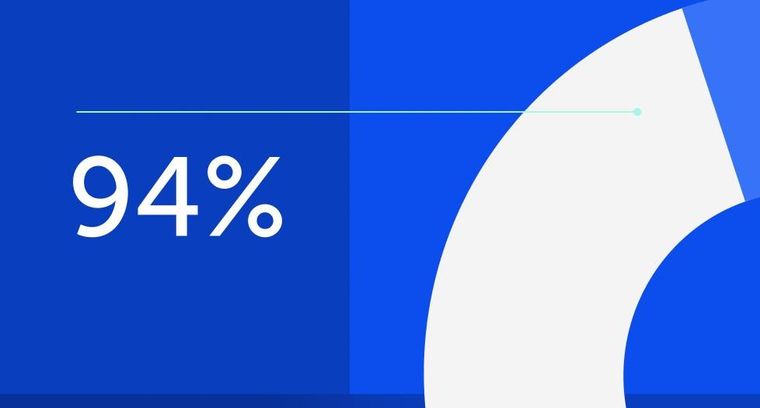
94% of researchers rate our articles as excellent or good
Learn more about the work of our research integrity team to safeguard the quality of each article we publish.
Find out more
REVIEW article
Front. Phys., 19 March 2018
Sec. Chemical Physics and Physical Chemistry
Volume 6 - 2018 | https://doi.org/10.3389/fphy.2018.00025
The vibrational theory of olfaction is an attempt to describe a possible mechanism for olfaction which is explanatory and provides researchers with a set of principles which permit predictions allowing for structure-odor relations. Similar theories have occurred several times throughout olfactory science; this theory has again recently come to prominence by Luca Turin, who suggested that inelastic electron tunneling is the method by which vibrations are detected by the olfactory receptors within the hose. This work is intended to convey to the reader an up-to-date account of the vibrational theory of olfaction, both the historical iterations as well as the present iteration. This text is designed to give a chronological account of both theoretical and experimental studies on the topic, while providing context, comments, and background where they were found to be needed.
Olfaction is the oldest and most fundamental chemical sense by which lifeforms interpret their surroundings. This sense has always fascinated the sciences, delivering a great number of unique theories each divining the mechanism of this process. Richard Axel and Linda B. Buck published a landmark paper reporting their the discovery and cloning of the genetic code for several mammalian olfactory receptors (ORs) within a larger gene family [1]. Soon thereafter the first insect olfactory receptors were independently discovered by three research teams, all working on Drosophila melanogaster [2–4]. Olfaction within vertebrates and insects differs in the types of receptors used; in humans olfaction is performed via G-protein coupled receptors (GPCRs), whereas olfaction in insects primarily uses insect olfactory receptors (insect ORs). At present, the most accepted theory by which olfaction operates is based on the electrostatics and van der Waals surface of the odorant permitting binding to the receptor, after which the receptor undergoes a conformation change from its inactive state to its active state. The interplay between active and inactive conformations was validated as a likely description of activation in central nervous system (CNS) GPCRs through the analysis of the dynamics of the histidine and adenosine receptors [5–10].
Quantum effects in biology are a growing field of interest; this includes coherent energy transfer within photosynthetic bacteria proteins, mechanism of the avian magnetic compass and the possibility of inelastic electronic transfer (IET) occurring in olfactory receptors [11–15]. Considering the possible importance of quantum mechanics in biology, each suspected case of non-trivial quantum effects in biology should be examined skeptically [16]. Our intention in compiling this review is to present the vital findings and models used during the validation and examination of the contemporary Vibrational Theory of Olfaction (VTO), and to address an important question suggested by Barwich [17]: What, if anything, is so special about smell?
The present vibrational theory is an iteration in a historical movement within olfactory science to propose a relationship between molecular vibration and osmic properties. The proposition of vibrational modes controlling the osmic mechanism originates from the works of Malcolm Dyson [18, 19]. Coming off the contemporaneous development of Raman spectroscopy, Dyson believed that probing molecular vibrations of a molecule would elucidate correlations between these vibrations and the osmic properties of the odorant. The proposition was that the thermalized vibrations inherent to a molecule at a given (physiological) temperature would activate the receptor protein. Within his works he made it clear that the considerations of the vibrational components were independent of other properties necessary for odorant detections, such as the molecular volatility. Dyson continued to suggest that vibrational modes within the range of 1,400–3,500 cm−1 were responsible for the activation of olfactory receptor sites. Due to the contemporaneous nature of the application of Maxwell-Boltzmann statistics within his work, the ability of vibrational modes to be thermally excited at physiological temperatures was not addressed; a consideration later taken up by proponents of vibrational theories.
The VTO was revisited by Wright [20] who examined Dyson's model, noting that in higher energy regions vibrations correlate specifically with functional groups; therefore odor could equally be correlated to either vibrational frequency or functional groups. Additionally, the high energy ranges proposed by Dyson were found to have so small a population that it was unlikely that they could be responsible for a reliable olfactory sense. By considering this, Wright proposed that it was vibrations of ~700 cm−1 or less that were responsible for any activation process. Additionally, Wright espoused that the modes below 700 cm−1 were both “more characteristic of the molecule as a whole” and less dependent on the particulars of specific functional groups. In a second work Lloyd [21] analyzed the low energy Raman spectral region of several almond odorants, finding that the collection of almond-odorants all possessed a similarly strong band within the 150–200 cm−1 range. They emphasized that the nose need not act as a Raman spectrographic device, but that the underlying molecular motion is detected through a mechanical—not spectroscopic—means by the receptor.
Contemporaneously, Amoore and Moncrieff independently published the basic concepts and arguments that formed a stereochemical/steric theory governing olfaction; an important theory whose aspects have remained with us to the present [22–26]. Later Wright and Michels completed a survey of 50 test odorants. First, using trained human subjects within two counter-ordered groups to place the odorants into one of nine training classes and then to use these data through a standard similarity matrix method to compare with the Raman spectra. Although the raw Raman data had a low correlation score, the rotationally oriented data (along several olfactory factors) were found to have a high significance factor, implying strong correlation [27].
Doolittle et al. completed a study of the olfactory responses of melon flies (Bactrocera cucurbitae) with deuterated variants of a known attractant. They concluded that there was no relationship between the low-energy infrared frequencies and the perceived effect on the behavior of the exposed melon flies [28, 29]. Blum et al. conducted a similar deuteration study with the Florida harvester ant (Pogonomyrmex badius). Ants were exposed to several ketones to determine which are capable of mimicking the natural alarm pheromone—4-mthyl-3-heptanone—and the successful candidates were then deuterated to test the VTO. They found no evidence supporting a molecular vibration related mechanism for olfaction. A brief report was published by Barker et al. [30] providing highly suggestive evidence that Apis mellifera L. are also incapable of discerning between deuterated variants of nitrobenzene. This paper prompted a response from Wright as the negative conclusion drawn did not include information concerning the infrared spectrum, explicitly lacking information concerning the low energy region. In 1977, Wright completed the basis of his—and several facets of the modern iteration VTO, asserting the feasibility of the theory within a biological context [31]. Subsequent studies were conducted on both the German and the American cockroaches with mixed results. Sugawara et al. [32] and Havens and Meloan [33] Hara contextualized his results as altered molecular motion—vis-á-vis Wright's theory—in his report finding that whitefish (Coregonus clupeaformis) are capable of discriminating between glycine and d5-glycine in a behavioral study [34].
In 1978, Wright completed an information theory analysis that maintained the plausibility of the VTO in the context of a limiting number of receptor sites, and that the osmic spectrum could be feasibly detectable under his theory [35]. Wright attempted to justify the inclusion of his VTO within the context of enantiomeric osmic differences, by noting that the sterochemical aspect alone is insufficient to account for differences [36]. Eriksson et al. completed a study comparing the predictive nature of the contemporary olfactory theories on substituted pyridine and the perseverance of the pure pyridine odor; it was found that a particular out-of-plane collective vibration was present in all the osmically pyridine odorants. It was determined that vibrations were no more predictive than the molecular shape and space filling nature of the electron cloud; additionally, both examined properties were found to be necessary, but not essential properties [37]. Wright further attempted to provide a vibrational explanation for human perception of odor blending [38]. It was not long after these final works that it was clear that the VTO—as proposed by Wright—had no more predictive ability than considering facets of the odorant's structure alone [37, 39, 40].
A contemporary iteration of the VTO was introduced in an effort to generate a systematic approach to the olfactory process, as well as to forward better odorant classification, prediction, and molecular design [41, 42]. Here we will provide a brief description of aspects associated with the VTO as Turin presents it.
Beginning with the works of Dyson and Wright, Turin appends the general theory with a mechanism physically capable of detecting vibrational modes feasibly within biological environments: IET. Many of the important early theoretical methods were devised by Lambe and Jaklevic [43], Kirtley et al. [44], Lambe and McCarthy [45], Khanna and Lambe [46], and Phillips and Adkins [47], while an excellent introductory overview of this spectroscopic method is available from Reed [48]. Speaking generally, this is a method capable of detecting the vibrational modes of a molecule. Importantly, as no light-matter interaction is involved, both IR and Raman active modes appear in IET spectra with roughly equal weight as no invocation of the dipole rule is required. Furthermore, this non-photon driven process would permit a larger range (0–4,000 cm−1) of possible olfactory vibrations to be examined, rather than the highly truncated range (~0–500 cm−1) attributable to a thermally driven process [40].
Specifically, within a laboratory environment, an analyte molecule is deposited upon a metal surface in close proximity to another metal surface, while a tunable electrostatic potential is generated across the insulating gap between the plates. This potential will drive electrons from one side of the gap to the other via evanescent tunneling. During the elastic tunneling processes, electrons are driven from the donor (D) to the acceptor (A) while obeying strict energy conservation; if this is the only process, the junction is Ohmic. This elastic process is shown in Figure 1 as the red arrow; as electrons traversing the gap from the left (donor) are deposited in an energy level on the right (acceptor) with no change in energy. As we have deposited an analyte molecule on one surface, other processes may take place. The tunneling electron will be capable of donating a quantum of energy to the analyte before moving on to the acceptor; this process being termed first-order inelastic tunneling, shown as the green arrow within Figure 1. The electron leaves the donor energy level, and traverses the gap, falling into an energy level at the acceptor with less energy than initially, due to the electron having donated a quantum of energy to the “molecule” within the figure. Within the figure, the “molecule” is represented by a one-dimensional quantum harmonic oscillator, while in reality the molecule is comprised of anharmonic collective oscillations. Yet this distinction makes no difference in the physics as the important facets remain: the molecule retains the ability to resonantly absorb specific quanta of energy, absorption of off-resonant energy will result in emission, and highly local absorption/interaction can result in energy redistribution throughout the normal coordinates of a specific phase-consistent motion. Higher energy levels of a real molecule may undergo energetic shifts due to the incorporation of more anharmonic character, yet a system evolved to detect molecules in this fashion would likely (entirely hypothetically) have evolved with an “understanding” of the typical energy required to provide a quantum of energy to the molecule (on average) while it sits in a typical (most populated, average) vibrational energy distribution. Subsequently, the vibrational state population of the oscillator will increase from merely thermally activated (magenta shading) to a new distribution incorporating the additional excitation originating from the electron (green shading). This new pathway for electron transfer presents itself as a minute increase in the measured tunneling current across the gap: non-Ohmic behavior. These increases are typically small enough that plotting the second derivative of the IR curve with respect to V, , is beneficial for their visualization. There are other processes involved, such as second-order elastic processes (where the electron donates and accepts quanta from the molecule), but such higher-order processes (not pictured in Figure 1) typically present diminishing contributions to the over-all current with an increase in order.
Figure 1. A schematic representation of the electron-donor(left)-acceptor (right) system as is of concerning in IET. A full description is discussed within the main text.
