- 1Systems Medicine and Bioengineering Laboratory, Houston Methodist Research Institute, Houston, TX, United States
- 2Texas A&M University Health Science Center School of Medicine, College Station, TX, United States
- 3Department of Biomedical Engineering, Texas A&M University College of Engineering, College Station, TX, United States
- 4Beckman Laser Institute and Medical Clinic, Professor Department of Surgery and Biomedical Engineering, University of California Irvine, Irvine, CA, United States
This review examines circadian dysregulation and the role of Müller glial cells (MGCs) in retinal degeneration associated with Alzheimer’s disease (AD). Evidence supporting the interdependence of circadian rhythm (CR) disruption and AD progression is presented. Also reviweed are reports substantiating the role of MGCs in maintaining CR. Studies documenting MGC dysfunction in AD retinas suggest that gliosis, altered diurnal patterns in water homeostasis, blood-retina barrier breakdown, and impaired ocular glymphatic clearance are relevant to disease progression. Similarities between AD and various retinopathies are explored with respect to MGC physiology and CR dysfunction. We propose that MGC circadian dysregulation is diagnostically and therapeutically relevant to AD retinopathy.
Circadian Rhythm (CR) disruption and Alzheimer’s disease (AD)
CR refers to the timing of biological processes based on a 24-h master clock. These processes are further controlled through the oscillating expression of core clock genes. Clock gene oscillations are influenced by external environmental cues. In their review, Xie et al. (2019) describes CR coordination as consisting of three pathways: the input pathway, the “master clock” pathway, and the output pathway. The input pathway senses external CR synchronizing cues. These are predominantly photic (light intensity) cues detected by the retina. Non-photic cues that regulate CR include external temperature, sustenance, and stress. Both photic and nonphotic cues are sent to the master clock—the suprachiasmatic nuclei (SCN) of the hypothalamus. The SCN communicates with peripheral tissues through endocrine and neural pathways to dictate clock gene oscillations. Two core clock transcriptional activators are Clock and Bmal-1. Clock and Bmal-1 promote expression of its own repressors, such as Per1 and Per2, to “reset the clock” of circadian gene expression. These gene expression oscillations modulate the sleep-wake cycle, metabolism, and temperature homeostasis, among other physiological processes.
Sleep cycle and CR disruption have been noted in both early and late stages of AD. AD patients show a significant loss of REM sleep (Prinz et al., 1982; D’Atri et al., 2021). Interestingly, Park et al. (2020) found decreased pineal gland size in patients with concomitant REM sleep behavior disorder and AD. In Apoε4 carrier patients, degraded REM sleep is associated with faster AD progression (Pyun et al., 2019; Baril et al., 2020). NREM sleep is also diminished in AD; however, the utility of K-complex density as a prognostic EEG biomarker is controversial (De Genarro et al., 2017; Reda et al., 2017; Lucey, 2020; Chylinski et al., 2022). AD patients also show alterations in diurnal temperature regulation. Some of these changes include higher proximal amplitudes of core body temperature oscillations and delayed temperature peaks (Homolak et al., 2018). Most et al. (2012) reported that daytime sleepiness in AD patients positively correlates with skin temperature of the thighs, abdomen, and infraclavicular regions. AD patients commonly exhibit reduced daytime motor activity and increased nocturnal activity (Homolak et al., 2018). This nocturnal activity is frequently associated with agitation and aggression, a behavior referred to as ‘sundowning.’ These temperature and sleep regulation disturbances can be linked to SCN dysfunction. AD animal models show SCN degeneration (Zhou et al., 2016; Roy et al., 2019). A human postmortem study of AD hypothalami revealed neuronal loss and neurofibrillary tau tangle formation in the SCN (Stopa et al., 1999). Chronotherapeutic studies indicate that morning light therapy improves cognitive function in dementia patients by preserving CR (Mishima et al., 1994; Ancoli-Isreal et al., 2003). Clinical observations and knowledge of SCN degeneration indicate that protein accumulation in AD brains may be correlated to CR dysfunction.
One of the best-studied mechanisms indicating CR dysregulation’s contribution to AD is impaired glymphatic clearance. Glymphatic clearance refers to paravascular transport of cerebrospinal fluid (CSF) which flushes out toxic solutes from neural tissues. Furtado et al. (2020) found dysregulated rhythmicity of Bmal-1 and Per1 in APP/PS1 mouse choroid plexus cells, which are the cells that produce CSF. Choroid plexus rhythmicity was successfully reestablished with melatonin treatment. Furtado et al. (2020) found decreased rhythmicity of murine Aβ-degrading proteins produced by the choroid plexus in female APP/PS1 mice. These studies suggest that choroid plexus dysfunction is related to CR disruption and perpetuates neurotoxic Aβ plaque formation. Glymphatic fluid transport increases dramatically during sleep. Xie et al. (2019) performed two-photon imaging studies of CSF circulation in mice and found that: a) the volume of the cerebral interstitial space increases by 60% during sleep, b) this increase is associated with CSF-interstitial fluid exchange, and c) sleep enhances glymphatic clearance of Aβ. Another AD biomarker affected by CR and poor glymphatic clearance is Apolipoprotein E (ApoE). Achariyar et al. (2016) found that sleep deprivation alters cerebral distribution of ApoE. Kress et al. (2018) found that global knockout of Bmal-1 in mouse brains promotes Aβ plaque formation and upregulates ApoE. These findings indicate that sleep-wake cycle dysregulation hinders glymphatic clearance, thereby promoting neurotoxic protein deposition. Lax et al. (2011) found that daily exogenous melatonin administration in Sprague-Dawley rodents homozygous for the P23H-3 rhodopsin mutation delayed and temporarily reversed vision loss.
A bidirectional relationship exists between AD-associated protein aggregation and glymphatic clearance: impaired glymphatic clearance allows toxic solutes to accumulate, and these solutes then alter cellular pathways to further impair glymphatic clearance. Aβ induces astrocyte gliosis, which promotes neuroinflammation (Batarseh et al., 2016). Part of the gliosis cascade is downregulated aquaporin-4 (AQP4), the water channel responsible for glymphatic fluid exchange. Decreased AQP4 expression indicates Aβ is not cleared from the brain, so it remains in the brain to perpetuate glial activation. Harrison et al. (2020) found that impaired tau clearance is associated with improper AQP4 polarization. Uncleared tau can activate inflammatory cascades in microglia (Joly-Amado et al., 2020; Wang et al., 2020) and epigenetically alter astrocyte-neuron-immune crosstalk (Zhou et al., 2023).
Neuroinflammation is also consistent with blood-brain barrier (BBB) disruption as a link between CR and AD. BBB disruption diminishes Aβ receptor-mediated transport out of the brain (Deane et al., 2008). Bellesi et al. (2017) found that chronic sleep restriction promotes astrocytic phagocytosis and microglial activation in wild-type mouse cerebral cortices, well before obvious signs of neuroinflammation appear. Kaneshwaran et al. (2019) conducted an antemortem microglial activation study of humans with and without AD. Actigraphy results indicating greater sleep fragmentation correlated with microglial aging and activation in the neocortex. These microglial changes were associated with poorer cognition test scores proximate to death in both normal and AD subjects. Gómez-González et al. (2013) found that REM sleep deprivation in rats increased BBB permeability in the whole brain. Brief periods of sleep recovery failed to recover BBB integrity in the hippocampus and cerebellum. REM sleep deprivation also increased caveolae formation and tripled the number of pinocytotic vesicles in the hippocampus. A separate REM sleep deprivation study in a rodent model by He et al. (2014) found decreased mRNA expression of several tight junction proteins. Loss of PDGFR-β is implicated in AD pathology (Janota et al., 2015; Ding et al., 2020; Smyth et al., 2022). Medina-Flores et al. (2020) found in male Wistar rats that sleep restriction downregulated PDGFR-β. CD44-mediated epithelial-immune interactions is also associated with BBB dysfunction and may have a connection to CR dysregulation. Multiple studies have explored increases in BBB permeability mediated by CD44-hyaluronan binding in various diseases (Winkler et al., 2012; Ventorp et al., 2016; Al-Ahmad et al., 2019). In AD, CD44 is thought to create a pro-inflammatory state that further promotes Aβ aggregation (Miners et al., 2018; Lee et al., 2023.) Sun et al. (2020) found that sleep deprivation impaired cognition, increased inflammatory cytokine production, astrocyte activation, and BBB permeability in a CD44 overexpression mouse model. These studies correlating CR alterations with BBB permeability correlate with why poor sleep increases risk of developing AD.
Müller glial cells (MGCs)
MGCs are the dominant glial cell type in vertebrate retinas and support neuron survival via extracellular environment regulation, trophic factor release, GABA and glutamate recycling, and intercellular transport facilitation (Goldman, 2014). MGC intracellular responses and pliability help maintain structural integrity in the retina (Lindqvist et al., 2010; Govetto et al., 2020). MGCs secrete angiogenesis modulators that interact with capillary endothelial cells during blood-retina barrier formation (Abukuwa et al., 2009). MGCs have neurogenic stem cell properties akin to embryonic radial glia (Das et al., 2006) and are especially effective at regenerating rod photoreceptors (Gianelli et al., 2011). Animal studies of retinal injury responses show MGC dedifferentiation and polarized cell division to produce retinal neuron progenitors (Fischer and Reh, 2003; Lenkowski and Raymond, 2014). These progenitors migrate along MGC radial processes to replace lost neurons. MGCs mediate innate immunity through Toll-like receptors and complement protein secretion (Vecino et al., 2016). The human MGC response to retinal injury–reactive gliosis–consists of inflammation, cell proliferation, and cell hypertrophy (Goldman, 2014; Vecino et al., 2016). Although gliosis can protect retinal neurons by preventing glutamate neurotoxicity and releasing trophic factors, excessive degrees can induce retinal neurodegeneration by creating an inflammatory state.
