous fluorescence recorded in our sample of living moss was in the red spectral region and reached maximum at 680 nm. This finding is in agreement with previous observations that the main fluorescence recorded naturally in all plants is derived from chlorophyll a, a light-absorbing pigment [15]. Chlorophyll fluorescence is highly sensitive to changes in the functional state and we employed it previously as a biosensing tool for tracking algae responsiveness to environmental modulators [20]. In the presented study, heavy metal NPs Co and Cu significantly lowered the chlorophyll fluorescence intensity. A comparable effect was previously noted in the Chlorella sp. algae after 2 weeks exposure to NPs [31]. It is well known that heavy metals change the chlorophyll fluorescence yield and content of photosynthetic pigments representing sensitive indicators of environmental stress [41]. The toxic effect of Cu was proposed to be due to its oxidative potential causing the reduction of chlorophylls, leading to a decrease of oxygen rates and depletion of ATP. The observed effect of Cu can also be related to the fact that the Cu was demonstrated to have a higher adsorption capacity than Ni and Zn in the moss [42]. On the other hand, Co NPs were shown to inhibit fresh water algal bloom by decreasing super oxide dismutase (SOD) activity with increased NPs concentration, indicating the formation of stress in the microalgae [29]. Notwithstanding, even though cellular alterations were described by NiO NPs, including effects on the photosynthetic apparatus via chlorophyll synthesis, photochemical reactions of photosynthesis and oxidative stress [28], we noted little or no effect of the Ni NPs on the overall fluorescence intensity. This indicates lesser sensitivity of the moss to Ni and/or the need for longer exposure times.
In order to better understand the action of NPs on the red chlorophyll fluorescence, we have employed time-resolved fluorescence imaging microscopy. Fluorescence lifetimes depend on multiple factors that include pH, temperature, oxygenation, density, as well as presence or absence of quenchers {reviewed in [43]} and its use represents an important instrumental advancement. Temporal characteristics of the endogenous fluorescence served as a biomarker for changing environment, namely evidencing Cd toxicity noninvasively in algal cells [44]. In this contribution, FLIM recordings showed that most of the fluorescence signal in the Fontinalis antipyretica was situated inside the cell chloroplasts of the moss leaves. In control conditions, moss leafs exhibited short fluorescence lifetimes (200–350 ps), which is in agreement with others [36, 45–47], as well as with our findings in algae [19, 20]. In pine wood middle lamella, lignin was also shown to have a 300 ns lifetime. However, in our settings, FLIM images did not reveal fluorescence in the intercellular space due to its weak fluorescence when compared to chlorophylls; time-resolved fluorescence evaluated in this contribution are therefore related mostly to chlorophylls.
Following exposure to all NPs but Zn, we noted changes in the fluorescence lifetimes. More precisely, the ratio of a1/a2 components, fixed at 100 and 350 ps, was modified: while in control conditions, the a2 component was prevailing, in the presence of Ni, Co and mainly Cu, the contribution of the a1 (100 ps) component became more pronounced. Surprisingly, this modification was also observed in the presence of Ni, where the overall fluorescence was unchanged, suggesting higher sensitivity of the fluorescence lifetimes to environmental stress than that of the overall fluorescence. Shortening of the chlorophyll fluorescence lifetime was noted following water stress in plants [48]. Our previous results in algae demonstrated a decrease in the chlorophyll fluorescence with acidification, related to the shortening of the fluorescence lifetimes [20]. Heavy metals are known to affect the chloroplast ultrastructure, causing lipid peroxidation in photosynthetic membranes, degradation of photosynthetic pigments, inhibition of photosystem II (PSII) activity and electron transport, thus restraining the net photosynthetic rate [49]. Previously, we have demonstrated the sensitivity of marine algae Dunalliela tertiolecta to laboratory-induced stress with heavy metal cadmium [19, 33], with the role of stress-related light-harvesting complexes (LHC). The comparable effect can also be involved in the observed actions, but the exact mechanisms involved still remain to be elucidated. Nevertheless, our findings indicate that the observed change in the fluorescence lifetime component ratio, induced by heavy metals, can be employed for tracing their presence in the sweet water environment.