Within the context of Turin's theory the odorant binding site of the OR acts as the tunneling junction. The electron donor and acceptor sites within the biological system have been suggested to be charged amino acid sidechains or, possibly, a redox ready metal. Odorants, in general, are not redox ready or charged; both of these traits would hinder the volatility of the odorant, detrimentally affecting the ability of the molecule to act as an odorant. As there is no continuum of unoccupied states within the likely biological acceptor, this biological tunneling gap is capable of electron transfer at a finite number of specific energy values; that carries two important implications: (1) The probability of a biological Ohmic junction is very low (and would be entirely undesirable for a spectroscopic junction, as non-Ohmic junctions would prevent constant misfiring of the receptor in the absence of an appropriately bound ligand). (2) Contrary to laboratory IET spectroscopy, the biological case is likely to be many single apparatuses (unique ORs) each operating at a single difference frequency. Also, the interaction between the electron and dipole is very close in proximity, thereby preventing the application of the dipole approximation, forcing one to employ the exact form of the interaction. This leads to an IETS intensity , where and describe the displacement of the partial charge, , along a single coordinate, . This implies the sensitivity of the method to both partial charge and to displacement (from equilibrium position); it is for this reason that the effects of large atom isotopic replacement would be smaller than that of hydrogen, as hydrogen displacements within normal mode coordinates are typically much larger.
Within the receptor environment, an electron will likely originate from an electron donor site—either the metal cofactor or an electron rich amino acid sidechain—and travel through or near the bound ligand to an electron acceptor site's energy level. Within Turin's original proposal, no concern was given to electron spin; this is likely due to his nearly direct application (and thereby close mirroring) of standard IETS theory, where electrons would originate from the electron sea of the donor and traverse toward a vacant conduction band at the acceptor. Under this situation, spin is able to be treated as an ergodic property in the system, as it lacks a dance partner. That stated, there is little present understanding of the exact (hypothetical) origin or destination of the ballistic electron; that is, there is no observation that the metal undergoes oxidation and no evidence of a charged side-chain (both suggested scenarios require a direct excitation as either oxidation or a charge side-chain would lose energy through donating its electron). Complicating the problem even further, Turin's proposal is an activation method for the protein, not a method of describing the totality of the events determining perception. This is more noteworthy as Turin's theory cannot describe any perireceptor events, aspects of intermolecular interactions during the docking of the ligand, nor consider any degree of complexity in the olfactory coding.
Finally, the standard laboratory procedure typically requires the IET spectroscopic junction to be cooled to cryogenic or near-cryogenic temperatures to reduce the effects of thermal broadening as the rotational states couple with the vibrations, or vibrations coupling with electronic states, where one performs IETS to activate electronic states. Thermal broadening cannot be eliminated in the biological environment, as the homeostatic temperature of the body (or near epidermal temperature for nasal olfactory receptors) is much higher than cryogenic temperatures. Yet, within the confines of the orthosteric binding site the ability of the ligand to freely rotate or translate is severely hindered, likely narrowing the Gaussian profile contribution for these motions. Competing with the aforementioned reduction, thermally active vibronic coupling between the ligand and protein environment will remain, and will increase the overall number of degrees of freedom. These effects were considered in a recent theoretical work to be discussed later [49].
The adaptation of the standard metal-electrode model mechanism of an IETS to an olfactory receptor is ad hoc; yet within his works, Turin provides a number of tertiary supporting facts. Foremost, electron transfer and tunneling mechanisms are prevalent throughout biological systems [50–53], whose early models were hypothesized by Marcus [54] and Siddarth and Marcus [55]. Secondly, he hypothesizes that there should be a redox-ready metal near the activation site to donate the electron necessary to the process. The possibility of a metal cofactor playing an important role in either binding or activation at GPCRs and other non-GPCR chemokine receptors is largely substantiated. It is known that divalent cations such as Mg(II) and Zn(II) are vital for both binding and the formation of activated complexes for opiate, MC1, MC4, and CXCR4 receptors [56–60]. Na+ has been shown to allosterically modulate several GPCRs, including opiate and dopamine receptors via a specific binding site between helices 1, 2, and 7 [61]. Zn0 nanopartiles, specific to oxidation and size, have been shown to enhance olfaction in rodents and canines [62–66] and have been shown to inhibit certain rodent ORs through competitive binding [67]; circumstantially, it should be noted that large concentrations of zinc have been found in specific areas of the brain [68–71]. Ionic copper has been shown to be vital for the robust activation of thiol-detecting olfactory receptors [72–74]. Ethylene hormone receptors of the Arabidopsis genus require a copper cofactor and specific mercury complexes have been known to inhibit the olfaction of Heliothis virescens [75, 76]. Finally, an extensive number studies (especially in rodents) have shown both the presence and the importance of metals/metal ions in olfaction [77–81].
The prior two decades have provided several experimental works concerning Turin's proposed mechanism. The works have taken place in vacillating waves of pro and con stances, and have utilized—for purpose of experimentation—both human and insect test subjects. We will now delineate these studies, addressing each article in chronological order of publication and illuminating the article both within the contest of the conflicting theories.
The earliest experimental work evaluating Turin's theory was performed by Haffenden et al. [82]. Those authors obtained several highly pure, commercially available labeled benzaldehydes; three isotope-substituted variants, as well as the natural abundance compound. Panelists were assigned to complete a series of three forced judgement, duo-trio tests [83, 84]. Unadjusted P-values suggested that the 13C analogs were not discerned at statistically relevant rates, whereas the deuterated variant was. After further correcting the values for perceived sequence bias, it was found that after considering the 50% chance of guessing there existed a 47 ± 32% “actual true” detection rate of the deuterated compound, while neither 13C-labeled compound was discernable. These findings were in line with the initial suggestions made by Turin that the difference in vibration-mode displacement of the carbon atoms is too small to perhaps be perceived, whereas deuteration causes a more appreciable response. Haffenden et al. argue that their findings—coupled with infrared spectrum studies—indicate that the 3,000–2,500 cm−1 range could play host to one of the bitter-almond bands. Although their finds provide some support for this region playing host to the cause of the bitter-almond scent, it should be further noted that this spectral region is characteristic of such a plethora of functional groups (including the saturated C-H, S-H, N-H salt, aldehyde and carboxylic acid stretching bands) that it is an entirely non-descript descriptor; especially, as any structural aspect (whichever in the list) causing this band would become the primary correlation between odorant and odor.
A series of experiments were conducted to test several claims put forth by Keller and Vosshall [85]. The first test examined the claim of odor blending originating from Wright [38]. The claim of odor blending appears twice in Turin's original paper [41], most notably the addition of guaiacol and ethylbenzaldehyde to replicate a vanilla note through the addition of spectral features. Keller and Voshall determined that at either of the concentrations presented of a 1:1 mixture of guaiacol:benzaldehyde panelists discerned no additional vanilla odor when compared to a respective concentration of either individual component. This is sensible as even under Turin's proposal, this was the least feasible assertion: that two molecules—which clearly would not occupy the same receptor—adding their individual spectra together (in uncorrelated and unrelated environments) to generate a third “ghost” odor, and without any foreknowledge of the structural olfactory code. Secondly, within Turin's original paper, it was claimed that for aldehydes of chain length C8 through C12, aldehydes with an even number of carbons are perceived to be fruity (citrusy) whereas those with an odd number of carbons are found to be floral (or waxy). Pairs of aldehydes—from a selection of unbranched aldehydes spanning butanal to dodecanal (L.B. Voshall, personal communication April 16-18, 2017)—with the same parity of carbons did not present with more dissimilar odors than those with different parity; in fact, the difference in perceived odor increased generally with an increase in carbon chain length. It should be noted that several of the aldehyde molecules examined by Keller and Vosshall fall outside the range of aldehyde chain lengths discussed in Turin's initial claim and thus may very well bias the statistics concerning the C8-C10 range through out-of-scope sampling. Though, Turin himself likely determined the root cause of this odor difference, yet ascribed it to a IET-scattering mechanism rather than simple conformation space. It was found that the rotation of the aldehyde group was more hindered in the odd numbered chains; this fact would present any receptors able to encapsulate (in whole or par) the aldehyde to perceive a different free energy pathway toward the ground state of the complex, this additional hindrance to rotation (larger energy barrier to rotation) could negatively affect the receptor's ability to reach the active conformation. Finally, the claimed difference in odor between acetophenone and d6-acetophenone was examined; the human panelists employed perceived no difference in odor between the compounds when they were presented via a triangle test. Secondary testing of those who perceived the odor differences returned statically average findings.
To test the VTO, Franco et al. [86]. employed several strains of (Drosophila melanogaster, which is commonly used in biological research. Untrained Drosophila were examined and found able to both recognize and react (attraction/aversion) to various levels of deuterated ACP during a simple T-maze test [87], and the aversion response roughly scaled with the level of deuteration. These flies were also trained to react to specific levels of deuterated vs. natural abundance species through application of negative electronic stimulus in a similar T-maze. A generalization experiment was also performed showing that Drosophila trained to avoid 1-octanol vs. d17-1-octanol were able to generalize some characteristics and showed selective avoidance for ACP vs. d5-ACP, thereby suggesting that there is a salient odor feature provided by deuteration that is generalizable across molecules. A group of mutated ansomic flies no longer displayed untrained avoidance of either d8-ACP or d17-1-octanol. The evidence provided by the anosmic flies is chiefly that the flies discerned these compounds through olfaction. The scaling of aversion response and trainability could likely have been due to (larger degrees of deuteration requiring) larger concentrations of a reactant/contaminant within the samples, where anosmic flies would detect neither the analyte nor any contaminant present.
Gane et al. [88, 89] examined the VTO through two tests employing both trained and untrained human subjects. Furthermore, great effort was expended to address the concern of impurities; assuring GC-level purities of all odorants, even those purchased at high purity. In a forced-choice same-difference test 5 panelists (3 perfumers, 2 untrained) were presented with GC purified, stored samples of commercially available 99% ACP and d8-ACP. Agreement was reached with the findings of Keller and Vosshall [85]; explicitly, humans are not able to distinguish between these two isotopologues of ACP. The second test examined the human ability to discriminate deuterated variants of musk-class odorants, including: cyclopentadecanone, cyclopentadecanolide, 1,4-dioxa cyclohepta decane-5,17-dione, and 1-(3,5,5,6,8,8 -hexamethyl-6,7-dihydronaphthalen-2-yl) ethanone. This test was conceptualized by considering the larger ratio between carbons and hydrogens in the (typically saturated) musk compounds, and that this may generate a system where the possible isotopic characteristics may present the largest differences. Upon analysis of the data from 11 subjects, the p-values of the individual subjects ranged from 0.109 to 7.62 * 106; the statistical chance of the aggregate trials (119 correct identifications during the aggregated 132 total trials) to have occurred accidentally was found to be 5.9 * 10−23. In brief, deuterated musk odorants were determined discernible from their non-deuterated counterparts by the study subjects. Gane et al. attribute the musk odor perception to be symptomatic of groups responsible for a strong absorption within the 1,380–1,550 cm−1 IR region. Yet a broader examination of the IR and Raman absorptions of musks, including popular nitro-musks, structurally similar non-musks, and recently examined silio-musks (see [90]), show no clean relationship between musk odor and any single spectral feature.
Gronenberg et al. examined the ability of the western honey bee (Apis mellifera) to discern between isotopomers [91]. Bees were tested with three chromatographically pure compounds ACP, BNZ, and OCT against their fully deuterated counterparts. Although the learning curve response was flatter than expected by the authors, it was clear that the bees were able to discriminate between these two related molecules. Discrimination testing over the deuterated and un-deuterated pairs revealed that the bees were able to differentiate between the two isotopomers; this effect was truly independent of whether the deuterated or natural analog was the “trained” stimulus. The authors did find it noteworthy that the deuterated odorants tended to confuse the bees more than the h-BNZ vs. h-ACP test—possibly alluding to a greater degree of difficulty in isotope discrimination compared to chemical discrimination. This confusion could also be a product of unintended contamination of samples during the deuteration, minute amounts of solvent or reactant causing confusion as they present as accidentally introduced, untrained stimuli. A control experiment was also undertaken, generating mixtures (95:5) and pure samples of h-ACP and h-BZN. The addition of a minor component did not affect the bees' response compared to the pure training test. Gronenberg believes this shows that the bee's ability to respond to minor impurities within the samples is minimal. It should be noted that although the 95:5 mixture of h-ACP:h-BZN did not elicit a novel response, neither of the components were deuterated, and could have possibly lacked a systemic contaminant which elicited the trainable responses seen in the previous experiments.
