- 1Biophysics and Medical Technology, Department of Physics, Norwegian University of Science and Technology, Trondheim, Norway
- 2Department of Orthopaedics, All India Institute of Medical Sciences, Patna, India
Second harmonic microscopy (SHM) has emerged as a powerful non-destructive optical imaging modality that has high potential to perform the advanced structural characterization of intact articular cartilage. This article provides the current status, opportunities, and challenges of SHM for the use in cartilage evaluation. A novel perspective on SHM is being addressed that includes the reason, need and the importance of SHM in the assessment of articular cartilage. By taking the advantage of collagen specificity and high-resolution imaging, SHM can detect the structural and morphological changes in early stage cartilage damage that would otherwise be overlooked by standard clinical imaging methods such as radiography and arthroscopy. The current status of SHM for articular cartilage analysis and the major progress reported in recent literature are briefly described herein. We summarize the status of scientific efforts and the challenges that need to overcome before moving toward clinical applications of SHM in the context of cartilage evaluation.
Introduction
Articular cartilage has a multilayered architecture, with three distinct (superficial, middle, and deep) zones of cartilage that can be viewed using high-resolution imaging tools [1, 2]. Collagen type-II is the most abundant structural molecule present in the extracellular matrix (ECM) of articular cartilage, with proteoglycan being the second most abundant molecule. In addition, elastin, hyaluronan, and other types of collagen (such as type-I, IV, V, VI, IX, and XI) are also found in relatively small amounts. The arrangement and interaction between proteoglycan (negatively charged), water and collagen-II makes the tissue capable of withstanding compressive and tensile physiological loading [1].
Accurate assessment of articular cartilage is essential in diagnosis, treatment-planning, and monitoring procedures in various orthopedic diseases. Articular cartilage is a connective tissue that covers the end of a bone in the synovial-joint and facilitates smooth movement by providing a low-friction surface and distributing any applied stress during motion. Articular cartilage lacks blood-vessels and hence associated with a lack of a regular nutrient supply. Therefore, unlike other tissues in the body, its capability to heal itself (regeneration) is very low.
The degradation of articular cartilage is directly associated with the progression of osteoarthritis (OA), which is one of the major causes of disability among elderly people and a rapidly increasing individual and socio-economic burden worldwide [3]. Changes in collagen architecture and organization are extensively associated with the stage of osteoarthritic degradation and age associated degeneration in articular cartilage.
In cartilage tissue engineering, the expression of collagen type-II is one of the most important markers for the quality assessment of articular cartilage constructs. In the treatment of osteochondral defects, regenerated articular cartilage needs to be monitored postoperatively because, the repaired cartilage is observed as fibrocartilage (collagen type-I) instead of hyaline cartilage (collagen type-II), which deteriorates over time [4, 5]. Thus, the early evaluation of collagen microstructural alteration, changes in collagen architecture and detection of collagen types within intact articular cartilage are essential current needs of orthopedics that can support accurate assessment of cartilage damage and can facilitate the better treatment procedures.
Cartilage Assessment—The Need for Better Tool
Non-invasive imaging methods such as radiography and magnetic resonance imaging (MRI) are limited in their use for articular cartilage evaluation since they are not sensitive to the early abnormalities [6, 7]. They are unable to detect early changes on the articular surface mainly because they are limited in resolution (~0.1–0.4 mm) and lack the molecular level specificity. Ultra-structural alterations in collagen are one of the prominent hallmarks to identify early stage cartilage abnormalities. To assess the quality of articular cartilage, non-invasive imaging methods have been tested [6–8]. However, direct assessment methods such as arthroscopy and histology are required for reliable and more precise evaluation of articular cartilage.
Arthroscopic assessment of a synovial-joint provides the magnified and direct visualization of the cartilage surface and currently represents a clinical standard to determine the extent of cartilage damage in several cartilage-disorders including OA, treatment of focal-lesion and, monitoring of “repair and degradation processes” over time. However, due to lack of early feature visibility (e.g., structural alterations in collagen fiber and changes in its integrity, fibrillation, chondrocyte cloning and death, micro-cracks and wrinkle-like pattern on articular surface etc.), the problems such as identification of accurate size of cartilage-lesions and estimation for severity of lesions remain persistent even when cartilage evaluation is performed using an arthroscope [9–13]. In addition, inter-observer variations raise a question mark regarding its reliability, which indicates the need for specialized clinical professionals. In several cases, histopathology is required in addition to arthroscopy for the further evaluation of cartilage. For histopathology, an invasive biopsy of cartilage is performed. Notably, in the long-run an invasive biopsy of articular cartilage can create clinical problems for the synovial-joint. Additionally, the results of a biopsy performed by a pathologist may take hours to days, thus, representing an intensive and time-consuming process.
Therefore, the inherent heterogeneity in cartilage-lesions, lack of early feature visibility, inter-observer variations, unreliability, and the further requirement of invasive biopsy are all major reasons that clearly indicate the need for a better tool to assist orthopedic surgeons. In addition, among the various histologic components, collagen fibers and bundles are the most influential parameters in acute and stress-related injuries of cartilage [14]. A technique that can provide reliable in-situ information about collagen alteration in addition to the surface morphology at the microscopic level without invasive biopsy would be of utmost importance for articular cartilage assessment in both research clinical settings (Figure 1).
