- Istituto Nazionale Fisica Nuclere Sezione di Roma, Sapienza Università di Roma, Rome, Italy
Astronomical and cosmological observations support the existence of invisible matter that can only be detected through its gravitational effects, thus making it very difficult to study. Dark matter makes up about 27% of the known universe. As a matter of fact, one of the main goals of the physics program of the experiments at the Large Hadron Collider of the CERN laboratory is the search of new particles that can explain dark matter. This review discusses both experimental and theoretical aspects of searches for Weakly Interacting Massive Particle candidates for dark matter at the LHC. An updated overview of the various experimental search channels performed by the ATLAS experiment is presented in order to pinpoint complementarity among different types of LHC searches and the interplay between the LHC and direct and indirect dark matter searches.
1. Introduction
Understanding the nature of dark matter (DM) is one of today's major open questions in fundamental physics. Astrophysical and cosmological observations provide strong evidence that most of our universe's energy budget consists of unknown entities. Analysis of anisotropies of the cosmic microwave background provides overwhelming evidence in support of the existence of dark matter five times more abundant than ordinary baryonic matter [1].
The Standard Model (SM) does not provides a viable candidate dark matter particle—consequently, new physics is needed to explain it under the common hypothesis that dark matter is made of massive neutral particles featuring a weak self-interaction (WIMPs). A WIMP is an electrically neutral, colorless, stable particle with a mass in the range from a few MeV to the electroweak scale. The WIMP paradigm naturally accounts for the DM relic density in the universe observed today and is highly attractive both experimentally, because of the feasibility of WIMP searches with several techniques, and from the point of view of theoretical physics, as viable candidates for DM particles are predicted in several models of physics beyond the SM.
Experimentally, three approaches are pursued today to probe the WIMP DM particle hypothesis: production at particle accelerators, indirectly by searching for signals from annihilation products, or directly via scattering on target nuclei. In Figure 1 a schematic representation of the coupling of SM and DM particles is shown, illustrating possible dark matter detection channels.
Searches for DM at colliders require the dark matter particle and its mediator to be within the energetic reach of the collider itself, a limitation not present in direct and indirect searches. By contrast, direct and indirect searches are less sensitive to low mass DM and generally are affected by larger experimental uncertainties, due to uncertainties in the knowledge of the distribution of DM in the universe.
All this makes collider searches for dark matter highly complementary and competitive to direct and indirect DM detection methods, and the ATLAS [2] and CMS [3] experiments at the Large Hadron Collider (LHC) are in a privileged position to try to provide a answer to the dark matter problem.
It is important to notice here that WIMP dark matter it is not the only possibility to provide a particle-based solution to the dark matter problem. Several dark matter models that do not follow the WIMP paradigm have been proposed, many of them testable at the LHC energy scale, hidden valley and dark-sector models [4–6], or asymmetric dark-matter models [7], to name a few. Given the constraints imposed by the mini-review setting of this write-up, the results of these very interesting and complementary searches carried on in ATLAS will be not discussed here, but a list of the most updated results of searches from ATLAS can be found in ATLAS Collaboration [8] while an example of the discussion regarding the general approach to probing for dark matter physics at accelerators can be found in Belotsky et al. [9] and Khlopov [10].
The LHC completed at the end of 2018 its second phase of operations (Run 2), which started in 2015. In three years of data-taking at a center-of-mass energy of 13 TeV, the LHC has delivered a total integrated luminosity of about 150 fb−1 to the ATLAS and CMS experiments, a significant increase over the initial three-year LHC Run 1. The accumulated data allows us to extend the experimental sensitivity to the production of new particles and rare new processes, including WIMPS particles with cross-sections at the level of the femtobarn. The results reported in this short summary are based on data collected by the ATLAS [8] experiment in the first two years of Run 2, a relatively small portion of the whole Run 2 data set in our hands today.
2. Theoretical Framework
Current ignorance of the particle physics nature of DM requires that several different interpretative approaches need to be considered in order to cover the wider possible portion of the DM theory space. Each approach features strengths and weaknesses, so it is highly desirable from the phenomenological point of view to pursue all the different approaches in parallel.
One of the approaches widely used, especially in early work at the LHC, is the effective field theory (EFT). In EFT minimal assumptions on the new particle spectrum are made: the DM particle is the only new state beyond the SM kinematically accessible at the LHC. In this context, EFT describes a general contact-like interaction between SM and dark matter mediated by particles with masses above the kinematical reach of the machine. As the EFT assumes that the kinematic distributions are independent from the new physics scale Λ, it is customary to present the results of the searches for DM in terms of limits on the new physics scale as a function of the DM mass. For DM masses smaller than the missing transverse momentum requirements imposed by the experimental selection, the bounds become independent of the DM mass and so LHC results can be sensitive down to very small DM masses. In the context of EFT it is also possible to have contact interactions between DM and Higgs or vector bosons at the LHC, resulting in characteristic experimental signatures indicated for simplicity with the names mono-Higgs and mono-W/Z (mono-V).