MGCs are the only cells whose processes span the entire retinal thickness. Their cell bodies are in the inner nuclear layer. Their processes contact photoreceptors and other retinal neurons. MGCs’ length aids their ability to support neurons throughout the retina and enables MGCs to guide incident light toward photoreceptors. MGCs are oriented in the direction of incoming light propagation. MGCs have higher refractive indices than surrounding cells to limit light scattering and beam widening (Franze et al., 2007; Agte et al., 2011). Extensive branching of MGC processes increases the effective refractive index. Funnel-shaped endfeet at the vitreous-retinal interface cover nearly the whole inner surface of the retina. These funnels collect incoming light entering the retina, channel toward the cell body, and deliver to a small-diameter receptive field made of photoreceptor cells (Agte et al., 2011; Szabo et al., 2022). Optical simulations by Szabo et al. (2022) illustrate how parafoveal MGCs behave like multimodal optical fibers and guide incoming light. MGCs exhibit low light collection efficiency and optical smearing when spot size exceeds endfoot diameter. However, power is still concentrated in the “core” of parafoveal MGC pillars because increased incidence angles in these areas increase the number of guided modes. Moving further away from the pupil-fovea axis, higher order modes dominate and thus become more important in determining light transmission. These findings indicate that MGCs effectively function as multimodal optical fibers that guide visible light to photoreceptors.
MGCs play a role in retinal CR synchronization. The retina sets its own tissue-specific clock gene rhythms, which are distinct from the SCN’s rhythms as well as more sensitive to gene perturbation (Ruan et al., 2012). According to Xu et al. (2016), human and murine MGCs express the whole spectrum of core clock genes and exhibit Bmal-1-dependent diurnal oscillations independently of surrounding tissues. Ko (2020) proposed an ATP-based mechanism for how MGCs communicate with other retinal oscillators. MGCs synthesize and accumulate extracellular ATP, which can be converted to adenosine. Extracellular adenosine in the retina is elevated at nighttime; perhaps MGC synthesis of ATP coordinated by clock genes is responsible for this. Retinal adenosine regulates circadian control of rod-cone coupling and light/dark adaptation. Wei et al. (2023) found some evidence supporting this proposal in murine retinas. Wei et al. found that light upregulates the MGC deiodinase Dio2. This promotes thyroid hormone T3 synthesis, which then increases ATP production for cone sodium-potassium pumps during light adaptation. Interestingly, Ríos et al. (2019) identified the blue light-sensitive pigments Opn3 and Opn5 in chicken embryo MGCs. Marchese et al. (2021) further identified that prolonged blue light exposure triggers Ca2+ release from endoplasmic reticula in Opn3-expressing MGCs. This observation may help explain how MGCs detect ambient light for CR control. Peak guidance of light wavelengths toward cones (center of MGC receptive field) occurs in the green-yellow range (Labin and Ribak, 2010; Labin et al., 2014). Blue-violet light leaks out of MGCs and enters nearby rods, which are more sensitive to these wavelengths. Directing certain light spectra toward cones enhances daytime vision and minimally affects nighttime vision. The oscillatory gene expression patterns, light/dark adaptation functions, and photoreceptor-like activities of MGCs make them candidate circadian synchronizers in the retina.
Key AD pathological processes manifest in the retina, which are elaborated on throughout this review. The role MGCs play in retinal neurodegeneration is not well understood. Studying MGCs and their role in CR regulation may help understanding the relationship between CR and AD progression. This review presents evidence that CR disruption occurs in AD MGCs based on a) current knowledge of AD and MGCs, and b) similarities between AD and other retinopathies. This review focuses on MGCs, but it should be noted that intrinsically photosensitive retinal ganglion cells (ipRGCs) entrain our twenty-four circadian clocks (Aranda and Schmidt, 2021). Although the morphology and function of various ipRGC subtypes in humans is under active investigation (Mure, 2021), their function in murine models (Contreras et al., 2021) has established their importance in circadian regulation and general wellbeing. Lax et al. (2019) has reviewed the connection between ipRGC alterations, neurodegenerative diseases and circadian disorders. To date, important TRP channels associated with ipRGCs (e.g., TRPC6 and TRPC7) and MGCs (e.g., TRPV4) appear to be distinct (Križaj et al., 2023). Furthermore, ipRGCs and their associated neural circuitry are believed to be important elements in the effect of light on human and murine cognition (Mahoney and Schimidt, 2024). ipRGCs are more resilient to injury than other RGCs in murine retinal damage models while number of ipRGC’s declines in humans with increased age (Zhu et al., 2022). La Morgia et al. (2016) observed significant ipRGC cell death and Aβ accumulation in post-mortem human AD retinas, although this did not necessarily correlate with severity of sleep-wake cycle disruption. Still, this is further indication that retinal circadian dysfunction is a characteristic of AD pathology.
MGCs and ocular Aβ clearance
Aβ accumulation in AD retinas (Gupta et al., 2016; Lee et al., 2020) may prompt MGC gliosis. For example, Xu et al. (2016) found microglial-dominant gliosis and decreased MGC metabolic activity in AD postmortem retinas. However, most studies indicate MGC-dominant gliosis. Blanks et al. (1996) was the first to report MGC gliosis and increased number of MGC processes in AD patients. Walsh et al. (2002) found that injecting rat vitreous humors with Aβ induced MGC gliosis. However, the MGCs did not uptake any Aβ. These results were later contradicted by den Haan et al. (2018), who found Aβ deposits in MGCs. These contrasting findings suggest that Aβ acts through both direct and indirect pathways to induce MGC gliosis. Dinet et al. (2012) found that MGC gliosis diminishes their ability to maintain water homeostasis in murine retinas. One day after subretinal injection of the highly toxic Aβ isoform, Aβ(1–42), multiple gliosis and inflammatory markers were upregulated. Three days post-injection, retinal edema and transient AQP4 upregulation were observed. Altered distribution of fluorescently labeled Kir4.1 in MGCs was also observed. Kir4.1 is an inwardly rectifying potassium channel that regulates MGC osmo-homeostasis. Kir4.1 downregulation is observed in retinal ischemia (Liu et al., 2007; Milton and Smith, 2018). In healthy control retinas, Kir4.1 was localized in the inner limiting membrane, as well as near blood vessels in the inner and outer nuclear layers. This polarization was lost in Aβ(1-42)-treated retinas. Similar Kir4.1 delocalization is associated with reactive gliosis in proliferative retinopathies (Bringmann et al., 2009). These findings suggest that Kir4.1 expression in MGCs may be an interesting topic of further study in relation to AD. Treating retinas with indomethacin, an anti-inflammatory drug, prior to Aβ(1–42) injection restored Kir4.1 distribution. However, indomethacin failed to reduce overall retinal edema. These findings suggest that retinal edema occurs independently of Kir4.1 and inflammatory processes. It should also be noted that there are conflicting studies regarding whether or not Kir4.1 dysregulation contributes to AD brain pathology (Huffels et al., 2022; Froggett et al., 2024). Still, MGC swelling and gliosis due to Kir4.1 dysregulation should be investigated in AD retinas, especially considering the independence of retinal and cerebral CR regulation. MGC structural changes are especially important to study with respect to waveguide function, which can be disrupted by either a change in shape or composition.
Kir4.1 expression is under circadian control. Studies have shown that adenosine monophosphate-activated protein kinase (Alex et al., 2020) and insulin receptor substrate-1 (Luo et al., 2019) regulate Bmal-1 control of KCNJ10 expression. KCNJ10 encodes for a member of the Kir4.1 channel family. According to Hassan et al. (2017), Bmal-1 and KCNJ10 oscillations are in phase with each other in clock synchronized MGCs. Hassan et al. showed that in diabetic rat retinas, damped KCNJ10 oscillations correlated with MGC swelling in diabetes. Luo et al. (2019) similarly found a loss of Bmal-1 gene expression and Kir4.1 protein density rhythmicity that correlated with MGC swelling in diabetic mice. Luo et al. also found a loss of Kir4.1 polarization, similar to what Dinet et al. found in Aβ-treated murine retinas. The similarities in these findings suggest that MGCs swelling in AD retinas may be due to Kir4.1 delocalization and CR disruption. Interestingly, Alex et al. (2020) found that metformin, the gold-standard diabetes drug, corrects MGC dysfunction in diabetic mouse models by restoring Kir4.1 and Bmal-1 rhythms. Studies that evaluate if MGCs exhibit diurnal swelling patterns in human AD retinas may be useful.
The recent discovery of an ocular glymphatic system likely connect MGCs and Aβ-induced retinal pathologies. Wang et al. (2020) identified a glymphatic pathway in mice for Aβ clearance. Fluorescently tagged Aβ was injected into the vitreous humor and cisterna magna, part of the glymphatic system in the brain. Aβ was anterogradely transported along the optic nerve, accumulates in the perivascular spaces, and then drains into dural lymphatic vessels. AQP4 facilitates clearance in MGCs and astrocytes. Impaired ocular glymphatic clearance may cause retinal edema. These processes mirror glymphatic transport in the brain.
Wang et al. (2020) found that retinal light exposure dramatically accelerated Aβ transport along the optic nerve. Treatment with atropine and pilocarpine to hinder the pupillary light response diminished Aβ transport. These results indicate that the mechanical forces created by pupillary constriction accelerates fluid flow through the ocular glymphatic system, thereby aiding Aβ clearance. Interestingly, AD patients exhibit a more sluggish pupillary light response (Chougule et al., 2019). One might hypothesize that this slower pupillary contraction may be related to the decrease in ocular Aβ glymphatic clearance. Further research might explore if increasing retinal light exposure accelerates ocular glymphatic fluid transport and Aβ clearance.
