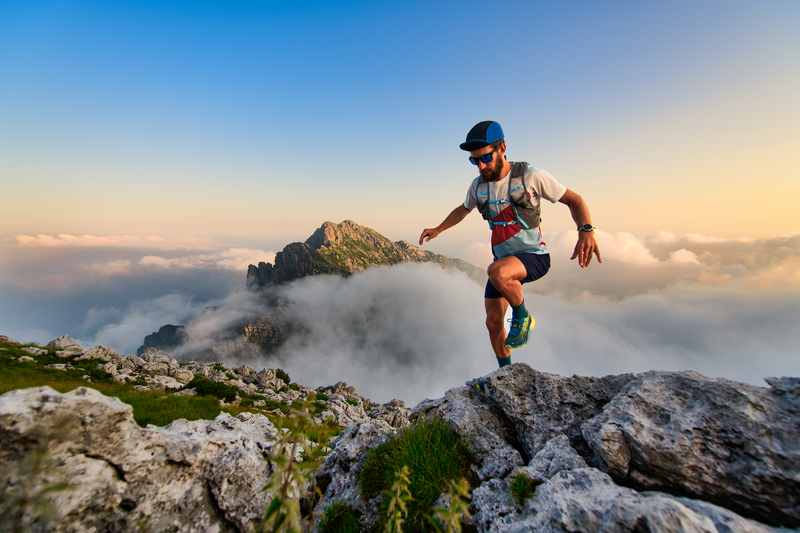
95% of researchers rate our articles as excellent or good
Learn more about the work of our research integrity team to safeguard the quality of each article we publish.
Find out more
ORIGINAL RESEARCH article
Front. Phys. , 13 November 2020
Sec. Medical Physics and Imaging
Volume 8 - 2020 | https://doi.org/10.3389/fphy.2020.564907
This article is part of the Research Topic Applied Nuclear Physics at Accelerators View all 57 articles
The main effort of the laser-driven ion acceleration community is aimed at improving particle beam features (in terms of final maximum energy, particle charge, and beam divergence) and to demonstrate reliable approaches for use for multidisciplinary applications. An ion acceleration target area based on unique laser capabilities is available at ELI-Beamlines (Extreme Light Infrastructure) in the Czech Republic; it is called ELIMAIA (ELI Multidisciplinary Applications of laser-Ion Acceleration) and aims to provide stable and characterized beams of particles accelerated by high-power lasers to offer them to the user community for interdisciplinary studies. The ELIMAIA section dedicated to ion focusing, selection, characterization, and irradiation is named ELIMED (ELI MEDical and multidisciplinary applications). Thanks to ELIMED, very high-dose-rate (around Gy/min) controlled proton and ion beams, with energy levels ranging from 5 to 250 MeV, will be transported to an in-air section dedicated to absolute and relative dosimetry of the laser-generated ions. A transmission dual-gap air ionization chamber will allow an on-line, non-destructive characterization of the ion dose at the user sample irradiation point. The uncertainty in the final dose released onto the sample is expected to be well below 5%. An ELIMED radiobiology pilot experiment is scheduled for 2021, during which in-vitro cell irradiations will be carried out with well-controlled proton beams. In this work, the status of the ELIMED/ELIMAIA beamline will be described along with a complete definition of the main dosimetric systems and of their calibrations carried out at conventional accelerators.
Ion acceleration driven by pulsed laser-plasma sources is a rapidly emerging field of Physics resulting from recent high-power laser technology achievements in terms of ultra-high intensities (> 1021 W/cm2) reached on target. This has allowed us to explore extreme regimes of laser-matter interaction. Ultra-high electric and magnetic fields are generated in the laser-target interaction, and plasma electrons (known as hot electrons) are accelerated to relativistic energies in a very compact approach (um-scale). Typically, such a plasma electron heating mechanism leads to the acceleration of high-energy ion beams from a thin solid target (um-thick foil) based on a sheath field, which is created on the target's rear surface. Charge separation and proton/ion acceleration occurs for a very short time, and ions and electrons ultimately co-propagate in vacuum. Recent experiments have recorded proton energies around 100 MeV [1]. However, laser-driven ion beams are still not ready for societal applications, which require advanced beam parameters in terms of divergence, energy spread, homogeneity, and stability. Newly developed laser technologies based on diode-pumped lasers, such as various systems recently installed at ELI-Beamlines [2], will enable users to explore new laser-plasma acceleration schemes of interest for multidisciplinary applications. However, for such novel high peak power (1–10 PW), high repetition rate (up to 10 Hz) laser systems will have to demonstrate that the key challenge to progressing laser-driven ion sources from the realms of scientific curiosity to applications of benefit for the economy and society, i.e., high rep-rated capability and stability both of the laser and the particle sources, is successfully solved.