The simplicity and model independence of the EFT approach clash with the observation that many interesting models describing the production of DM at the LHC are not correctly captured by the EFT approach [11]. Moreover, it has been shown how in specific conditions the effective operator approach makes unphysical predictions so that it becomes impossible to find an associated plausible complete model [12].
To avoid these limitations the use of the EFT approach is restricted to processes with sufficiently small momentum transfer, obtaining in these conditions conservative and model-independent bounds on the new physics scale [13].
At the opposite side—with respect to the EFT approach—are the complete models, like SUSY or Universal Extra Dimensions, just to mention two popular ones. Complete models are by definition more theoretically sound but, in contrast, the realization of DM depends crucially on the fine details of the model, and so complete models lack generality.
Between the EFT models and complete models are the so-called simplified dark matter models, which play a crucial role in interpreting today's results of DM searches at the LHC. In simplified models, the assumption that DM particles are the only new particles kinematically accessible is released, and the possibility to have a second light new particle responsible for mediating the interactions of quarks and DM is introduced [14].
These models resolve the EFT contact interaction into an s- or t-channel exchange of the mediator. Compared to EFT models, simplified models introduce additional parameters: DM mass, mediator mass, and couplings of the mediator to SM and DM particles.
Common benchmark scenarios for the simplified model baseline couplings scenarios have been agreed by the LHC DM Working Group [15]. In this approach DM is described as a Dirac particle, and mediators with scalar, pseudo-scalar, vector and axial-vector couplings are considered. To preserve minimal flavor violation content of the model, scalar, and pseudoscalar mediators are assumed to have Higgs-like couplings with quarks, while vector mediators are assumed to have universal couplings to quarks.
3. Searches for Dark Matter at the LHC
In the following sections, the most important aspects of the experimental strategies applied in searches for DM at the LHC are briefly highlighted, summarizing the most recent experimental results obtained by the ATLAS experiment.
DM searches performed in ATLAS can be subdivided into three main categories: searches in final states with dark matter, searches without dark matter itself in the final state, and search for light dark matter signals from dark sectors. The first case focus is the so-called mono-X searches, which arise when the DM mass is small compared to the mediator, and whereby the WIMP pair is then boosted opposite to the direction of the visible particle, leading to the mono-X signature. Searches for final states without dark matter become important when the DM mass is heavier than the mediator. Production cross-section suppression due to off-shell mediators results in fact in weaker constraint from mono-X searches, and a stronger constraint can be obtained looking for example at distortions in the di-jet invariant mass spectrum and/or angular correlations. Finally new physics models with dark sectors may provide a way for light dark matter particles to hide in the hidden sector and to escape the strict limits placed by standard searches. Dedicated searches for signals from dark sectors in both prompt and displaced topologies have been performed in ATLAS in Run 1 and Run 2 data [16–18], but due to space constraints will not be described in this review.
It is worth noticing at this stage of the summary that so far no significant excess has been observed in any of the performed searches. If a wide portion of the theory parameter space remains still open to exploration from ATLAS in the following years, the obtained results can nevertheless already be used to place significant constraints on a large number of interesting dark matter models and masses.
3.1. Searches Based on Missing Transverse Momentum
Light enough DM to be produced in proton-proton collisions at the LHC can be produced in association with one or more QCD jets from initial state radiation. Due to the large QCD coupling with respect to other interactions and the distinctive experimental signature, searches for events with one or more energetic jets produced in association with large missing transverse momentum () have been extensively studied at the LHC not only in the context of searches for DM but also in other contexts beyond SM physics, like large extra spatial dimensions and supersymmetry.
The final discriminant in the mono-jet analyses is the distribution of , in which DM is expected to produce an excess over SM expectations, typically at high . The signal includes also searches for jets from hadronic decay of W and Z bosons (more properly indicated with the name mono-V), where at the LHC energies the decay products are typically boosted, producing distinctive signatures with large-radius collimated jets with a substructure reflecting the origin process [19]. For low DM masses most of the analysis sensitivity comes from the mono-jet production mode, whereas for higher masses mono-V gains importance. The main backgrounds are and W(lν) + jets, and are estimated by data-driven techniques in a number of dedicated data control regions. The remaining backgrounds from di-boson processes are determined using MC simulated samples [20].
In a similar manner as in mono-jet events, DM may also be produced in association with a vector boson radiated off a quark in the initial state. This give rise to very clean signatures (mono-photon, mono-Z, mono-W) although with smaller production rates when compared with the mono-jet case. Mono-photon and mono-Z [21, 22] with leptonically decaying Z bosons are among the conceptually simplest searches for DM, with very low experimental backgrounds and with a discovery sensitivity substantially limited only by the available data statistic. It is worth noticing here that if DM particles couple directly to a pair of gauge bosons, mono-V processes may be the only way in which DM is produced at the LHC.