Interactions between MGCs and microglia are another mechanism for Aβ clearance. Microglia are the yolk sac-derived primary macrophages of the retina (Ginhoux et al., 2013). Hoh Kam et al. (2010) showed that Aβ accumulates in Bruch’s membrane and the outer segments of photoreceptors in normal aging. Severe Aβ-induced tissue damage induces microglia proliferation and osteopontin-mediated phagocytic activity to remove Aβ and other mineralized debris. During reactive gliosis, MGCs and microglia amplify each other’s phagocytic activities (Caicedo et al., 2005; Bringmann et al., 2006). MGCs perform osteopontin-mediated phagocytosis of large particles (Kumar et al., 2013). Interestingly, Wahl et al. (2013) found that osteopontin is upregulated in edematous MGCs in response to hypoosmolality caused by Kir4.1 downregulation. As previously mentioned, Kir4.1 downregulation has been observed in AD murine retinas. Osteopontin activates an autocrine cascade that opens potassium channels to restore osmolarity. Ample evidence indicates that Aβ accumulation in the retina corresponds with Aβ in the brain and occurs at higher levels than during normal aging (Ratnayaka et al., 2015; Lee et al., 2020). Phagocytic activity is known to contribute to retinal neurodegeneration both via direct tissue damage and release of inflammatory factors. Hence, further research might investigate a) if MGCs demonstrate osteopontin-mediated Aβ phagocytosis, and b) if this phagocytic activity contributes to retinal neurodegeneration in AD.
Da Mesquita et al. (2021) found in 5xFAD transgenic mice that meningeal T cells in middle-aged mice demonstrated CCR7 downregulation compared to younger mice. CCR7KO mice displayed increased regulatory T cell responses, decreased CSF flow, increased Aβ accumulation, and poorer spatial learning and memory. This finding suggests immune mechanisms link MGCs with poor ocular Aβ clearance. More research is needed on this topic.
The most direct evidence for the roles of MGCs and CR dysfunction in AD retinopathy comes from Carrero et al. (2023), which found CR-dependent downregulation of aquaporins in APP/PS1 mice. AQP1, AQP4, and AQP5 were remarkably reduced in 6- and 12-month-old mice. Aquaporin downregulation positively correlated with disrupted clock gene rhythms and Aβ retinal accumulation. These findings strongly suggest that MGC function in ocular glymphatic clearance is impaired in AD retinopathy due to CR changes.
Optical coherence tomography (OCT) studies of MGCs
Extensive research indicates that the retina is a “window to the brain” that can serve for noninvasive study of neuropathies. The brain and the retina share extensive neural and vascular connections. Neurodegenerative diseases, including AD, often present with retinal complications that are direct reflections of brain pathology. Both time-domain OCT (Ascaso et al., 2014) and spectral domain OCT (SD-OCT) (Chan et al., 2019; Jáñez-Escalada et al., 2019) studies reveal thinner retinal nerve fiber layers in AD and mild cognitive impairment (MCI)—the stage preceding AD symptom presentation. OCT image meta-analysis by den Haan et al. (2017) found that retinal nerve fiber layer thickness differences between AD and healthy controls are more pronounced using time domain OCT. SD-OCT is more commonly utilized due to its faster acquisition times, higher signal-to-noise ratio, and superior resolution. OCT imaging of retinal layers can give insights into AD pathology.
Previous research on the inner nuclear layer (INL), where MGC cell bodies reside, gives some clues on the relationship between neurodegenerative disease and MGCs. Many OCT studies of the INL have focused on multiple sclerosis. In multiple sclerosis patients, studies have found INL thickening (Knier et al., 2016; Balk et al., 2019) and microcystoid structures indicating edema (Gelfand et al., 2012; Lujan and Horton, 2013; Sigler, 2014). BRB dysfunction (Birch et al., 1996) and MGC gliosis (Green et al., 2010) have also been observed in multiple sclerosis retinopathies. As previously discussed, edema, BRB dysfunction, and gliosisare also features of AD retinas. Both types of changes are correlated to MGC inflammation and trauma-induced cell damage.
Another reason the INL is interesting is that most retinal circadian activity happens in this layer–not surprising given the presence of MGC cell bodies. INL thinning and nuclear loss is observed in AD patients (Koronyo et al., 2017). Baba et al. (2018) found that INL thinning occurs in Bmal-1KO mice. Savaskan et al. (2007) found that the INL has a high concentration of MT2 melatonin receptors, and MT2 receptor expression is decreased in AD patients’ retinas. The INL is also one of the retinal layers with greatest Aβ and phosphorylated tau deposition (Hart et al., 2016). This finding is curious considering the evidence pointing to altered CR and poor Aβ clearance by AD MGCs. INL thinning and edema can be further imaged and studied to understand better the relationship between AD and MGCs.
Bissig et al. (2020) found that retinal light responses can be used as a proxy for studying changes in MGCs during early AD. SD-OCT images showed that the decrease in outer nuclear layer reflectivity (ONL) upon light exposure from a white LED was more pronounced in AD retinas. AD also affected the ONL slow intrinsic optical signal. The ONL is an avascular layer containing primarily MGCs and photoreceptors. MGCs are the only cells that span the entire thickness of the retina, so light reflectivity changes in the overall retina may result from altered MGC function. To examine how MGCs affect retinal reflectivity, AD retinas were compared to neuromyelitis optica retinas. Neuromyelitis optica results from an autoimmune attack against AQP4. Neuromyelitis optica retinas showed a 1.5% reflectivity decrease—a small but significant change. This suggests that immune reactivity against AQP4 and resulting loss of water homeostasis directly alters light reflectivity. AD and neuromyelitis optica retinas showed reflectivity changes in the same layers. ONL reflectivity changes in response to optical excitation indicate altered MGC water homeostasis in AD.
SD-OCT is capable of directly detecting individual MGCs. Chung and Byeon (2017) found that MGCs surrounding the fovea appear Z-shaped. Vitreous traction distorts this Z-shape. Notably, MGC waveguide function may be of less significance in the fovea because a) the fovea almost entirely consists of cones, and b) simulations by Szabo et al. (2022) suggest that typical incidence angles and spot sizes in the retina render additional waveguide function unnecessary. Many more SD-OCT studies visualizing MGCs outside of the fovea and across all retinal layers may aid the understanding of the mechanistic links between CR, AD, and MGCs.
Similarities between retinal neurodegeneration in AD and diabetic retinopathy (DR)
Insulin resistance precedes symptom presentation in both type 2 diabetes and AD. There is a strong link between disturbed CR and insulin resistance. The SCN and peripheral tissue oscillators control glucose metabolism and secretion of metabolic hormones (Stenvers et al., 2019). Insulin-degrading enzyme breaks down Aβ. Hyperinsulinemia saturates insulin-degrading enzyme, thereby hindering Aβ clearance (Shiiki et al., 2004; Ferreira et al., 2018). Conversely, Aβ oligomers are thought to induce cerebral insulin resistance by causing insulin receptor internalization (Zhao et al., 2008; Bomfim et al., 2012; Molina-Fernández et al., 2022).
DR affects approximately one-third of diabetic patients worldwide (Wong and Sbanayagam, 2020). DR is one of the best studied diseases in relation to MGC pathophysiology. Because type 2 diabetes is a known risk factor for AD, exploring MGC-related retinal neurodegeneration pathways common to both may be worthwhile.
Kir4.1 dysregulation in MGCs is a striking similarity between AD and DR. As discussed previously, Dinet et al. (2012) established that altered Kir4.1 expression is not solely responsible for overall retinal edema in Aβ(1-42)-treated retinas. However, similarities between diabetes and AD MGCs in terms of Kir4.1 localization suggest that MGC swelling may be observed in AD retinas. Diminished Kir4.1 rhythmicity causes MGC swelling in diabetic retinas—does the same happen to AD retinas? Hassan et al. (2017) noted the greatest difference in KCNJ10 expression between control and diabetic rat MGCs correlates with the largest degree of MGC swelling. As discussed previously, Bmal-1 regulates KCNJ10 diurnal rhythms. Bmal-1 is also thought to regulate AQP4 expression. Investigating diurnal variations in MGC swelling may provide insights about the role of CR core clock genes in mediating AD retinopathy.
Similarities in mitochondrial dynamics between AD and DR
There is evidence that mitochondrial dysfunction contributes to retinal neurodegeneration in AD. Gardner et al. (2020) found that retinas of 3xTg mouse models of AD have different Scattering angle-resolved OCT (SAR-OCT) C-parameters than control retinas. OCT C-parameters represent the angular distribution of back-scattered light. AD retina C-parameters started at lower values and decreased more slowly versus age-matched controls. Gardner et al. associates the difference in OCT C-parameters to Aβ interference in mitochondrial function and resulting neuron loss. Bevan et al. (2020) found swelling and beading of retinal ganglion cells indicative of mitochondrial collapse (Greenwood et al., 2007) in 3xTg mice. These changes corresponded to loss of dendritic spines in the hippocampus.
Gardner et al. (2019) found altered retinal OCT C-parameters in murine models due to hypoxia-induced neuron death. Mitochondrial function and hypoxia are closely linked to CR. Communication between the Bmal-1 and HIFɑ genes modulate hypoxia signaling (Wu et al., 2017). HIFɑ upregulation during hypoxia increases mitochondrial fission, thereby promoting apoptosis (Zhang et al., 2018). Mitochondrial fission-fusion dynamics are linked to CR via circadian DRP1 gene oscillations (Leong, 2018). Studies have indicated that hypoxia affects functioning of AD and MCI retinas. Retinal oximetry studies indicate increased arterial oxygen saturation and decreased arteriovenous difference in AD and MCI retinas (Einarsdottir et al., 2016; Olafsdottir et al., 2018), indicating poor delivery of oxygen to retinal tissues. Li et al. (2018) found that hypoxia induces Aβ accumulation, and consequent mitochondrial oxidative stress, in retinal ganglion cells in vitro. Hypoxia is implicated in tau hyperphosphorylation in APP/PS1 transgenic mice retinas (Wang et al., 2013; Zhao et al., 2013). Investigating the circadian dynamics of hypoxia-induced retinal neurodegeneration in AD may be useful.