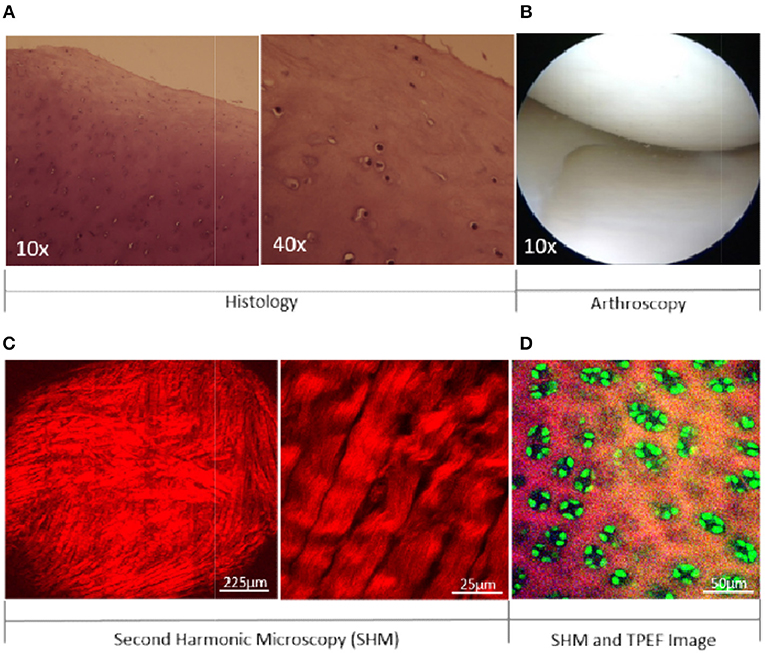
Figure 1. A comparison of visible features of articular cartilage between histological, arthroscopic, and SHM image. Cartilage imaging by H&E staining (A) and arthroscopy (B). Collagen imaging in cartilage at different magnifications (C). Collagen and Chondrocytes in cartilage (D). SHM: Second Harmonic Microscopy; TPEF: Two-photon Excited Fluorescence.
Second Harmonic Microscopy for Cartilage Assessment
Optical imaging has played a significant role in achieving the fundamental goal of biomedical imaging by retrieving structural and morphological information from tissues and cells with the highest possible diffraction-limited contrast and resolution. Second harmonic microscopy (SHM) is unique in its ability to generate the second harmonic signal from non-centrosymmetric structures (e.g., collagen, myosin, microtubules) when excited by ultra short (femtosecond) laser light. SHM provides highly specific molecular-level imaging by utilizing the endogenous signal to image collagen in cartilage at (sub-)micron level resolution. In addition, polarization-resolved second harmonic microscopy (p-SHM) is one step ahead of commercial state-of-the-art techniques that can differentiate the types of fibrillar collagen present in cartilage. The ability to detect different types of fibrillar collagen can be utilized in differentiating repaired (fibrous) cartilage from the natural (hyaline) articular cartilage in a synovial-joint. Thus, the surface morphology of cartilage, structural alterations in collagen and, the detection of collagen types can be achieved by high-resolution (submicron level) images of intact cartilage, without any need for the sectioning and staining of target tissue. Moreover, chondrocytes can also be imaged by utilizing the two-photon excited autofluorescence signal (Figure 1D, green color), which can be optically separated from the SHM signal. In clinical setting, further enhancement of the fluorescence contrast for cellular imaging can be accomplished by using sodium fluorescein that is an FDA approved clinical dye and can be conveniently used in the synovial-joint during arthroscopy [15].
Seven reasons that indicate why SHM can be useful in the assessment of articular cartilage.
• The first reason is that SHM imaging provides an excellent specificity to fibrillar collagen. The early features of cartilage degeneration are associated with changes in collagen fibers. Due to a lack of molecular specificity, collagen-associated early signs of cartilage degeneration are difficult to visualize—even in arthroscopy. Due to the inherent molecular characteristics, only collagen fibers can produce an SHM signal in cartilage and hence can be easily differentiated from other molecules present in the cartilage matrix, which makes it highly sensitive in detecting structural changes in collagen. Moreover, polarization-resolved SHM can be applied to differentiate collagen type-I and type-II, indicating that it can be utilize to differentiate hyaline cartilage from fibrous cartilage and hence demonstrate its capability for assessment of regenerated cartilage after repair.
• The second reason is high-resolution (submicron level) imaging by SHM. Due to a lack of high-resolution imaging, early stage pathological features of cartilage damage (e.g., fibrillation, cleft, fissure, chondrocyte-clustering etc.) are usually not visible in non-invasive clinical imaging methods (e.g., X-ray, CT, Ultrasound, MRI). Moreover, the novel structural features (Figure 2) of cartilage early degeneration, which include (i) architectural changes in the superficial-layer, (ii) microsplits (micron-level cracks) on articular surface, and (iii) the appearance of a wrinkle like pattern due to the loosening of cartilage articular surface cannot be detected using standard clinical imaging methods. Notably, such features can be observed by SHM due to its capability of high-resolution imaging.
• The third reason is real-time optical imaging, which is an essential need for emerging techniques that can potentially be translated to medical applications. Recently, SHM has been demonstrated for real time in-vivo endomicroscopy images with resolution 2.6 μm [16]. The acquired SHM micrographs are capable of detecting microsplits and ultra-structure fibril arrangements of collagen fiber in articular cartilage. Such technical advancements in SHM imaging at the endomicroscopic level are highly valuable for both translational and basic research [16, 17].
• The fourth reason relates to the capability of label-free imaging, which is highly welcomed in clinical use. SHM can be used in collagen imaging to analyze hyaline cartilage, fibrous cartilage, ligament, and tendon. Moreover, by combining two-photon excited fluorescence (TPEF) microscopy with SHM, the simultaneous label-free assessment of collagen alterations and changes in cellular morphology can highly complement the early assessment of cartilage damage [15].
• The fifth reason is the ability to perform optical sectioning and create a 3D image within the limits of penetration-depth (typically 100–200 μm) of laser light [18–20]. Tissue thickness in standard histological sections is normally 3–5 μm, which is very thin. Therefore, in histologically processed tissue, thickness-based morphological information is lost. For example, a normal articular surface is very smooth and firmly attached with underlying subchondral bone. The appearance of a “wrinkles- like” pattern indicates the possibility of cartilage loosening from the underlying bone. This pattern could only be seen via SHM in 3D-images with a thickness in the range of a few tens of microns. Another example is the visualization of changes in 3D cellular morphology, which is possible only if the thickness of tissue sections lie in the range of 10–20 μm. These features, exemplified by wrinkles and 3D cellular morphology, are lost in histological sections but could be observed in 3D-images acquired by SHM microscopy.
• The sixth reason is that the level of information acquired through SHM image bridges the gap between conventional light microscopy and electron microscopy of articular cartilage. Moreover, by exploiting polarization sensitivity, p-SHM is capable of differentiating collagen type-I and type-II, and can detect alterations in the helical pitch angle of collagen fiber in intact tissue that is beyond the diffraction limit.