If DM particles couple dominantly to heavy quark, for example when DM is produced via the exchange of a scalar or pseudo-scalar mediator, then to preserve minimum flavor violation Yukawa-like couplings proportional to the fermion mass are preferred, producing final states with heavy flavor quarks and large . Final states with DM+, DM+, DM+b, and DM+t have been probed by the ATLAS experiment, implementing several different discriminating variables in addition to transverse momentum and to cope with the large multiplicity of different final states [23–25].
Finally, the discovery of the Higgs boson provides additional ways to search for DM at the LHC. Searches for invisible Higgs boson decays, sensitive to decays of the Higgs in DM particles, have been performed using multiple production and decay channels. Among the different production mechanisms that have been studied by ATLAS, the vector boson fusion (VBF) production of Higgs bosons decaying into invisible particles is the most sensitive one. The most stringent limits to date still derive from the Run 1 analyses performed at = 8 TeV [26]. For the mono-Higgs searches in case of a SM-like Higgs boson, the couplings of the Higgs to initial state quarks are Yukawa suppressed, so the Higgs is directly produced by the mediator. Different theory models give rise to mono-Higgs signatures and have been studied by ATLAS looking at decay modes of the Higgs boson in photons and b-quarks [27, 28]. In the h(γγ)+ search two leading photons with pT > 25 GeV are chosen to reconstruct the Higgs candidate. The background is estimated from data by fitting an analytical function to the diphoton invariant mass spectrum. Non-resonant background distribution is also fitted on data assuming an empiric exponential function. Signal sensitivity in the analysis is optimized by dividing the events in two orthogonal signal regions addressing resolved and boosted Higgs boson candidates. The signal regions are then categorized according to the content in the number of b-tagged jets. Finally the signal is extracted by a simultaneous binned likelihood fit to the -jets invariant mass distribution. The dominant background from the multi-jet is estimated using data-driven techniques.
3.2. Indirect Searches for Dark Matter Mediators
Searches for final states without dark matter provide important constraints and complementarity with respect to the various mono-X searches discussed above. If DM can be produced at the LHC via, for example, a s-channel process, the mediator of the process may decay back into quarks, gluons, or leptons, and so the results of searches for narrow resonances—for example in the dijet mass spectrum—can be interpreted in terms of the DM models.
ATLAS has explored multiple final states in search of deviations from the SM background expectations, including dijet [29–31], dilepton [32], di-bjet [33], and [34] resonances.
The signature for the dijet search is two high pT jets, and the discriminant is the dijet invariant mass. The analysis looks for a bump on the smoothly falling invariant mass distribution modeled by an empirical parameterized function. Dijet searches excel at probing high mediator masses while sensitivity at low masses is limited by the high pT requirements imposed by the jet triggers. To cope with this limitation, novel strategies have been developed either based on trigger-object level analysis of data (TLA), in which a higher trigger rate is allowed by reducing the data-format size for the events used in the dijet specific analyses, or requiring hard ISR (jet or photon) in addition to a light dijet resonance. Loss of sensitivity in presence of resonances with large widths is instead recovered, at least partially, by looking not only at the invariant mass of the two jets but also their angular distribution.
The dilepton analysis selects events with at least two same-flavor leptons (muons or electrons). Background with two real leptons are modeled using MC samples, while data-driven methods are employed to estimate backgrounds with one or more misidentified lepton.
3.3. Interpretation
None of the searches for dark matter signals described above has shown a significant deviation from the expected background from SM processes. This can be interpreted as exclusion limits on the different signal models described in section 2. Confidence level exclusion limits of 95% are obtained from the signal regions, alone or combined, of the various searches performed by ATLAS by applying the CLs method [35]. Due to space limitations only the most important and general results are described in this short summary review, although a full and updated list of all the results produced by the ATLAS experiment is available in ATLAS Collaboration [36].
In Figure 2, the results of the various experimental signatures expressed as exclusion contours in the dark matter-mediator mass plane are reported for two representative scenarios: a leptophobic vector mediator scenario and a leptophilic vector-mediator scenario. The same results, but for the axial-vector mediator case, are presented in Figure 3 (left) and (right), respectively. For each scenario “thermal relic” curves indicate combinations of DM and mediator mass that are consistent with a DM density of Ω = 0.12 h2 and a standard thermal history. Dark matter models with vector or axial-vector mediators are mainly constrained by dijet resonance searches and associate production of DM and objects from initial state radiation. The very strong limits obtained from the dijet resonance searches are due to the particular choice of the universal quark couplings used as benchmark. Smaller values of gq reduce substantially the constraints from indirect searches for dark matter mediators.
