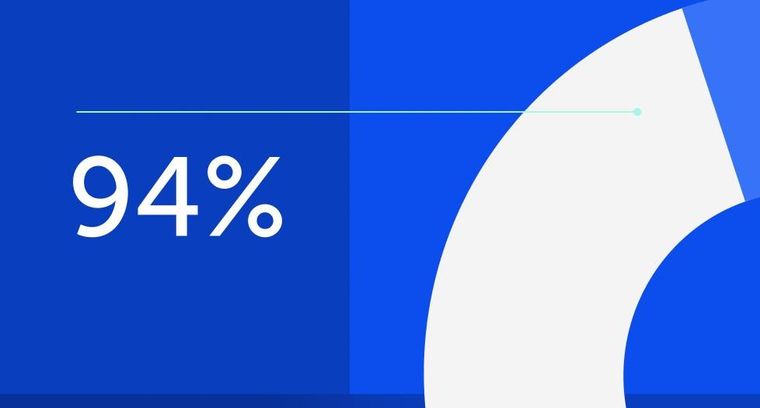
94% of researchers rate our articles as excellent or good
Learn more about the work of our research integrity team to safeguard the quality of each article we publish.
Find out more
MINI REVIEW article
Front. Phys., 06 August 2020
Sec. Optics and Photonics
Volume 8 - 2020 | https://doi.org/10.3389/fphy.2020.00251
This article is part of the Research TopicRecent Progress in Surface Electromagnetic ModesView all 17 articles
Surface plasmons are usually excited by diffraction-limited optical methods with the use of bulky optical components, which greatly limits the miniaturization and chip-scale high-density integration of plasmonic devices. By integrating a plasmonic nanostructure with a tunnel junction, plasmonic modes in the nanostructure can be directly excited by low-energy tunneling electrons with the advantages including an ultra-small footprint and an ultra-fast speed. In this mini-review, recent progress in the electric excitation of localized and propagating surface plasmons by inelastic electron tunneling is overviewed.
Surface plasmons are highly confined electromagnetic modes coherently coupled to collective oscillations of free carriers at metallic (or doped semiconductor) interfaces. They exist in the form of surface plasmon polaritons (SPPs) propagating at an interface between a conductor and a dielectric or as localized surface plasmons (LSPs) supported by confined conductive nanostructures [1, 2]. Their ability to localize electromagnetic fields at a subwavelength scale and produce greatly enhanced local fields for strong light–matter interaction offers the opportunity to combine the advantages of nanoelectronics (small size) and dielectric nanophotonics (high speed), opening an avenue for merging electronics and photonics at the nanoscale [3]. In the past 20 years, a great progress has been made in the area of plasmonics, which have stimulated a variety of applications, such as nano waveguides [4–6], plasmonic lasers [7–9], ultrafast electro-optical [10–12] and all-optical [13, 14] modulation, photodetection [15, 16], bio-chemical sensing [17, 18], and enhancement of non linear optics [19, 20].
Usually, surface plasmons are excited by diffraction-limited optical methods with the use of bulky optical components (e.g., prisms, grating, objectives, etc.) [1], which greatly limits the miniaturization and chip-scale high-density integration of plasmonic devices. At the same time, there are some alternatives. In his seminal work, Ritchie proposed that fast electrons can be used for the excitation of surface plasmons in metal [21]. Later, both the excitation of SPPs [22, 23] and LSPs [24] have been experimentally demonstrated with high-energy (~30 keV) electron beams with an advantage of highly precise and localized excitation (with a spatial resolution down to several nanometers). However, the requirements of a high electric voltage and a vacuum environment make it impossible for practical applications. Low-energy electrical excitation of SPPs has been demonstrated, e.g., by coupling plasmonic waveguides with electrically driven nano light sources [25, 26], but a highly compact and faster approach not related to the carrier lifetime would be highly desirable. In this mini-review, we focus on the recent breakthroughs in the low-energy direct excitation of surface plasmons based on an inelastic electron tunneling (IET) effect in tunnel junctions.