• The seventh reason is that the use of SHM may reduce the need for invasive biopsy of articular cartilage. In the diagnostic and surgical procedure, articular cartilage biopsy is not frequently indicated. However, the procedure is feasible, and occasionally performed due to diagnostic aid [21] or post-operative assessment [4]. The needle-based invasive biopsy of articular cartilage can be a potential danger for a synovial-joint over the long term. By performing an optical biopsy in intact tissue, SHM could minimize the need for needle-based biopsy followed by histology. This might hold a crucial advantage in clinical and intra-operative procedures.
A comparable technique that can create a cross sectional optical image of articular cartilage in real-time is optical coherence tomography (OCT) [22–25]. Although OCT shares several advantages with SHM such as label-free, non-destructive, in-situ, and real-time evaluation, it is a white-light interferometric image that provides structural morphology due to changes in tissue scattering properties while SHM is essentially based on the generation of second harmonic signals produced due to inherent non-linear interaction between excitation light and collagen fiber present in the articular cartilage. Therefore, SHM imaging is highly specific to fibrillar collagen and can provide improved contrast in the microscopic image of the articular surface. Thus, the structural and morphological changes associated with collagen fibers in early stage cartilage damage could be visualized in SHM, which might be relatively difficult in OCT due to a lack of collagen specificity. Moreover, differentiation between collagen type-II and type-I can be performed more accurately in p-SHM than in polarized-OCT [26]. However, to date, a comprehensive comparative analysis between SHM and OCT for the evaluation of articular cartilage remains missing from the literature.
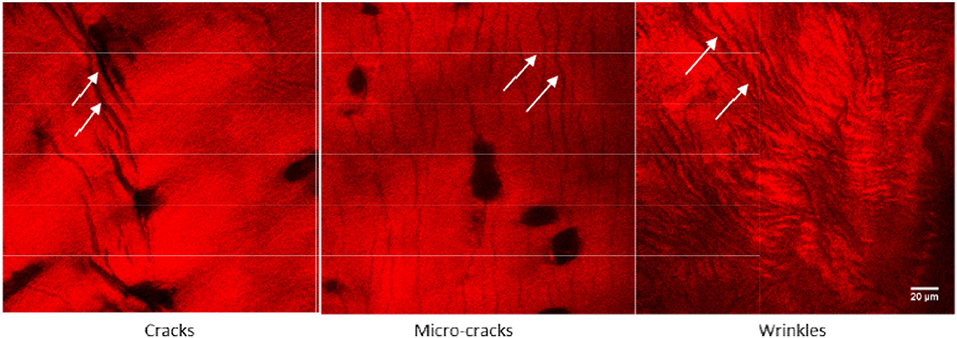
Figure 2. Novel structural features such as micro-cracks and wrinkle like patterns were observed in an early stage of human osteoarthritic cartilage. These features are visible because of collagen specific imaging, which is possible only through SHM imaging. Adapted with permission from Kumar et al. [12] © The Optical Society.
Current Status: A Short Review of Recent Advances
By comparing the standard clinical methods for cartilage imaging, Bo et al., described that high-resolution measurements of the multilayer ultra-structure of articular cartilage could be highly beneficial for both the research and clinical diagnosis [27]. For example, by imaging the elastin fiber in the articular surface, observing the arrangement of chondrocytes at different loading locations and targeting the transformation between collagen-II to collagen-I in articular cartilage could enhance our understanding of patho-physiology of cartilage disorders, including OA, and could assist in developing the engineered cartilage for repair. Elastin and chondrocytes can be imaged by TPEF while collagen-II and collagen-I can be detected by p-SHM. Alvin et al., assessed the ability of SHM to image ex-vivo bovine articular cartilage and presented (sub-) cellular resolution cartilage images at varying depths without fixing, sectioning, staining, and concluded that the method is sufficiently sensitive to detect pathological changes [28]. By evaluating the cellular pattern in induced cartilage injury, Novakofski et al., demonstrated that the detection of micro-cracks and associated chondrocyte death patterns could be helpful in the assessment of cartilage damage, while subsequent early intervention can assist in the prevention of post-traumatic OA [15]. In the equine model, Mansfield et al., described the distribution of elastin fibers and suggested their role in the mechanical function of articular cartilage [29]. Furthermore, Campagnola et al. performed quantitative measurements of structural changes at both the fiber and fibril assembly level in different cartilage zones to reveal articular cartilage zonal differentiation via SHM [30]. In another study, a step forward, Mansfield et al., recently investigated the microstructural response of articular cartilage zones to mechanical loading and demonstrated that local patterns at the submicron scale organization of collagen fibers could be detected using p-SHM [31]. The same group earlier showed that the polarization sensitivity of SHM may also detect changes in disrupted patterns of the pericellular area within the matrix of degenerated articular cartilage [32]. In histologically damaged articular cartilage, a study analyzed changes in collagen architecture using SHM and demonstrated that cartilage damage is directly related to the reorganization of collagen fiber [10]. In another associated study, histopathological changes in surgically-induced osteoarthritic cartilage were characterized by SHM followed by image analyses, demonstrating that SHM could be an ideal imaging approach for detecting pathological changes in articular cartilage [11]. Our group has demonstrated the novel features (such as microsplits and wrinkle-like patterns) in early stage osteoarthritic human cartilage, which could not otherwise be visualized by standard clinical imaging modalities [12], can be visualized using SHM. The discrimination ability of type-I and type-II collagen by p-SHM further adds to the characterization of articular cartilage, which might be advantageous for the post-operative evaluation of regenerated cartilage in the synovial-joint [26, 33].