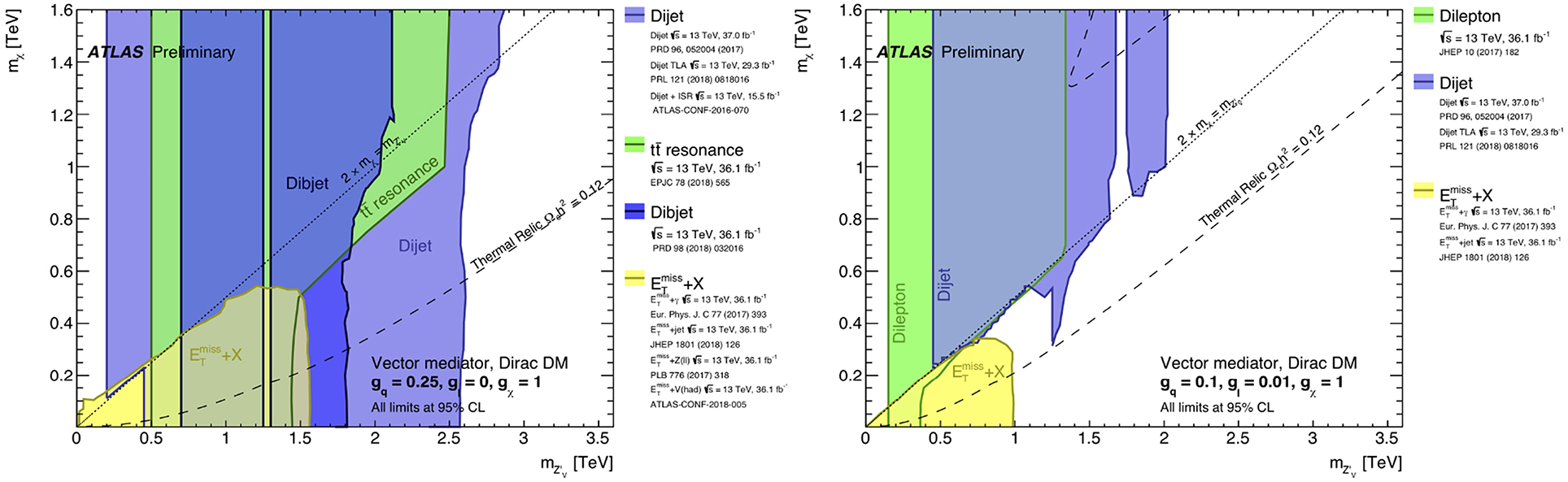
Figure 2. Regions in a DM mass-mediator mass plane excluded at 95% CL by the ATLAS dijet, dilepton and mono-X searches, for vector mediator simplified models with two choices of the couplings: leptophobic scenario (Left) and leptophilic scenario (Right). Exclusions are computed for the DM coupling gχ, the quark coupling qg, universal to all flavors, and the lepton coupling ql, indicated in each plot. The dashed curve “thermal relic" indicates combinations of DM and mediator mass that are consistent with a DM density of Ω = 0.12 h2 and a standard thermal history. A dotted curve indicates the kinematic threshold where the mediator can decay on-shell into DM. Excluded regions that are in tension with the perturbative unitary considerations are indicated by shading in the upper left corner.
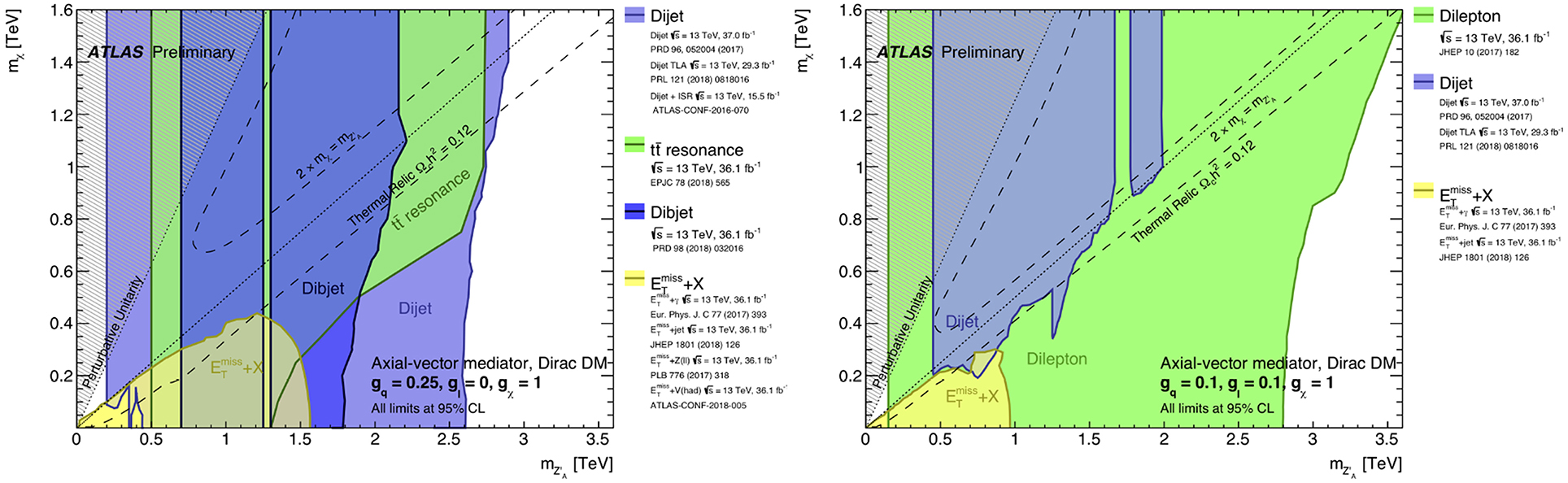
Figure 3. Regions in a DM mass-mediator mass plane excluded at 95% CL by the ATLAS dijet, dilepton and mono-X searches, for axial-vector mediator simplified models with two choices of the couplings: leptophobic scenario (Left) and leptophilic scenario (Right). Exclusions are computed for the DM coupling gχ, the quark coupling qg, universal to all flavors, and the lepton coupling ql, indicated in each plot. The dashed curve “thermal relic" indicate combinations of DM and mediator mass that are consistent with a DM density of Ω = 0.12 h2 and a standard thermal history. A dotted curve indicates the kinematic threshold where the mediator can decay on-shell into DM. Excluded regions that are in tension with the perturbative unitary considerations are indicated by shading in the upper left corner.