Based on similarities with DR retinas, mitochondrial dysfunction may occur specifically within MGCs affected by AD. In animals with vascularized retinas, such as humans, mitochondria are found throughout the length of MGCs, with the highest concentration at the endfeet (Lefevere et al., 2017). In animals with non-vascularized retinas, such as cows, mitochondria localize in areas with high cytoplasmic oxygen partial pressure (Germer et al., 1998; Lefevere et al., 2017). AD and MCI retinas show decreased blood vessel density and perfusion (Cheung et al., 2014; Czakó et al., 2020). Whether hypoxia and microvasculature changes alter MGC mitochondrial localization is an interesting question. Hypoxia is a known contributor to DR pathophysiology (Arden and Sivaprasad, 2011; Ramsey and Arden, 2015). In DR, mitochondrial oxidative stress induces MGC swelling (Pannicke et al., 2006; Krügel et al., 2011). Mitochondrial assays and SAR-OCT studies may determine if hypoxia contributes to the MGC swelling observed in AD retinas.
Another mitochondrial similarity between AD and DR involves thioredoxin interacting protein (TXNIP) signaling. TXNIP functions as a negative regulator of thioredoxin-1 and thioredoxin-2 to maintain a balanced redox state. Under high glucose conditions, TXNIP mediates mitophagy and oxidative stress in the retina (Devi et al., 2012; Devi et al., 2017). In AD brains, mitophagy is thought to be neuroprotective by clearing Aβ and tau (Fang et al., 2019; Kshirsagar et al., 2022). TXNIP is well studied for its contribution to insulin resistance in AD brains (Nasoohi, Parveen, and Ishrat, 2018). TXNIP overexpression in the retina has been implicated in increased oxidative stress and resulting neurodegeneration (Al-Gayyar et al., 2011). Lamoke et al. (2012) found increased TXNIP expression and decreased thioredoxin-1 activity in APP/PS1 transgenic mice. These changes in the thioredoxin system were associated with increased MGC GFAP expression. This finding suggests that thioredoxin system dysfunction may explain Aβ-induced gliosis. In Sprague-Dawley rodent models of DR, TXNIP induces MGC autophagy and apoptosis (Ao et al., 2021). TXNIP knockdown improved the visual light response in DR mice. Whether TXNIP knockdown in AD rat models similarly enhances the visual light response is an interesting question. Enhanced TXNIP signaling on mitophagy may be one connection between insulin resistance, AD progression, and retinal gliosis.
SIRT1 downregulation is a common pathway explaining mitochondrial dysfunction in both AD brains and DR retinas. SIRT1 is a NAD-dependent deacetylase that acts on a variety of proteins to regulate gene expression, metabolism, and mitochondrial activity. In the SCN, SIRT1 binds directly to the Bmal-1 promoter. Chang and Leonard (2013) found that SIRT1 is downregulated in aging mouse SCNs–a possible explanation for the link between AD and altered sleep-wake cycles. This explanation can be supported by Zhou et al. (2016), who found an 8 hour phase advance of SIRT1 and phase advance of Bmal-1, Per-1, and Per-2, in ApoE−/− mouse SCNs. Zhou et al. also found decreased SCN NAD+/NADH ratios attributable to downregulated nicotinamide phosphoribosyltransferase because of Bmal-1 dysfunction. SIRT1 interacts with PGC-1α to induce neuroprotective mitogenesis in a variety of brain disorders, from Parkinson’s disease to epilepsy to ischemic stroke (Chen et al., 2018; Chuang et al., 2019; Gao, Quian, and Wang, 2020; Chen et al., 2021). Jia et al. (2023) found that thioredoxin-1 promotes this process in vitro hippocampal neurons and in vivo AD rat models. Panes et al. (2020) found that SIRT1-induced mitogenesis is compromised by Aβ oligomers in vitro. SIRT1 is directly neuroprotective against tau (Min et al., 2018; Yin et al., 2022) and Aβ (Chen et al., 2005; Li et al., 2018). Likewise, SIRT1 mitigates MGC gliosis in DR models (Zhang et al., 2018; Kong and Zhang, 2021; Adu-Agyeiwaah, 2023). Ahmad et al. (2021) found that NADPH oxidase four induces reactive oxygen species which deplete SIRT1 in vitro hypoxic MGCs and in vivo mouse models of DR. SIRT1 control of mitochondrial dynamics and oxidative stress is a potential link between CR and AD-associated retinopathy, based on our knowledge of DR.
Melatonin neuroprotectively upregulates SIRT1 expression in AD and DR. In their review, Li et al. (2020) suggests that melatonin promotes Aβ degradation by ADAM10 by upregulating SIRT1 further upstream. Multiple in vitro and in vivo studies indicate an epigenetic pathway linking melatonin to SIRT1 upregulation in DR MGCs (Tu et al., 2020; Kong and Zhang, 2021; Zhang et al., 2018). In another review, Djordjevic et al. (2021) posits melatonin as an option to resolve BRB dysfunction in DR, with SIRT1 modulation as a possible therapeutic mechanism. Given the similarities between DR and AD pathologies, melatonin-SIRT1 signaling is an interesting neuroprotective topic of study in AD retinopathy. More studies investigating the neuroprotective relationship between melatonin and mitochondrial dynamics in AD retinopathy are warranted.
Similarities between retinal neurodegeneration in AD and age-related macular degeneration (AMD)
Conflicting research exists on whether a correlation exists between AMD and AD incidence rates (Williams et al., 2015; Smilnak et al., 2019; Wen et al., 2021). However, their pathophysiology shares many similarities. Aβ is implicated in both, but AMD drusen formation involves non-fibrillar Aβ while AD recruits fibrillar Aβ for plaque formation. Both non-fibrillar and fibrillar Aβ are derived from the same nonfibrillar amyloidogenic precursor oligomers. Drusen-associated vesicles contain reactive WO antibodies, which are present in mature AD cerebral plaques (Çerman et al., 2015). MGC dysfunction is heavily involved in AMD. Pilotto et al. (2019) noted increased INL thickness owing to MGC swelling and hypertrophy as visualized by OCT studies of AMD patients. Kim et al. (2021) observed MGC activation and migration in the outer retina for 5 days following subretinal injection of human lipid hydroperoxide to induce AMD in rats. These similarities suggest that our current knowledge on AMD may help predict what happens to AD MGCs.
A BRB protein that indicates dysregulated CR in dry AMD is claudin-5. Hudson et al. (2019) identified that claudin-5 expression is regualted by Bmal-1. OCT imaging of claudin-5 knockdown mice showed geographical atrophy lesions resembling dry AMD. Similar outcomes were shown in AAV-mediated claudin-5 inhibition in monkey maculae. Claudin-5 is the most enriched BBB protein and is thought to be neuroprotective in AD (Greene, Hanley, and Campbell, 2019; Zhu et al., 2022). Similarities between the AD brain and dry AMD retina suggest a common CR-controlled mechanism that may present in AD retinopathy.
Aberrant VEGF expression in wet AMD indicates MGC circadian dysfunction. Intravitreal injection of Aβ(1–42), but not the more common and less toxic variant Aβ(1–40), increases VEGF in the retinal pigmented epithelium of AMD retinas (Liu et al., 2013). Antes et al. (2015) found that following laser-induced retinal injury, mice expressing human Apolipoprotein E4 (apoE4) experienced a greater increase in VEGF and resultant neovascularization than apoE3 and control mice. ApoE4 is the strongest known genetic predictor of AD. Zhou et al. (2016) found that in the SCNs of ApoE−/− mice, expression of the clock genes Bmal1, Per1, and Per2 show phase advance and greater variation compared to control mice. In hypoxic MGCs, Per1 and Per2 downregulation increases VEGF production. Per1 controls Per2 cyclical expression patterns, and Per1 is required for maintaining CR in MGCs (Xu et al., 2016). The relationship between SCN and MGC physiology suggests that AD induces VEGF overproduction in both. Future research might explore how Aβ and ApoE affect MGC production of VEGF.
An interesting relationship exists between the BRB protein occludin and Aβ. Park et al. (2020) found retinal pathology resembling dry-type AMD in 5xFAD mouse models of AD. The breakdown of the retinal pigment epithelium was specifically associated with Aβ(142)-induced occludin degradation. MGCs produce matrix metalloproteinases (MMPs), namely, MMP-9, that degrade occludin. MMP-9 expression in the retina is altered in exudative AMD (Fiotti et al., 2005; Liutkeviciene et al., 2015; Lauwen et al., 2021) and DR (Kowluru, 2010) retinas. MMP-9 degradation of occludin is observed in DR. Non-exudative AMD often converts into wet-type AMD if untreated. It would be interesting to explore how Aβ(142) affects MMP-9 production in AD retinas, and if overproduction of MMP-9 by MGCs is a common link between AD, DR, and the transformation of dry-type into wet-type AMD.
BRB dysfunction and resulting albumin leakage are potential links between AMD and AD. Schultz et al. (2019) found increased retinal albumin in a post-mortem study of dry-type AMD retinas. Anderson et al. (2008) found that Aβ(1-42)-injected retinas experienced increased leakage of albumin from retinal blood vessels. It would be interesting to see if a connection exists between Kir4.1 downregulation in AD MGCs and leaked albumin. Löffler et al. (2010) reported that retinal albumin leakage induces MGC swelling in healthy rodent retina slices. Ivens et al. (2007) found that Kir4.1 was downregulated in normal rat brain slices exposed to albumin. Frigerio et al. (2012) found that intracerebroventricular injection of albumin into normal rat brains induced Kir4.1 downregulation in the hippocampus in vivo. How the effects of albumin on Kir4.1 would change in a diseased retina is unclear. No changes in Kir4.1 or AQP4 expression were found in a post-mortem study of AMD retinas (Madigan et al., 2014)— different from AD retinas and brains. This is quite interesting given that Aβ retinal accumulation occurs in both diseases. Future research might explore a) if albumin leakage from retinal microvasculature occurs in AD, and b) how albumin affects Kir4.1 expression patterns in AD.