Hadrontherapy is a radiation therapy technique used for cancer treatment known for its high ballistic precision and radiobiological effectiveness, which is peculiar for hadrons with respect to electrons and gamma-rays [3, 4]. The idea of using hadrons for cancer treatment was first proposed in 1946 by the physicist Robert Wilson. The first patients were treated in the 1950s in nuclear physics research facilities by means of non-dedicated accelerators. Initially, the clinical applications were limited to few parts of the body, as accelerators were not powerful enough to allow protons to penetrate deep in the tissues. In the late 1970s, improvements in accelerator technology, coupled with advances in medical imaging and computing, made proton therapy a viable option for routine medical applications. However, only in the beginning of the 1990s were proton facilities established in clinical settings, the first of these being in Loma Linda, USA. Currently, about 30 proton centers are either in operation or in construction worldwide. Although protons are used in several hospitals, the next step in radiation therapy is the use of carbon and other ions. These have some clear advantages even over protons in providing both local control of very aggressive tumors and a lower acute or late toxicity, thus enhancing the quality of life during and after cancer treatment. Since the birth of hadrontherapy, more than 120,000 patients have been treated globally with hadrons, including 20,000 with carbon ions. In Europe, the interest in hadrontherapy has grown rapidly, and the first dual ion (carbon and protons) clinical facility in Heidelberg, Germany, started treating patients in 2009. Three more of such facilities are now in operation treating patients: CNAO in Pavia, MIT in Marburg, and MedAustron in Wiener Neustadt. Globally, there is a huge momentum within particle therapy, especially concerning treatment with protons. By 2020, it was expected there would be almost 100 centers around the world with over 30 in Europe. Even if within expansion, the number of hadrontherapy centers worldwide is still limited mainly due to the associated large costs for implementation and operation; this stems not only from the need of sophisticate acceleration systems (particularly for heavier ions) but also for the complexity of ion beam delivery systems (known as “gantries”), radiation protection (shielding), and facility management. One of the main objectives of the scientific community working in the field of laser-plasma ion acceleration is to demonstrate stable, flexible and compact (cost-effective) acceleration schemes for potential societal applications [5]. Moreover, a laser-plasma source offers the possibility of simultaneous delivery of particle (ions and electrons) and photon (X/gamma rays) beams, thus enabling hybrid approaches of interest to cancer therapy [6].
The objective of the laser-driven ion target area ELIMAIA (ELI Multidisciplinary Applications of laser-Ion Acceleration) at ELI-Beamlines (Extreme Light Infrastructure) is to provide beams of particles accelerated by petawatt-class lasers suitable for multidisciplinary applications. Ion beams produced and accelerated in laser-matter interaction processes show high intensities, several ion charge states, broad energy spectra, and energy-dependent angular distributions. As a consequence, a beamline for laser-accelerated ions must be able to define and control particle energy and angular distribution. The ELIMAIA beamline consists of two principal subsystems: the Ion Accelerator section and the ELI MEDical applications (ELIMED) section. ELIMED, in turn, consists of three main parts: ion collection and focusing, ion energy selection, and in-air transport [7, 8]. The goal of the collection and focusing section is to collimate the ion beam and to reduce its initial high divergence. The focusing element is composed of a set of permanent magnet quadrupoles (PMQs). Quadrupoles are necessary to obtain a focusing effect on both transversal planes as well as for suitable matching with the acceptance of the following selection system. The energy selector system (ESS) consists of four tunable resistive dipoles arranged with alternating fields and whose magnetic characteristics can be varied depending on the energy and ion species to be selected. A rectangular aperture, locate after the second dipole, allows us to control the beam energy spread. The transported ion and proton beams are monitored along the beamline with several diagnostics based on solid-state detectors. In particular, diamond and silicon carbide (SiC) are extensively used along the beamline in a time-of-flight configuration (TOF) [9, 10]. The possibility to perform accurate shot-to-shot measurements of the dose released to user samples is a key feature guaranteed by the ELIMED diagnostic systems. In particular, ELIMED's absolute dosimetry system has been created to be independent from dose-rate and to allow on-line dosimetric evaluation with an error rate below 5%. The ELIMED dosimetry system is based on three main devices: a Secondary Electron Monitoring (SEM), a Multi-Gaps Ionization Chamber (MGIC), and a Faraday Cup (FC) for absolute dosimetry. Passive detectors, such as CR39 and radiochromic films (RCF), are also used to benchmark active ion diagnostic and dosimetry devices.