An image-processing algorithm that can automatically categorize two-photon autofluorescence images of human articular cartilage into states of OA has previously been demonstrated [34]. In this article, an algorithm based on artificial neuronal network (ANN) in combination with discrete wavelet transformation to determine image features for the classification of OA stage was presented. The validation result shows a sensitivity of 0.88 and a specificity of 0.93. However, these numbers should be treated with caution because the number of patients was not mentioned in the article, and number of cartilage sections was only 31 for all four grades of OA. Inclusion of sufficient interpatient variabilities is an important aspect in such a study. Based on the geometry of cells, Novakofski et al. developed an algorithm to determine the degree of ellipticity to identify the death pattern of chondrocytes after inducing an injury to the articular cartilage [15]. Identification of changes in collagen organization and its inclusion could have been improved the overall cartilage assessments in this study. Moreover, cartilage imaging prior to injury and inclusion of inter-animal variability can enhance the credibility of this work. In another study, recently the Bag of Features (BoF) method based on a statistical texture analysis using the gray-level co-occurrence matrix (GLCM) was explored to extract the image features of cartilage and bone acquired by SHM [35]. Texture features are extracted on individual patches derived from original images to capture the local structural patterns. Using this approach, the discrimination between cartilaginous and osseous tissues with 97.5% accuracy for cartilage images and 94.4% accuracy for bone images were demonstrated. In addition, for discriminating OA pathology, three distinct classes-hyaline, fibrous and bone exposure was detected by BoF algorithm. Hyaline and bone image classifications achieved greater than 90% accuracy while fibrous images only achieved 60–70% accuracy. Cartilage fibrillation on articular surface and collagen microstructure alteration could be early markers of cartilage degeneration. Therefore, detection of such early features with high accuracy will be more important and advantageous for clinical application. In future, a BoF-based algorithm could contribute in this direction.
Notably, SHM images can provide relatively improved morphological features with much higher contrast than two-photon autofluorescence images of articular cartilage [12]. Thus, an image-processing algorithm for SHM images could provide improved results for the automatic categorization of damaged cartilage. Lilledahl et al., has highlighted that extracted quantitative parameters from Fourier image analysis of SHM images may describe the 3D orientation of collagen fibers in cartilage [36].
As a recent breakthrough in technological advancement, SHM images acquired using a flexible fiber-optic-based probe head possess a quality level comparable to images captured by large bench-top laser-scanning microscopes was demonstrated by Liang et al., that shows a promising potential of SHM for in-vivo arthroscopic applications [17]. In another study Stephen et al., recently demonstrated the pre-clinical translation of SHM using a microendoscope and a prototype of a SHM-based arthroscope for the in-vivo assessment of articular cartilage [16]. A summary of major recent advancements in articular cartilage assessments by SHM are included in Table 1.
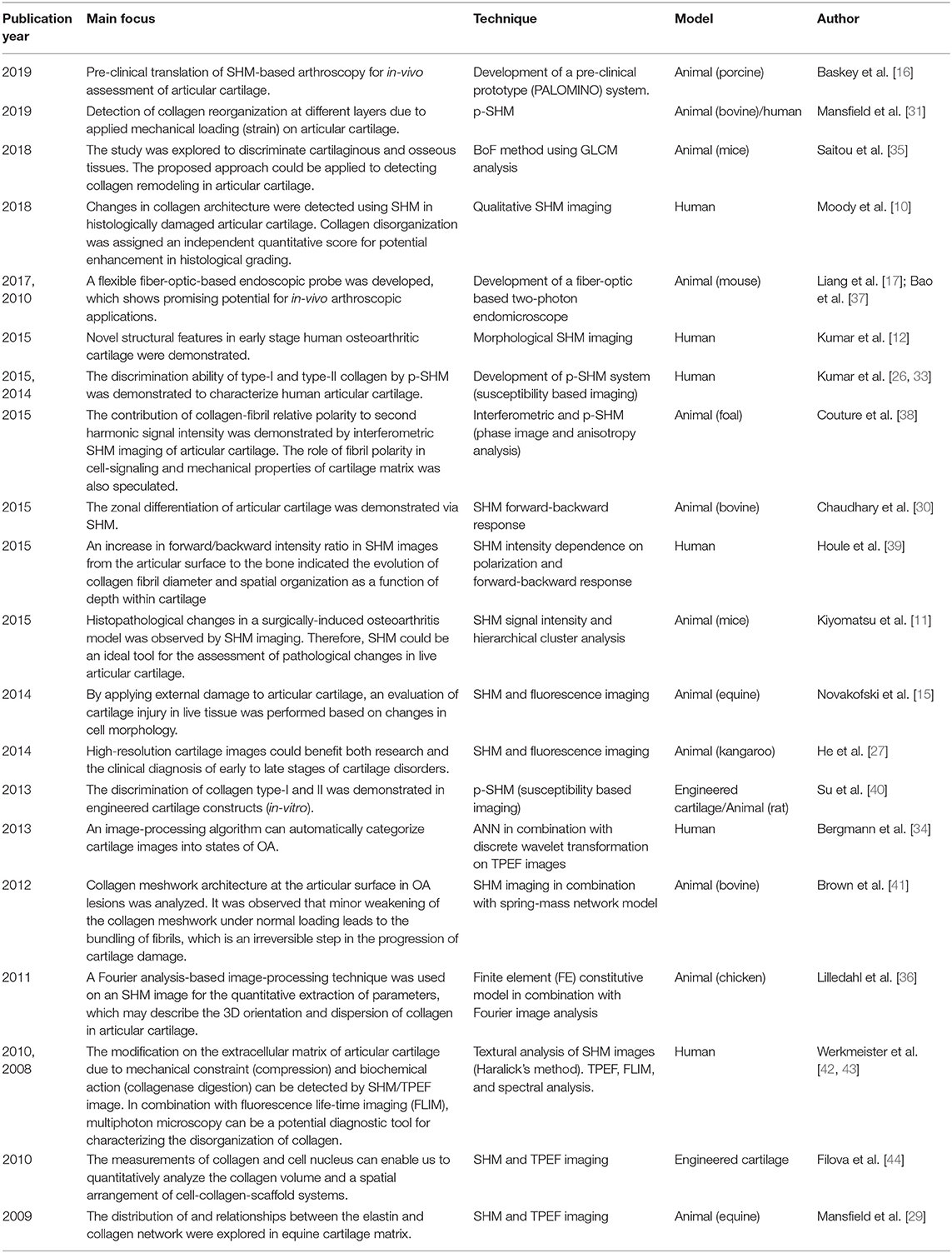
Table 1. Cartilage assessment by second harmonic microscopy: a summary of major recent advancements within the past 10 years only.