Similarities between retinal neurodegeneration in AD and glaucoma
AD and glaucoma pathology share several similarities. Yan et al. (2017) found elevated levels of Aβ(1–42) and phosphorylated tau in the lateral geniculate nucleus in a Rhesus monkey model of chronic glaucoma. MGC gliosis happens in glaucoma (Graf et al., 1993; Guo et al., 2010). MGC physiology changes observed in glaucoma could give insights into what happens in AD.
Guo et al. (2010) found that chloride ion channel CLIC1 is upregulated over four-fold in a rat glaucoma model. Interestingly, Maraschi et al. (2023) found that CLIC1 activates MGCs in response to Aβ accumulation in vitro. More research is needed to understand the function of CLIC1 in healthy, glaucoma, and AD retinas.
Extracellular ATP release is a common response to mechanical stress throughout the human body, such as the pressure on MGCs resulting from increased intraocular pressure in glaucoma. Multiple studies of human glaucoma patients have indicated increased ATP release into the aqueous humor (Zhang et al., 2007; Li et al., 2018). Lu et al. (2015) found increased extracellular ATP concentration in the vitreous humors of rodent and primate models of glaucoma, as well as altered purinergic signaling. Excess ATP stimulates P2X7 purinergic receptors (P2X7R) on retinal ganglion cells, causing cell death. Xue et al. (2016) found that both extracellular ATP and MGC activation upregulates P2X7R in a rodent glaucoma model. This suggests that activated MGCs may cause ganglion cell death by releasing ATP. In healthy mammalian retinas, there is bidirectional purinergic communication between MGCs and ganglion cells (Newman, 2006). ATP released by MGCs hyperpolarizes ganglion cells. ATP released by ganglion cells amplifies calcium potentials in MGCs upon flickering light exposure. In an APP/PS1 mouse model of Alzheimer’s disease, retinal extracellular ATP increased in the first 14 months, then decreased to normal in the following 18 months (Perez de Lara and Pintor, 2015). Exploring how changing extracellular ATP levels in AD retinas affects MGC activity, and thereby affects the rate of retinal ganglion cell death may be beneficial. Light exposure is involved in MGC-ganglion ATP signaling, so investigating this pathway may also provide insight into how CR affects retinal ganglion death.
Interestingly, BRB disruption in glaucoma could serve a neuroprotective function. Keaney et al. (2015) found that siRNA inhibition of occludin and claudin-5, another essential blood-retina barrier (BRB) protein, in DBA/2J glaucomatous mice decreased the retina/plasma ratio of Aβ(1–40). Aβ(1–40) loss indicates diminished BRB function that may potentially enhance Aβ clearance. Enhanced Aβ(1–42) diffusion across the BRB was also observed. Exploration of whether limited BRB opening in AD retinas may be neuroprotective may be useful. A research area that has gained interest is the application of transcranial therapeutic ultrasound in AD patients for opening the BBB to accelerate Aβ clearance (Lustbader et al., 2004; Rezai et al., 2020). Understanding more about BRB opening in glaucoma can help better understand how MGCs behave in AD.
Glaucoma has been specifically linked to non-proliferative gliosis. Inman and Horner (2007) found that reactive gliosis in DBA/2J mice was not accompanied by MGC proliferation. Bolz et al. (2018) found that DBA/2J mouse models of glaucoma did not experience a change in MGC potassium currents, which indicates that rapid proliferation does not occur. Since previous studies have found potassium current alterations in AD MGCs, suggests that gliosis in AD retinas is not limited to the non-proliferative kind. More research that quantifies the rate of MGC proliferation in AD retinas versus other retinal conditions may be beneficial.
Light-damaged animal retinas as putative models of MGC gliosis in AD
Sambamurti et al. (2007) found that prolonged exposure to both low and high-intensity light caused APP C-terminal fragments to accumulate in the vitreous humors of BALBC mice. This is the only report describing a light-induced retinal amyloidosis model. However, further development of this model may contribute to our understanding of the effects of artificial light on the retina. As discussed throughout the paper, AD is associated with CR dysfunction. Blue light is especially important for CR synchronization. Blue light daytime therapy for AD has gained increasing interest as a research topic. Blue light’s negative effects on CR, however, especially regarding nighttime exposure and how that may contribute to AD is poorly understood. Such a model could be developed and studied with respect to MGC physiology.
MGC gliosis contributes to light-induced retinal degeneration chracterized by CR disruption. Chan et al. (2023) observed BRB breakdown in mice after prolonged low-level blue light exposure that correlated with altered electroretinogram signals. In vitro culture of human retinal microvascular endothelial cells suggest these results are due to claudin-5 degradation by metalloproteinase ADAM17. They attribute ADAM17 hyperactivity to failure of ADAM17 sequestration by GNAZ, a blue-light and CR-sensitive retinal G protein. Iandiev et al. (2008) characterized rat MGC alterations due to 30 minutes of intense blue light exposure. They found increased GFAP, MGC swelling, loss of Kir4.1 polarization, and loss of AQP1. Interestingly, AQP4 was upregulated in the outer regulatory layers, further contributing to MGC swelling. Bruera et al. (2022) found that Wistar rodent MGCs undergo marked gliosis after 6 days of low-level light exposure. This was a late response compared to the deaths of photoreceptors within the first 4 days, indicating that MGC gliosis is a secondary response. Kang et al. (2021) found bright light induced MGC gliosis in mouse retinas after only 8 hours of exposure. Subsequently, another group of mice were exposed to bright light for 7 days. These 7-day bright light-damaged MGCs exhibited miRNA profiles resembling DicercKO mice. Dicer is an enzyme required for post-translational processing of most miRNAs. Interestingly, DicercKO mice exhibit retinal layer disorganization resembling retinitis pigmentosa. Seven-day bright light-damaged and DicercKO mice both feature MGC cell body migration to the outer nuclear layer. In both groups of mice, the center of the retina is thinned due to photoreceptor death. Only DicercKO mice retinas also have dilated and stretched areas. Despite this difference in retinal layer structure, both sets of MGCs upregulate the Atf3 and Egr2 genes that normally protect from retinal stretching (Wang et al., 2013; Kole et al., 2020). Interestingly, Atf3 and Egr2 are upregulated even though thinning, not stretching, results from bright light damage.
Atf3 is an interesting gene to study with respect to MGCs and AD. The Atf gene family is thought to have a causative role in AD (Yang et al., 2023). Atf3 is an inducible transcription factor that regulates cellular stress responses and reduces neuroinflammation. Atf3 is not highly expressed in neurons, except during axonal regeneration. Heinrich et al. (2018) found that Atf3 downregulates acetylcholinesterase to prevent apoptosis in the retinal inner nuclear layer (INL), the layer containing MGC cell bodies. The INL is thinned in AD patients. Aβ and APP accumulate in the INL of AD animal models and human patients (den Haan et al., 2018; Maran et al., 2023). Acetylcholinesterase forms complexes with Aβ that worsen amyloid-induced neurotoxicity (Alvarez et al., 1997; Alvarez et al., 1998; Melo et al., 2003). The neuroprotective role of MGC-expressed Atf3 against retinal Aβ deposition may be a research topic that provides useful insights.
Discussion
As summarized in Table 1, MGC dysfunction is a potential link between AD, CR, and retinal neurodegeneration. CR dysregulation is directly involved in multiple pathways related to gliosis and AD. Impaired ocular Aβ clearance—both glymphatic and macrophagic—induces gliosis and Kir4.1 downregulation, causing hypo-osmolarity and swelling. SD-OCT imaging studies reveal INL thinning in AD retinas which can be attributed to MGC cell death. The ONLs of AD retinas exhibit altered light reflectivity responses resembling that of neuromyelitis optica, an autoimmune attack against the channel responsible for glymphatic clearance of AD-associated proteins, AQP4. Similarities in AD and DR retinas indicate that insulin resistance is common to both. Such similarities include loss of Kir4.1 rhythms and mitochondrial dysfunction. Pharmacological agents, such as metformin, that target Kir4.1 rhythmicity might be investigated for their therapeutic potential in AD retinopathy. OCT studies of mitochondrial dysfunction in AD retinas may beneficially look for fission, altered distribution throughout the lengths of the MGC fibers, and hypoxia. Based on findings in AMD, research in AD retinas should investigate VEGF expression, occludin degradation, and albumin leakage due to BRB dysfunction. Similarities between AD and glaucoma indicate that CLIC1 expression and extracellular ATP signaling may represent directions for mechanistic research. Also, of interest may be to investigate a chronotherapeutic approach of intentionally opening the BRB to allow faster Aβ clearance. Because regular aging alters retinal physiology, and because multiple retinal diseases are comorbid with AD, care should be taken to ensure the observed MGC changes are specifically correlated to AD. Still, these findings indicate that a) optical studies of MGCs can reflect MCI/AD changes in the brain, and b) a chronotherapeutic approach may help resolve AD-associated retinopathy.