Figure 1 shows a photo of the ELIMAIA beamline. The Ion Accelerator section is visible in the photo only as a large laser-target interaction vacuum chamber (top-left), and laser/plasma optical and diagnostic devices for the two available PW-class lasers are not visible. Since the basic features of laser-driven ions are adverse to efficient beam manipulation (large divergence and energy spread), the use of several beam transport stages is mandatory. The ELIMED ion beam transport section is entirely shown in Figure 1 (blue and orange color). It consists of three main sub-systems. The Ion Collection section, located in a dedicated vacuum chamber close to the laser-target interaction point, is used to capture ions within a certain energy range, inject them into the energy selection system, and also provide a rough energy selection capability. This section consists of a set of five PMQs with high magnetic field gradient of 50 T/m [11, 12] (1.8 T over a 36 mm magnetic bore) placed on a displacement system to adjust the beam optical elements for different ion energies based on the energy selection system (ESS) configuration. Ions with a given energy spread are focused into a position where the energy selection occurs (radial plane) and their angular aperture is reduced angular aperture in the transverse plane, which is as required for an optimal matching between the acceptance and the transmission efficiency of the ESS. This device consists of a double dispersive mode magnetic chicane, made of four C-shaped electromagnetic dipoles with laminated core and a selection slit set in the middle plane [13], to cut the undesired energy components of incoming ions. The ELIMED ESS can select protons with 300 MeV maximum energy and heavier ions with 60 MeV per nucleon. The chicane is based on a fixed reference trajectory with variable magnetic field (0.063–1.22 T) to cover a large expected ion energy range. The energy spread of the selected ion beam has a linear dependence on the slit aperture (10 mm aperture corresponds to a spread of 10% FWHM). After the chicane, the ion beam is delivered to a final beam shaping section, which consists of two resistive quadrupoles (gradients up to 10 T/m over a 50 mm bore) and two correction elements for a fine focusing and alignment of the ion beam onto the user sample. The use of a laminated core for the electromagnets guarantees a fast change in the magnetic field, reducing the hysteresis effect. The whole ELIMED beamline, but the collection section, can thus be used as an active energy modulator. The possibility to carry out high resolution on-axis ion spectrometry has recently been investigated and is being implemented as an upgrade of the ESS, which will be used as a combined device: a selection chicane (as described) or a Thomson Parabola spectrometer using the first dipole and a set of electrodes placed inside the selector chamber. A feasibility study proposed in [14] shows the main feature of the upgraded chicane and the diagnostic performances in the case of its use as a Thomson Parabola spectrometer. It basically consists of the first dipole in the chicane used as a magnetic deflector plus two electrostatic deflectors to be installed in the vacuum chamber. In this way, the spectrometer will have a sector with a wide dynamic range and wide charge separation but relatively low energy resolution of about 10.
Figure 1. ELIMED ion beam line connected to the Interaction chamber (first chamber from the left), where the laser-acceleration occurs and where the collection systems sits. It follows the ESS and the beam shaping section. After this last section, the beam is delivered in the in-air dosimetry station.
The whole ELIMED beamline for laser-driven ion beam transport and characterization (including detectors for ion diagnostics and dosimetry) can be modeled by numerical simulations using the Geant4 Monte Carlo toolkit [15–17].
The ELIMED beamline simulation [18–20] is a powerful and reliable tool with which to predict the dosimetric parameters as well as the biological effects of high-dose-rate, pulsed ion beams on the human body. The ELIMED simulation software is divided into three different parts following the three main beamline sections as described in section 2.1: a part related to ion beam collection and diagnostics, one related to ion beam selection and transport, and finally a section related to ion beam dosimetry and samples irradiation [7]. A sketch of the ELIMED beamline as simulated by the Geant4 application is reported in Figure 2. Accurate magnetic field maps, obtained with the COMSOL and OPERA software for each quadrupole, are included in the application for a realistic simulation of the focusing effect by an accurate implementation of the high field gradients. A grid of the magnetic field maps for each single dipole of the ESS is also included in the ESS simulation.
The dosimetric system has been realized to meet the specific requirements of radiobiological experiments that cannot accept uncertainties above 5% in the absorbed dose measured at each shot. The definition of a protocol (including the detectors to be used, methods, and procedures) for absolute and relative dosimetry is, in fact, mandatory for the future clinical use of laser-driven proton beams in order to obtain absolute dose measurements with an accuracy and a precision comparable to what is typically required in clinical applications. The system must be suitable for operation with pulsed, high-intensity beams also in the presence of a strong electromagnetic noise associated with the interaction of ultra-high intensity lasers with a plasma target. All the detectors involved must be capable of measuring bunches of particles that are very short in time (1–10 ns) and very intense (typically 108 particle per bunch at the sample/detector). In order to accomplish this task, fast, low-noise, and variable-gains charge amplifiers were designed and realized at INFN-LNS and installed at ELI-Beamlines. The developed amplifier will allow to measure the total charge in each dosimetric system device at a repetition rate of 1 Hz, simultaneously minimizing the error.