Challenges to SHM for Cartilage Assessment
The advantages of SHM can be utilized for in-situ and in-vivo assessments of intact articular cartilage. The major limitation of SHM imaging relates to penetration depth, which is typically limited to hundreds of micrometers. This can be further improved by the application of tissue clearing [45] and adaptive optics [46]. However, SHM cannot achieve full-depth images of articular cartilage, which typically vary between 2 and 4 mm thickness in human knee articular cartilage. The technique is rather useful for surface analysis, and it would be highly interesting to determine whether the articular surface features represent disease progression in the full depth of cartilage. This issue is currently being addressed in our ongoing study associated with OA analysis [47]. A clinically relevant SHM scoring system to represent articular cartilage degradation and aging degeneration would also be highly useful. An initial effort has been undertaken by Hayley et al., which demonstrated that cartilage damage is associated with collagen redistribution, and collagen disorganization could be assigned an independent score [10]. A similar effort associated with SHM scoring system for the assessment of tissue-engineered cartilage has been also reported by Islam et al. [48].
The application of SHM to in-vivo imaging of articular cartilage could be highly complementary and useful for orthopedic surgeon, because a SHM-based arthroscope can provide new information associated with collagen alterations in addition to visual and tactile information acquired through standard arthroscope. An optical-fiber-based SHM probe can be integrated with existing modern clinical arthroscopy, while a miniaturized fiber-optics-based probe head can be designed as a long and thin tube, with several optics inside the tube [17]. The laser light emerging from the probe head can illuminate the cartilage, and the back-scattered light (second harmonic signal) can be collected through the same probe head and guided by fiber-optics to the detector. The first and foremost challenge is the development of a compact, long-wavelength pulsed laser complete with an effective cooling mechanism. The second major challenge lies in maintaining the high peak power of the laser pulse through the propagation of optical fiber, as laser pulses distort due to dispersion along the propagation through the optical fiber. Minimizing the dispersion of femtosecond pulses by introducing the prechirping of pulses prior to launching into fiber-optics-based endoscopic probe could represent one solution. As another solution, adaptive optics can be utilized to enhance peak intensity by shaping the spatial, temporal, and spectral characteristics of the laser pulse [49]. The specialized optical fiber must be designed to maintain the characteristics of the laser pulse as the pulse travels throughout the excitation and collection path. Moreover, the maintenance of polarization states of the laser beam during propagation through the optical-fiber represents another challenge in performing endoscopic p-SHM. However, it has also been demonstrated that the core of double-clad fiber can maintain the polarization state of excitation laser beam [37]. The third major challenge is related to the integration of the SHM probe to an existing arthroscope for orthopedic applications. The optics related to excitation and emission wavelength should be designed and placed in such a manner that they do not affect the existing visual performance of standard arthroscope, which currently utilizes white light. Additionally, providing the choice to change the magnification in order to obtain sub-micron level information and, inclusion of polarization-optics for the detection of collagen types are certainly beneficial, but it further complicates the technical design [50, 51]. Depending on the biomarker or morphological feature, it is expected that a rapid and accurate decision can be made via the direct visualization of SHM images.
The inclusion of an automated image analysis algorithm to categorize the structural pattern and biomarker, as well as its relation to cartilage degeneration stage, can be more helpful [15, 34]. Automated detection and categorizations of those features which couldn't be detected by arthroscope are highly desirable. It could minimize the need of an invasive biopsy of articular cartilage during intra-operative diagnosis. The validation of automated image analysis process for a clinical application needs to follow a robust and rigorous procedure. Although morphology dependent (e.g., Fourier transform, Curvelets, GLCM, Textons) and morphology independent (e.g., p-SHM and SHM directionality) image analysis tools have been used for collagen analysis in tumor, ovary and liver tissues, the progress on assessments of articular cartilage using these tools is slow and needs more attention [52–54]. It would highly interesting to apply these tools for cartilage assessments, especially in human models to explore its clinical relevancy. The pros and cons of these tools are described by Tilbury and Campagnola [55].
Summary and Outlook
Traditionally, the majority of data regarding collagen alterations stems from histological analyses. During cartilage degradation or degeneration, a redistribution of collagen fibers occurs that eventually appears as a disorganization on the articular surface of cartilage by wide-field microscopy. The early alteration in collagen structure, arrangement, and architecture could be a significant pathological marker. Thus, an improved understanding of collagen organization in intact articular cartilage using SHM would be highly significant for research, pre-clinical, and clinical studies. The aforementioned reasons for applying SHM to articular cartilage assessment demonstrate that SHM has a strong potential to reveal microstructural features associated with collagen fibers that could assist researchers and orthopedic surgeons to improve the quality assessment of articular cartilage.
The feasibility of SHM for the assessment of articular cartilage in animals [11, 28, 30, 56], humans [12, 33], and tissue-engineered [44, 48, 57, 58] cartilage constructs have been demonstrated several times. SHM can provide a wealth of information at several levels, including, but not limited to, micron-level surface morphology, changes in collagen architecture, detection of collagen types in regenerated cartilage, label-free analysis, and 3D imaging capability (optical biopsy) within the limit of laser penetration depth. The structural and morphological features acquired by SHM may serve as endogenous biomarkers for the early damage of articular cartilage. The conventional visual and tactile information acquired using a standard arthroscope could be complemented by in-vivo SHM images; therefore, the integration of SHM could ultimately be useful in managing joint-disorders more efficiently. In addition to cartilage evaluation, a SHM-based arthroscope could be a highly rewarding tool for ligament evaluation and repair surgeries by assisting surgeons in the evaluation of correct ligament tension, thus stabilizing the joint and improving the long-term success of anterior cruciate ligament repair. 3D optical biopsy by SHM could not only be utilized for diagnostic and surgical purposes, but also for treatment planning and evaluation procedures. Furthermore, SHM is also emerging as a powerful imaging tool in cartilage tissue engineering. Label-free in-situ detection of collagen expression, collagen types, and associated submicron level structural information could also be highly useful in optimizing the fabrication procedures of bioprinted regenerative articular cartilage [59].