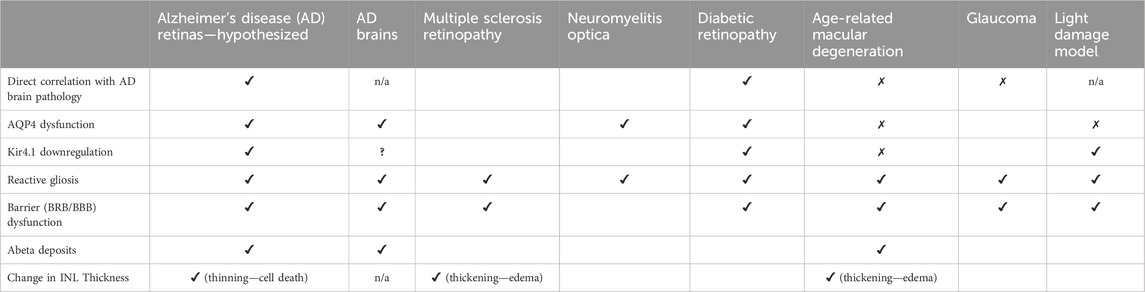
Table 1. Comparison of hypothesized pathological features of AD retinopathy vs other conditions as discussed in this review.
Author contributions
GD: Conceptualization, Investigation, Writing–original draft, Writing–review and editing. TM: Conceptualization, Supervision, Writing–review and editing.
Funding
The author(s) declare that no financial support was received for the research, authorship, and/or publication of this article.
Acknowledgments
The authors thank Hong Zhao, MD, PhD; Jianrong Li, DVM, PhD; and Stephanie Dolores Kirk, BS, for their feedback and encouragement during the writing process.
Conflict of interest
The authors declare that the research was conducted in the absence of any commercial or financial relationships that could be construed as a potential conflict of interest.
The author(s) declared that they were an editorial board member of Frontiers, at the time of submission. This had no impact on the peer review process and the final decision.
Publisher’s note
All claims expressed in this article are solely those of the authors and do not necessarily represent those of their affiliated organizations, or those of the publisher, the editors and the reviewers. Any product that may be evaluated in this article, or claim that may be made by its manufacturer, is not guaranteed or endorsed by the publisher.
References
Abukawa, H., Tomi, M., Kiyokawa, J., Hori, S., Kondo, T., Terasaki, T., et al. (2009). Modulation of retinal capillary endothelial cells by Müller glial cell-derived factors. Mol. Vis. 15, 451–457.
Achariyar, T. M., Li, B., Peng, W., Verghese, P. B., Shi, Y., McConnell, E., et al. (2016). Glymphatic distribution of CSF-derived apoE into brain is isoform specific and suppressed during sleep deprivation. Mol. Neurodegener. 11 (1), 74. doi:10.1186/s13024-016-0138-8
Adu-Agyeiwaah, Y., Vieira, C. P., Asare-Bediako, B., Li Calzi, S., DuPont, M., Floyd, J., et al. (2023). Intravitreal administration of AAV2-SIRT1 reverses diabetic retinopathy in a mouse model of type 2 diabetes. Transl. Vis. Sci. Technol. 12 (4), 20. doi:10.1167/tvst.12.4.20
Agte, S., Junek, S., Matthias, S., Ulbricht, E., Erdmann, I., Wurm, A., et al. (2011). Müller glial cell-provided cellular light guidance through the vital Guinea-pig retina. Biophysical J. 101 (11), 2611–2619. doi:10.1016/j.bpj.2011.09.062
Ahmad, A., Nawaz, M. I., Siddiquei, M. M., and Abu El-Asrar, A. M. (2021). Apocynin ameliorates NADPH oxidase 4 (NOX4) induced oxidative damage in the hypoxic human retinal Müller cells and diabetic rat retina. Mol. Cell. Biochem. 476 (5), 2099–2109. doi:10.1007/s11010-021-04071-y
Al-Ahmad, A. J., Patel, R., Palecek, S. P., and Shusta, E. v. (2019). Hyaluronan impairs the barrier integrity of brain microvascular endothelial cells through a CD44-dependent pathway. J. Cereb. Blood Flow Metabolism 39 (9), 1759–1775. doi:10.1177/0271678X18767748
Alex, A., Patel, R., Mathew, D., and Bhatwadekar, A. D. (2020). Metformin corrects abnormal circadian rhythm and KIR4.1 channels in diabetes. Investigative Ophthalmol. Vis. Sci. 61 (6), 46. doi:10.1167/IOVS.61.6.46
Al-Gayyar, M. M. H., Abdelsaid, M. A., Matragoon, S., Pillai, B. A., and El-Remessy, A. B. (2011). Thioredoxin interacting protein is a novel mediator of retinal inflammation and neurotoxicity. Br. J. Pharmacol. 164 (1), 170–180. doi:10.1111/j.1476-5381.2011.01336.x
Alvarez, A., et al. (1997). Acetylcholinesterase promotes the aggregation of amyloid-(beta)-peptide fragments by forming a complex with the growing fibrils.
Alvarez, A., et al. (1998). Stable complexes involving acetylcholinesterase and amyloid-peptide change the biochemical properties of the enzyme and increase the neurotoxicity of Alzheimer’s fibrils.
Ancoli-Israel, S., Gehrman, P., Martin, J. L., Shochat, T., Marler, M., Corey-Bloom, J., et al. (2003). Increased light exposure consolidates sleep and strengthens circadian rhythms in severe Alzheimer’s disease patients. Behav. Sleep. Med. 1 (1), 22–36. doi:10.1207/S15402010BSM0101_4
Anderson, P. J. B., Watts, H. R., Hille, C. J., Philpott, K. L., Clark, P., Gentleman, M. C. S., et al. (2008). Glial and endothelial blood-retinal barrier responses to amyloid-beta in the neural retina of the rat. Clin. Ophthalmol. 2 (4), 801–816. doi:10.2147/opth.s3967
Antes, R., Salomon-Zimri, S., Beck, S., Garrido, M., Livnat, T., Maharshak, I., et al. (2015). VEGF mediates ApoE4-induced neovascularization and synaptic pathology in the choroid and retina. Curr. Alzheimer Res. 12 (4), 323–334. doi:10.2174/1567205012666150325182504
Ao, H., Zhao, X., Liu, B., and Lu, L. (2021a). TXNIP positively regulates the autophagy and apoptosis in the rat müller cell of diabetic retinopathy. Life Sci. 267, 118988. doi:10.1016/j.lfs.2020.118988
Aranda, M. L., and Schmidt, T. M. (2021). “Diversity of intrinsically photosensitive retinal ganglion cells: circuits and functions,” in Cellular and molecular life sciences. Springer science and business media deutschland GmbH, 889–907. doi:10.1007/s00018-020-03641-5
Arden, G., and Sivaprasad, S. (2011). Hypoxia and oxidative stress in the causation of diabetic retinopathy. Curr. diabetes Rev. 7 (5), 291–304. doi:10.2174/157339911797415620
Ascaso, F. J., Cruz, N., Modrego, P. J., Lopez-Anton, R., Santabárbara, J., Pascual, L. F., et al. (2014). Retinal alterations in mild cognitive impairment and Alzheimer’s disease: an optical coherence tomography study. J. Neurology 261 (8), 1522–1530. doi:10.1007/s00415-014-7374-z
Baba, K., Piano, I., Lyuboslavsky, P., Chrenek, M. A., Sellers, J. T., Zhang, S., et al. (2018). Removal of clock gene Bmal1 from the retina affects retinal development and accelerates cone photoreceptor degeneration during aging. Proc. Natl. Acad. Sci. U. S. A. 115 (51), 13099–13104. doi:10.1073/pnas.1808137115
Baril, A., Beiser, A. S., DeCarli, C., Sanchez, E., Mysliwiec, V., Seshadri, S., et al. (2020). Greater REM sleep associates with lower subcortical gray matter in APOE4 carriers. Alzheimer’s Dementia 16 (S3). doi:10.1002/alz.045255
Baril, A. A., Beiser, A. S., Redline, S., McGrath, E. R., Aparicio, H. J., Gottlieb, D. J., et al. (2021). Systemic inflammation as a moderator between sleep and incident dementia. Sleep 44 (2), zsaa164. doi:10.1093/sleep/zsaa164
Batarseh, Y. S., Duong, Q. V., Mousa, Y. M., Al Rihani, S. B., Elfakhri, K., and Kaddoumi, A. (2016). Amyloid-β and astrocytes interplay in amyloid-β related disorders. Int. J. Mol. Sci. 17 (3), 338. doi:10.3390/ijms17030338
Bellesi, M., de Vivo, L., Chini, M., Gilli, F., Tononi, G., and Cirelli, C. (2017). Sleep loss promotes astrocytic phagocytosis and microglial activation in mouse cerebral cortex. J. Neurosci. 37 (21), 5263–5273. doi:10.1523/JNEUROSCI.3981-16.2017
Bevan, R. J., Hughes, T. R., Williams, P. A., Good, M. A., Paul Morgan, B., and Morgan, J. E. (2020). Retinal ganglion cell degeneration correlates with hippocampal spine loss in experimental Alzheimer’s disease. Acta Neuropathol. Commun. 8 (1), 216. doi:10.1186/s40478-020-01094-2
Biedermann, B., et al. (1998). Distribution of mitochondria within Mü ller cells-I. Correlation with retinal vascularization in different mammalian species. J. Neurocytol.