Block et al. [92]—instead of providing another psychophysical experiment with either insects or humans—examined the theory at the receptor level [92]. Within the main paper, Block conducted three unique series of experiments: (i) Examination of receptor activation by isotopologues of Exaltone; (ii) Activation by isotopomers of acetopheonone and benzaldehyde; and (iii) Testing isotopomers of ten receptors and ten h/d ligand pairs. In the first series of experiments, a collection of 330 human ORs were screened with h-cyclopentadecanone (Exaltone, a musk odorant) and four deuterated variants (d4, d24, and d28). A single OR, OR5AN1, was found to be a “bona fide” receptor for this musk. They reported no receptor responded to only one of the four compounds (a claim refuted by [93]), which would contest the possibility that different ORs respond to different isotopomers. EC50's and dose-response curves were found to be very similar for each of the 4 isotope-specific compounds. Secondly, Block and colleges tested acetophenone and benzaldehyde at various mouse ORs, and repeated this with isotopologues of these compounds. No significant differences were found between the activations of the receptors by the isotope-specific ligands. Finally, several ligands—most importantly MTMT and bis(methylthiomethyl) disulfide—were examined with their isotopomers. In none of the cases was a significant difference in activation found. MTMT and bis(methylthiomethyl) disulfide are known to require a copper cofactor in their overall activation scheme, most likely during binding; for this reason, Block et al. repeated the above series of tests while also introducing 30 μM of Cu+2. Introduction of copper—to provoke a differential response due to increased cofactor concentration—provided no results divergent from the original conclusion.
Acknowledged by Block et al., differentiation of a pair of isotopomers does not necessarily prove an IET-esque mechanism of OR activation; nor does a collection of negative results absolutely disprove such a theory. (I speak here of proper evidence of absence, not absence of evidence!) A possible reason that previous behavioral studies (explicitly [41, 82]) that determined various abilities at discerning isotopologues—whereas the present study did not—are those events that occur before the activation of the receptor itself, perireceptor events. Three pertinent perireceptor events for behavioral studies conducted above would be (i) Enzymatic activity within the nasal mucosa converting chemical species or exchanging hydrogen [94, 95]. (ii) The action of odorant binding proteins within the nasal mucosa in transporting specific species through the hydrophobic environment. (iii) The ability of the nasal mucosa itself to act as a separatory media through differential diffusion or solubility [96, 97]. Alteration of protium to deuterium has other important effects on systems resembling the prescient solvated ligand-receptor system, e.g., isotopic alteration changes the zero point energy of the bond and will have an effect on intermolecular forces, for H/D this effect is manifest in hydrogen bonds. A possible IET-esque mechanism would be extremely sensitive to both geometry and orientation. The geometry of the bound ligand will differ greater from the gas phase geometry, affecting the vibrational modes. Also, orientation has been described to be so important in IETS that it is likened to a selection rule, and electron tunneling is known to be a very local process. We do not know the true crystal structure of an OR, it's ligand-bound structure, nor the possible donor or acceptor sites, thus we cannot possibly predict the appropriate IETS of any such system. It should also be noted that Block reported that d4-muscone lacked activity in the IR region (1,380–1,550 cm−1) that Gane et al. [88] had claimed should have an “intense” absorption to realize a musk odorant. One should recall that any electron tunneling mechanism would be evident in both Raman and IR spectra [44, 47], therefore solely examining the IR spectra will not elucidate the totality of possible vibrational modes.
To better address the conflicting accounts of odorant isotopologues sensitivity, two independent works revealed that insects can differentiate isotopologues of odorants. First, Paoli et al. [98] examined the local activity of specific odorants at the glomeruli of the honey bee. Four odorants (1-octanol, benzaldehyde, acetophenone, and isoamyl acetate) and a single deuterated variant of each (d17, d5, d8, and d3, respectively) were examined at 19 Apis glomeruli. Initially calcium flux was used to determine the activity of the glomeruli, and that was followed up by employing two-photon functional microscopy to assist in visualizing the local activity on the topography of the sensory organ. It was noted that several of the glomeruli displayed differential flux responses with respect to exposure to isotopologues of an odorant. When regional activity of the glomeruli were examined it was found that—although there was differential detection of isotopes—the ability of Apis to differentiate isotopologues is smaller than the ability to differentiate unique chemical species. Drimyli et al. [99] examined the ability of drosophila to differentiate isotopologues. Both behavioral (T-maze) and electroantennography (EAG) tests were conducted; the behavior tests were performed to corroborate that any differences found in the EAG were salient and perceived by the insects themselves. EAG revealed a differential response between deuterated and non-deuterated ligands. With forethought to possible criticisms and non-VTO explanations, Drimyli et al. conducted a preemptory discussion concerning purity, perireceptor events, and the extent to which size or number of deuteriums may affect the EAG. As there was no variation in the EAG rise times (the time at which a ⅔'s maximal response was recorded [100]), Drimyli et al. argue that the effect of polarity difference between 1H and 2H has a minimal effect on the totality of perireceptor events [101], as well as the binding/activation steps. In a cross comparison of maximal EAG response between each of the odorants, prodeutero-analogs and several non-prodeuterated versions it was found that the differential response to any deuteration was similar; implying that the detection ability is less sensitive to the extent of deuteration than the difference between deuterated and non-deuterated, implying that the insect detects deuterium or it does not. That does suggest—thought not conclusively—that the extent of isotopic exchange is unimportant, in opposition to the commentaries: [93, 102]. This dichotomy of detection also supports the hypothesis that the effect is caused by the presence of impurities, as unique impurities may likely be present in deuterated samples compared to the natural abundance sample.
Voshall [103] made two poignant observations: (1) Generally, psychological experiments are both poorly reproducible and have many (both obvious and obfuscating) variables that complicate the interpretation of results, and (2) The unlikelihood that a molecular-level mechanism could be clearly discerned through perception-based, in vivo psychological studies on humans. Therefore, the finding of humanity's ability to discern isotopologues of musk compounds is important; yet, it does not prove that an IET-esque mechanism—or any activation mechanism—is dependent on vibrations. Human subject examination—such as the Gane [88] or Haffenden [82] studies—are entirely in vivo; for this reason the examiner is provided no ability to attribute this isotope-sensitivity to events that occur in the nasal mucosa [94], with odorant binding proteins [104–106], at the antechamber of the receptor [10], during docking [107], or during activation itself.
Block et al. [92] provided an extensive discussion over their concerns about impurities in previous works, and various isotope effects within biology and the nasal passage. In a reexamination of an early experimental work, Turin determined that the ability of D. melanogaster to discriminate between acetophenone and d5-acetophenone was very likely due to minute impurities within the commercially “pure” isotopologue samples; this finding does not invalidate the VTO, yet does remove one of the most important experimental findings in support of the theory, as well as calling into question several other studies where GC-levels of purity were not observed [108]. As an example, throughout Franco's experiments the deuterated compounds used were commercially available at highly pure levels (~99%); this was done in reaction to one of the many counter-points to a previous experimental evaluation of the VTO. Though, commercially pure extremely minute concentrations of any impurity involved in the synthesis of either isotopomer—which could not be removed through the performed GC purification—would be present. In the light provided by the complexity provided by olfactory coding, the spatial mapping of olfactory lobes, the building of olfaction spaces and the developing picture of dynamic protein activation (coupling dynamics with VDW/ΔG/volume sensitive receptor and low reorganization energies), the question pertaining to the VTO which must be immediately addressed is: Is it needed?
Before the present iteration of the VTO gained attention, Quantitative Structure-Activity Relations (QSARs) were developed to determine novel drug molecules from known batches of molecules and their known experimental activities by developing statistical relationships and machine learning algorithms to find correlation between particular protein activations by ligands and specific characteristics of those ligands. A particular descriptor used within QSAR is the EigenVAlue (EVA), developed at Shell Research Ltd., [109, 110] which attempts to correlate activities to vibrational eigenvalues as opposed to characteristics that more logically allude to binding (e.g., size, polarity, functional groups, hydrogen bond forming groups, etc.). An early implementation of the EVA descriptor to predict odor relationships was performed by Takane and Mitchell [111]. Within this work, 47 structurally diverse molecules were sampled and dendrograms were formed by considering the region of the IR spectra where each molecule possessed vibrational modes. They found that the EVA descriptor was an excellent zero-order approximation, and determined into which of 7 categories (ambergris, bitter almond, camphoraceous, jasmine, rose, mugeut, and musk) an odorant fits. The authors of this work note that although the vibrational frequencies of the molecules naturally placed the odorants into their appropriate categories, this was merely likely due to the vibrations reflecting structural aspects of the molecules; making the EVA a “once-removed” structural analysis tool. This conclusion is in direct relation to why Wright's theory originally failed (and the clear problem with the Haffenden et al. experimental conclusions); a common cause likely exists between the particularities of odorant perception and the existence of particular absorption bands, yet that does not mean the bands cause the perception.
The first attempt at building a model to determine the viability of Turin's VTO re-constructed a Marcus-type expression for the time-scales of electron transfer in a biological environment and determined parameter ranges reasonable to physiological conditions [112]. Within the context of the Marcus theory formulation, a Coulombic interaction was introduced between the tunneling electron and an introduced intermediate set of oscillating partial charges representing the odorant molecule. Important biological factors determined by Brookes et al. include: the Huang-Rhys factors, the importance of limiting D → A electron transfers in the absence of an odorant (likely due to either distance between D and A or a non-Ohmic junction), and the possibility that the electron/hole source may likely be a biological oxidizing agent. It was determined that the charge transfer rate was adequately feasible for use in neurological detection and signaling. Additionally, bounds for a reasonable reorganization energy were formulated by considering a hydrated system to display an upper bound (~1eV) and resonance condition to define the lower bound. They determined that if the reorganization energy was only slightly larger than kBT a large signal to noise ratio was obtainable. Brookes et al. conclude that the overall non-mechanical mechanism suggested by Turin was feasible within the system, and incorporate it as a possible mechanism in a general Swipe Card model of olfaction (where selective docking plays a role, but activation of the OR protein is based on a non-mechanical actuation) [113].
The question as to the relative rates between the elastic and inelastic processes with respect to both the donor-acceptor energy difference and the reorganization energies associated with real molecules was addressed by Solovyov [114]. They devised a model Hamiltonian coupling the olfactory receptor and odorant via a harmonic approximation, simulated an environment through a collection of harmonic oscillators and a tunneling term built around the hopping integral. The odorant molecule was optimized and vibrational frequencies and IR activities were determined through a B3LYP-DFT approach. They approximate the electric field of the receptor to be equal to a Zn+2 dication at a distance of 15.4Å, as histidine and zinc ions have been previously discussed to be a probable acceptor/donor pair [41]. Several protio and dueterio variants of acetophenone, citronellyl nitrile, and octanol were examined; it was determined that energy regions of highly IR active vibrations were also parametric regions where there were large enhancements to the inelastic/elastic tunneling ratio; additionally, some less active modes were able to make contributions. Additionally, Solov'yov et al. propose a scenario by which deuterated analogs of similarly-sized (or functionalized) molecules may accidentally activate novel (non-endogenous, non-intended) receptors. The authors conclude that it is possible to determine molecules based on tunneling rate ratio enhancement because they correspond to characteristic vibrations under physiological conditions (as the analogous non-physiological statement has generally been known for decades).
Turin then provides several insights as to the molecular and physiological factors concerning his theory, and revises the underlying theory [115]. The insights concerning his theory are threefold: (1) The discriminatory factor of ORs on odorants cannot be merely electronic as isotopomers are detected (even only sometimes) as distinct odors. (2) The basis for discrimination cannot be mass alone, as a fully deuterated acetophenone differs only 3% from the protio isotopomer. (3) Under physiological conditions, the C-H/C-D modes do not drastically alter the heat capacity. A revision of the VTO is undertaken as it is argued that the standard IET spectroscopy theory is not valid because all orbitals within the biophysical system are localized; this lack of direct overlap requires some slight alteration in the theory. A new simulation model is developed based on a donor-bridge-acceptor model Hamiltonian. This new approach predicts the probable discrimination between acetophenone and d8-acetophenone, while predicting that deuterated isotopomers of ethylene cannot be discriminated. The paper continues by presenting the results of a T-maze experiment testing and validating the predicted hypothesis concerning acetophenone and d8-acetophenone, yet no evidence was provided to support the claims concerning ethylene.