This system includes three main devices: a secondary emission monitor, a multi-gap in-transmission ionization chamber for relative dose measurements, and a Faraday cup, which is designed to carry out absolute dosimetry. A sample irradiation system with sub-mm precision, dedicated to user samples (e.g., biological cells), is located at the end of the in-air section. Figure 3 shows a general outlook of the ELIMED beamline dosimetric section. The SEM detector, positioned in-vacuum just before the in-air section, is made of a thin (15 μm thick) tantalum foil. It is electrically insulated from the rest of the beamline by a polymethylmethacrylate (PMMA) frame. An actuator allows to move the SEM detector in/out the beamline axis. The SEM operating principle is based on the measurement of secondary electron emission (SEE) generated when multi-MeV charged particles pass through the tantalum foil. The detected signal is correlated to the incident beam current and can provide online information directly connected to the released dose at a given point if properly cross-calibrated against a reference dosimeter. The MGIC, developed by DE.TEC.TOR. S.r.l., Italy, is an innovative device realized for real-time dose monitoring delivered shot-to-shot. It consists of two consecutive ionization chambers, with a different inter-electrode gap of 5 and 10 mm, independently supplied with a voltage ranging from −1,000 to +1,000 V as well as −2,000 to +2,000 V, respectively. The presence of the second gap allows us, exploiting the different collection efficiency among the two chambers, to correct for any ion recombination effect caused by the expected extremely high dose rate (MGy-GGy per second). In order to overcome saturation effects typical of conventional dosimeters when used with laser-driven ions, an innovative FC has been designed and realized for absolute dosimetry. The FC was designed on the basis of previous works [21, 22], and the introduction of new geometrical solutions, aiming to optimize the charge collection efficiency and reduce uncertainties, related to the charge collection, which was explored by [23]. An additional electrode with a special beveled shape-coaxial and internal to the standard one in which a bias can be applied in the range between −2,000 and +2,000V, has been introduced. The final resulting electric field inside the FC is the combination of two field coming from the two coaxial electrodes. This special configuration of the electrodes produces an asymmetric electric field able to minimize the negative effects of the secondary electrons generated by the interaction of protons with the thin entrance window and the cup material itself. The beam energy spectra and the surface of its spot on the FC are necessary for the dose evaluation and will be measured using stacks of radiochromic films. They can be used both as a single detector, to precisely measure the transversal dose distributions profiles, or in stack configuration, in order to obtain information on the energy of the accelerated protons through an iterative procedure based on deconvolution methods (see section 2.4). The use of RCF films, if properly calibrated, also allows for an accurate measurement of the released dose distributions at the irradiation point with a spatial resolution less than 200 μm [24]. Solid state nuclear track detectors (CR-39 type) will also be used, representing a useful tool for charged particle fluences and spectroscopic analysis by measuring the track diameter arising from incident particles and extracting the corresponding energy from a suitable calibration curve [25].
Figure 3. Dosimetric section of ELIMED beamline: SEM and MG ionization chambers compose the relative dosimetry section, and FC is the element devoted to the absolute dosimetry.
The FC was firstly characterized with conventional (not pulsed and with intensities of the order of 400 pA) proton beams in terms of accuracy in absolute dose estimation during an experimental campaign carried out at the Zero Degree beamline of the INFN-LNS laboratory (Catania, Italy) [26]. The detector was positioned at the beamline isocenter and irradiated with a 35 MeV proton beam. The proton beam field was circular with a diameter of 15 mm. Size and homogeneity of the beam spot (in terms of space distribution) were measured for each experimental session using radiochromic film detectors (EBT3 type). The dose delivered was monitored online by an in-transmission ionization chamber previously calibrated with a Markus chamber (mod. 3002). A fixed dose of 5.13 ± 0.15 Gy was delivered for each measurement. The FC efficiency was evaluated by varying the voltage of the two electrodes. The working configuration corresponds to the maximum charge collection efficiency coupled with the minimum uncertainty due to the contribution of secondary electrons generated in the interaction between the beam and the detector itself [21]. The two-dimensional scatter plot of Figure 4 reports the percentage difference (expressed as a color gradient) between the dose measured by the FC and the dose released for each couple of applied voltage. The optimal configuration for which we register the minimal discrepancy corresponds to an electric field generated when −800 and +1,500 V are applied (circle indicated with the arrow) to the external and internal electrodes, respectively. In this case, the percentage discrepancy between the dose measured with the FC and the absolute dose monitored with the ionization chamber is 0.7%. Discrepancies up to 18% are observed for the other configurations.
Figure 4. Two dimensional scatter plot indicating the percentage difference between the dose measured by the Faraday Cup and the released dose. The discrepancy level is represented by a different color. The best configuration, obtained by applying the voltage of +1,500 to −800 V to the internal and external electrode, respectively, is indicated in the figure with a black arrow.