Birch, M. K., Barbosa, S., Blumhardt, L. D., O'Brien, C., and Harding, S. P. (1996). Retinal venous sheathing and the blood-retinal barrier in multiple sclerosis. Arch. Ophthalmol. 114 (1), 34–39. doi:10.1001/archopht.1996.01100130032005
Bissig, D., Zhou, C. G., Le, V., and Bernard, J. T. (2020). Optical coherence tomography reveals light-dependent retinal responses in Alzheimer’s disease. NeuroImage 219, 117022. doi:10.1016/j.neuroimage.2020.117022
Blanks, J. C., Schmidt, S. Y., Torigoe, Y., Porrello, K. V., Hinton, D. R., and Blanks, R. H. (1996). Retinal pathology in alzheimer’s disease. II. Regional neuron loss and glial changes in GCL. Neurobiol. Aging 17, 385–395. doi:10.1016/0197-4580(96)00009-7
Bomfim, T. R., Forny-Germano, L., Sathler, L. B., Brito-Moreira, J., Houzel, J. C., Decker, H., et al. (2012). An anti-diabetes agent protects the mouse brain from defective insulin signaling caused by Alzheimer’s disease–associated Aβ oligomers. J. Clin. Investigation 122 (4), 1339–1353. doi:10.1172/JCI57256
Bringmann, A., Iandiev, I., Pannicke, T., Wurm, A., Hollborn, M., Wiedemann, P., et al. (2009). Cellular signaling and factors involved in Müller cell gliosis: neuroprotective and detrimental effects. Prog. Retin. Eye Res. 28, 423–451. doi:10.1016/j.preteyeres.2009.07.001
Bringmann, A., Pannicke, T., Grosche, J., Francke, M., Wiedemann, P., Skatchkov, S., et al. (2006). Müller cells in the healthy and diseased retina. Prog. Retin. Eye Res. 25, 397–424. doi:10.1016/j.preteyeres.2006.05.003
Bruera, M. G., Benedetto, M. M., Guido, M. E., Degano, A. L., and Contin, M. A. (2022). Glial cell response to constant low light exposure in rat retina. Vis. Neurosci. 39, E005. doi:10.1017/s0952523822000049
Caicedo, A., Espinosa-Heidmann, D. G., Piña, Y., Hernandez, E. P., and Cousins, S. W. (2005). Blood-derived macrophages infiltrate the retina and activate Muller glial cells under experimental choroidal neovascularization. Exp. Eye Res. 81 (1), 38–47. doi:10.1016/j.exer.2005.01.013
Carrero, L., Antequera, D., Alcalde, I., Megias, D., Ordoñez-Gutierrez, L., Gutierrez, C., et al. (2023). Altered clock gene expression in female APP/PS1 mice and aquaporin-dependent amyloid accumulation in the retina. Int. J. Mol. Sci. 24 (21), 15679. doi:10.3390/ijms242115679
Çerman, E., Eraslan, M., and Çekİç, O. (2015). Age-related macular degeneration and Alzheimer disease. Turkish J. Med. Sci. Turkiye Klinikleri J. Med. Sci. 45, 1004–1009. doi:10.3906/sag-1406-146
Chan, V. T. T., Sun, Z., Tang, S., Chen, L. J., Wong, A., Tham, C. C., et al. (2019). Spectral-domain OCT measurements in alzheimer’s disease: a systematic review and meta-analysis. Ophthalmology 126, 497–510. doi:10.1016/j.ophtha.2018.08.009
Chan, Y. J., Hsiao, G., Wan, W. N., Yang, T. M., Tsai, C. H., Kang, J. J., et al. (2023). Blue light exposure collapses the inner blood-retinal barrier by accelerating endothelial CLDN5 degradation through the disturbance of GNAZ and the activation of ADAM17. Fluids Barriers CNS 20 (1), 31. doi:10.1186/s12987-023-00430-7
Chang, H.-C., and Leonard, G. (2013). SIRT1 mediates central circadian control in the SCN by a mechanism that decays with aging. Cell. 153 (7), 1448–1460. doi:10.1016/j.cell.2013.05.027
Chen, J., Zhou, Y., Mueller-Steiner, S., Chen, L. F., Kwon, H., Yi, S., et al. (2005). SIRT1 protects against microglia-dependent amyloid-β toxicity through inhibiting NF-κB signaling. J. Biol. Chem. 280 (48), 40364–40374. doi:10.1074/jbc.M509329200
Chen, T., Dai, S. H., Li, X., Luo, P., Zhu, J., Wang, Y. H., et al. (2018). Sirt1-Sirt3 axis regulates human blood-brain barrier permeability in response to ischemia. Redox Biol. 14, 229–236. doi:10.1016/j.redox.2017.09.016
Chen, Y., Jiang, Y., Yang, Y., Huang, X., and Sun, C. (2021). SIRT1 protects dopaminergic neurons in Parkinson’s disease models via PGC-1α-mediated mitochondrial biogenesis. Neurotox. Res. 39 (5), 1393–1404. doi:10.1007/s12640-021-00392-4
Cheung, C. Y. L., Ong, Y. T., Ikram, M. K., Chen, C., and Wong, T. Y. (2014). Retinal microvasculature in Alzheimer's disease. J. Alzheimer's Dis. 42 (s4), S339–S352. doi:10.3233/jad-141596
Chougule, P. S., Najjar, R. P., Finkelstein, M. T., Kandiah, N., and Milea, D. (2019). Light-induced pupillary responses in Alzheimer's disease. Front. neurology 10, 360. doi:10.3389/fneur.2019.00360
Chuang, Y. C., Chen, S. D., Jou, S. B., Lin, T. K., Chen, S. F., Chen, N. C., et al. (2019). Sirtuin 1 regulates mitochondrial biogenesis and provides an endogenous neuroprotective mechanism against seizure-induced neuronal cell death in the hippocampus following status epilepticus. Int. J. Mol. Sci. 20 (14), 3588. doi:10.3390/ijms20143588
Chung, H., and Byeon, S. H. (2017). “New insights into the pathoanatomy of macular holes based on features of optical coherence tomography,” in Survey of ophthalmology (USA: Elsevier), 506–521. doi:10.1016/j.survophthal.2017.03.003
Chylinski, D., Van Egroo, M., Narbutas, J., Muto, V., Bahri, M. A., Berthomier, C., et al. (2022). Timely coupling of sleep spindles and slow waves linked to early amyloid-β burden and predicts memory decline. eLife 11, e78191. doi:10.7554/eLife.78191
Contreras, E., Nobleman, A. P., Robinson, P. R., and Schmidt, T. M. (2021). Melanopsin phototransduction: beyond canonical cascades. J. Exp. Biol. 224 (23), jeb226522. doi:10.1242/jeb.226522
Czakó, C., et al. (2020). “Retinal biomarkers for Alzheimer’s disease and vascular cognitive impairment and dementia (VCID): implication for early diagnosis and prognosis,” in GeroScience (Springer Science and Business Media Deutschland GmbH), 1499–1525. doi:10.1007/s11357-020-00252-7
Da Mesquita, S., Herz, J., Wall, M., Dykstra, T., de Lima, K. A., Norris, G. T., et al. (2021). Aging-associated deficit in CCR7 is linked to worsened glymphatic function, cognition, neuroinflammation, and β-amyloid pathology. Sci. Adv. 7, eabe4601. doi:10.1126/sciadv.abe4601
Das, A. V., Mallya, K. B., Zhao, X., Ahmad, F., Bhattacharya, S., Thoreson, W. B., et al. (2006). Neural stem cell properties of Müller glia in the mammalian retina: regulation by Notch and Wnt signaling. Dev. Biol. 299 (1), 283–302. doi:10.1016/j.ydbio.2006.07.029
D’Atri, A., Scarpelli, S., Gorgoni, M., Truglia, I., Lauri, G., Cordone, S., et al. (2021). EEG alterations during wake and sleep in mild cognitive impairment and Alzheimer’s disease. iScience 24 (4), 102386. doi:10.1016/j.isci.2021.102386
Deane, R., Sagare, A., Hamm, K., Parisi, M., Lane, S., Finn, M. B., et al. (2008). apoE isoform–specific disruption of amyloid β peptide clearance from mouse brain. J. Clin. Investigation 118 (12), 4002–4013. doi:10.1172/JCI36663
De Gennaro, L., Gorgoni, M., Reda, F., Lauri, G., Truglia, I., Cordone, S., et al. (2017). The fall of sleep K-complex in alzheimer disease. Sci. Rep. 7, 39688. doi:10.1038/srep39688
den Haan, J., Janssen, S. F., van de Kreeke, J. A., Scheltens, P., Verbraak, F. D., and Bouwman, F. H. (2018b). Retinal thickness correlates with parietal cortical atrophy in early-onset Alzheimer’s disease and controls. Alzheimer’s Dementia Diagnosis, Assess. Dis. Monit. 10, 49–55. doi:10.1016/j.dadm.2017.10.005
den Haan, J., Morrema, T. H. J., Verbraak, F. D., de Boer, J. F., Scheltens, P., Rozemuller, A. J., et al. (2018a). Amyloid-beta and phosphorylated tau in post-mortem Alzheimer’s disease retinas. Acta neuropathol. Commun. 6 (1), 147. doi:10.1186/s40478-018-0650-x
den Haan, J., Verbraak, F. D., Visser, P. J., and Bouwman, F. H. (2017). Retinal thickness in Alzheimer’s disease: a systematic review and meta-analysis. Alzheimer’s Dementia Diagnosis, Assess. Dis. Monit. 6, 162–170. doi:10.1016/j.dadm.2016.12.014
Devi, T. S., et al. (2017). “TXNIP regulates mitophagy in retinal Müller cells under high-glucose conditions: implications for diabetic retinopathy,” in Cell death and disease (London, United Kingdom: Nature Publishing Group). doi:10.1038/cddis.2017.190
Devi, T. S., Lee, I., Hüttemann, M., Kumar, A., Nantwi, K. D., and Singh, L. P. (2012). TXNIP links innate host defense mechanisms to oxidative stress and inflammation in retinal muller glia under chronic hyperglycemia: implications for diabetic retinopathy. Exp. Diabetes Res. 2012, 1–19. doi:10.1155/2012/438238