In 1976, Lambe and McCarthy [27] observed a broadband light emission from macroscopic planar metal-insulator-metal (MIM) tunnel junctions with an external quantum efficiency (EQE, i.e., electron-to-photon conversion efficiency) around 10−5. This phenomenon can be explained in terms of IET-based excitation of surface plasmon modes subsequently coupled to photons on rough planar MIM tunnel junctions. When an electric bias is applied across an MIM structure with a nanometer-scale insulator thickness, electrons can quantum-mechanically tunnel through the insulating barrier. During the tunneling process (Figure 1A), most electrons tunnel elastically without energy loss, appearing as high-energy (in respect to the Fermi level) electrons on the other side of the junctions, so called “hot electrons.” Some small fraction of electrons, however, tunnel inelastically, giving part of their energy to the excitation of plasmonic modes in the junction, which can then couple to extended propagating SPP modes or to free-space photons. The resulting emission spectral profile I(ω) ∝ Itc(V, ω)ρLDOSηrad is defined by the electromagnetic intensity spectrum of the tunneling current (which can be found from the calculation of the quantum transition matrix elements [30, 31] or from a Fourier transform of the tunneling current shot noise [32]), local density of optical states (LDOS) ρLDOS in the junction region and the radiative efficiency of the tunneling system in terms of generation of output photonic and/or plasmonic modes ηrad. In other words, the intrinsic electromagnetic spectrum from the tunneling current, Itc(V, ω), is highly dependent on the applied bias V with a high-frequency cutoff ωco (defined by the quantum relation for the maximal energy conversion ℏωco = eV) and a monotonic increase toward lower frequencies, and it is shaped into the final emission spectrum by the optical (frequently resonant) properties of the tunneling structure, defined by ρLDOS and ηrad. Later in the 1980's, IET-induced light emission was also reported from plasmonic tunnel junctions formed between a scanning tunneling microscope (STM) tip and a metallic substrate [33–35]. By analyzing the leakage radiation of a tunnel junction formed between an STM tip and a thin gold film in both image and Fourier planes, Wang et al. found that up to 99.5% of the detected photons come from leakage radiation of SPPs propagating on the gold film with the remaining photon emission attributed to the radiative decay of a localized plasmonic mode excited between the STM tip and the gold film [36], explicitly demonstrating the possibility of highly efficient coupling of inelastic tunneling to propagating plasmonic modes. Furthermore, despite its low EQE, this technique provides a high-spatial-resolution method for the study of LSPRs in metallic nanostructures [37–39]. At the same time, in combination with the atomic-scale spatial resolution of an STM, this approach has been developed into a useful optical spectroscopic method for single-molecule characterizations [40].
Figure 1. (A) Schematic diagram of generation of surface plasmons and photons through IET. (B) Electroluminescence (data points) and scattering spectra (solid lines) for antennas with various geometries and a micrometer-long non-resonant wire, together with the corresponding SEM images. (C) Atomic force microscopy image of a gold nanorod array with the dips marking the positions of Au few nanometers below the surface of an Al2O3 matrix. (D) Photograph of an Au nanorod array electrically driven at a bias of 2.5 V. (B) is reprinted with permission from Ref. [28]. Copyright © 2015, Springer Nature. (C,D) are reprinted with permission from Ref. [29]. Copyright © 2018, Springer Nature.
Together with the success of IET-induced plasmon excitation and light emission in the STM research community comes its main challenge for the application in practical devices related to its low efficiencies, including internal quantum efficiency (IQE, inelastic tunneling efficiency, which is defined by the ratio of the generated plasmonic quanta and the number of overall tunneling events) and EQE (for photon-related applications). Overcoming this has attracted continuous research interest in the past four decades because of the ultra-small footprint of tunnel junctions, which allows for high-density integration, and the ultra-fast speed of the IET process (at a scale of few femtoseconds [41]), which offers the potential for ultra-fast direct modulation of the excitation. These efforts are further motivated by a theoretical prediction that the IQE can be of the order of 10%, known from the early days of the research [42]. From the theoretical point of view, the IQE of a plasmonic tunnel junction is defined by the electronic densities of states in both electrodes (as well as any other electronic