The most stringent limits on scalar and pseudo-scalar mediator models come from DM+heavy flavors analyses, in particular from the DM+ final states. In Figure 4 the 95% exclusion limits for color-neutral scalar and pseudo-scalar mediator models as a function of the mediator mass obtained by ATLAS are shown.
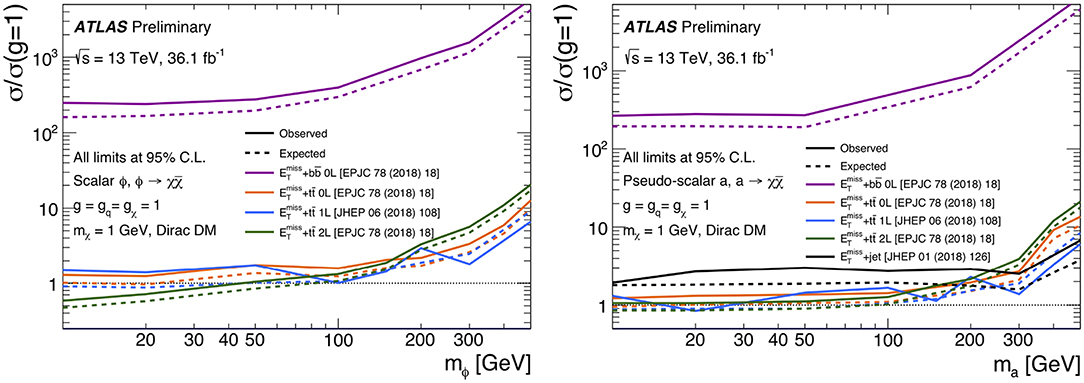
Figure 4. Exclusion limits for color-neutral scalar (Left) or pseudo-scalar (Right) mediator models as a function of the mediator mass for a DM mass of 1 GeV. The limits are calculated at 95% CL and are expressed in terms of the ratio of the excluded cross-section to the nominal cross-section for a coupling assumption of gq = gχ = 1. The solid (dashed) lines show the observed (expected) exclusion limits for each channel.
An interesting comparison between results from searches for DM at colliders and indirect and direct detection experiments can be obtained by translating vector and axial-vector mediator limits on the spin-dependent DM-proton and spin-independent DM-nucleon scattering cross-section as a function of the DM mass. A comparison of the inferred limits from the ATLAS searches to the constraints from direct detection experiments is shown in Figure 5. ATLAS exclusion contours for vector and axial-vector neutral mediator models are complementary to the ones obtained by direct detection, with a better sensitivity for low values of the DM mass where direct experiment loose sensitivity to the below detection threshold energy recoils, induced by the DM scattering. It is important to observe here that the results of the interpretation in both the spin-dependent and spin-independent cross sections depends on the mediator mass and the couplings assumptions, so the comparison is only valid in the context of the specific model and couplings that is assumed.