One of the problems faced in detecting metal ions with fluorescent sensors is the emission suppressing effects of some metal ions, which results in strong quenching of the fluorescence. Inherently quenching metal ions such as Hg2+, Cu2+, Co2+, Ni2+, and Fe3+ can interfere with the fluorescent signal of the sensors in detecting other metals [50]. Non-photochemical quenching of the chlorophyll fluorescence, described in the presence of heavy metals [38, 51], can a play role in the observed effect of the decrease in the fluorescence by both Co and Cu, accompanied by a rise in the contribution of the shorter fluorescence lifetime component a1. At the same time, our experiments demonstrate accumulation of the NPs mainly in the intercellular space, while the red fluorescence was derived from chloroplasts inside the cells. This fact, together with the lack of changes in the fluorescence lifetime during the decrease in the red chlorophyll fluorescence in the monocellular algae Chlorella sp. after NPs exposure [31] rather suggests an employment of intracellular pathways, the effect on LHC, PSII and/or modification in the intrachloroplast environment in this action than a direct non-photochemical quenching. Various parameters, including pH, temperature and water quality were proposed to play role in the heavy metal adsorption by the Fontinalis antipyretica moss [38]. However, an exact mechanism explaining how the NPs enter and/or attach to the Fontinalis antipyretica moss leaves was not yet elucidated and needs to be the subject of future studies. Also, the concentration effects, as well as the time-dependence of the effects of NPs on the moss should be explored in more details.
Since heavy metals are nonbiodegradable, they accumulate in the environment and subsequently contaminate the food chain. This contamination poses a risk to environmental and human health. The biomass of the aquatic moss Fontinalis antipyretica was proposed to be suitable for the removal of Cd and Zn from aqueous solutions [39], as this natural material is widely available in most European lakes and rivers. An improved understanding of heavy metal uptake by plants from soil can thus help in promoting phytomining - plant-based eco-friendly mining of metals, which can be used for the extraction of metals even from low-grade ores [52]. Data gathered in this contribution demonstrate the fast capacity of the Fontinalis antipyretica moss to reabsorb heavy metals, including small size NPs, from the environment. It can thus be considered as a sorbent for purification of metal-bearing wastewaters and play role in water cleansing and remediation.
In conclusion, we present the application of spectrally- and time-resolved imaging of endogenous fluorescence in biosensing of environmental pollution by heavy metal NPs (Zn, Ni, Co, Cu). Gathered results demonstrate the sensitivity of both, the green flavonoid and/or lignin fluorescence and the red chlorophyll fluorescence of Fontinalis antipyretica moss to the short (3–5 day) presence of various heavy metal (mostly Zn, Co and Cu) NPs. The action of all NPs but Zn also uncovered changes in the fluorescence lifetimes of the red chlorophyll fluorescence, suggesting an effect on the molecular environment, as well as increased sensitivity of this parameter to environmental stress. Our work is the first step towards fast and non-invasive biosensing of environmental pollution in living moss cells and their potential use in remediation.
The raw data supporting the conclusions of this article will be made available by the authors, without undue reservation.
All authors contributed equally to the paper. MCA overviewed the work presented in the ms, performed key experiments together with their analysis and wrote the ms. CD participated in the preparation of setups and experimental protocols, as well as in the data analysis and UM performed a large part of experiments and analysed primarily the FLIM data.
This publication was supported by the Grant Agency of the Ministry of Education, Science, Research and Sports of the Slovak Republic under the contract No. VEGA 2/0070/21. This work also received funding from the European Union's Horizon 2020 research and innovation programme under the grant agreement no 871124 Laserlab-Europe. The authors would like to thank T. Teplicky and J. Bruncko for their help with NPs preparation.