states inside the junction area) and the LDOS in the tunnel junction, while the EQE is defined by a product of the IQE and the radiation efficiency of the tunnel junction [43–45]. By engineering the LDOS and radiation efficiency, a significant increase in both IQE and EQE has been recently demonstrated [28, 46–48]. For example, in 2015, Kern et al. demonstrated the first electrically driven optical antenna by integrating a tunnel junction into it [28]. In this experiment, the tunnel junction was fabricated by placing a gold nanoparticle into a gap formed between two arms of a linear dipole antenna as shown in the left panel of Figure 1B. The emission spectrum from the electrically driven optical antenna is then defined by the applied bias and the nanoantenna plasmonic resonance, which can be tuned by changing the geometry of the nanoantenna (right panel of Figure 1B). Taking advantage of the high LDOS and radiation efficiency of the resonant antenna design, the EQE was increased to ~10−4, which is about two orders of magnitude higher than that for a non-resonant design. Later in the same year, Parzefall et al. achieved resonantly enhanced light emission by structuring an array of slot antennas on the bottom electrode of a vertical MIM tunnel junction formed by two gold electrodes and an insulating h-BN crystal [46]. Compared with an unstructured MIM tunnel junction, the EQE of the nanostructured junctions is increased by two orders of magnitude from ~4 × 10−7 to ~2.5 × 10−5 at a bias of 2.5 V due to the enhanced radiation efficiency ~4 × 10−3 provided by the slot antennas. The authors further demonstrated direct temporal modulation of light emission from the MIM tunnel junctions at frequencies up to 1 GHz. In 2018, implementing a tunnel junction produced by two chemically synthesized silver nanocubes assembled into an edge-to-edge configuration with the stabilizing polymer simultaneously working as the insulating barrier, Qian et al. obtained a record-high EQE of up to 2% at near-infrared frequencies [47]. Such excellent efficiency values are underlined by a very high LDOS in the tunneling junction (a factor of 3.1 × 105 higher than in vacuum) provided by an atomic-level quality of the gap between the silver single crystals and prominent 24.6% radiative efficiency of the implemented edge-to-edge nanoantenna design. In 2019, by cross-placing an Ag nanowire and an Au nanostripe, He et al. demonstrated the excitation of cavity plasmons with highly tuneable multiple emission peaks and narrow (tens of nanometers) line widths [49]. By using a dielectrophoresis trapping method, they further demonstrated efficient fabrication of nanoparticle-based electrically driven optical antennas with a measured EQE of ~2.5 × 10−4 [50]. Looking into the tunneling system from a conceptual point of view, Uskov et al. theoretically showed that the close-to-unity IQE can be achieved by introducing a quantum well structure in the tunneling gap with the energy level in the well aligned in a way that the inelastic tunneling happens in a resonant manner while the elastic counterpart does not [44]. However, as the authors noticed, this is done on the expense of the overall value of the tunneling probability, which dramatically decreases.
Although the IQE and EQE in plasmonic tunnel junctions have been significantly improved by engineering the LDOS and radiation efficiency, the overall generated plasmonic or photonic power is still quite low (pW level or smaller), which causes a difficulty in the signal detection and greatly limits their applications. This, however, is mainly due to the intrinsically low tunneling current in single nanoscale tunnel junctions. A promising way to solve this problem is increasing the number and density of the optical antenna-coupled tunnel junctions. For example, by constructing a macroscopic and high-density plasmonic tunnel junction array at the top of a plasmonic metamaterial produced by vertically oriented gold nanorods (Figure 1C, nanorod areal density is around 1 × 1010 cm−2), Wang et al. realized IET-driven light emission visible by the naked eye (Figure 1D) [29, 51]. The spectrum of the emission in this case is shaped by the metamaterial plasmonic modes, which can be tuned throughout the visible and near-infrared ranges by tuning the metamaterial modes via the nanostructure geometric parameters [51]. The measured emission power was around 100 nW, which makes the signal detection trivial for applications such as optical sensing. Based on this, Wang et al. further demonstrated an ultra-compact electrically driven optical sensor by exploiting hot electrons generated via elastic tunneling (usually ignored, as it decays by the generation of heat) for the activation of chemical reactions in the junctions and IET-generated photons for the monitoring of this process [29].