Radiochromic film is a dosimetric detection medium with high dynamic range and independent response from the incident dose rate. In general, RCF detectors contain one or more active layers of microcrystalline monometric dispersion buried in a plastic substrate [27, 28]. When an ionizing radiation hits it, the active material undergoes polymerization and changes its color with a degree of color conversion that, in a first approximation, is proportional to the absorbed dose. This makes possible the correlation (or calibration) between the absorbed dose and film darkening, which can be evaluated in terms of Optical Density (OD) [29]. The OD is the quantity able to quantify the intensity reduction of light transmitted through the film after the exposure. The correctness and precision in the OD calibration process represents the basis of an accurate measurement with an RCF. The OD value is obtained using a commercial scanner. RCFs arranged in a stack configuration can be used to detect the typical large divergence and continuous energy spectrum of laser-driven ion beams. The thickness of RCF is of the order of a hundred micrometers and can easily be crossed by protons of few MeVs. The stack consists of alternating layers of RCFs. Each layer allows detecting protons with different energies thus the entire spectrum of the incident proton beam can be reconstructed. In the framework of the ELIMED project, an analytical procedure able to reconstruct the incident energy spectra in a RCF stack was developed and validated [30]. The algorithm considers the dose read in the last irradiated layer of the stack and subtracts it from all previous layers applying specific weight coefficients for each layer. The whole process can be summarized the following:
where represent the dose matrix related to the primary protons on the k-th layer of the stack; N is the number of layers in a stack, is the dose matrix of the k-th layer taking into account all the traversing protons, and the ratio represents the fraction of energy loss in the k-th layer of the stack of primary protons able to reach the i-th layer (i>k), which was acquired adopting the SRIM simulation programme [31]. The is then converted in particle fluence through:
where A is the area of the selected ROI constituted by LxM pixels (l=1,7,…, L, m=1,…, M), and is the dose delivered by a single primary proton, and is calculated by SRIM. In order to verify the analysis approach above described, we tested the whole procedure irradiating the films with protons accelerated by the Superconducting Cyclotron available at Laboratori Nazionali del Sud-INFN (Catania, I). We positioned a stack of 31 RCF (EBT3 type) with the first film positioned at the isocenter of the CATANA experimental room; the stack was then irradiated with a monoenergetic beam of 35 MeV in energy. In Figure 5, the incident proton energy spectra derived with the described approach is shown.
Figure 5. Incident proton energy spectra obtained irradiating a stack of RCFs with 35 MeV monoenergetic beam of CATANA experimental room of LNS-INFN.
The most recent studies performed with laser-driven particle bunches aim to compare the characteristics of such novel beams with the ones available at conventional accelerators. This is a key requirement to establish and validate irradiation procedures in analogy with the ones already existing when user samples are irradiated with conventional sources. The ELIMED beamline, indeed, was designed and realized with the long-term goal of exploring medical applications and to obtain extremely high shot-to-shot reproducibility, high precision in the delivered dose, quasi-monoenergetic beam spectra at the biological sample, and reproducibility of the transported beams. These specific characteristics can be certainly exploited for various multidisciplinary applications other than the medical ones; one example is the broad (Boltzman-like) energy spectrum of laser accelerated ions [32]. Most radiation occurring in space has an exponential or power-law energy distribution that cannot easily be reproduced with conventional accelerators. Radiation exposure is regarded as one of the main problems in long-term manned space missions. In this framework, it can be worthwhile to investigate the effects of beams with broad energy distributions on biological samples as well as on electronic devices and materials, and this can be easily carried out at the user sample irradiation station of ELIMED. Laser-accelerated proton beams transported and selected at ELIMED could be also used as an innovative diagnostic technique in the field of Cultural Heritage. Adopting these beams could have considerable advantages over conventional PIXE (proton-induced X-ray emission), PIGE (proton-induced gamma-ray emission), and DPAA [33] (Depth Proton Activation Analysis) spectroscopy. Protons accelerated by laser-matter interaction have the advantage of allowing complete chemical analysis on a larger volume of the artworks and deeper and more precise “layer by layer” analysis [34]. Moreover, the future perspective to develop extremely feasible and portable PIXE systems based on laser-matter interaction can be studied and consolidated. Finally, a laser-driven ion acceleration user beamline could open the possibility to use plasma and ions generated in the high-power laser interaction for production of medical isotopes by innovative production schemes [35]. Lasers could provide many advanced features with respect to methods based on conventional accelerators, where target activation represents a strong limitation. Presently, two different isotope-based imaging techniques are used in medical clinics: SPECT (Single Photon Emission Computed Tomography) and PET (Positron Emission Tomography). Both are mainly based on 18F, 11C, 15O, and 13N decay produced by the interaction of protons with solid targets. ELIMED could be the first worldwide facility able to produce radioisotopes for imaging techniques as well as clinical proton beams with the same accelerator machine based on laser-target interaction [36]. The ELIMAIA beamline will additionally offer the possibility to explore innovative schemes of interaction between well-controlled and characterized ion beams (thanks to the ELIMED section) and high-density plasmas for pump-probe investigations of warm dense matter [37], fast ignition approach to inertial confinement fusion [38], and estimation of ion-stopping power in plasma [39].