The authors declare that the research was conducted in the absence of any commercial or financial relationships that could be construed as a potential conflict of interest.
The Supplementary Material for this article can be found online at: https://www.frontiersin.org/articles/10.3389/fphy.2021.634324/full#supplementary-material.
1. Mohammed AS, Kapri A, Goel R. Heavy metal pollution: source, impact, and remedies. In: M Khan, A Zaidi, R Goel, and J Musarrat, editors. Biomanagement of metal-contaminated soils. Environmental pollution, 20. Dordrecht: Springer (2011) doi:10.1007/978-94-007-1914-9_1
2. Srivastava NK, Majumder CB. Novel biofiltration methods for the treatment of heavy metals from industrial wastewater. J Hazard Mater (2008) 151:1–8. doi:10.1016/j.jhazmat.2007.09.101
3. Jadoon S, Malik A. DNA damage by heavy metals in animals and human beings: an overview. Biochem Pharmacol (2017) 06:1000235. doi:10.4172/2167-0501.1000235
4. Hoet PH, Brüske-Hohlfeld I, Salata OV. Nanoparticles – known and unknown health risks. J Nanobiotechnol (2004) 2:12. doi:10.1186/1477-3155-2-12
5. Jaishankar M, Tseten T, Anbalagan N, Mathew BB, Beeregowda KN. Toxicity, mechanism and health effects of some heavy metals. Interdiscip Toxicol (2014) 7(2):60–72. doi:10.2478/intox-2014-0009
6. Wilde EW, Benemann JR. Bioremoval of heavy metals by the use of microalgae. Biotechnol Adv (1993) 11:781–812. doi:10.1016/0734-9750(93)90003-6
7. Parys E, Romanowska E, Siedlecka M, Poskuta JW. The effect of lead on photosynthesis and respiration in detached leaves and in mesophyll protoplasts of Pisum sativum. Acta Physiol Plant (1998) 20:313. doi:10.1007/s11738-998-0064-7
8. Prasad MNV. Heavy metal stress in plants - from biomolecules to ecosystems. Berlin, Germany: Springer-Verlag (2004).
9. Cenci RM. The use of aquatic moss (Fontinalis antipyretica) as monitor of contamination in standing and running waters: litmits and advantages. J Limnol (2001) 60: 53–61. doi:10.4081/jlimnol.2001.s1.53
10. de Traubenberg C, Ah-Peng C. A procedure to purify and culture a clonal strain of the aquatic moss Fontinalis antipyretica for use as a bioindicator of heavy metals. Arch Environ Contam Toxicol (2004) 46:289–295. doi:10.1007/s00244-003-3040-7
11. Bruns I, Friese K, Markert B, Krauss G-J. The use of Fontinalis antipyretica L. ex Hedw. as a bioindicator for heavy metals. 2. Heavy metal accumulation and physiological reaction of Fontinalis antipyretica L. ex Hedw. in active biomonitoring in the River Elbe. Sci The Total Environ (1997) 204(2):161–76. doi:10.1016/S0048-9697(97)00174-5
12. Rau S, Miersch J, Neumann D, Weber E, Krauss G-J. Biochemical responses of the aquatic moss Fontinalis antipyretica to Cd, Cu, Pb and Zn determined by chlorophyll fluorescence and protein levels. Environ Exp Bot (2007) 59(3):299–306. doi:10.1016/j.envexpbot.2006.03.001
13. Chorvatova A, Chorvat D Tissue fluorophores and their spectroscopic characteristics. In: L Marcu, PMW French, and DSV Elson, editors. Fluorescence lifetime spectroscopy and imaging for tissue biomedical diagnostics. Boca Raton, FL: CRC Press Publ (2014). 47–84. doi:10.1201/b17018-5
14. Becker W. Advanced time-correlated single photon counting applications. New York, NY: Springer (2015). doi:10.1007/978-3-319-14929-5
15. Govindjee G, Rabinowitch E Chlorophyll fluorescence and photosynthesis. In: GG Guilbaut, editor. Fluorescence instrumentation and practice. Newyork, NY: Marcel Dekker Inc. (1967). p. 511–64.