Apart from coupling to free-space light emission and 2D plasmonic modes, IET can also be coupled to waveguided plasmonic or photonic modes, which is highly desired for on-chip applications, as they have a crucial advantage as information carriers in comparison with traditional electronic signals in terms of a higher bandwidth and lower loss. In 2011, Bharadwaj et al. reported an electrical excitation of propagating SPPs in a Au nanowire (Figure 2A) [52]. A plasmonic mode excited with an STM tip at the left end of the nanowire by IET, was subsequently coupled to SPPs propagating along the nanowire and then converted to free-space photons at the right end. However, the excitation of propagating SPPs with the use of STM is difficult for practical applications where on-chip integration is highly desired. In this respect, a promising design was realized by integrating an electromigrated tunnel junction on the top of a dielectric-loaded surface plasmon waveguide (DLSPPW) (Figure 2B) [53] or by crossing a gold plasmonic waveguide and an thin aluminum strip covered with a nanoscale oxide layer [56, 57]. For the latter case, an SPP excitation efficiency exceeding 1% was reported [56], which was further explained by surface roughness–induced momentum matching between the MIM modes in the junction and the output SPP modes present in the system [57]. In 2019, Zhang et al. further demonstrated enhanced excitation of SPPs along an aluminum–air interface by fabricating an array of linear gold antennas on the top of an oxidized aluminum surface [58]. The emitted SPP power was increased to ~10 pW, and the emission spectrum/polarization was controlled by the design of the antenna arrays. According to a recent calculation by Parzefall et al. [59], the IET-induced excitation efficiency of SPPs in extended conventional plasmonic waveguides is limited by a low coupling efficiency between the extremely confined MIM modes excited in the tunnel junction and the propagating waveguided SPPs due to the dramatic mismatch between their propagation constants. An additional problem might be caused by the low modal overlap. This shows that more attention is required in the future to improve the coupling efficiency, e.g., via structural design of the coupling area.
Figure 2. (A) A map of optical emission intensity from an Au nanowire excited by an STM tip. (B) False color SEM image of a tunnel junction on the top of a DLSPPW waveguide, together with an optical intensity map, showing generation of the propagating plasmonic mode at the junction region and its outcoupling to the free-space radiation at the other end of the waveguide. (C) Tunneling-driven highly directional emission from a V-shaped nanoantenna. (D) The dependence of the directivity of the SPP excitation on the structural characteristics of self-assembled S(CH2)nBPh polymer molecules filling the tunneling gap defined by the chain parameter n. The inset shows an experimental measure defocused patterns corresponding to a tunnel junction with n = 2. (A) is reprinted with permission from Ref. [52]. © 2011 American Physical Society. (B) is reprinted with permission from Ref. [53]. Copyright © 2016 Optical Society of America. (C) is reprinted with permission from Ref. [54]. Copyright © 2017 American Chemical Society. (D) is reprinted with permission from Ref. [55]. Copyright © 2019 American Chemical Society.
It is worth mentioning that in addition to plasmon excitation based on metallic tunnel junctions plasmon and light emission can also be generated with metal-insulator-semiconductor (MIS) tunnel junctions [60]. The advantage of the MIS tunnel junctions is that they can be directly integrated into, e.g., a silicon photonic waveguides for on-chip applications [61, 62]. Particularly, with the coupling efficiency of the hybrid junction optical mode to the silicon waveguide of ~75%, Doderer et al. experimentally generated a waveguided optical power of 6.8 pW [61].
The ability to control the flow of optical energy is of great importance in nanophotonic applications. The directional control of SPPs and light emission excited by IET has been demonstrated in a variety of systems [54, 55, 63–65]. For example, Dong et al. demonstrated a directional control of SPP-assisted light emission from a gold stripe cavity with a directivity of extinction ratio around 2.6 : 1, which was realized by varying the distance between an STM probe and the edge of the cavity to attain a constructive or destructive interference with the generated and reflected SPP waves [63]. Taking advantage from an excellent directivity provided by optical antennas, Gurunarayanan et al. achieved a directivity of light emission of ~5 dB by aligning two nanorod antennas edge-to-edge at an angle of 90° (Figure 2C) [54]. Such a strong directivity is provided by an interplay between the dipolar radiation pattern of the tunnel junction emission and the quadrupole-like resonance of the rod antennas. Recently, Kullock et al. obtained a directivity of light emission as high as 9.1 dB in an optical Yagi-Uda antenna with a tunneling feed [64]. The directivity control can also be achieved by placing molecules in the junction region, particularly utilizing their chemical composition and/or orientation [55, 65]. For example, implementing tunneling through a self-assembled monolayer of polymer molecules (Figure 2D, inset), Du et al. experimentally achieved directional launching of SPPs by adjusting the tilt angle of a self-assembled monolayer of S(CH2)nBPh (BPh = biphenyl) molecules in respect to the electrode surface, which was realized by controlling the length of the alkyl chain n [55]. The highest directivity (defined as , where IL and IR are the maximum intensities of left and right lobes of the emission pattern, respectively) of 0.4 was obtained for n = 2 (Figure 2D, main graph), corresponding to a left/right intensity ratio of ~2.3.