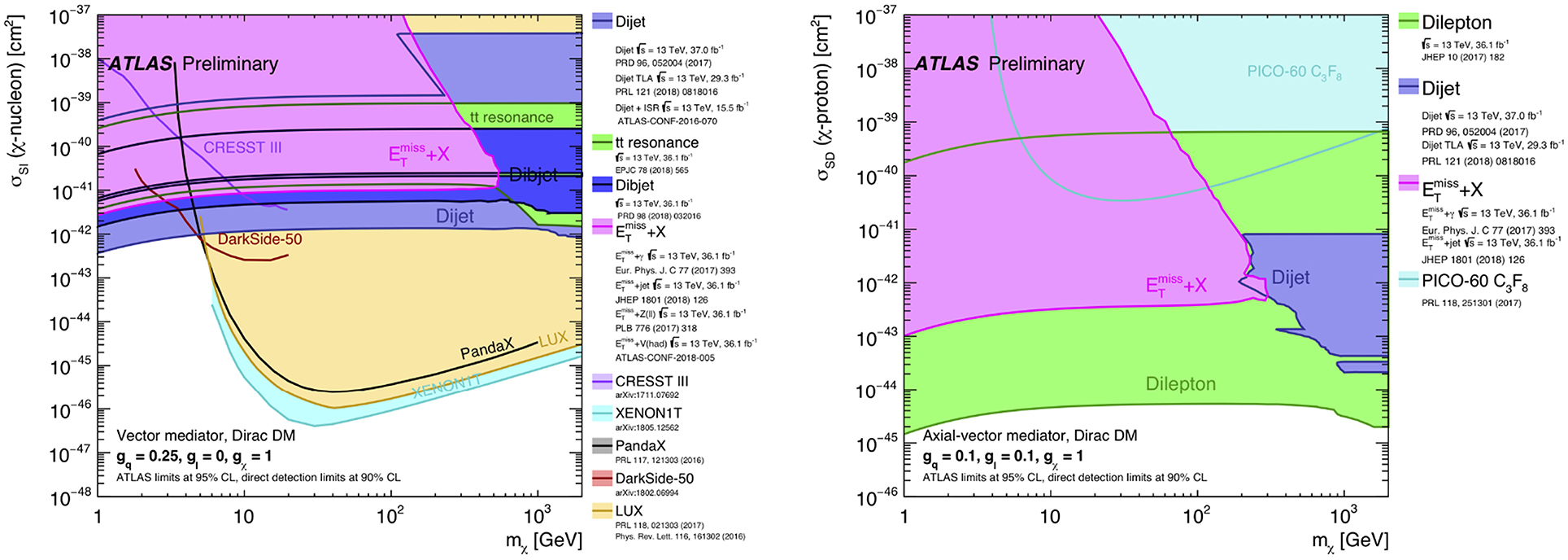
Figure 5. A comparison of the inferred limits to the constraints from direct detection experiments on the spin-dependent WIMP-proton scattering cross-section in the context of the vector leptophobic model (Left) and on the spin-independent WIMP-nucleon scattering cross-section in the context of the axial-vector leptophilic model (Right). The ATLAS results are compared with limits from direct detection experiments. ATLAS limits are shown at 95% CL and direct detection limits at 90% CL. ATLAS searches and direct detection experiments exclude the shaded areas. Exclusions beyond the canvas are not implied for the ATLAS results. The dijet and mono-X exclusion regions represent the union of exclusions from all analyses of that type.
4. Outlook and Future Perspectives
The ATLAS experiment is actively searching for dark matter at the LHC, a thriving research field in both theoretical and experimental fields. There is sensitivity to DM under many model assumptions for the interaction and the mediator, and a large number of different searches have already been performed in final states with both missing transverse momentum and in indirect searches for dark matter mediators. No evidence for DM has been observed so far and present searches performed by ATLAS, within the choice of models and couplings that have been used to interpret the results, already allow for probing a large portion of the parameters space.
One of the most important features of the DM searches at LHC is the strong complementarity with the searches implemented for indirect and direct detection experiments, so that the union of the three efforts allows for probing the full parameter space of many of the most viable and motivated theory models of dark matter.
The ATLAS analyses summarized in this short review are all based on up to 37 fb−1 of proton-proton collision data at a center of mass energy of 13 TeV collected in 2015 and 2016, only a small fraction of the total LHC data set already collected by ATLAS in Run 2. Moreover an even larger data set is expected to be delivered by the LHC in the future, first in Run 3 which is expected to start in 2021 and to accumulate 300 fb−1 of data in three years of data taking, and then in the HL-LHC phase that will eventually deliver to the LHC experiments up to 3,000 fb−1 of proton-proton collisions at 14 TeV.
Using a simplified model in which WIMP pairs are produced from the s-channel exchange of an axial-vector mediator coupling to quarks with a coupling strength of 0.25 and to WIMPs with unit coupling strength, ATLAS has estimated the expected 95% CL limit on the mediator mass in the mono-Jet search for a target luminosity of 3,000 fb−1. An expected 95% CL limit on the mediator mass of 2.65 TeV could be reached for a signal with a WIMP mass of 1 GeV and can be extended up to 2.88 TeV by reducing the systematic uncertainties by a factor of four, an improvement of about 80% with respect to the latest published results from the ATLAS experiment [37].
Only a limited portion of the dark matter model phase space has been explored so far, and much more has yet to be investigated. Combining this with the impressive advances in theoretical developments and in the design and deployment of innovative experimental analysis techniques expected in the following years, we are quite convinced that the experiment-based journey for ATLAS and the LHC in search if dark matter has just begun.
Author Contributions
The author contributed to review and summarize the current status of searches for dark matter signals in the ATLAS experiment at the LHC, and in the writing, and editing of the whole manuscript.
Conflict of Interest Statement
The author declares that the research was conducted in the absence of any commercial or financial relationships that could be construed as a potential conflict of interest.