Clinical implementation can only occur if current technological challenges can be overcome through close collaboration between physicists, biologists, engineers, and orthopedic professionals working together to achieve the common goal. The combination of a miniaturized arthroscopic fiber-optics-based probe and image-processing algorithm for the automated categorization of cartilage states will open the opportunity for paradigm-shifting application of SHM in orthopedics for both research and clinical applications. New and bold approaches, such as the addition of SHM into the existing pool of arthroscopy, can be complementary to the standard clinical method and can further assist in fulfilling current orthopedic needs for the ultimate benefit of patients. The growing interest supported by progress in endoscopic probe development is anticipated to continue for the future establishment of SHM for the assessment of articular cartilage.
Author Contributions
RK was the major contributor for this article. RK and AK were involved during the design, drafting, and revision of the manuscript. They have both read and approved the final version of the manuscript for submission.
Funding
RK acknowledges support by Helse Midt-Norge RHF (project number: 46084300) and The Research Council of Norway (project number: 250158).
Conflict of Interest
The authors declare that the research was conducted in the absence of any commercial or financial relationships that could be construed as a potential conflict of interest.
Acknowledgments
The authors would like to acknowledge Professor Catharina de-Lange Davis for the useful discussions and proofreading.
References
1. Sophia Fox AJ, Bedi A, Rodeo SA. The basic science of articular cartilage: structure, composition, and function. Sports Health. (2009) 1:461–8. doi: 10.1177/1941738109350438
2. Fujioka R, Aoyama T, Takakuwa T. The layered structure of the articular surface. Osteoarthr Cartil. (2013) 21:1092–8. doi: 10.1016/j.joca.2013.04.021
3. Hunter DJ, Schofield D, Callander E. The individual and socioeconomic impact of osteoarthritis. Nat Rev Rheumatol. (2014) 10:437–41. doi: 10.1038/nrrheum.2014.44
4. Falah M, Nierenberg G, Soudry M, Hayden M, Volpin G. Treatment of articular cartilage lesions of the knee. Int Orthop. (2010) 34:621–30. doi: 10.1007/s00264-010-0959-y
5. Oussedik S, Tsitskaris K, Parker D. Treatment of articular cartilage lesions of the knee by microfracture or autologous chondrocyte implantation: a systematic review. Arthroscopy. (2015) 31:732–44. doi: 10.1016/j.arthro.2014.11.023
6. Blackburn WD Jr, Bernreuter WK, Rominger M, Loose LL. Arthroscopic evaluation of knee articular cartilage: a comparison with plain radiographs and magnetic resonance imaging. J Rheumatol. (1994) 21:675–9.
7. Blackburn WD Jr, Chivers S, Bernreuter W. Cartilage imaging in osteoarthritis. Semin Arthritis Rheum. (1996) 25:273–81. doi: 10.1016/S0049-0172(96)80037-0
8. Acebes C, Roman-Blas JA, Delgado-Baeza E, Palacios I, Herrero-Beaumont G. Correlation between arthroscopic and histopathological grading systems of articular cartilage lesions in knee osteoarthritis. Osteoarthr Cartil. (2009) 17:205–12. doi: 10.1016/j.joca.2008.06.010
9. Horton WE Jr, Bennion P, Yang L. Cellular, molecular, and matrix changes in cartilage during aging and osteoarthritis. J Musculoskelet Neuronal Interact. (2006) 6:379–81.
10. Moody HR, Afara IO, Singh S, Oloyede A. Potential enhancement of articular cartilage histological grading with collagen integrity. Clin Biomech. (2018) 56:1–10. doi: 10.1016/j.clinbiomech.2018.04.016
11. Kiyomatsu H, Oshima Y, Saitou T, Miyazaki T, Hikita A, Miura H, et al. Quantitative SHM imaging in osteoarthritis model mice, implying a diagnostic application. Biomed Opt Express. (2015) 6:405–20. doi: 10.1364/BOE.6.000405
12. Kumar R, Grønhaug KM, Davies CL, Drogset JO, Lilledahl MB. Nonlinear optical microscopy of early stage (ICRS Grade-I) osteoarthritic human cartilage. Biomed Opt Express. (2015) 6:1895–903. doi: 10.1364/BOE.6.001895