In this review, we have overviewed the recent developments in the IET-assisted excitation of surface plasmons, including both LSPRs and SPPs, which open an opportunity for the miniaturization and chip-scale integration of plasmonic devices. However, for practical applications, there are still many things to be done and questions to be answered. For example, how to improve the overall output power from single tunnel junctions? How to optimize the coupling efficiency between an MIM mode excited by IET and SPPs in an extended waveguide for on-chip integration? How to achieve narrow-band excitation of surface plasmons? Finally, the question of long-term stability of tunnel junctions is a key concern for applications. Despite these challenges, as an ultra-fast and compact approach that can bridge electronics and plasmonics directly at the nanoscale, IET-based plasmonic excitation will continue to attract research interest and find applications in areas, such as optical interconnections and sensing.
PW and LL organized and wrote the article. All the authors participated in discussion and revision.
This work was supported by the Fundamental Research Funds for the Central Universities.
The authors declare that the research was conducted in the absence of any commercial or financial relationships that could be construed as a potential conflict of interest.
1. Zayats AV, Smolyaninov II, Maradudin AA. Nano-optics of surface plasmon polaritons. Phys Rep. (2005) 408:131–314. doi: 10.1016/j.physrep.2004.11.001
2. Schuller JA, Barnard ES, Cai W, Jun YC, White JS, Brongersma ML. Plasmonics for extreme light concentration and manipulation. Nat Mater. (2010) 9:193–204. doi: 10.1038/nmat2630
3. Brongersma ML, Shalaev VM. The case for plasmonics. Science. (2010) 328:440–1. doi: 10.1126/science.1186905
4. Lal S, Link S, Halas NJ. Nano-optics from sensing to waveguiding. Nat Photonics. (2007) 1:641–8. doi: 10.1038/nphoton.2007.223
5. Guo X, Ying Y, Tong L. Photonic nanowires: from subwavelength waveguides to optical sensors. Acc Chem Res. (2014) 47:656–66. doi: 10.1021/ar400232h
6. Krasavin AV, Zayats AV. Guiding light at the nanoscale: numerical optimization of ultrasubwavelength metallic wire plasmonic waveguides. Opt Lett. (2011) 36:3127–9. doi: 10.1364/OL.36.003127
7. Hill MT, Gather MC. Advances in small lasers. Nat Photonics. (2014) 8:908–18. doi: 10.1038/nphoton.2014.239
8. Ma R, Oulton RF. Applications of nanolasers. Nat Nanotechnol. (2019) 14:12–22. doi: 10.1038/s41565-018-0320-y
9. Wu H, Gao Y, Xu P, Guo X, Wang P, Dai D, et al. Plasmonic nanolasers: pursuing extreme lasing conditions on nanoscale. Adv Opt Mater. (2019) 7:1900334. doi: 10.1002/adom.201900334
10. Krasavin AV, Zayats AV. Photonic signal processing on electronic scales: electro-optical field-effect nanoplasmonic modulator. Phys Rev Lett. (2012) 109:053901. doi: 10.1103/PhysRevLett.109.053901
11. Melikyan A, Alloatti L, Muslija A, Hillerkuss D, Schindler PC, Li J, et al. High-speed plasmonic phase modulators. Nat Photonics. (2014) 8:229–33. doi: 10.1038/nphoton.2014.9
12. Ayata M, Fedoryshyn Y, Heni W, Baeuerle B, Josten A, Zahner M, et al. High-speed plasmonic modulator in a single metal layer. Science. (2017) 358:630–2. doi: 10.1126/science.aan5953
13. MacDonald KF, Samson ZL, Stockman MI, Zheludev NI. Ultrafast active plasmonics. Nat Photonics. (2009) 3:55–8. doi: 10.1038/nphoton.2008.249
14. Wurtz GA, Pollard R, Hendren W, Wiederrecht GP, Gosztola DJ, Podolskiy VA, et al. Designed ultrafast optical nonlinearity in a plasmonic nanorod metamaterial enhanced by nonlocality. Nat Nanotechnol. (2011) 6:107–11. doi: 10.1038/nnano.2010.278