16. Cerovic ZG, Bergher M, Goulas Y, Tosti S, Moya I. Simultaneous measurement of changes in red and blue fluorescence in illuminated isolated chloroplasts and leaf pieces: the contribution of NADPH to the blue fluorescence signal. Photosynth Res (1993) 36:193–204. doi:10.1007/BF00033038
17. Cerovic ZG, Langrand E, Latouche G, Morales F, Moya I. Spectral characterization of NAD(P)H fluorescence in intact isolated chloroplasts and leaves: effect of chlorophyll concentration on reabsorption of blue-green fluorescence. Photosynth Res (1998) 56:291–301. doi:10.1023/a:1006081209887
18. Donaldson L Autofluorescence in plants. Molecules (2020) 25(10):2393. doi:10.3390/molecules25102393
19. Pavlinska Z, Chorvat D, Mateasik A, Jerigova M, Velic D, Ivošević DeNardis N, et al. Fluorescence responsiveness of unicellular marine algae Dunaliella to stressors under laboratory conditions. J Biotechnol X (2020) 6:100018. doi:10.1016/j.btecx.2020.100018
20. Marcek Chorvatova A, Uherek M, Mateasik A, Chorvat D Time-resolved endogenous chlorophyll fluorescence sensitivity to pH: study on Chlorella sp. algae. Methods Appl Fluoresc (2020b) 8:024007. doi:10.1088/2050-6120/ab77f4
21. Teplicky T, Chorvat D, Michalka M, Chorvatova AM. Preparation of metal nanoparticles by femtosecond laser ablation. Nova Biotechnologica Chim (2018) 17(1):38–47. doi:10.2478/nbec-2018-0004
22. Gupta SM, Tripathi M. A review of TiO2 nanoparticles. Chin Sci Bull (2011) 56:1639–57. doi:10.1007/s11434-011-4476-1
23. Kim JS, Kuk E, Yu KN, Kim J-H, Park SJ, Lee HJ, et al. Antimicrobial effects of silver nanoparticles. Nanomedicine: Nanotechnology, Biol Med (2007) 3(1):95–101. doi:10.1016/j.nano.2006.12.001
24. Langer J, Novikov SM, Liz-Marzán LM. Sensing using plasmonic nanostructures and nanoparticles. Nanotechnology (2015) 26(32):322001. doi:10.1088/0957-4484/26/32/322001
25. Padmavathy N, Vijayaraghavan R. Enhanced bioactivity of ZnO nanoparticles-an antimicrobial study. Sci Technol Adv Mater (2008) 9(3):035004. doi:10.1088/1468-6996/9/3/035004
26. Li M, Ahammed GJ, Li C, Bao X, Yu J, Huang C, et al. Brassinosteroid ameliorates zinc oxide nanoparticles-induced oxidative stress by improving antioxidant potential and redox homeostasis in tomato seedling. Front Plant Sci (2016) 7:615. doi:10.3389/fpls.2016.00615
27. El-Kassas HY, Okbah MAE-A. Phytotoxic effects of seaweed mediated copper nanoparticles against the harmful alga: lyngbya majuscula. J Genet Eng Biotechnol (2017) 15(1):41–8. doi:10.1016/j.jgeb.2017.01.002
28. Oukarroum A, Zaidi W, Samadi M, Dewez D. Toxicity of nickel oxide nanoparticles on a freshwater green algal strain of Chlorella vulgaris. Biomedresearch Int (2017) 8:9528180. doi:10.1155/2017/9528180
29. Anusha L, Chingangbam Sushmita D, Sibi G. Inhibition effects of Cobalt nano particles against fresh water algal blooms caused by microcystis and oscillatoria. Am J Appl Scientific Res (2017) 3(4):26–32. doi:10.11648/j.ajasr.20170304.12
30. Bajguz A. Suppression of Chlorella vulgaris growth by cadmium, lead, and copper stress and its restoration by endogenous brassinolide growth by cadmium, lead, and copper stress and its restoration by endogenous brassinolide. Arch Environ Contam Toxicol (2011) 60(3):406–16. doi:10.1007/s00244-010-9551-0