13. Link TM. Cartilage Imaging: Significance, Techniques, and New Developments. New York, NY: Springer-Verlag (2011).
14. Pathria MN, Chung CB, Resnick DL. Acute and stress-related injuries of bone and cartilage: pertinent anatomy, basic biomechanics, and imaging perspective. Radiology. (2016) 280:21–38. doi: 10.1148/radiol.16142305
15. Novakofski KD, Williams RM, Fortier LA, Mohammed HO, Zipfel WR, Bonassar LJ. Identification of cartilage injury using quantitative multiphoton microscopy. Osteoarthr Cartil. (2014) 22:355–62. doi: 10.1016/j.joca.2013.10.008
16. Baskey SJ, Andreana M, Lanteigne E, Ridsdale A, Stolow A, Schweitzer ME. Pre-clinical translation of second harmonic microscopy of meniscal and articular cartilage using a prototype nonlinear microendoscope. IEEE J Transl Eng Health Med. (2018) 7:1800211. doi: 10.1109/JTEHM.2018.2889496
17. Liang W, Hall G, Messerschmidt B, Li M.-J, Li X. Nonlinear optical endomicroscopy for label-free functional histology in vivo. Light Sci Appl. (2017) 6:e17082. doi: 10.1038/lsa.2017.82
18. Centonze VE, White JG. Multiphoton excitation provides optical sections from deeper within scattering specimens than confocal imaging. Biophys J. (1998) 75:2015–24. doi: 10.1016/S0006-3495(98)77643-X
20. Campagnola P. Second harmonic generation imaging microscopy: applications to diseases diagnostics. Anal Chem. (2011) 83:3224–31. doi: 10.1021/ac1032325
21. Nebelung W, Pap G, Machner A, Eberhardt R, Neumann HW, Roessner A. Evaluation of arthroscopic articular cartilage biopsy for osteoarthritis of the knee. Arthroscopy. (2001) 17:286–9. doi: 10.1053/jars.2001.21488
22. Michalik R, Pauer T, Brill N, Knobe M, Tingart M, Jahr H, et al. Quantitative articular cartilage sub-surface defect assessment using optical coherence tomography: an in-vitro study. Ann Anat. (2019) 221:125–34. doi: 10.1016/j.aanat.2018.10.001
23. Li X, Martin S, Pitris C, Ghanta R, Stamper DL, Harman M, et al. High-resolution optical coherence tomographic imaging of osteoarthritic cartilage during open knee surgery. Arthritis Res Ther. (2005) 7:R318–23. doi: 10.1186/ar1491
24. Malley MJ, Chu CR. Arthroscopic optical coherence tomography in diagnosis of early arthritis. Minim Invasive Surg. (2011). 2011:671308. doi: 10.1155/2011/671308
25. Zhou X, Ju MJ, Huang L, Tang S. Correlation between polarization sensitive optical coherence tomography and SHM microscopy in articular cartilage. In: SPIE. San Francisco, CA (2017) doi: 10.1117/12.2254929
26. Kumar R, Grønhaug KM, Romijn EI, Finnøy A, Davies CL, Drogset JO, et al. Polarization second harmonic generation microscopy provides quantitative enhanced molecular specificity for tissue diagnostics. J Biophotonics. (2015) 8:730–9. doi: 10.1002/jbio.201400086
27. He B, Wu JP, Kirk TB, Carrino JA, Xiang C, Xu J. High-resolution measurements of the multilayer ultra-structure of articular cartilage and their translational potential. Arthritis Res Ther. (2014) 16:205. doi: 10.1186/ar4506
28. Yeh AT, Hammer-Wilson MJ, Van Sickle DC, Benton HP, Zoumi A, Tromberg BJ, et al. Nonlinear optical microscopy of articular cartilage. Osteoarthr Cartil. (2005) 13:345–52. doi: 10.1016/j.joca.2004.12.007
29. Mansfield J, Yu J, Attenburrow D, Moger J, Tirlapur U, Urban J, et al. The elastin network: its relationship with collagen and cells in articular cartilage as visualized by multiphoton microscopy. J Anat. (2009) 215:682–91. doi: 10.1111/j.1469-7580.2009.01149.x
30. Chaudhary R, Campbell KR, Tilbury KB, Vanderby R Jr, Block WF, Kijowski R, et al. Articular cartilage zonal differentiation via 3D Second-Harmonic Generation imaging microscopy. Connect Tissue Res. (2015) 56:76–86. doi: 10.3109/03008207.2015.1013192
31. Mansfield Jessica C, Mandalia V, Toms A, Winlove CP, Brasselet S. Collagen reorganization in cartilage under strain probed by polarization sensitive second harmonic generation microscopy. J R Soc Interface. (2019) 16:20180611. doi: 10.1098/rsif.2018.0611
32. Mansfield JC, Winlove CP, Moger J, Matcher SJ. Collagen fiber arrangement in normal and diseased cartilage studied by polarization sensitive nonlinear microscopy. J Biomed Opt. (2008) 13:044020. doi: 10.1117/1.2950318
33. Kumar R, Grønhaug KM, Romijn EI, Drogset JO, Lilledahl MB. Analysis of human knee osteoarthritic cartilage using polarization sensitive second harmonic generation microscopy. In: SPIE Photonics Europe, SPIE (2014). p. 9. doi: 10.1117/12.2051989
34. Bergmann T, Maeder U, Fiebich M, Dickob M, Nattkemper TW, Anselmetti D. Categorization of two-photon microscopy images of human cartilage into states of osteoarthritis. Osteoarthr Cartil. (2013) 21:1074–82. doi: 10.1016/j.joca.2013.04.019
35. Saitou T, Kiyomatsu H, Imamura T. Quantitative morphometry for osteochondral tissues using second harmonic generation microscopy and image texture information. Sci Rep. (2018) 8:2826. doi: 10.1038/s41598-018-21005-9
36. Lilledahl MB, Pierce DM, Ricken T, Holzapfel GA, de Davies CL. Structural analysis of articular cartilage using multiphoton microscopy: input for biomechanical modeling. IEEE Trans Med Imaging. (2011) 30:1635–48. doi: 10.1109/TMI.2011.2139222
37. Bao H, Boussioutas A, Jeremy R, Russell S, Gu M. Second harmonic generation imaging via nonlinear endomicroscopy. Opt Express. (2010) 18:1255–60. doi: 10.1364/OE.18.001255
38. Couture CA, Bancelin S, Van der Kolk J, Popov K, Rivard M, Legare K, et al. The impact of collagen fibril polarity on second harmonic generation microscopy. Biophys J. (2015) 109:2501–10. doi: 10.1016/j.bpj.2015.10.040
39. Houle MA, Couture CA, Bancelin S, Van der Kolk J, Auger E, Brown C, et al. Analysis of forward and backward Second Harmonic Generation images to probe the nanoscale structure of collagen within bone and cartilage. J Biophotonics. (2015) 8:993–1001. doi: 10.1002/jbio.201500150