15. Falk AL, Koppens FHL, Yu CL, Kang K, Snapp NdL, Akimov AV, et al. Near-field electrical detection of optical plasmons and single-plasmon sources. Nat Phys. (2009) 5:475–9. doi: 10.1038/nphys1284
16. Knight MW, Sobhani H, Nordlander P, Halas NJ. Photodetection with active optical antennas. Science. (2011) 332:702–4. doi: 10.1126/science.1203056
17. Nie S, Emory SR. Probing single molecules and single nanoparticles by surface-enhanced Raman scattering. Science. (1997) 275:1102–6. doi: 10.1126/science.275.5303.1102
18. Liu N, Tang ML, Hentschel M, Giessen H, Alivisatos AP. Nanoantenna-enhanced gas sensing in a single tailored nanofocus. Nat Mater. (2011) 10:631–6. doi: 10.1038/nmat3029
19. Kauranen M, Zayats AV. Nonlinear plasmonics. Nat Photonics. (2012) 6:737–48. doi: 10.1038/nphoton.2012.244
20. Krasavin AV, Ginzburg P, Zayats AV. Free-electron optical nonlinearities in plasmonic nanostructures: a review of the hydrodynamic description. Laser Photon Rev. (2018) 12:1700082. doi: 10.1002/lpor.201700082
21. Ritchie RH. Plasma losses by fast electrons in thin films. Phys Rev. (1957) 106:874–81. doi: 10.1103/PhysRev.106.874
22. Bashevoy MV, Jonsson F, Krasavin AV, Zheludev NI, Chen Y, Stockman MI. Generation of traveling surface plasmon waves by free-electron impact. Nano Lett. (2006) 6:1113–5. doi: 10.1021/nl060941v
23. Vesseur EJR, de Waele R, Kuttge M, Polman A. Direct observation of plasmonic modes in Au nanowires using high-resolution cathodoluminescence spectroscopy. Nano Lett. (2007) 7:2843–6. doi: 10.1021/nl071480w
24. Nelayah J, Kociak M, Stéphan O, García de Abajo FJ, Tencé M, Henrard L, et al. Mapping surface plasmons on a single metallic nanoparticle. Nat Phys. (2007) 3:348–53. doi: 10.1038/nphys575
25. Huang KCY, Seo M-K, Sarmiento T, Huo Y, Harris JS, Brongersma ML. Electrically driven subwavelength optical nanocircuits. Nat Photonics. (2014) 8:244–9. doi: 10.1038/nphoton.2014.2
26. Koller D, Hohenau A, Ditlbacher H, Galler N, Reil F, Aussenegg F, et al. Organic plasmon-emitting diode. Nat Photonics. (2008) 2:684–7. doi: 10.1038/nphoton.2008.200
27. Lambe J, McCarthy SL. Light emission from inelastic electron tunneling. Phys Rev Lett. (1976) 37:923–5. doi: 10.1103/PhysRevLett.37.923
28. Kern J, Kullock R, Prangsma J, Emmerling M, Kamp M, Hecht B. Electrically driven optical antennas. Nat Photonics. (2015) 9:582–6. doi: 10.1038/nphoton.2015.141
29. Wang P, Krasavin AV, Nasir ME, Dickson W, Zayats AV. Reactive tunnel junctions in electrically driven plasmonic nanorod metamaterials. Nat Nanotechnol. (2018) 13:159–64. doi: 10.1038/s41565-017-0017-7
30. Persson BNJ, Baratoff A. Theory of photon emission in electron tunneling to metallic particles. Phys Rev Lett. (1992) 68:3224–7. doi: 10.1103/PhysRevLett.68.3224
31. Johansson P. Light emission from a scanning tunneling microscope: fully retarded calculation. Phys Rev B. (1998) 58:10823–34. doi: 10.1103/PhysRevB.58.10823
32. Rendell RW, Scalapino DJ. Surface plasmons confined by microstructures on tunnel junctions. Phys Rev B. (1981) 24:3276–94. doi: 10.1103/PhysRevB.24.3276
33. Coombs J, Gimzewski J, Reihl B, Sass J, Schlittler R. Photon emission experiments with the scanning tunnelling microscope. J Microsc. (1988) 152:325–36. doi: 10.1111/j.1365-2818.1988.tb01393.x
34. Gimzewski JK, Reihl B, Coombs JH, Schlittler RR. Photon-emission with the scanning tunnelling microscope. Eur Phys J B. (1988) 72:497–501. doi: 10.1007/bf01314531
35. Johansson P, Monreal R, Apell P. Theory for light emission from a scanning tunneling microscope. Phys Rev B Condens Matter. (1990) 42:9210–3. doi: 10.1103/physrevb.42.9210