A spin-boson model was developed to describe the importance of dissipation in electron transfer processes under a polar master equation by Chȩcińska [116]. Within the model, the environment is again described by a collection of harmonic oscillators, the physical parameters being determined by physiological arguments similar to previous works [112, 114], and the odorant molecule is modeled as a single oscillator in an expandable manner. They determined that under resonant conditions reverse transfer (A→D) is a likely event without preventative mediation from the surrounding protein environment, likely in the form of reorganization. They also found that reduced dissipation limits the frequency detection and discrimination of the system. Operating under a similar model, Tirandaz et al. [117] examined the system's ability to discriminate enantiomers based on an asymmetric double well potential. They found that such a system was able to discriminate enantiomers based on the ratio of the tunneling frequency to the localization frequency, used as an overall measure of chiral interactions.
Block et al. [92] had previously made three claims to be answered by those supporting the VTO. These claims are: (1) The suggested mechanism by which electrons are delivered to the system is too unreliable to support such a vital system as olfaction. (2) Electron transfer mechanisms are highly sensitive to both bonding and average environmental fields that are not adequately considered in present models. (3) Assumptions concerning environmental fluctuations are not realistic. To answer claims 2 and 3, Solov'yov et al. determined acetophenone to be a viable odorant molecule to examine, selected based on experimental utility and docking into a homology model of an OR based on rhodopsin apoprotein [49]. The vibrational properties of the odorant were calculated under the influence of the OR while the newly perturbed Huang-Rhys factors were determined. Finally, alterations in binding due to post-ET reorganization are considered and comments on this are provided. Within the homology model, particular candidate amino acid residues—favorable to the binding of acetophenone—were selected as putative donor and acceptor sites; interestingly, methionine was selected as a possibly active residue as it was previously suggested to be involved in electron relays [118]. It was determined that the electron transfer has only a minor impact on the potential energy of the OR, and that the changes in vibrational character of the odorant will likely not affect electron transfer in the system. Furthermore, chemical fluctuations (including proton and methyl radical transfers) and dynamic fluctuations were considered and shown to have no appreciable impact on the average IR spectra of the odorant. Finally, it was reiterated that the reorganization energies of membrane proteins can be so low as to be negligible, e.g., a reorganization energy of 0.03 eV is observed for the photosynthetic protein of Rhodobacter capulatus at room temperature [119].
Apart from directly modeling the electrostatics and dynamics of the system, there have been several studies attempting to divine other testable aspects of the odorant-receptor system to validate/invalidate the VTO. A possible test for the VTO was designed based on the use of a 13C isotope instead of the more commonly employed 2H isotope [120]. Therein, it was suggested that committing a number of 12C→ 13C exchanges to match the mass shift in a deuterated isotopomer of the same molecule you are capable of generating odorants with (roughly) the same molecule, differing vibrational spectra, and roughly the same Gibb's free energy. That allows one to probe the on/off rate of—let's say 13C8-acetophenone and d8-acetophenone—and determine whether any change in this quantity is due to a free energy difference or possibly due to a difference in vibrational spectra. It should be here noted that no perceived effect was seen in the behavioral study by Haffenden et al. that included 13C isotopic exchange, possibly giving credence to the VTO within the context of this test [82]. Additionally, Maia et al. completed MM/MD calculations to obtain geometries of 23 molecules (16 musks and the remainder being structurally similar to the musks, yet without musk odor character) and employed B3LYP-DFT calculations to obtain the vibrational spectra. Considering central frequency bands centered at intense peaks ±200 cm−1 at 700 cm−1, 1,000 cm−1, 1,500 cm−1 and 1,750 cm−1; it was determined that no single frequency or band could be responsible for the activation of a musk receptor (otherwise musks would not activate or non-musks would activate). The response was likely—if at all related—due to a collection of modes sitting roughly between 700 and 1,700 cm−1; the authors further disputed Turin's assessment that a carbonyl stretch at 2,000–2,400 cm−1 was responsible for the musk odor because this band appears at 1,680–1,810 cm−1 in the present work and is not present in several of the studied musk odorants.
Saberi and Seyed-allaei [121] examined the molecular volume (considering both size and flexibility of the binding pocket, characteristics featured in other works, such as [122, 123]) and attempted to correlate it to olfactory sensitivity for the olfactory receptors of D. melanogaster. They cite neural measurements that allude to the use of a combinatorial coding in olfaction, yet wished to determine the vital discriminatory properties of the olfactory system. They, logically, assumed that any mismatch in the volume/receptor relationship will affect the physiological response. An initial assumption was that the total activity was a separable function of volume effects and all remaining considerations; where molecular volumes are determined by a Gaussian distribution built on tuning curves constructed by neural responses of individual neurons. It was thought that if all receptors had the same preference for molecular volume/flexibility, then changing the volume of an odorant would affect the intensity of odor and not the character. Molecular volume was found to be a factor, but not the sole one involved in olfactory discrimination; yet there did seem to be a non-linear volume dependence that may be capable of masking other factors involved in olfaction. This study was explicitly concerned with D. melanogaster, yet could be repeated for other species.
Finally, it is prescient to consider the more overt ways by which isotopic exchange may affect enzymatic processes. Krzan et al. [107] examined the effects of 2H isotope exchange via saturation in deuterated media. They—of course—found contributions to the enzymatic rates and binding affinities due to the Ubbelohde effect as 1H→ 2H exchange within the protein effects intramolecular bonds and thereby alters the energy balance between the active and inactive states of the protein. This is—the authors claim—the first attempt at examining isotopic effects within the context of the protein's atoms; yet there are decades of work examining the effects of isotopic specific endogenous and synthetic ligands. Within these previous studies the binding isotope effect, alterations in zero-point energy, and changes in vibrations effecting hydrogen bonding have all been discussed [124–126].
Recently, Tirandaz et al. [127] reexamined their chiral-odorant polaron Hamiltonian model—expanding it to determine a non-planar vibrational state—and determined several aspects of the model that could provide new tests of the vibrational theory, while also displaying the versatility of a system behaving within the model. Therein they constructed a rate expression describing the IET frequency based on an odorant capable of undergoing a tunneling interconversion between two chiral states, similar to the classical ammonia tunneling problem. Using this model, the authors determined a lower thermal limit to the possible detection of an odorant within the VTO context, and limiting relationships and trends with respect to odorant concentration (thermodynamic pressure) and isotopic mass substitutions. Importantly, they determined that the substitution of higher isotopic masses increases the system's sensitivity under the VTO. Finally, though very narrowly, they find that the VTO is capable of chiral recognition under biological conditions. In a series of papers, Kraft et al. examined—through both in vivo calcium ion imagining and computational methods—the validity of the “shape-based” theory of olfaction by examining the response to several Group 14-for-carbon substitution of the t-butyl functional group in the lilal and Bourgeonal odorants [128–130]. As these authors state, the benefit of this substitution series is that it maintains the molecular geometry while increasing the hydrophobic bulk of the molecule. Within the series of papers, their focus was to examine the molecular mechanism responsible for odor detection and perception through a three pronged approach: (1) conduct in vivo detection threshold experiments, (2) in vitro calcium ion imaging of activation, and (3) computational support. They determined that the human OR17-4 receptor was activated—and likely solely activated—by the odorants above through both structure-odor-relationships and in vivo analysis. Afterward, a homology model was constructed for the receptor based on the rhodopsin protein and docking simulations of the various substituted odorants were made, noting their change in free energy. They then compared the requirements for breaking of the disulfide bond, which binds the intracellular G-protein to the transmembrane GPCR. They take that the energy required to directly break the disulfide bond to be a bound for the energy harvested from the vibrational quantum; using this bound they find that the energy ranges defined by these criteria do not match the activation patterns seen in their (both in vivo and in vintro) works. The free energy changes seen in the docking calculations with respect to the increasing hydrophobic bulk do match those results. It was finally determined that olfaction was largely based on van der Waals (VDW) surface and electrostatic surface properties, finding no evidence that the VTO played any role in olfaction [130].
Dating back to the unicellular stages of evolution, chemoreception was the earliest form of communication between cells and their environments (including other cells) [131]. Similar chemoreception systems solve similar problems between those early unicellular life forms and the individual cells of today's multicellular life. It should not be surprising that many metazoan signaling systems are constructed from components already found in their unicellular ancestors [132], while other systems were coopted entirely from pre-existent systems [133–135]. Note that in the case of GPCRs, the unicellular precursors of metazoans already possessed most of the cytoplasmic components of GPCRs, including all of the Gα subunits. Nature seemed to grab the right (or closest-to-right) tool for a job and set it to a new task.
One of these novel receptor classes developed by metazoans was the GPCR system, where most GPCRs families have their ancient origins in the last common eukaryotic ancestor [136]. Generally speaking, GPCRs' transmembrane signaling proteins are comprised of seven helical domains crossing from the extra- to the intra- cellular environments. Each receptor possesses a ligand binding pocket near the extraceullular surface and an intracellular heterotrimeric G-protein complex, which dissociates after the GPCR is activated. Members of the family mediate extracellular chemical signals and share homologous structure [137]. Speaking generally once more, GPCRs are divided into 5 major categories by the most used classification system: Glutamate, Rhodopsin, Adhesion, Secretin, and Frizzled. Although this system is designed specifically for GPCRs, it has been suggested to extend a similar system to include non-GPCRs, including insect ORs [138].
GPCRs populate batteries of receptors in the mammalian olfactory system (including: ORs, vomeronasal receptors, olfactory TAAR, Formyl peptide receptors, GC-D receptors) and neurotransmitter receptors (NRs) in many species, e.g., vertebrates and terrestrial varieties of both Nemertea and Annelida, use GPCRs as ORs [139, 140]. At a fundamental system level these jobs are similar, as both accept a chemical species traveling through space to interact with a binding protein whose signal is eventually detected by an ionotropic receptor (IR) after having undergone several intermediate processes [141]. NRs typically have lower sensitivity (nano- to mili- molar range) and deal with a smaller, controlled number of chemical compounds, many of which are highly specific ligand/receptor relationships [141]. By contrast, in olfaction, individual odorants activate subsets of receptors instead of single receptors and activation/inhibition/antagonism all play a role and possibly generate temporal dynamics that are important to the overall odorant code and possibly novel odorant perceptions [142]. Slight changes in either structure or concentration of an odorant are capable of affecting the activation pattern, leading to a different odorant code [139]. This odorant code permits mammals, including humans, to perceive a larger variety of odors than ORs expressed, as humans are capable of perceiving over 10,000 odorants with a trillion being an extremely liberal estimate under contention [131, 143–145].
The architecture of terrestrial vertebrate olfactory systems is fairly conserved. Air is up-taken through a stereo arrangement of nostrils, an arrangement important to odor tracking. This air, laden with odorant molecules, passes along the epidermis of the nasal cavity where receptor lined neurons project into the cavity; each olfactory receptor neuron (ORN) expresses one receptor type [139]. Within this span of receptors and neurons, some pairs are considered generalists (those that are typically involved in the activation of odors) and some pairs are specialists (those involved in the detection of pheromones) [139]. After the receptor is activated, it's neuron conducts the signal to a glomerulus, an organ that acts as a encoding pre-process site before the signal reaches the olfactory lobe of the brain [146–148].
Similar to the terrestrial vertebrate system, the overall system-level olfactory procedure is well conserved in insects. Air passes through a porous cuticular wall—typically along the antennae—where the sensillium contains dendrites for ~5 ORNs. The axons of these ORNs project into glomeruli within the antennal lobes of the insect brain. This physical pathway is utilized by neurons transferring mechanosensory, thermosensory, hygrosensory, and gustatory information as well [141, 149]. The zonal organization of the glomeruli is not precisely understood [149], but important electrophysiological studies are being done to understand these patterns and to build representations of odorant spaces [4, 98, 99, 150]. Importantly, these glomeruli patterns show similarity between individuals within a species [151]. Generally, different ORNs respond to different odors, and these responses are varied in type (excitatory or inhibitory) and dynamics [149]. The importance of this glomeruli pattern-based code is supported by cisgenic monoexpression of an OR in its non-wildtype neuron, which changes the overall innate behavioral response to an odorant [152].