31. Marcek Chorvatova A, Teplicky T, Valica M, Mateasik A, Chorvat D Biosensing the presence of nanoparticles using endogenous fluorescence in live algae. In: AN Cartwright, and DV Nicolau, editors. Proceedings of SPIE on Nanoscale imaging, sensing and actuation for biomedical applications XVII book series. 11254 (2020a). p. 1125402–1. doi:10.1117/12.2543332
32. Alkorta I, Hernández-Allica J, Becerril JM, Amezaga I, Albizu I, Garbisu C. Recent findings on the phytoremediation of soils contaminated with environmentally toxic heavy metals and metalloids such as zinc, cadmium, lead, and arsenic. Re/Views Environ Sci Bio/Technology (2004) 3:71–90. doi:10.1023/b:resb.0000040059.70899.3d
33. Ivošević DeNardis N, Pečar Ilić J, Ružić I, Novosel N, Mišić Radić T, Weber A, et al. Algal cell response to laboratory-induced cadmium stress: a multimethod approach. Eur.Biophys J (2019) 48(2):124–42. doi:10.1007/s00249-019-01347-6
34. Marcek Chorvatova A, Teplicky T, Pavlinska Z, Kronekova Z, Trelova D, Razga , et al. A bio-inspired design of live-cell biosensors. Proc SPIE (2018) 10506:105060R–1. doi:10.1117/12.2288789
35. Xue Y, Qiu X, Wu Y, Qian Y, Zhou M, Deng Y, et al. Aggregation-induced emission: the origin of lignin fluorescence Polymer chemistry. Polym Chem (2016) 7:3502–8. doi:10.1039/C6PY00244G
36. Kristoffersen AS, Hamre B, Frette Ø, Erga SR. Chlorophyll a fluorescence lifetime reveals reversible UV-induced photosynthetic activity in the green algae Tetraselmis. Eur Biophys J (2016) 45:259–68. doi:10.1007/s00249-015-1092-z
37. Martínez-Abaigar J, Nuñez-Olivera E, Crespo MA, Tomas R, Beaucourt N, Otero S. Effects ultraviolet Radiat Aquat bryophytes Limnetica (2006) 25(1-2):81–94. doi:10.23818/limn.25.06
38. Chen Y-E, Wu N, Zhang Z-W, Yuan M, Yuan S. Perspective of monitoring heavy metals by moss visible chlorophyll fluorescence parameters. Front Plant Sci (2019) 10(35):1–7. doi:10.3389/fpls.2019.00035
39. Martins RJE, Pardo R, Boaventura RAR. Cadmium(II) and zinc(II) adsorption by the aquatic moss Fontinalis antipyretica: effect of temperature, pH and water hardness. Water Res (2004) 38(3):693–9. doi:10.1016/j.watres.2003.10.013
40. Xue Y, Qiu X, Ouyang X. Insights into the effect of aggregation on lignin fluorescence and its application for microstructure analysis. Int J Biol Macromolecules (2020) 154:981–8. doi:10.1016/j.ijbiomac.2020.03.056
41. Lichtenthaler HK, Miehé JA Fluorescence imaging as a diagnostic tool for plant stressRemoval of copper from solution using moss. Trends in Plant ScienceEnviron Technology Lett (1997) 2:316–20. doi:10.1016/s1360-1385(97)89954-242
42. Lee CK, Low KS. Removal of copper from solution using moss. Environ Technology Lett (1989) 10(4):395–404. doi:10.1080/09593338909384755
43. Berezin MY, Achilefu S Fluorescence lifetime measurements and biological imaging. Chem Rev (2010) 110(5):2641–84. doi:10.1021/cr900343z
44. Zeng Y, Wu Y, Li D, Zheng W, Wang W-X, Qu JY. Two-photon excitation chlorophyll fluorescence lifetime imaging: a rapid and noninvasive method for in vivo assessment of cadmium toxicity in a marine diatom Thalassiosira weissflogii. Planta (2012) 236(5):1653–63. doi:10.1007/s00425-012-1703-1
45. Singhal GS, Rabinowitch E, Camenen L, Goulas YGG, Cerovic ZG, Schmuck G. Measurement of the fluorescence lifetime of chlorophyll a in vivo. Biophysical JRemote Sens Environ (1969) 9:586–91. doi:10.1016/S0006-3495(69)86405-2
46. Moya I. Estimation of the chlorophyll fluorescence lifetime of plant canopies: validation of a deconvolution method based on the use of a 3-D Canopy Mockup58157168. Remote Sens Environ (1996) 58:157–68.