References
1. Ade PAR, Aghanim N, Arnaud M, Ashdown M, Aumont J, Baccigalupi C, et al. Planck 2015 results. XIII. Cosmological parameters. Astron Astrophys. (2016) 594:A13. doi: 10.1051/0004-6361/201525830
2. ATLAS Collaboration, Aad G, Abat E, Abdallah J, Abdelalim AA, Abdesselam A, et al. The ATLAS Experiment at the CERN Large Hadron Collider. J Instrum. (2008) 3:S08003. doi: 10.1088/1748-0221/3/08/S08003
3. CMS Collaboration, Chatrchyan S, Hmayakyan G, Khachatryan V, Sirunyan AM, Adam W, Bauer T, et al. The CMS experiment at the CERN LHC. J. Instrum. (2008) 3:S08004. doi: 10.1088/1748-0221/3/08/S08004
4. Strassler MJ, Zurek KM. Echoes of a hidden valley at hadron colliders. Phys Lett. (2007) 651:374–9. doi: 10.1016/j.physletb.2007.06.055
5. Curtin D, Essig R, Gori S, Shelton J. Illuminating dark photons with high-energy colliders. J High Energy Phys. (2015) 02:157. doi: 10.1007/JHEP02(2015)157
6. Falkowski A, Ruderman JT, Volansky T, Zupan J. Hidden higgs decaying to lepton jets. J High Energy Phys. (2010) 05:077. doi: 10.1007/JHEP05(2010)077
7. Khlopov MY. Composite dark matter from 4th generation. JETP Lett. (2006) 83:1–4. doi: 10.1134/S0021364006010012
8. ATLAS Collaboration. ATLAS Public Results (2018). Available online at: https://twiki.cern.ch/twiki/bin/view/AtlasPublic/Publications (accessed March 30, 2019).
9. Belotsky KM, Yu Khlopov M, Sakharov AS, Sudarikov AL, Shklyaev AA. Experimental cosmoparticle physics: experimental probes for dark matter physics at particle accelerators. Gravitat Cosmol. (1998) 4:70–78.
10. Khlopov MY. Direct and indirect probes for composite dark matter. Front Phys. (2019) 7:4. doi: 10.3389/fphy.2019.00004
11. Bai Y, Fox PJ, Harnik R. The tevatron at the frontier of dark matter direct detection. J High Energy Phys. (2010) 12:048. doi: 10.1007/JHEP12(2010)048
12. Shoemaker IM, Vecchi L. Unitarity and monojet bounds on models for DAMA, CoGeNT, and CRESST-II. Phys Rev D. (2012) 86:015023. doi: 10.1103/PhysRevD.86.015023
13. ID Racco AW, Zwirner F. Robust collider limits on heavy-mediator dark matter. J High Energy Phys. (2015) 06:126. doi: 10.1007/JHEP05(2015)009
14. Abdallah J, Ashkenazi A, Boveia A, Busoni G, De Simone A, Doglioni C, et al. Simplified models for dark matter and missing energy searches at the LHC. arXiv. (2014) arXiv:1409.2893v2.
15. Albert A, Backovic M, Boveia A, Buchmueller O, Busoni G, De Roeck A, et al. Recommendations of the LHC dark matter working group: comparing LHC searches for heavy mediators of dark matter production in visible and invisible decay channels. arXiv. (2017) arXiv:1703.05703.
16. ATLAS Collaboration. Search for long-lived neutral particles decaying into lepton jets in proton–proton collisions at = 8 TeV with the ATLAS detector. J High Energy Phys. (2014) 11:088. doi: 10.1007/JHEP11(2014)088
17. ATLAS Collaboration. A search for prompt lepton-jets in pp collisions at = 8 TeV with the ATLAS detector. J High Energy Phys. (2016) 02:062. doi: 10.1007/JHEP02(2016)062
18. ATLAS Collaboration. Search for Higgs boson decays to beyond-the-Standard-Model light bosons in four-lepton events with the ATLAS detector at = 13 TeV. J High Energy Phys. (2018) 06:166. doi: 10.1007/JHEP06(2018)166
19. ATLAS Collaboration. Search for dark matter in events with a hadronically decaying vector boson and missing transverse momentum in pp collisions at = 13 TeV with the ATLAS detector. J High Energy Phys. (2018) 10:180. doi: 10.1007/JHEP10(2018)180
20. ATLAS Collaboration. Search for dark matter and other new phenomena in events with an energetic jet and large missing transverse momentum using the ATLAS detector. J High Energy Phys. (2018) 01:126. doi: 10.1007/JHEP01(2018)126
21. ATLAS Collaboration. Search for dark matter at = 13 TeV in final states containing an energetic photon and large missing transverse momentum with the ATLAS detector. Eur Phys J. (2017) 77:393. doi: 10.1140/epjc/s10052-017-4965-8
22. ATLAS Collaboration. Search for an invisibly decaying Higgs boson or dark matter candidates produced in association with a Z boson in pp collisions at = 13 TeV with the ATLAS detector. Phys Lett B. (2018) 776:318. doi: 10.1016/j.physletb.2017.11.049