The raw data supporting the conclusions of this article will be made available by the authors, without undue reservation.
GAPC and DM are the inventors of the ELIMAIA/ELIMED beamlines. DM is in charge of the laser beam transport and focusing, laser-target interaction, ion acceleration and diagnostics at the source, while GAPC is in charge of the ion beam transportation and selection, of the design of the in-air irradiation section of the beamline and of the dosimetry and diagnostic systems. GP, RC, GC, RL, AV, VS, and GMi were involved in the development and tests of the dosimetric systems. FS was the main designer of the electromagnetic transport elements. AR supported him in many aspects. GMi, FR, MZ, and JP were involved in the Monte Carlo and PIC simulations. LA, AA, RA, MC, AF, GG, LG, EL, GMe, SP, DR, SS, SV, and EZ performed the beamline installation at the ELI-Beamlines premise. GC and GK supervise the entire activity. All authors contributed to the article and approved the submitted version.
This work has been supported by the project ELI—Extreme Light Infrastructure—phase 2 (CZ.02.1.01/0.0/0.0/15-008/0000162) from European Regional Development Fund, by the Ministry of Education, Youth and Sports of the Czech Republic (Project No. LQ1606) and by the project Advanced Research Using High Intensity Laser Produced Photons and Particles (CZ.02.1.01/0.0/0.0/16-019/0000789). This work has also been financially supported by the ELIMED INFN project.
The authors declare that the research was conducted in the absence of any commercial or financial relationships that could be construed as a potential conflict of interest.
1. Higginson A, Gray RJ, King M, Dance RJ, Williamson SDR, Butler NMH, et al. Near-100 MeV protons via a laser-driven transparency-enhanced hybrid acceleration scheme. Nat Commun. (2018) 9:724. doi: 10.1038/s41467-018-03063-9
2. Hein J, Silva LO, Korn G, Gizzi LA, et al. Outline of the ELI-Beamlines facility. In: Diode-Pumped High Energy and High Power Lasers; ELI: Ultrarelativistic Laser-Matter Interactions and Petawatt Photonics; and HiPER: the European Pathway to Laser Energy. Prague (2011). 808010-1–808010-10.
3. Orecchia R, Zurlo A, Loasses A, Krengli M, Tosi G, Zurrida S, et al. Particle beam therapy (hadrontherapy): basis for interest and clinical experience. Eur J Cancer. (2008) 34:459–68. doi: 10.1016/S0959-8049(97)10044-2
4. Official page of the Particle Therapy Cooperative Group. An organisation for those interested in proton, light ion and heavy charged particle radiotherapy. Available online at: http://www.ptcog.ch//
5. Romano F, Schillaci F, Cirrone GAP, Cuttone G, Scuderi V, Allegra L, et al. The ELIMED transport and dosimetry beamline for laser-driven ion beams. Nuclear Instrum Methods Phys Res A. (2016) 829:153–8. doi: 10.1016/j.nima.2016.01.064
6. DeLaney TF, Liebsch NJ, Pedlow FX, Adams J, Dean S, Yeap BY, et al. Phase II study of high-dose photon/proton radiotherapy in the management of spine sarcomas. Int J Radiat Oncol Biol Phys. (2009) 74:732–9. doi: 10.1016/j.ijrobp.2008.08.058
7. Margarone D, Cirrone GAP, Cuttone G, Amico AG. ELIMAIA: a laser-driven ion accelerator for multidisciplinary applications. Quant Beam Sci. (2018) 8:2. doi: 10.3390/qubs2020008
8. Cirrone GAP, Romano F, Scuderi V, Amato A, Candiano G, Cuttone G, et al. Transport and dosimetric solutions for the elimed laser-driven beam line. Nuclear Instrum Methods Phys Res A. (2015) 796:99–103. doi: 10.1016/j.nima.2015.02.019
9. Milluzzo G, Scuderi V, Amico AG, Borghesi M, Cirrone GAP, Cuttone G, et al. Laser-accelerated ion beam diagnostics with TOF detectors for the ELIMED beam line. J Instrum. (2017) 12:C02025. doi: 10.1088/1748-0221/12/02/C02025
10. Scuderi V, Milluzzo G, Doria D, Alejo A, Amico A, Booth N, et al. Time of Flight based diagnostics for high energy laser driven ion beams. J Instrum. (2017) 12:C03086. doi: 10.1088/1748-0221/12/03/C03086
11. Schillaci F, Cirrone GAP, Cuttone G, Romano F, Scuderi V, Allegra L, et al. Status of the ELIMED Beamline at the ELIMAIA facility. J Instrum. (2016) 11:C12052. doi: 10.1088/1748-0221/11/12/c12052