Insect olfaction utilizes three types of chemosensory protein (CSP) receptors: insect ORs, IRs, and gustatory receptors (GRs) [139, 141]. An insect OR is a transmembrane protein of roughly 44 kilodaltons showing no homology to GPCRs, yet having similar topologies and functionalities. Of note, insect ORs possess inverted topology to GPCRs, as they have an extracellular C-terminus and an intracellular N-terminus [153, 154]. It is generally suggested that insect ORs act as ligand-gated ion channels [155], yet may also—through odorant sensing complexes—activate a G-protein pathway [156]. This dual channel mechanism is capable of providing both a rapid (ionotropic) and sensitive (metabotropic) detector [156]. This dual channel construction comes from a heteromultimer composition of one ligand-specific receptor acting as a ligand-gated ion channel coupled with a (highly conserved) ORco-type receptor [141, 157]. Whereas most ORs vary widely in sequence [158, 159], the ORco receptors show good sequence identity; these coreceptors have been identified in Lepidoptera, Diptera, Coleopteran, Hymenoptera, Hemipteran and Orthopteran [154, 157].
Insects typically express two types of olfactory protein on a single ORN (or sometimes four IRs, see [139]); this is different from their vertebrate counterparts. It is vital to note that the secondary protein expressed on the ORN is typically the ORco receptor for that species; this is to say, an ORN contains a single OR and the species' ORco [151, 158]. As ORs very widely in their sequence identity—suggesting that species developed their present repertoire of ORs individually—the ORco receptors show amazing similarity across species [151, 158]; a study of several species have shown a 65-87% sequence similarity for their ORco analogs, including: D. Melanogaster, A. Gambaie, H. Zea, and C. Captitata [158]. It has been thought that the expression of multiple olfactory proteins per ORN was an adaptation to expand the number of odorants perceived while maintaining a low number of neurons and ORs [151]; it is more likely that the glomeruli pattern holds the sensory diversity, and the OR-ORco pairing is designed to exploit multiple signaling pathways. That is evidenced by the fact that OR83b (the ORco analog of D. Melanogaster) is not directly involved in odorant recognition [139]. Suppression of the expression of the ORco analog has been shown to deplete the overall activity of the olfactory system [158, 160]. Additionally, these ORco analogs are capable of fulfilling the role of their interspecies counterparts; an anosmic mutant D. Melanogaster—without expressed OR83b—regains its electrophysiological response to odorant stimuli when transgenic Or83b orthologs were inserted and expressed in the fly's ORN [158].
Some studies have attempted to relate the vibrational theory to GCPRs within the CNS. Oh et al. [161] have completed studies attempting to find relationships between the molecular vibrations and activity of compounds at the histamine and adenosine receptors. A selection of several agonists for the candidate receptor were optimized at the BLYP-DFT level of theory and their molecular vibration frequencies were calculated. Analysis within a Corralled Intensity of Molecular Vibrational Frequency (CIMVF) framework suggested that the 0–5,000 cm−1 energy range of molecular vibrations is divided into 1,000 partitions of 5 cm−1, and the value of each partition is the sum of the intensities within the partition. Upon completing a hierarchical clustering of the CIMFV data, it was determined that this method above others was capable of clustering the agonists of both the histidine and adenosine receptors within a tree. Dendrographic analysis of ligand activity correlated with vibrational frequencies [161–163]. Oh does not attribute that directly to the mechanism by which these CNS GPCRs are activated, yet describes it as a possible useful tool in divining novel ligands for these receptors.
In a similar vein to the discussion of Barwich [17], Hoehn et al. attempted to expand Turin's IET-based VTO by applying it to predict possible effects of deuterated ligands for non-olfactory GPCRs in the CNS [164]. They initially examined several well-known psychedelic 5-HT receptor agonists and algorithmically determined shared spectral features that could be responsible for their collective activation properties through a spectral indexing method. This putative active peak was similar in frequency to those previously reported for the histamine and adenosine receptors [161–163]; that is not entirely unreasonable as a steric/docking consideration providing additional discriminatory facilities to the receptor, as it is known that receptors interact with several ligands and a ligand is capable of interacting with several receptors [165]. After ascertaining a putative peak, their focus shifted to validation by comparing the peak volume—proportional to the tunneling probability associated with this vibrational mode—to the activities of known ligands at the receptors. The selected ligands were DOX and 2C-X (X = Br, I, trifluoromethyl, and an ethyl silyl ether). The tunneling probability did roughly scale with the efficacies of the selected substances. Based on these findings, the relative efficacies of several deuterated analogs of the LSD molecule were predicted and proposed as a test of the vibrational theory for general GPCRs. In a follow-up paper, the authors performed the prescribed test on DAM-57, a less legally controlled variant of the LSD molecule [166]. The findings conclusively showed that the predicted pattern of efficacy for isotopologues of DAM-57 did not match the experimental findings. Thus the VTO is unlikely to participate in non-olfactory GPCRs; and as the lineage of GPCR maintains many similarities in both form and function, it makes it unlikely that the VTO participates in olfaction, as well.
A great deal of recent work has been directed toward determining the nature and the cipher of the odorant olfactory code [167, 168]. Olfactory lobe maps have begun to be made for both insect [169–172] and vertebrate species [146, 147, 173, 174]. The coding has proven to be sufficiently complex as to have evaded deciphering and provides an ample enough vector space to encode all perceivable odors [143, 144, 175]. It was suggested, though likely hyperbolic, that the total number of individual odors perceivable by the human olfactory system was upwards of 1 trillion [143], but that has been contested [144, 145]. The dimensionality of the odorant space has been examined to determine the minimal number of relevant olfactory classes for characterization [145, 176, 177]. Recent evidence has also shown—through inserted electrical probes in live mice—that the encoding of the olfactory lobe appears in the piriform cortex and posterolateral cortical amygdala, brain regions responsible for olfactory learning and innate behavioral response to odorants [178]. Interestingly, it was recently determined through exploring the perception of multi-component odorant mixtures that these mixtures are better understood as unique vectors within odor space than as averages between the two component odorant vectors [168]; this possibly explains early claims of odorant mixing in the vibrational theories of Wright and Turin [38, 41, 85]. As the complexity inherent in understanding structure-odor relationships led to the VTO, it should be noted that the unexplored complexity associated with olfactory coding may justify the lack of a simple structure-odor relationship and remove the impetus behind the VTO.
The topic of artificial olfaction is growing and has been adopting the more useful aspects of the discussion of organic olfaction, such as coding, metalloprotein-based detection and IETS [142, 179–182]. It is worth stating that even if all of this work does nothing to describe the biophysical and physiological process of olfaction, we are inadvertently proposing novel methods and tools for the development of artificial olfaction. Herein alone, electron transfer mechanics have been shown to be a possible means of artificial olfaction, while multiple sensors can provide a level of detection sensitivity and versatility that single detectors cannot. In general, many articles pertaining to the VTO have appeared within highly ranked journals; it is apparent that this conflict in vision—a conflict that neither side may win in the end—is one that the greater community would like to see resolved. Such a resolution will require far better communication between disciplines and a far more fair discussion between the entrenched camps on both sides of the debate.
All authors conducted background research for this article. RH completed most of the writing, while other authors heavily edited the text.
The authors declare that the research was conducted in the absence of any commercial or financial relationships that could be construed as a potential conflict of interest.
The authors would like to thank Dr. Shuhao Yeh both for constructive discussions and for his critical readings of the contiguously evolving drafts of this review.
1. Buck L, Axel R. A novel multigene family may encode odorant receptors: a molecular basis for odor recognition. Cell (1991) 65:175–87. doi: 10.1016/0092-8674(91)90418-X
2. Clyne PJ, Warr CG, Freeman MR, Lessing D, Kim J, Carlson JR. A novel family of divergent seven-transmembrane proteins: candidate odorant receptors in Drosophila. Neuron (1999) 22:327–38. doi: 10.1016/S0896-6273(00)81093-4
3. Gao Q, Chess A. Identification of candidate drosophila olfactory receptors from genomic DNA sequence. Genomics (1999) 60:31–39. doi: 10.1006/geno.1999.5894
4. Vosshall LB, Amrein H, Morozov PS, Rzhetsky A, Axel R. A spatial map of olfactory receptor expression in the Drosophila antenna. Cell (1999) 96:725–36. doi: 10.1016/S0092-8674(00)80582-6
5. Rosenbaum DM, Cherezov V, Hanson MA, Rasmussen SGF, Thian FS, Kobilka TS, et al. GPCR engineering yields high-resolution structural insights into 2 adrenergic receptor function. Science (2007) 318:1266–73. doi: 10.1126/science.1150609
6. Manglik A, Kim TH, Masureel M, Altenbach C, Yang Z, Hilger D, et al. Structural insights into the dynamic process of β2-adrenergic receptor signaling. Cell (2015) 161:1101–11. doi: 10.1016/j.cell.2015.04.043
7. Sounier R, Mas C, Steyaert J, Laeremans T, Manglik A, Huang W, et al. Propagation of conformational changes during Mu-opioid receptor activation. Nature (2015) 524:375–8. doi: 10.1038/nature14680
8. Huang W, Manglik A, Venkatakrishnan AJ, Laeremans T, Feinberg EN, Sanborn AL, et al. Structural insights into Mu-opioid receptor activation. Nature (2015) 524:315–21. doi: 10.1038/nature14886
9. Nygaard R, Zou Y, Dror RO, Mildorf TJ, Arlow DH, Manglik A, et al. The dynamic process of β2-adrenergic receptor activation. Cell (2013) 152:532–42. doi: 10.1016/j.cell.2013.01.008
10. Dror RO, Pan AC, Arlow DH, Borhani DW, Maragakis P, Shan Y, et al. Pathway and mechanism of drug binding to G-protein-coupled receptors. Proc Natal Acad Sci USA (2011) 108:13118–23. doi: 10.1073/pnas.1104614108
11. Davies PCW. Does quantum mechanics play a non-trivial role in life? Biosystems (2004) 78:69–79. doi: 10.1016/j.biosystems.2004.07.001
12. Lloyd S. Quantum coherence in biological systems. J Phys. (2011) 302:012037. doi: 10.1088/1742-6596/302/1/012037
13. Fleming GR, Scholes GD, Cheng YC. Quantum effects in biology. Proc Chem. (2011) 3:38–57. doi: 10.1016/j.proche.2011.08.011
14. Lambert N, Chen YN, Cheng YC, Li CM, Chen GY, Nori F. Quantum biology. Nat Phys. (2013) 9:10–8. doi: 10.1038/nphys2474
15. Brookes JC. Quantum effects in biology: golden rule in enzymes, olfaction, photosynthesis and magnetodetection. Proc R Soc Lond A Math Phys Eng Sci. (2017) 473:20160822. doi: 10.1098/rspa.2016.0822
16. Wiseman H, Eisert J. Nontrivial Quantum Effects in Biology: A Skeptical Physicists' View. 1st Edn. Singapore : World Scientific Publishing CO. (2012).