47. Nordlund TM, Knox WH. Lifetime of fluorescence from light-harvesting chlorophyll a/b proteins. Excitation intensity dependence. Biophysical J (1981) 36(1):193–201. doi:10.1016/S0006-3495(81)84723-6
48. Schmuck G, Moya I, Pedrini A, van der Linde D, Lichtenthaler HK, Stober F, et al. Chlorophyll fluorescence lifetime determination of waterstressed C3- and C4-plants. Radiat Environ Biophys (1992) 31:141–51. doi:10.1007/bf01211212
49. Mishra S, Dubey R. Heavy metal toxicity induced alterations in photosynthetic metabolism in plants. In: M Pessarakli, editor. Handbook of photosynthesis. Boca Raton, FL: CRC Press (2005). p. 827–44.
50. Tan SS, Kim SJ, Kool ET. Differentiating between fluorescence-quenching metal ions with polyfluorophore sensors built on a DNA backbone. J Am Chem Soc (2011) 133(8):2664–71. doi:10.1021/ja109561e
51. Niyogi KK, Truong TB. Evolution of flexible non-photochemical quenching mechanisms that regulate light harvesting in oxygenic photosynthesis. Curr Opin Plant Biol (2013) 16:307–14. doi:10.1016/j.pbi.2013.03.011
Keywords: Autofluorescence (AF), FLIM (fluorescence lifetime imaging microscopy), nanoparticle (NP), chlorophyll, moss, heavy metal, biosensor
Citation: Marcek Chorvatova A, Uherek M and Chorvat D (2021) Biosensing the Presence of Metal Nanoparticles by Spectrally- and Time-Resolved Endogenous Fluorescence in Water Moss Fontinalis antipyretica. Front. Phys. 9:634324. doi: 10.3389/fphy.2021.634324
Received: 27 November 2020; Accepted: 18 January 2021;
Published: 12 April 2021.
Edited by:
Klaus Suhling, King's College London, United KingdomReviewed by:
Saikiran Vadavalli, Gandhi Institute of Technology and Management (GITAM), IndiaCopyright © 2021 Marcek Chorvatova, Uherek and Chorvat. This is an open-access article distributed under the terms of the Creative Commons Attribution License (CC BY). The use, distribution or reproduction in other forums is permitted, provided the original author(s) and the copyright owner(s) are credited and that the original publication in this journal is cited, in accordance with accepted academic practice. No use, distribution or reproduction is permitted which does not comply with these terms.
*Correspondence: Alzbeta Marcek Chorvatova, YWx6YmV0YS5tYXJjZWtjaG9ydmF0b3ZhQGN2dGlzci5zaw==
Disclaimer: All claims expressed in this article are solely those of the authors and do not necessarily represent those of their affiliated organizations, or those of the publisher, the editors and the reviewers. Any product that may be evaluated in this article or claim that may be made by its manufacturer is not guaranteed or endorsed by the publisher.
Research integrity at Frontiers
Learn more about the work of our research integrity team to safeguard the quality of each article we publish.