12. Schillaci F, Cirrone GAP, Cuttonea G, Maggiorec M, Andoa L, Amatoa A, et al. Design of the ELIMAIA ion collection system. J Instrum. (2014) 10:T12001. doi: 10.1088/1748-0221/10/12/t12001
13. Schillaci F Maggiore M Andó L Cirrone GAP Cuttone G Romano F . Design of a large acceptance, high efficiency energy selection system for the ELIMAIA beam-line. J Instrum. (2016) 11:P08022. doi: 10.1088/1748-0221/11/08/P08022
14. Schillaci F, Nevrkla M, Maggiore M, Goncalves L, Cirrone PGA, Velyhan A, et al. Design of a high energy Thomson Parabola ion spectrometer for the ELIMAIA beamline. J Instrum. (2019) 14:T06004. doi: 10.1088/1748-0221/14/06/T06004
15. Agostinelli S, Allison J, Amako K, Apostolakis J, Araujo H, Arce P, et al. Geant4 - A simulation toolkit. Nuclear Instrum Methods Phys Res A. (2003) 506:250–303. doi: 10.1016/S0168-9002(03)01368-8
16. Alliso J, Amako K, Apostolakis J, Araujo H, Arce P, Asai M, et al. Geant4 developments and applications. IEEE Trans Nuclear Sci. (2006) 53:270–8. doi: 10.1109/TNS.2006.869826
17. Allison J, Amako K, Apostolakis J, Arce P, Asai M, Aso T, et al. Recent developments in Geant4. Nuclear Instrum Methods A. (2016) 835:186–225. doi: 10.1016/j.nima.2016.06.125
18. Milluzzo G, Pipek J, Amico AG, Cirrone GAP, Cuttone G, Korn G, et al. Transversal dose distribution optimization for laser-accelerated proton beam medical applications by means of Geant4. Phys Med. (2018) 54:166–72. doi: 10.1016/j.ejmp.2018.07.008
19. Milluzzo G, Pipek J, Amico AG, Cirrone GAP, Cuttone G, Korn G, et al. Geant4 simulation of the ELIMED transport and dosimetry beam line for highenergy laser-driven ion beam multidisciplinary applications. Nuclear Instrum Methods Phys Res A. (2018) 909:298–302. doi: 10.1016/j.nima.2018.02.066
20. Milluzzo G, Pipek J, Amico AG, Cirrone GAP, Cuttone G, Korn G, et al. Monte Carlo simulation of the ELIMED beamline using Geant4. J Instrum. (2017) 12:C03027. doi: 10.1088/1748-0221/12/03/C03027
21. Cambria R. Proton beam dosimetry: a comparison between the Faraday cup and an ionization chamber. Phys Med Biol. (1997) 42:1185–96. doi: 10.1088/0031-9155/42/6/014
22. Cuttone G, Raffaele L, Barone-Tonghi L, Rovelli A, Sabini MG, Egger E, et al. First Dosimetry Intercomparison Results for the CATANA project. Phys Med. (1999) 15:121–130.
23. Thomas DJ, Hodges GS, Seely DG, Moroz NA, Kvale TJ. Performance enhancement study of an electrostatic Faraday cup detector. Nuclear Instrum Methods Phys Res Sec A. (2005) 536:11–21. doi: 10.1016/j.nima.2004.07.211
24. Cirrone GAP, Petringa G, Cagni BM, Cuttonea G, Fustaino GF, Guarreraa M, et al. Use of radiochromic films for the absolute dose evaluation in high dose-rate proton beams. J Instrum. (2020) 15:C04029. doi: 10.1088/1748-0221/15/04/C04029
25. Jeong TW, Singh PK, Scullion C, Ahmed H, Hadjisolomou P, Jeon C, et al. CR-39 track detector for multi-MeV ion spectroscopy. Sci Rep. (2017) 7:2152. doi: 10.1038/s41598-017-02331-w
26. Cuttone G, Cirrone GAP, Di Franco G, La Monaca V, Lo Nigro S, Ott J, et al. CATANA protontherapy facility: the state of art of clinical and dosimetric experience. Eur Phys J Plus. (2011) 126:65. doi: 10.1140/epjp/i2011-11065-1
27. Xu XH, Liao Q, Wu JM, Geng YX, Li DY, Zhu JG, et al. Detection and analysis of laser driven proton beams by calibrated gafchromic HD-V2 and MD-V3 radiochromic films. Rev Sci Instrum. (2019) 90:033306. doi: 10.1063/1.5049499
28. Devic S. Radiochromic film dosimetry: past, present, and future. Phys Med. (2011) 27:122–34. doi: 10.1016/j.ejmp.2010.10.001
29. Karsch L, Beyreuther E, Burris-Mog T, Kraft S, Richter C, Zeil K, et al. Dose rate dependence for different dosimeters and detectors: TLD, OSL, EBT films and diamond detectors. Med Phys. (2012) 39:2447–55. doi: 10.1118/1.3700400
30. Karsch L, Margarone D, Candiano G, Kim IJ, Jeong TM, Psikal J, et al. Radiochromic film diagnostics for laser-driven ion beams. In: Research Using Extreme Light: Entering New Frontiers with Petawatt-Class Lasers II. Prague (2012).