17. Barwich AS. What is so special about smell? Olfaction as a model system in neurobiology. Postgrad Med J. (2015) 92:27–33. doi: 10.1136/postgradmedj-2015-133249
19. Malcolm Dyson G. The scientific basis of odour. J Soc Chem Indust. (1938) 57:647–51. doi: 10.1002/jctb.5000572802
20. Wright RH. Odour and molecular vibration. I. Quantum and thermodynamic considerations. J Appl Chem. (1954) 4:611–5. doi: 10.1002/jctb.5010041104
21. Wright RH, Serenius RSE. Odour and molecular vibration. II. Raman spectra of substances with the nitrobenzene odour. J Appl Chem. (1954) 4:615–21. doi: 10.1002/jctb.5010041105
22. Amoore JE. Stereochemical specificities of human olfactory receptors. Perfum Essent Oil Rec. (1952) 43:321–3.
24. Amoore JE. Current status of the steric theory of odor. Ann NY Acad Sci. (1964) 116:457–76. doi: 10.1111/j.1749-6632.1964.tb45075.x
25. Moncrieff RW. The characterization of odours. J Physiol. (1954) 125:453–65. doi: 10.1113/jphysiol.1954.sp005172
26. Moncrieff RW. The odorants. Ann NY Acad Sci. (1954) 58:73–82. doi: 10.1111/j.1749-6632.1954.tb54846.x
27. Wright RH, Michels KM. Evaluation of far infrared relations to odor by a standards similarity method*. Ann NY Acad Sci. (1964) 116:535–51. doi: 10.1111/j.1749-6632.1964.tb45083.x
28. Doolittle RE, Beroza M, Keiser I, Schneider EL. Deuteration of the melon fly attractant, cue-lure, and its effect on olfactory response and infra-red absorption. J Insect Physiol. (1968) 14:1697–712. doi: 10.1016/0022-1910(68)90202-3
29. Blum MS, Doolittle RE, Beroza M. Alarm pheromones: utilization in evaluation of olfactory theories. J Insect Physiol. (1971) 17:2351–2361. doi: 10.1016/0022-1910(71)90083-7
30. Barker RJ, Berdel RL, Waller GD. The molecular basis for scent discrimination: response to nitrobenzene-d5 of honey bees (Apis mellifera L.) conditioned with nitrobenzene. Experientia (1973) 29:418–9. doi: 10.1007/BF01926754
31. Wright RH. Odor and molecular vibration: neural coding of olfactory information. J Theor Biol. (1977) 64:473–502. doi: 10.1016/0022-5193(77)90283-1
32. Sugawara R, Tominaga Y, Iida Y. A deuterium effect in insect attraction. Agricult Biol Chem. (1978) 42:1289–90.
33. Havens BR, Meloan CE. The application of deuterated sex pheromone mimics of the american cockroach (Periplaneta americana, L.), to the study of wright's vibrational theory of olfaction. In: Charalambous G, editor. Food Flavors: Generation, Analysis and Process InfluenceProceedings of the 8th International Flavor Conference. vol. 37 of Developments in Food Science. New York, NY : Elsevier (1995). p. 497–524.
34. Hara J. Olfactory discrimination between glycine and deuterated glycine by fish. Experientia (1977) 33:618–9. doi: 10.1007/BF01946534
35. Wright RH. The perception of odor intensity: physics or psychophysics? Chem Sens. (1978) 3:73–9. doi: 10.1093/chemse/3.1.73
36. Wright RH. Odor and molecular vibration: optical isomers. Chem Sens. (1978) 3:35–7. doi: 10.1093/chemse/3.1.35
37. Eriksson A, Lindner P, Mrtensson O. Molecular properties and odour. Effects of substituents on pyridine and pyridine odour. J Theor Biol. (1981) 90:477–86. doi: 10.1016/0022-5193(81)90300-3
38. Wright RH. Molecular vibration and odour blending. Chem Sens. (1983) 8:103–6. doi: 10.1093/chemse/8.1.103
39. Pfaffmann C. Taste and smell. Annu Rev Psychol. (1956) 7:391–408. doi: 10.1146/annurev.ps.07.020156.002135
40. Klopping HL. Olfactory theories and the odors of small molecules. J Agricult Food Chem. (1971) 19:999–1004. doi: 10.1021/jf60177a002
41. Turin L. A spectroscopic mechanism for primary olfactory reception. Chem Sens. (1996) 21:773–91. doi: 10.1093/chemse/21.6.773
42. Turin L. A method for the calculation of odor character from molecular structure. J Theor Biol. (2002) 216:367–85. doi: 10.1006/jtbi.2001.2504
43. Lambe J, Jaklevic RC. Molecular vibration spectra by inelastic electron tunneling. Phys Rev. (1968) 165:821–32. doi: 10.1103/PhysRev.165.821
44. Kirtley J, Scalapino DJ, Hansma PK. Theory of vibrational mode intensities in inelastic electron tunneling spectroscopy. Phys Rev B (1976) 14:3177–84. doi: 10.1103/PhysRevB.14.3177
45. Lambe J, McCarthy SL. Light emission from inelastic electron tunneling. Phys Rev Lett. (1976) 37:923–5. doi: 10.1103/PhysRevLett.37.923
46. Khanna SK, Lambe J. Inelastic electron tunneling spectroscopy. Science (1983) 220:1345–51. doi: 10.1126/science.220.4604.1345
47. Phillips WA, Adkins CJ. A theory for the intensities of inelastic electron-tunnelling spectra. Philos Mag B (1985) 52:739–50. doi: 10.1080/13642818508240633
48. Reed MA. Inelastic electron tunneling spectroscopy. Mater Today (2008) 11:46–50. doi: 10.1016/S1369-7021(08)70238-4
49. Reese A, List NH, Kongsted J, Solovyov IA. How far does a receptor influence vibrational properties of an odorant? PLoS ONE (2016) 11:e0152345. doi: 10.1371/journal.pone.0152345
50. Farid RS, Moser CC, Dutton PL. Electron transfer in proteins. Curr Opin Struct Biol. (1993) 3:225–33. doi: 10.1016/S0959-440X(05)80157-5
51. Ichiye T. Electron transfer through proteins: overview. In: Roberts GCK, editor Encyclopedia of Biophysics. Berlin; Heidelberg : Springer-Verlag (2013). p. 614–21.
52. Beratan DN, Skourtis SS. Electron transfer through proteins. In Roberts GCK, editor Encyclopedia of Biophysics. Berlin; Heidelberg : Springer-Verlag (2013). p. 625–30. doi: 10.1007/978-3-642-16712-6_13
53. Saen-Oon S, Lucas MF, Guallar V. Electron transfer in proteins: theory, applications and future perspectives. Phys Chem Chem Phys. (2013) 15:15271–85. doi: 10.1039/c3cp50484k
54. Marcus RA. Electron transfer reactions in chemistry: theory and experiment (nobel lecture). Angew Chem Int Edn English. (1993) 32:1111–21. doi: 10.1002/anie.199311113
55. Siddarth P, Marcus RA. Correlation between theory and experiment in electron-transfer reactions in proteins: electronic couplings in modified cytochrome c and myoglobin derivatives. J Phys Chem. (1993) 97:13078–82. doi: 10.1021/j100152a008
56. Ananthanarayanan VS, Kerman A. Role of metal ions in ligand receptor interaction: insights from structural studies. Mol Cell Endocrinol. (2006) 246:53–9. doi: 10.1016/j.mce.2005.11.023
57. Kinouchi K, Standifer KM, Pasternak GW. Modulation of μ1, 2, and opioid binding by divalent cations. Biochem Pharmacol. (1990) 40:382–4. doi: 10.1016/0006-2952(90)90704-O
58. Standifer KM, Clark JA, Pasternak GW. Modulation of Mu1 opioid binding by magnesium: evidence for multiple receptor conformations. J Pharmacol Exp Therapeut. (1993) 266:106–13.
59. Holst B, Elling CE, Schwartz TW. Metal ion-mediated agonism and agonist enhancement in melanocortin MC1 and MC4 receptors. J Biol Chem. (2002) 277:47662–670. doi: 10.1074/jbc.M202103200
60. Gerlach LO, Jakobsen JS, Jensen KP, Rosenkilde MR, Skerlj RT, Ryde U, et al. Metal ion enhanced binding of AMD3100 to Asp262 in the CXCR4 receptor. Biochemistry (2003) 42:710–7. doi: 10.1021/bi0264770
61. Wang S, Wacker D, Levit A, Che T, Betz RM, McCorvy JD, et al. D4 dopamine receptor high-resolution structures enable the discovery of selective agonists. Science (2017) 358:381–6. doi: 10.1126/science.aan5468
62. Viswaprakash N, Dennis JC, Globa L, Pustovyy O, Josephson EM, Kanju P, et al. Enhancement of odorant-induced responses in olfactory receptor neurons by Zinc nanoparticles. Chem Sens. (2009) 34:547. doi: 10.1093/chemse/bjp031
63. Vodyanoy V. Zinc nanoparticles interact with olfactory receptor neurons. BioMetals (2010) 23:1097. doi: 10.1007/s10534-010-9355-8
64. Moore CH, Pustovyy O, Dennis JC, Moore T, Morrison EE, Vodyanoy VJ. Olfactory responses to explosives associated odorants are enhanced by zinc nanoparticles. Talanta (2012) 88:730–3. doi: 10.1016/j.talanta.2011.11.024
65. Hagerty S, Daniels Y, Singletary M, Pustovyy O, Globa L, MacCrehan WA, et al. After oxidation, zinc nanoparticles lose their ability to enhance responses to odorants. BioMetals (2016) 29:1005–18. doi: 10.1007/s10534-016-9972-y
66. Jia H, Pustovyy OM, Wang Y, Waggoner P, Beyers RJ, Schumacher J, et al. Enhancement of odor-induced activity in the canine brain by zinc nanoparticles: a functional MRI study in fully unrestrained conscious dogs. Chem Sens. (2016) 41:53. doi: 10.1093/chemse/bjv054
67. Seebungkert B, Lynch JW. A common inhibitory binding site for zinc and odorants at the voltage-gated K+ channel of rat olfactory receptor neurons. Eur J Neurosci. (2001) 14:353–62. doi: 10.1046/j.0953-816x.2001.01646.x
68. Horning MS, Trombley PQ. Zinc and copper influence excitability of rat olfactory bulb neurons by multiple mechanisms. J Neurophysiol. (2001) 86:1652–60. doi: 10.1152/jn.2001.86.4.1652
69. Takeda A. Zinc homeostasis and functions of zinc in the brain. Biometals (2001) 14:343–51. doi: 10.1023/A:1012982123386
70. Persson E, Henriksson J, Tallkvist J, Rouleau C, Tjlve H. Transport and subcellular distribution of intranasally administered zinc in the olfactory system of rats and pikes. Toxicology (2003) 191:97–108. doi: 10.1016/S0300-483X(03)00208-7
71. Frederickson CJ, Giblin LJ, Krez̧èl A, McAdoo DJ, Muelle RN, Zeng Y, et al. Concentrations of extracellular free zinc (pZn)e in the central nervous system during simple anesthetization, ischemia and reperfusion. Exp Neurol. (2006) 198:285–93. doi: 10.1016/j.expneurol.2005.08.030
72. Duan X, Block E, Li Z, Connelly T, Zhang J, Huang Z, et al. Crucial role of copper in detection of metal-coordinating odorants. Proc Natl Acad Sci USA (2012) 109:3492–7. doi: 10.1073/pnas.1111297109
73. Li S, Ahmed L, Zhang R, Pan Y, Matsunami H, Burger JL, et al. Smelling Sulfur: copper and silver regulate the response of human odorant receptor OR2T11 to low-molecular-weight thiols. J Amer Chem Soc. (2016) 138:13281–8. doi: 10.1021/jacs.6b06983
74. Block E. Fifty years of smelling sulfur: from the chemistry of garlic to the molecular basis for olfaction. Phosphorus Sulf Silicon Relat Elements (2017) 192:141–4. doi: 10.1080/10426507.2016.1247091
75. Rodrguez FI, Esch JJ, Hall AE, Binder BM, Schaller GE, Bleecker AB. A copper cofactor for the ethylene receptor ETR1 from Arabidopsis. Science (1999) 283:996–8. doi: 10.1126/science.283.5404.996
76. Frazier JL, Hetiz JR. Inhibition of olfaction in the moth heliothis virescens by the sulfhydryl reagent fluorescein mercuric acetate. Chem Sens. (1975) 1:271. doi: 10.1093/chemse/1.3.271
77. Persson E, Henriksson J, Tjlve H. Uptake of cobalt from the nasal mucosa into the brain via olfactory pathways in rats. Toxicol Lett. (2003) 145:19–27. doi: 10.1016/S0378-4274(03)00266-2
78. Frederickson CJ, Koh JY, Bush AI. The neurobiology of zinc in health and disease. Nat Rev Neurosci. (2005) 6:449–62. doi: 10.1038/nrn1671
79. Takeda A, Ohnuma M, Sawashita J, Okada S. Zinc transport in the rat olfactory system. Neurosci Lett. (1997) 225:69–71. doi: 10.1016/S0304-3940(97)00181-X
80. Henriksson J, Tallkvist J, Tjlve H. Uptake of nickel into the brain via olfactory neurons in rats. Toxicol Lett. (1997) 91:153–62. doi: 10.1016/S0378-4274(97)03885-X
81. Henriksson J, Tjlve H. Uptake of inorganic mercury in the olfactory bulbs via olfactory pathways in rats. Environ Res. (1998) 77:130–40. doi: 10.1006/enrs.1997.3817
82. Haffenden LJW, Yaylayan VA, Fortin J. Investigation of vibrational theory of olfaction with variously labelled benzaldehydes. Food Chem. (2001) 73:67–72. doi: 10.1016/S0308-8146(00)00287-9
84. 04 ASE. Standard Test Method for Sensory Analysis Duo-Trio Test. Conshohocken, PA : ASTM International (2011).