40. Su PJ, Chen WL, Li T-H, Chou C-K, Chen T-H, Ho Y-Y, et al. The discrimination of type I and type II collagen and the label-free imaging of engineered cartilage tissue. Biomaterials. (2010) 31:9415–21. doi: 10.1016/j.biomaterials.2010.08.055
41. Brown CP, Houle MA, Chen M, Price AJ, Légaré F, Gill HS. Damage initiation and progression in the cartilage surface probed by nonlinear optical microscopy. J Mech Behav Biomed Mater. (2012) 5:62–70. doi: 10.1016/j.jmbbm.2011.08.004
42. Werkmeister E, de Isla N, Netter P, Stoltz JF, Dumas D. Collagenous extracellular matrix of cartilage submitted to mechanical forces studied by second harmonic generation microscopy. Photochem Photobiol. (2010) 86:302–10. doi: 10.1111/j.1751-1097.2009.00648.x
43. Werkmeister E, de Isla N, Marchal L, Mainard D, Stoltz J-F, Dumas D. Interest of second harmonic generation imaging to study collageneous matrix modification in osteoarthritis disease. In: Proc. SPIE 6995, Optical Micro- and Nanometrology in Microsystems Technology II (2008). p. 69950K–10. doi: 10.1117/12.781051
44. Filova E, Burdikova Z, Rampichova M, Bianchini P, Capek M, Kostakova E, et al. Analysis and three-dimensional visualization of collagen in artificial scaffolds using nonlinear microscopy techniques. J Biomed Opt. (2010) 15:066011. doi: 10.1117/1.3509112
45. Ochoa LF, Kholodnykh A, Villarreal P, Tian B, Pal R, Freiberg AN, et al. Imaging of murine whole lung fibrosis by large scale 3D microscopy aided by tissue optical clearing. Sci Rep. (2018) 8:13348. doi: 10.1038/s41598-018-31182-2
46. Olivier N, Débarre D, Beaurepaire E. Dynamic aberration correction for multiharmonic microscopy. Opt Lett. (2009) 34:3145–7. doi: 10.1364/OL.34.003145
47. Kumar R, Davies C, Drogset J, Lilledahl M. Multiphoton microscopy of osteoarthritic articular cartilage. In: Optics in the Life Sciences Congress, San Diego, CA: Optical Society of America (2017). p. NW4C.4. doi: 10.1364/NTM.2017.NW4C.4
48. Islam A, Romijn EI, Lilledahl MB, Martinez-Zubiaurre I. Non-linear optical microscopy as a novel quantitative and label-free imaging modality to improve the assessment of tissue-engineered cartilage. Osteoarthr Cartil. (2017) 25:1729–37. doi: 10.1016/j.joca.2017.06.008
49. Tu H, Liu Y, Turchinovich D, Marjanovic M, Lyngsø JK, Lægsgaard J, et al. Stain-free histopathology by programmable supercontinuum pulses. Nat. Photonics. (2016) 10:534. doi: 10.1038/nphoton.2016.94
50. Jones CW, Smolinski D, Willers C, Yates PJ, Keogh A, Fick D, et al. Laser scanning confocal arthroscopy of a fresh cadaveric knee joint. Osteoarthr Cartil. (2007) 15:1388–96. doi: 10.1016/j.joca.2007.05.003
51. Jones CW, Willers C, Keogh A, Smolinski D, Fick D, Yates PJ, et al. Matrix-induced autologous chondrocyte implantation in sheep: objective assessments including confocal arthroscopy. J Orthop Res. (2008) 26:292–303. doi: 10.1002/jor.20502
52. Stanciu S, Hristu R, Dumitru A, Buga RM, Totu T, Popescu M, et al. Towards automated tissue characterization using parallel bag-of-features experts dealing with two-photon excitation fluorescence and second harmonic generation microscopy datasets. In: 20th International Conference on Transparent Optical Networks (ICTON). Bucharest (2018). p. 1–4. doi: 10.1109/ICTON.2018.8473668
53. Huttunen MJ, Hassan A, McCloskey CW, Fasih S, Upham J, Vanderhyden BC, et al. Automated classification of multiphoton microscopy images of ovarian tissue using deep learning. J Biomed Opt. (2018) 23:1–7. doi: 10.1117/1.JBO.23.6.066002
54. Scodellaro R, Bouzin M, Mingozzi F, D'Alfonso L, Granucci F, Collini M, et al. Whole-section tumor micro-architecture analysis by a two-dimensional phasor-based approach applied to polarization-dependent second harmonic imaging. Front Oncol. (2019) 9:527. doi: 10.3389/fonc.2019.00527
55. Tilbury K, Campagnola PJ. Applications of second-harmonic generation imaging microscopy in ovarian and breast cancer. Perspect Med Chem. (2015) 7:21–32. doi: 10.4137/PMC.S13214
56. Brockbank KG, MacLellan WR, Xie J, Hamm-Alvarez SF, Chen ZZ, Schenke-Layland K. Quantitative second harmonic generation imaging of cartilage damage. Cell Tissue Bank. (2008) 9:299–307. doi: 10.1007/s10561-008-9070-7
57. Calle EA, Vesuna S, Dimitrievska S, Zhou K, Huang A, Zhao L, et al. The use of optical clearing and multiphoton microscopy for investigation of three-dimensional tissue-engineered constructs. Tissue Eng. Part C Methods. (2014) 20:570–7. doi: 10.1089/ten.tec.2013.0538
58. Rice WL, Firdous S, Gupta S, Hunter M, Foo CWP, Wang Y, et al. Non-invasive characterization of structure and morphology of silk fibroin biomaterials using non-linear microscopy. Biomaterials. (2008) 29:2015–24. doi: 10.1016/j.biomaterials.2007.12.049
Keywords: second harmonic microscopy, collagen imaging, articular cartilage, orthopedics, collagen type-II, cartilage degeneration, label-free tissue imaging and diagnostics
Citation: Kumar R and Kumar A (2019) Assessment of Articular Cartilage by Second Harmonic Microscopy: Challenges and Opportunities. Front. Phys. 7:137. doi: 10.3389/fphy.2019.00137
Received: 07 March 2019; Accepted: 06 September 2019;
Published: 24 September 2019.
Edited by:
Paolo Bianchini, Italian Institute of Technology, ItalyReviewed by:
Francesca Rossi, Nello Carrara Institute of Applied Physics (IFAC), ItalyShu-Chi A. Yeh, Wellman Center for Photomedicine, Massachusetts General Hospital, United States
Copyright © 2019 Kumar and Kumar. This is an open-access article distributed under the terms of the Creative Commons Attribution License (CC BY). The use, distribution or reproduction in other forums is permitted, provided the original author(s) and the copyright owner(s) are credited and that the original publication in this journal is cited, in accordance with accepted academic practice. No use, distribution or reproduction is permitted which does not comply with these terms.
*Correspondence: Rajesh Kumar, MTAxcmFqZXNoQGdtYWlsLmNvbQ==