36. Wang T, Boer-Duchemin E, Zhang Y, Comtet G, Dujardin G. Excitation of propagating surface plasmons with a scanning tunnelling microscope. Nanotechnology. (2011) 22:175201. doi: 10.1088/0957-4484/22/17/175201
37. Silly F, Gusev AO, Taleb A, Charra F, Pileni MP. Coupled plasmon modes in an ordered hexagonal monolayer of metal nanoparticles: a direct observation. Phys Rev Lett. (2000) 84:5840–3. doi: 10.1103/PhysRevLett.84.5840
38. Le Moal E, Marguet S, Rogez B, Mukherjee S, Dos Santos P, Boer-Duchemin E, et al. An electrically excited nanoscale light source with active angular control of the emitted light. Nano Lett. (2013) 13:4198–205. doi: 10.1021/nl401874m
39. Le Moal E, Marguet S, Canneson D, Rogez B, Boer-Duchemin E, Dujardin G, et al. Engineering the emission of light from a scanning tunneling microscope using the plasmonic modes of a nanoparticle. Phys Rev B. (2016) 93:035418. doi: 10.1103/PhysRevB.93.035418
40. Kuhnke K, Grosse C, Merino P, Kern K. Atomic-scale imaging and spectroscopy of electroluminescence at molecular interfaces. Chem Rev. (2017) 117:5174–222. doi: 10.1021/acs.chemrev.6b00645
41. Fevrier P, Gabelli J. Tunneling time probed by quantum shot noise. Nat Commun. (2018) 9:4940. doi: 10.1038/s41467-018-07369-6
42. Davis LC. Theory of surface-plasmon excitation in metal-insulator-metal tunnel junctions. Phys Rev B. (1977) 16:2482–90. doi: 10.1103/PhysRevB.16.2482
43. Chen C, Bobisch CA, Ho W. Visualization of fermi's golden rule through imaging of light emission from atomic silver chains. Science. (2009) 325:981–5. doi: 10.1126/science.1174592
44. Uskov AV, Khurgin JB, Protsenko IE, Smetanin IV, Bouhelier A. Excitation of plasmonic nanoantennas by nonresonant and resonant electron tunnelling. Nanoscale. (2016) 8:14573–9. doi: 10.1039/c6nr01931e
45. Bigourdan F, Hugonin JP, Marquier F, Sauvan C, Greffet JJ. Nanoantenna for electrical generation of surface plasmon polaritons. Phys Rev Lett. (2016) 116:106803. doi: 10.1103/PhysRevLett.116.106803
46. Parzefall M, Bharadwaj P, Jain A, Taniguchi T, Watanabe K, Novotny L. Antenna-coupled photon emission from hexagonal boron nitride tunnel junctions. Nat Nanotechnol. (2015) 10:1058–63. doi: 10.1038/nnano.2015.203
47. Qian H, Hsu S, Gurunatha K, Riley CT, Zhao J, Lu D, et al. Efficient light generation from enhanced inelastic electron tunnelling. Nat Photonics. (2018) 12:485–8. doi: 10.1038/s41566-018-0216-2
48. Parzefall M, Szabo A, Taniguchi T, Watanabe K, Luisier M, Novotny L. Light from van der waals quantum tunneling devices. Nat Commun. (2019) 10:292. doi: 10.1038/s41467-018-08266-8
49. He X, Tang J, Hu H, Shi J, Guan Z, Zhang S, et al. Electrically driven highly tunable cavity plasmons. ACS Photonics. (2019) 6:823–9. doi: 10.1021/acsphotonics.8b01620
50. He X, Tang J, Hu H, Shi J, Guan Z, Zhang S, et al. Electrically driven optical antennas based on template dielectrophoretic trapping. ACS Nano. (2019) 13:14041–7. doi: 10.1021/acsnano.9b06376
51. Krasavin AV, Wang P, Nasir ME, Jiang Y, Zayats AV. Tunneling-induced broadband and tunable optical emission from plasmonic nanorod metamaterials. Nanophotonics. (2020) 9:427–34. doi: 10.1515/nanoph-2019-0411
52. Bharadwaj P, Bouhelier A, Novotny L. Electrical excitation of surface plasmons. Phys Rev Lett. (2011) 106:226802. doi: 10.1103/PhysRevLett.106.226802
53. Cazier N, Buret M, Uskov AV, Markey L, Arocas J, Des Francs GC, et al. Electrical excitation of waveguided surface plasmons by a light-emitting tunneling optical gap antenna. Opt Express. (2016) 24:3873–84. doi: 10.1364/oe.24.003873
54. Gurunarayanan SP, Verellen N, Zharinov VS, Shirley FJ, Moshchalkov VV, Heyns M, et al. Electrically driven unidirectional optical nanoantennas. Nano Lett. (2017) 17:7433–9. doi: 10.1021/acs.nanolett.7b03312