85. Keller A, Vosshall LB. A psychophysical test of the vibration theory of olfaction. Nat Neurosci. (2004) 7:337–8. doi: 10.1038/nn1215
86. Franco MI, Turin L, Mershin A, Skoulakis EMC. Molecular vibration-sensing component in Drosophila melanogaster olfaction. Proc Natl Acad Sci USA (2011) 108:3797–802. doi: 10.1073/pnas.1012293108
87. Olton DS. Mazes, maps and memory. Amer Psychol. (1979) 34:583–96. doi: 10.1037/0003-066X.34.7.583
88. Gane S, Georganakis D, Maniati K, Vamvakias M, Ragoussis N, Skoulakis EMC, et al. Molecular vibration-sensing component in human olfaction. PLoS ONE (2013) 8:e55780. doi: 10.1371/journal.pone.0055780
89. Gane S, Georganakis D, Maniati K, Vamvakias M, Ragoussis N, Skoulakis EMC, et al. Correction: molecular vibration-sensing component in human olfaction. PLoS ONE (2013) 8. doi: 10.1371/annotation/2f278ed8-d5e7-440a-9e49-c8d1df20d1f1
90. Büttner MW, Penka M, Doszczak L, Kraft P, Tacke R. Silicon analogues of the musk odorant versalide. Organometallics (2007) 26:1295–8. doi: 10.1021/om060934h
91. Gronenberg W, Raikhelkar A, Abshire E, Stevens J, Epstein E, Loyola K, et al. Honeybees (Apis mellifera) learn to discriminate the smell of organic compounds from their respective deuterated isotopomers. Proc R Soc Lond B Biol Sci. (2014) 281:20133089. doi: 10.1098/rspb.2013.3089
92. Block E, Jang S, Matsunami H, Sekharan S, Dethier B, Ertem MZ, et al. Implausibility of the vibrational theory of olfaction. Proc Natl Acad Sci USA (2015) 112:E2766–74. doi: 10.1073/pnas.1503054112
93. Turin L, Gane S, Georganakis D, Maniati K, Skoulakis EMC. Plausibility of the vibrational theory of olfaction. Proc Natl Acad Sci USA (2015) 112:E3154. doi: 10.1073/pnas.1508035112
94. Nagashima A, Touhara K. Enzymatic conversion of odorants in nasal mucus affects olfactory glomerular activation patterns and odor perception. J Neurosci. (2010) 30:16391–8. doi: 10.1523/JNEUROSCI.2527-10.2010
95. Seok WK, Meyer TJ. Mechanism of oxidation of benzaldehyde by polypyridyl oxo complexes of Ru(IV). Inorgan Chem. (2005) 44:3931–41. doi: 10.1021/ic040119z
96. Hahn I, Scherer PW, Mozell MM. A mass transport model of olfaction. J Theor Biol. (1994) 167:115–28. doi: 10.1006/jtbi.1994.1057
97. Wilkes FJ, Laing DG, Hutchinson I, Jinks AL, Monteleone E. Temporal processing of olfactory stimuli during retronasal perception. Behav Brain Res. (2009) 200:68–75. doi: 10.1016/j.bbr.2008.12.031
98. Paoli M, Anesi A, Antolini R, Guella G, Vallortigara G, Haase A. Differential odour coding of isotopomers in the honeybee brain. Sci Rep. (2016) 6:21893. doi: 10.1038/srep21893
99. Drimyli E, Gaitanidis A, Maniati K, Turin L, Skoulakis EMC. Differential electrophysiological responses to odorant isotopologues in drosophilid antennae. eNeuro (2016) 3:e0152–15. doi: 10.1523/ENEURO.0152-15.2016
100. Alcorta E. Characterization of the electroantennogram in Drosophila melanogaster and its use for identifying olfactory capture and transduction mutants. J Neurophysiol. (1991) 65:702–14. doi: 10.1152/jn.1991.65.3.702
101. Wade D. Deuterium isotope effects on noncovalent interactions between molecules. Chem Biol Interact. (1999) 117:191–217. doi: 10.1016/S0009-2797(98)00097-0
102. Block E, Jang S, Matsunami H, Batista VS, Zhuang H. Reply to Turin et al.: vibrational theory of olfaction is implausible. Proc Natl Acad Sci USA (2015) 112:E3155. doi: 10.1073/pnas.1508443112
103. Vosshall LB. Laying a controversial smell theory to rest. Proc Natl Acad Sci USA (2015) 112:6525–6. doi: 10.1073/pnas.1507103112
104. Pevsner J, Hou V, Snowman AM, Snyder SH. Odorant-binding protein. Characterization of ligand binding. J Biol Chem. (1990) 265:6118–25.
105. Vogt RG, Prestwich GD, Lerner MR. Odorant-binding-protein subfamilies associate with distinct classes of olfactory receptor neurons in insects. J Neurobiol. (1991) 22:74–84. doi: 10.1002/neu.480220108
106. Pelosi P. Odorant-binding proteins. Crit Rev Biochem Mol Biol. (1994) 29:199–228. doi: 10.3109/10409239409086801
107. Krzan M, Vianello R, Marsavelski A, Repic M, Zaksek M, Kotnik K, et al. The quantum nature of drug-receptor interactions: deuteration changes binding affinities for histamine receptor ligands. PLoS ONE (2016) 11:e0154002. doi: 10.1371/journal.pone.0154002
108. Paoli M, Münch D, Haase A, Skoulakis E, Turin L, Galizia CG. Minute impurities contribute significantly to olfactory receptor ligand studies: tales from testing the vibration theory. eNeuro (2017) 4:ENEURO.0070-17.2017. doi: 10.1523/ENEURO.0070-17.2017
109. Jonathan P, McCarthy WVM, Roberts AMI. Discriminant analysis with singular covariance matrices. A method incorporating cross-validation and efficient randomized permutation tests. J Chemometr. (1996) 10:189–213. doi: 10.1002/(SICI)1099-128X(199605)10:3<189::AID-CEM410>3.0.CO;2-I
110. Ferguson AM, Heritage T, Jonathon P, Pack SE, Phillips L, Rogan J, et al. EVA: a new theoretically based molecular descriptor for use in QSAR/QSPR analysis. J Comput Aided Mol Design (1997) 11:143–52. doi: 10.1023/A:1008026308790
111. Takane Sy, Mitchell JBO. A structure-odour relationship study using EVA descriptors and hierarchical clustering. Org Biomol Chem. (2004) 2:3250–5. doi: 10.1039/B409802A
112. Brookes JC, Hartoutsiou F, Horsfield AP, Stoneham AM. Could humans recognize odor by phonon assisted tunneling? Phys Rev Lett. (2007) 98:038101. doi: 10.1103/PhysRevLett.98.038101
113. Brookes JC, Horsfield AP, Stoneham AM. The swipe card model of odorant recognition. Sensors (2012) 12:15709–49. doi: 10.3390/s121115709
114. Solov'yov IA, Chang PY, Schulten K. Vibrationally assisted electron transfer mechanism of olfaction: myth or reality? Phys Chem Chem Phys. (2012) 14:13861–71. doi: 10.1039/c2cp41436h
115. Bittner ER, Madalan A, Czader A, Roman G. Quantum origins of molecular recognition and olfaction in drosophila. J Chem Phys. (2012) 137:22A551. doi: 10.1063/1.4767067
116. Chȩcińska A, Pollock FA, Heaney L, Nazir A. Dissipation enhanced vibrational sensing in an olfactory molecular switch. J Chem Phys. (2015) 142:025102. doi: 10.1063/1.4905377
117. Tirandaz A, Taher Ghahramani F, Shafiee A. Dissipative vibrational model for chiral recognition in olfaction. Phys Rev E (2015) 92:032724. doi: 10.1103/PhysRevE.92.032724
118. Wang M, Gao J, Mller P, Giese B. Electron transfer in peptides with cysteine and methionine as relay amino acids. Angew Chem Int Edn. (2009) 48:4232–4. doi: 10.1002/anie.200900827
119. Jia Y, DiMagno TJ, Chan CK, Wang Z, Popov MS, Du M, et al. Primary charge separation in mutant reaction centers of Rhodobacter capsulatus. J Phys Chem. (1993) 97:13180–91. doi: 10.1021/j100152a024
120. Klika KD. The potential of 13C isotopomers as a test for the vibrational theory of olfactory sense recognition. ISRN Org Chem. (2013) 2013:515810. doi: 10.1155/2013/515810
121. Saberi M, Seyed-allaei H. Odorant receptors of Drosophila are sensitive to the molecular volume of odorants. Sci Rep. (2016) 6:25103. doi: 10.1038/srep25103
122. Boesveldt S, Olsson MJ, Lundstrm JN. Carbon chain length and the stimulus problem in olfaction. Behav Brain Res. (2010) 215:110–3. doi: 10.1016/j.bbr.2010.07.007
123. Brookes JC, Horsfield AP, Stoneham AM. Odour character differences for enantiomers correlate with molecular flexibility. J R Soc Interf. (2009) 6:75–86. doi: 10.1098/rsif.2008.0165
124. Rakowski K, Paneth P. Isotope effects on binding. J Mol Struct. (1996) 378:35–43. doi: 10.1016/0022-2860(95)09146-7
125. Dybala-Defratyka A, Rostkowski M, Paneth P. Enzyme mechanisms from molecular modeling and isotope effects. Arch Biochem Biophys. (2008) 474:274–82. doi: 10.1016/j.abb.2008.01.012
126. Świderek K, Paneth P. Binding isotope effects. Chem Rev. (2013) 113:7851–79. doi: 10.1021/cr300515x
127. Tirandaz A, Taher Ghahramani F, Salari V. Validity examination of the dissipative quantum model of olfaction. Sci Rep. (2017) 7:4432. doi: 10.1038/s41598-017-04846-8
128. Doszczak L, Kraft P, Weber HP, Bertermann R, Triller A, Hatt H, et al. Prediction of perception: probing the hOR17-4 olfactory receptor model with silicon analogues of bourgeonal and lilial. Angew Chem Int Edn. (2007) 46:3367–71. doi: 10.1002/anie.200605002
129. Drrich S, Gelis L, Wolf S, Sunderktter A, Mahler C, Guschina E, et al. Comparative analysis of the olfactory properties of silicon/germanium/tin analogues of the lily-of-the-valley odorants lilial and bourgeonal. ChemPlusChem (2014) 79:1747–52. doi: 10.1002/cplu.201402160
130. Wolf S, Gelis L, Drrich S, Hatt H, Kraft P. Evidence for a shape-based recognition of odorants in vivo in the human nose from an analysis of the molecular mechanism of lily-of-the-valley odorants detection in the Lilial and Bourgeonal family using the C/Si/Ge/Sn switch strategy. PLoS ONE (2017) 12:e0182147. doi: 10.1371/journal.pone.0182147
131. Hoover KC. Smell with inspiration: the evolutionary significance of olfaction. Amer J Phys Anthropol. (2010) 143:63–74. doi: 10.1002/ajpa.21441
132. Gazave E, Lapébie P, Richards GS, Brunet F, Ereskovsky AV, Degnan BM, et al. Origin and evolution of the Notch signalling pathway: an overview from eukaryotic genomes. BMC Evol Biol. (2009) 9:249. doi: 10.1186/1471-2148-9-249
133. King N, Westbrook MJ, Young SL, Kuo A, Abedin M, Chapman J, et al. The genome of the choanoflagellate Monosiga brevicollis and the origin of metazoans. Nature (2008) 451:783–8. doi: 10.1038/nature06617
134. Manning G, Young SL, Miller WT, Zhai Y. The protist, Monosiga brevicollis, has a tyrosine kinase signaling network more elaborate and diverse than found in any known metazoan. Proc Natl Acad Sci USA (2008) 105:9674–9. doi: 10.1073/pnas.0801314105