31. SRIM. The Stopping and Range of Ions in Matter; SRIM2019. Available online at: http://www.srim.org
32. Bolton P, Parodi K, Schreiber J. Applications of Laser-Driven Particle Acceleration. Boca Raton; New York, NY: CRC Press (2018). doi: 10.1201/9780429445101
33. Pappalardo G, Esposito A, Cirrone GA, Cuttone G, Garraffo S, Pappalardo L, et al. Effects of the behaviour of the proton-induced isotopes production on the analysis of ancient alloys. Nuclear Instrum Methods Phys Res Sec B. (2008) 266:2286–91. doi: 10.1016/j.nimb.2008.03.074
34. Barberio CM, Veltri S, Sciscio M, Antici P. Laser-accelerated proton beams as diagnostics for cultural heritage. Sci Rep. (2017) 7:40415. doi: 10.1038/srep40415
35. Cucoanesa AS, Balabanskia DL, Canova F, Cuong P, Negoita F, Puicea F, et al. On the potential of laser driven isotope generation at ELI-NP for positron emission tomography. In: Medical Applications of Laser-Generated Beams of Particles IV: Review of Progress and Strategies for the Future. Prague (2017). doi: 10.1117/12.2273769
36. Amato E, Italiano A, Margarone D, Pagano B, Baldari S, Korn G. Future laser-accelerated proton beams at ELI-Beamlines as potential source of positron emitters for PET. J Instrum. (2016) 11:C04007. doi: 10.1088/1748-0221/11/04/C04007
37. Patel PK, Mackinnon AJ, Key MH, Cowan TE, Foord ME, Allen M, et al. Isochoric heating of solid-density matter with an ultrafast proton beam. Phys Rev Lett. (2003) 91:125004. doi: 10.1103/PhysRevLett.91.125004
38. Roth M, Cowan TE, Key MH, Hatchett SP, Brown C, Fountain W, et al. Fast ignition by intense laser-accelerated proton beams. Phys Rev Lett. (2001) 86: 436–9. doi: 10.1103/PhysRevLett.86.436
Keywords: protontherapy, dosimetry, Monte Carlo, laser-driven, ELIMED, laser-driven ions
Citation: Cirrone GAP, Petringa G, Catalano R, Schillaci F, Allegra L, Amato A, Avolio R, Costa M, Cuttone G, Fajstavr A, Gallo G, Giuffrida L, Guarrera M, Korn G, Larosa G, Leanza R, Lo Vecchio E, Messina G, Milluzzo G, Olsovcova V, Pulvirenti S, Pipek J, Romano F, Rizzo D, Russo AD, Salamone S, Scuderi V, Velyhan A, Vinciguerra S, Zakova M, Zappalà E and Margarone D (2020) ELIMED-ELIMAIA: The First Open User Irradiation Beamline for Laser-Plasma-Accelerated Ion Beams. Front. Phys. 8:564907. doi: 10.3389/fphy.2020.564907
Received: 22 May 2020; Accepted: 02 September 2020;
Published: 13 November 2020.
Edited by:
Vincenzo Patera, Sapienza University of Rome, ItalyReviewed by:
Kenneth Long, Imperial College London, United KingdomCopyright © 2020 Cirrone, Petringa, Catalano, Schillaci, Allegra, Amato, Avolio, Costa, Cuttone, Fajstavr, Gallo, Giuffrida, Guarrera, Korn, Larosa, Leanza, Lo Vecchio, Messina, Milluzzo, Olsovcova, Pulvirenti, Pipek, Romano, Rizzo, Russo, Salamone, Scuderi, Velyhan, Vinciguerra, Zakova, Zappalà and Margarone. This is an open-access article distributed under the terms of the Creative Commons Attribution License (CC BY). The use, distribution or reproduction in other forums is permitted, provided the original author(s) and the copyright owner(s) are credited and that the original publication in this journal is cited, in accordance with accepted academic practice. No use, distribution or reproduction is permitted which does not comply with these terms.
*Correspondence: Giuseppe A. P. Cirrone, cGFibG8uY2lycm9uZUBsbnMuaW5mbi5pdA==
Disclaimer: All claims expressed in this article are solely those of the authors and do not necessarily represent those of their affiliated organizations, or those of the publisher, the editors and the reviewers. Any product that may be evaluated in this article or claim that may be made by its manufacturer is not guaranteed or endorsed by the publisher.
Research integrity at Frontiers
Learn more about the work of our research integrity team to safeguard the quality of each article we publish.