23. ATLAS Collaboration. Search for dark matter produced in association with bottom or top quarks in = 13 TeV pp collisions with the ATLAS detector. Eur Phys J. (2018) 78:18. doi: 10.1140/epjc/s10052-017-5486-1
24. ATLAS Collaboration. Search for top-squark pair production in final states with one lepton, jets, and missing transverse momentum using 36 fb-1 of = 13 TeV pp collision data with the ATLAS detector. J High Energy Phys. (2018) 06:08. doi: 10.1007/JHEP06(2018)108
25. ATLAS Collaboration. Search for a scalar partner of the top quark in the jets plus missing transverse momentum final state at = 13 TeV with the ATLAS detector. J High Energy Phys. (2017) 12:085. doi: 10.1007/JHEP12(2017)085
26. ATLAS Collaboration. Constraints on new phenomena via Higgs boson couplings and invisible decays with the ATLAS detector. J High Energy Phys. (2015) 11:206. doi: 10.1007/JHEP11(2015)206
27. ATLAS Collaboration. Collaboration, Search for dark matter in association with a Higgs boson decaying to two photons at = 13 TeV with the ATLAS detector. Phys Rev D. (2017) 96:112004. doi: 10.1103/PhysRevD.96.112004
28. ATLAS Collaboration. Search for Dark Matter Produced in Association with a Higgs Boson Decaying to using 36 fb-1 of pp collisions at = 13 TeV with the ATLAS Detector. Phys Rev Lett. (2017) 119:181804. doi: 10.1103/PhysRevLett.119.181804
29. ATLAS Collaboration. Search for new phenomena in dijet events using 37 fb-1 of pp collision data collected at = 13 TeV with the ATLAS detector. Phys Rev D. (2017) 96:052004. doi: 10.1103/PhysRevD.96.052004
30. ATLAS Collaboration. Search for low-mass dijet resonances using trigger-level jets with the ATLAS detector in pp collisions at = 13 TeV. Phys Rev Lett. (2018) 121:081801. doi: 10.1103/PhysRevLett.121.081801
31. ATLAS Collaboration. Search for light resonances decaying to boosted quark pairs and produced in association with a photon or a jet in proton-proton collisions at = 13 TeV with the ATLAS detector. Phys Lett B. (2018) 788:316–35. doi: 10.1016/j.physletb.2018.09.062
32. ATLAS Collaboration. Search for new high-mass phenomena in the dilepton final state using 36 fb-1 of proton-proton collision data at = 13 TeV with the ATLAS detector. J High Energy Phys. (2017) 10:182. doi: 10.1007/JHEP10(2017)182
33. ATLAS Collaboration. Search for resonances in the mass distribution of jet pairs with one or two jets identified as b-jets in proton-proton collisions at = 13 TeV with the ATLAS detector. Phys Rev D. (2018) 98:032016. doi: 10.1103/PhysRevD.98.032016
34. ATLAS Collaboration. Search for heavy particles decaying into top-quark pairs using lepton-plus-jets events in proton-proton collisions at = 13 TeV with the ATLAS detector. Eur Phys J. (2018) 78:565. doi: 10.1140/epjc/s10052-018-5995-6
35. Read AL. Presentation of search results: the CLS technique. J Phys G. (2002) 28:2693–704. doi: 10.1088/0954-3899/28/10/313
36. ATLAS Collaboration. Constraints on Mediator-Based Dark Matter Models Using = 13 TeV pp Collisions at the LHC With the ATLAS Detector (2018). Available online at: https://atlas.web.cern.ch/Atlas/GROUPS/PHYSICS/CONFNOTES/ATLAS-CONF-2018-051/ (accessed March 30, 2019).
Keywords: dark matter, WIMP, Hadron Collider, ATLAS, LHC, particle physics
Citation: Giagu S (2019) WIMP Dark Matter Searches With the ATLAS Detector at the LHC. Front. Phys. 7:75. doi: 10.3389/fphy.2019.00075
Received: 11 February 2019; Accepted: 25 April 2019;
Published: 17 May 2019.
Edited by:
Antonino Marciano, Fudan University, ChinaReviewed by:
Andrea Addazi, Fudan University, ChinaMaxim Yurievich Khlopov, UMR7164 Astroparticule et Cosmologie (APC), France
Copyright © 2019 Giagu. This is an open-access article distributed under the terms of the Creative Commons Attribution License (CC BY). The use, distribution or reproduction in other forums is permitted, provided the original author(s) and the copyright owner(s) are credited and that the original publication in this journal is cited, in accordance with accepted academic practice. No use, distribution or reproduction is permitted which does not comply with these terms.
*Correspondence: Stefano Giagu, c3RlZmFuby5naWFndUByb21hMS5pbmZuLml0