55. Du W, Han Y, Hu H, Chu H, Annadata HV, Wang T, et al. Directional excitation of surface plasmon polaritons via molecular through-bond tunneling across double-barrier tunnel junctions. Nano Lett. (2019) 19:4634–40. doi: 10.1021/acs.nanolett.9b01665
56. Du W, Wang T, Chu H, Nijhuis CA. Highly efficient on-chip direct electronic-plasmonic transducers. Nat Photonics. (2017) 11:623–7. doi: 10.1038/s41566-017-0003-5
57. Makarenko K, Hoang T, Duffin T, Radulescu A, Kalathingal V, Lezec H, et al. Efficient surface plasmon polariton excitation and control over outcoupling mechanisms in metal-insulator-metal tunneling junctions. Adv Sci. (2020) 7:1900291. doi: 10.1002/advs.201900291
58. Zhang C, Hugonin JP, Coutrot AL, Sauvan C, Marquier F, Greffet JJ. Antenna surface plasmon emission by inelastic tunneling. Nat Commun. (2019) 10:4949. doi: 10.1038/s41467-019-12866-3
59. Parzefall M, Novotny L. Light at the end of the tunnel. ACS Photonics. (2018) 5:4195–202. doi: 10.1021/acsphotonics.8b00726
60. Goktas H, Gokhan FS, Sorger VJ. Electrical-driven plasmon source of silicon based on quantum tunneling. ACS Photonics. (2018) 5:4928–36. doi: 10.1021/acsphotonics.8b01106
61. Doderer M, Parzefall M, Joerg A, Chelladurai D, Dordevic N, Fedoryshyn Y, et al. Light emission from a waveguide integrated MOS tunnel junction. In 2019 Conference on Lasers and Electro-Optics. Conference on Lasers and Electro-Optics (2019).
62. Huang B, Gao S, Liu Y, Wang J, Liu Z, Guo Y, et al. Nano-antenna enhanced waveguide integrated light source based on an MIS tunnel junction. Opt Lett. (2019) 44:2330–3. doi: 10.1364/ol.44.002330
63. Dong Z, Chu H, Zhu D, Du W, Akimov YA, Goh WP, et al. Electrically-excited surface plasmon polaritons with directionality control. ACS Photonics. (2015) 2:385–91. doi: 10.1021/ph5004303
64. Kullock R, Ochs M, Grimm P, Emmerling M, Hecht B. Electrically-driven Yagi-Uda antennas for light. Nat Commun. (2020) 11:115. doi: 10.1038/s41467-019-14011-6
Keywords: surface plasmons, tunnel junctions, inelastic tunneling, optical antennas, light emission, waveguiding
Citation: Liu L, Xu Y, Zhu J, Wang P, Tong L and Krasavin AV (2020) Excitation of Surface Plasmons by Inelastic Electron Tunneling. Front. Phys. 8:251. doi: 10.3389/fphy.2020.00251
Received: 01 May 2020; Accepted: 08 June 2020;
Published: 06 August 2020.
Edited by:
Lin Chen, University of Shanghai for Science and Technology, ChinaReviewed by:
Shuiyan Cao, Nanjing University of Aeronautics and Astronautics, ChinaCopyright © 2020 Liu, Xu, Zhu, Wang, Tong and Krasavin. This is an open-access article distributed under the terms of the Creative Commons Attribution License (CC BY). The use, distribution or reproduction in other forums is permitted, provided the original author(s) and the copyright owner(s) are credited and that the original publication in this journal is cited, in accordance with accepted academic practice. No use, distribution or reproduction is permitted which does not comply with these terms.
*Correspondence: Pan Wang, bmFub3BhbkB6anUuZWR1LmNu; Alexey V. Krasavin, YWxleGV5LmtyYXNhdmluQGtjbC5hYy51aw==
Disclaimer: All claims expressed in this article are solely those of the authors and do not necessarily represent those of their affiliated organizations, or those of the publisher, the editors and the reviewers. Any product that may be evaluated in this article or claim that may be made by its manufacturer is not guaranteed or endorsed by the publisher.
Research integrity at Frontiers
Learn more about the work of our research integrity team to safeguard the quality of each article we publish.