135. Suga H, Dacre M, de Mendoza A, Shalchian-Tabrizi K, Manning G, Ruiz-Trillo I. Genomic survey of premetazoans shows deep conservation of cytoplasmic tyrosine kinases and multiple radiations of receptor tyrosine kinases. Sci Signal. (2012) 5:ra35. doi: 10.1126/scisignal.2002733
136. de Mendoza A, Sebé-Pedrós A, Ruiz-Trillo I. The evolution of the GPCR signaling system in eukaryotes: modularity, conservation, and the transition to metazoan multicellularity. Genome Biol Evol. (2014) 6:606–19. doi: 10.1093/gbe/evu038
137. Zhang Z, Wu J, Yu J, Xiao J. A brief review on the evolution of GPCR: conservation and diversification. Open J Genet. (2012) 2:11–7. doi: 10.4236/ojgen.2012.24B003
138. Nordström KJV, Lagerström MC, Wallér LMJ, Fredriksson R, Schiöth HB. The secretin GPCRs descended from the family of adhesion GPCRs. Mol Biol Evol. (2009) 26:71–84. doi: 10.1093/molbev/msn228
139. Kaupp UB. Olfactory signalling in vertebrates and insects: differences and commonalities. Nat Rev Neurosci. (2010) 11:188–200. doi: 10.1038/nrn2789
140. Fleischer J, Breer H, Strotmann J. Mammalian olfactory receptors. Front Cell Neurosci. (2009) 3:9. doi: 10.3389/neuro.03.009.2009
141. Bohbot JD, Pitts RJ. The narrowing olfactory landscape of insect odorant receptors. Front Ecol Evol. (2015) 3:39. doi: 10.3389/fevo.2015.00039
142. Su CY, Menuz K, Carlson JR. Olfactory perception: receptors, cells, and circuits. Cell (2009) 139:45–9. doi: 10.1016/j.cell.2009.09.015
143. Bushdid C, Magnasco MO, Vosshall LB, Keller A. Humans can discriminate more than 1 trillion olfactory stimuli. Science (2014) 343:1370–2. doi: 10.1126/science.1249168
144. Gerkin RC, Castro JB. The number of olfactory stimuli that humans can discriminate is still unknown. eLife (2015) 4:e08127. doi: 10.7554/eLife.08127
145. Meister M. On the dimensionality of odor space. eLife (2015) 4:e07865. doi: 10.7554/eLife.07865
146. Vassar R, Chao SK, Sitcheran R, Nunez JM, Vosshall LB, Axel R. Topographic organization of sensory projections to the olfactory bulb. Cell (1994) 79:981–91. doi: 10.1016/0092-8674(94)90029-9
147. Ressler KJ, Sullivan SL, Buck LB. A zonal organization of odorant receptor gene expression in the olfactory epithelium. Cell (1993) 73:597–609. doi: 10.1016/0092-8674(93)90145-G
148. Belluscio L, Lodovichi C, Feinstein P, Mombaerts P, Katz LC. Odorant receptors instruct functional circuitry in the mouse olfactory bulb. Nature (2002) 419:296–300. doi: 10.1038/nature01001
149. Hallem EA, Dahanukar A, Carlson JR. Insect odor and taste receptors. Annu Rev Entomol. (2006) 51:113–35. doi: 10.1146/annurev.ento.51.051705.113646
150. Liu F, Chen Z, Liu N. Molecular basis of olfactory chemoreception in the common bed bug, cimex lectularius. (2017) 7:45531. doi: 10.1038/srep45531
151. Krieger J, Breer H. Olfactory reception in invertebrates. Science (1999) 286:720–3. doi: 10.1126/science.286.5440.720
152. Troemel ER, Kimmel BE, Bargmann CI. Reprogramming chemotaxis responses: sensory neurons define olfactory preferences in C. elegans. Cell (1997) 91:161–9. doi: 10.1016/S0092-8674(00)80399-2
153. Sanchez-Gracia A, Vieira FG, Rozas J. Molecular evolution of the major chemosensory gene families in insects. Heredity (2009) 103:208–16. doi: 10.1038/hdy.2009.55
154. Hill CA, Fox AN, Pitts RJ, Kent LB, Tan PL, Chrystal MA, et al. G protein-coupled receptors in Anopheles gambiae. Science (2002) 298:176–8. doi: 10.1126/science.1076196
155. Sato K, Pellegrino M, Nakagawa T, Nakagawa T, Vosshall LB, Touhara K. Insect olfactory receptors are heteromeric ligand-gated ion channels. Nature (2008) 452:1002–6. doi: 10.1038/nature06850
156. Wicher D, Schafer R, Bauernfeind R, Stensmyr MC, Heller R, Heinemann SH, et al. Drosophila odorant receptors are both ligand-gated and cyclic-nucleotide-activated cation channels. Nature (2008) 452:1007–11. doi: 10.1038/nature06861
157. Missbach C, Dweck HK, Vogel H, Vilcinskas A, Stensmyr MC, Hansson BS, et al. Evolution of insect olfactory receptors. eLife (2014) 3:e02115. doi: 10.7554/eLife.02115
158. Jones WD, Nguyen TAT, Kloss B, Lee KJ, Vosshall LB. Functional conservation of an insect odorant receptor gene across 250 million years of evolution. Curr Biol. (2005) 15:R119–21. doi: 10.1016/j.cub.2005.02.007
159. Swaney WT, Keverne EB. The evolution of pheromonal communication. Behav Brain Res. (2009) 200:239–47. doi: 10.1016/j.bbr.2008.09.039
160. Zhang R, Gao G, Chen H. Silencing of the olfactory co-receptor gene in Dendroctonus armandi leads to EAG response declining to major host volatiles. Sci Rep. (2016) 6:23136. doi: 10.1038/srep23136
161. Oh SJ. Characteristics in molecular vibrational frequency patterns between agonists and antagonists of histamine receptors. Genomics Inform. (2012) 10:128–32. doi: 10.5808/GI.2012.10.2.128
162. Chee HK, Oh SJ. Molecular vibration-activity relationship in the agonism of adenosine receptors. Genom Inform. (2013) 11:282–8. doi: 10.5808/GI.2013.11.4.282
163. Chee HK, Yang JS, Joung JG, Zhang BT, Oh SJ. Characteristic molecular vibrations of adenosine receptor ligands. FEBS Lett. (2015) 589:548–52. doi: 10.1016/j.febslet.2015.01.024
164. Hoehn RD, Nichols D, Neven H, Kais S. Neuroreceptor activation by vibration-assisted tunneling. Sci Rep. (2015) 5:9990. doi: 10.1038/srep09990
165. Buck LB. Olfactory receptors and odor coding in mammals. Nutr Rev. (2004) 62:S184–8. doi: 10.1111/j.1753-4887.2004.tb00097.x
166. Hoehn RD, Nichols DE, McCorvy JD, Neven H, Kais S. Experimental evaluation of the generalized vibrational theory of G protein-coupled receptor activation. Proc Natl Acad Sci USA (2017) 114:5595–600. doi: 10.1073/pnas.1618422114
167. Malnic B, Hirono J, Sato T, Buck LB. Combinatorial receptor codes for odors. Cell (1999) 96:713–23. doi: 10.1016/S0092-8674(00)80581-4
168. Snitz K, Yablonka A, Weiss T, Frumin I, Khan RM, Sobel N. Predicting odor perceptual similarity from odor structure. PLoS Comput Biol. (2013) 9:e1003184. doi: 10.1371/journal.pcbi.1003184
169. Sachse S, Rappert A, Galizia CG. The spatial representation of chemical structures in the antennal lobe of honeybees: steps towards the olfactory code. Eur J Neurosci. (1999) 11:3970–82. doi: 10.1046/j.1460-9568.1999.00826.x
170. Hallem EA, Carlson JR. The odor coding system of Drosophila. Trends Genet. (2004) 20:453–9. doi: 10.1016/j.tig.2004.06.015
171. Silbering AF, Okada R, Ito K, Galizia CG. Olfactory information processing in the Drosophila antennal lobe: Anything goes? J Neurosci. (2008) 28:13075–87. doi: 10.1523/JNEUROSCI.2973-08.2008
172. Grabe V, Strutz A, Baschwitz A, Hansson BS, Sachse S. Digital in vivo 3D atlas of the antennal lobe of Drosophila melanogaster. J Compar Neurol. (2015) 523:530–44. doi: 10.1002/cne.23697
173. Takahashi YK, Kurosaki M, Hirono S, Mori K. Topographic representation of odorant molecular features in the rat olfactory bulb. J Neurophysiol. (2004) 92:2413–27. doi: 10.1152/jn.00236.2004
174. Mori K, Takahashi YK, Igarashi KM, Yamaguchi M. Maps of odorant molecular features in the mammalian olfactory bulb. Physiol Rev. (2006) 86:409–33. doi: 10.1152/physrev.00021.2005
175. Sell CS. On the unpredictability of odor. Angew Chem Int Edn. (2006) 45:6254–61. doi: 10.1002/anie.200600782
176. Haddad R, Khan R, Takahashi YK, Mori K, Harel D, Sobel N. A metric for odorant comparison. Nat Meth. (2008) 5:425–9. doi: 10.1038/nmeth.1197
177. Khan RM, Luk CH, Flinker A, Aggarwal A, Lapid H, Haddad R, et al. Predicting odor pleasantness from odorant structure: pleasantness as a reflection of the physical world. J Neurosci. (2007) 27:10015–23. doi: 10.1523/JNEUROSCI.1158-07.2007
178. Iurilli G, Datta SR. Population coding in an innately relevant olfactory area. Neuron (2017) 93:1180–1197.e7. doi: 10.1016/j.neuron.2017.02.010
179. Raman B, Stopfer M, Semancik S. Mimicking biological design and computing principles in artificial olfaction. ACS Chem Neurosci. (2011) 2:487–99. doi: 10.1021/cn200027r
180. Sen A, Suslick KS. Shape-selective discrimination of small organic molecules. J Am Chem Soc. (2000) 122:11565–6. doi: 10.1021/ja000002j
181. Rivera JF, Arce JC, Velasco-Medina J. Specific single-molecule detection based on orbital mediated tunneling in nanotube double-barrier heterostructures. IEEE Trans Nanotechnol. (2012) 11:1174–82. doi: 10.1109/TNANO.2012.2219553
Keywords: olfaction, biophyiscs, vibration detection, review literature as topic, GPCRs
Citation: Hoehn RD, Nichols DE, Neven H and Kais S (2018) Status of the Vibrational Theory of Olfaction. Front. Phys. 6:25. doi: 10.3389/fphy.2018.00025
Received: 23 November 2017; Accepted: 28 February 2018;
Published: 19 March 2018.
Edited by:
Alkwin Slenczka, University of Regensburg, GermanyReviewed by:
Jens Riedel, Bundesanstalt für Materialforschung und Prüfung (BAM), GermanyCopyright © 2018 Hoehn, Nichols, Neven and Kais. This is an open-access article distributed under the terms of the Creative Commons Attribution License (CC BY). The use, distribution or reproduction in other forums is permitted, provided the original author(s) and the copyright owner are credited and that the original publication in this journal is cited, in accordance with accepted academic practice. No use, distribution or reproduction is permitted which does not comply with these terms.
*Correspondence: Ross D. Hoehn, cmhvZWhuODcwMUBnbWFpbC5jb20=
Sabre Kais, a2Fpc0BwdXJkdWUuZWR1
Disclaimer: All claims expressed in this article are solely those of the authors and do not necessarily represent those of their affiliated organizations, or those of the publisher, the editors and the reviewers. Any product that may be evaluated in this article or claim that may be made by its manufacturer is not guaranteed or endorsed by the publisher.
Research integrity at Frontiers
Learn more about the work of our research integrity team to safeguard the quality of each article we publish.