Dinet, V., Bruban, J., Chalour, N., Maoui, A., An, N., Jonet, L., et al. (2012). Distinct effects of inflammation on gliosis, osmohomeostasis, and vascular integrity during amyloid beta-induced retinal degeneration. Aging Cell. 11 (4), 683–693. doi:10.1111/j.1474-9726.2012.00834.x
Ding, R., Hase, Y., Ameen-Ali, K. E., Ndung’u, M., Stevenson, W., Barsby, J., et al. (2020). Loss of capillary pericytes and the blood–brain barrier in white matter in poststroke and vascular dementias and Alzheimer’s disease. Brain Pathol. 30 (6), 1087–1101. doi:10.1111/bpa.12888
Djordjevic, B., Cvetkovic, T., Milenkovic, J., Stojiljkovic, V., Veljkovic, A.-D., and Sokolovic, D. (2021). Blood-retinal barrier breakdown in diabetic retinopathy-the protective role of melatonin. doi:10.5281/zenodo.5512518
Eichler, W., Kuhrt, H., Hoffmann, S., Wiedemann, P., and Reichenbach, A. (2000). VEGF release by retinal glia depends on both oxygen and glucose supply. Neuroreport 11 (16), 3533–3537. doi:10.1097/00001756-200011090-00026
Einarsdottir, A. B., Hardarson, S. H., Kristjansdottir, J. V., Bragason, D. T., Snaedal, J., and Stefánsson, E. (2016). Retinal oximetry imaging in Alzheimer’s disease. J. Alzheimer's Dis. 49 (1), 79–83. doi:10.3233/jad-150457
Fang, E. F., Hou, Y., Palikaras, K., Adriaanse, B. A., Kerr, J. S., Yang, B., et al. (2019). Mitophagy inhibits amyloid-β and tau pathology and reverses cognitive deficits in models of Alzheimer’s disease. Nat. Neurosci. 22 (3), 401–412. doi:10.1038/s41593-018-0332-9
Ferreira, L. S. S., et al. (2018). “Insulin resistance in Alzheimer’s disease,” in Frontiers in neuroscience (Lausanne, Switzerland: Frontiers Media S.A). doi:10.3389/fnins.2018.00830
Fiotti, N., Pedio, M., Parodi, M. B., Altamura, N., Uxa, L., Guarnieri, G., et al. (2005). MMP-9 microsatellite polymorphism and susceptibility to exudative form of age-related macular degeneration. Genet. Med. 7 (4), 272–277. doi:10.1097/01.GIM.0000159903.69597.73
Fischer, A. J., and Reh, T. A. (2003). Potential of Müller glia to become neurogenic retinal progenitor cells. GLIA 43 (1), 70–76. doi:10.1002/glia.10218
Franze, K., Grosche, J., Skatchkov, S. N., Schinkinger, S., Foja, C., Schild, D., et al. (2007). Mü ller cells are living optical fibers in the vertebrate retina. Proc. Natl. Acad. Sci. U. S. A. 104, 8287–8292. doi:10.1073/pnas.0611180104
Frigerio, F., Frasca, A., Weissberg, I., Parrella, S., Friedman, A., Vezzani, A., et al. (2012). Long-lasting pro-ictogenic effects induced in vivo by rat brain exposure to serum albumin in the absence of concomitant pathology. Epilepsia 53 (11), 1887–1897. doi:10.1111/j.1528-1167.2012.03666.x
Froggett, P., Hussan, S., Greenstein, A., Robinson, A., Giggs, J., and Hawkins, V. (2024). Summer meeting of the anatomical society: Dublin 2022. J. Anat. doi:10.1111/joa.13797
Furtado, A., Astaburuaga, R., Costa, A., Duarte, A. C., Gonçalves, I., Cipolla-Neto, J., et al. (2020). The rhythmicity of clock genes is disrupted in the choroid plexus of the APP/PS1 mouse model of alzheimer’s disease. J. Alzheimer’s Dis. 77 (2), 795–806. doi:10.3233/JAD-200331
Gao, J., Qian, T., and Wang, W. (2020). CTRP3 activates the AMPK/SIRT1-PGC-1α pathway to protect mitochondrial biogenesis and functions in cerebral ischemic stroke. Neurochem. Res. 45 (12), 3045–3058. doi:10.1007/s11064-020-03152-6
Gardner, M. R., Baruah, V., Vargas, G., Motamedi, M., Milner, T. E., and Rylander, H. G. (2020). Scattering angle resolved optical coherence tomography detects early changes in 3xTg Alzheimer’s disease mouse model. Transl. Vis. Sci. Technol. 9 (5), 18–14. doi:10.1167/TVST.9.5.18
Gardner, M. R., Rahman, A. S., Milner, T. E., and Rylander, H. G. (2019). Scattering-angle-resolved optical coherence tomography of a hypoxic mouse retina model. J. Exp. Neurosci. 13, 117906951983756. doi:10.1177/1179069519837564
Gastón Bruera, M., et al. (2021). “Glial cell responses to constant low light exposure,” in Rat retina. doi:10.1101/2021.05.10.443423
Gelfand, J. M., Nolan, R., Schwartz, D. M., Graves, J., and Green, A. J. (2012). Microcystic macular oedema in multiple sclerosis is associated with disease severity. Brain 135 (6), 1786–1793. doi:10.1093/brain/aws098
Germer, A., Schuck, J., Wolburg, H., Kuhrt, H., Mack, A. F., and Reichenbach, A. (1998). Distribution of mitochondria within Muller cells–II. Post-natal development of the rabbit retinal periphery in vivo and in vitro: dependence on oxygen supply. J. Neurocytol. 27, 347–359. doi:10.1023/a:1006938825474
Giannelli, S. G., Demontis, G. C., Pertile, G., Rama, P., and Broccoli, V. (2011). Adult human Müller glia cells are a highly efficient source of rod photoreceptors. Stem Cells 29 (2), 344–356. doi:10.1002/stem.579
Giannelli, S. G., Demontis, G. C., Pertile, G., Rama, P., and Broccoli, V. (2011). Adult human Müller glia cells are a highly efficient source of rod photoreceptors. Stem cells 29 (2), 344–356. doi:10.1002/stem.579
Ginhoux, F., Lim, S., Hoeffel, G., Low, D., and Huber, T. (2013). Origin and differentiation of microglia. Front. Cell. Neurosci. 7, 45. doi:10.3389/fncel.2013.00045
Goldman, D. (2014). “Müller glial cell reprogramming and retina regeneration,” in Nature reviews neuroscience (London, United Kingdom: Nature Publishing Group), 431–442. doi:10.1038/nrn3723
Gómez-González, B., Hurtado-Alvarado, G., Esqueda-Leon, E., Santana- Miranda, R., Rojas-Zamorano, J., and Velazquez-Moctezuma, J. (2013). REM sleep loss and recovery regulates blood-brain barrier function. Curr. Neurovascular Res. 10, 197–207. doi:10.2174/15672026113109990002
Govetto, A., Hubschman, J. P., Sarraf, D., Figueroa, M. S., Bottoni, F., dell'Omo, R., et al. (2020). The role of Müller cells in tractional macular disorders: an optical coherence tomography study and physical model of mechanical force transmission. Br. J. Ophthalmol. 104 (4), 466–472. doi:10.1136/bjophthalmol-2019-314245
Graf, T., Flammer, J., Prünte, C., and Hendrickson, P. (1993). Gliosis-like retinal alterations in glaucoma patients. J. glaucoma 2 (4), 257–259. doi:10.1097/00061198-199300240-00006
Green, A. J., McQuaid, S., Hauser, S. L., Allen, I. V., and Lyness, R. (2010). Ocular pathology in multiple sclerosis: retinal atrophy and inflammation irrespective of disease duration. Brain a J. neurology 133 (Pt 6), 1591–1601. doi:10.1093/brain/awq080
Greene, C., Hanley, N., and Campbell, M. (2019). Claudin-5: gatekeeper of neurological function. Fluids Barriers CNS 16, 3. doi:10.1186/s12987-019-0123-z
Greenwood, S. M., and Connolly, C. N. (2007). Dendritic and mitochondrial changes during glutamate excitotoxicity. Neuropharmacology 53 (8), 891–898. doi:10.1016/j.neuropharm.2007.10.003
Guo, Y., Cepurna, W. O., Dyck, J. A., Doser, T. A., Johnson, E. C., and Morrison, J. C. (2010). Retinal cell responses to elevated intraocular pressure: a gene array comparison between the whole retina and retinal ganglion cell layer. Investigative Ophthalmol. Vis. Sci. 51 (6), 3003–3018. doi:10.1167/iovs.09-4663
Gupta, V. K., Chitranshi, N., Golzan, M., Dheer, Y., Wall, R. V., et al. (2016). Amyloid β accumulation and inner retinal degenerative changes in Alzheimer’s disease transgenic mouse. Neurosci. Lett. 623, 52–56. doi:10.1016/j.neulet.2016.04.059
Harrison, I. F., Ismail, O., Machhada, A., Colgan, N., Ohene, Y., Nahavandi, P., et al. (2020). Impaired glymphatic function and clearance of tau in an Alzheimer’s disease model. Brain 143 (8), 2576–2593. doi:10.1093/brain/awaa179
Hart, N. J., Koronyo, Y., Black, K. L., and Koronyo-Hamaoui, M. (2016a). Ocular indicators of Alzheimer’s: exploring disease in the retina. Acta Neuropathol. 132, 767–787. doi:10.1007/s00401-016-1613-6
Hassan, I., Luo, Q., Majumdar, S., Dominguez, J. M., Busik, J. V., and Bhatwadekar, A. D. (2017). Tumor necrosis factor alpha (TNF-α) disrupts Kir4.1 channel expression resulting in müller cell dysfunction in the retina. Investigative Ophthalmol. Vis. Sci. 58 (5), 2473–2482. doi:10.1167/iovs.16-20712
He, J., Hsuchou, H., He, Y., Kastin, A. J., Wang, Y., and Pan, W. (2014). Sleep restriction impairs blood-brain barrier function. J. Neurosci. 34 (44), 14697–14706. doi:10.1523/JNEUROSCI.2111-14.2014
Heinrich, R., Hertz, R., Zemel, E., Mann, I., Brenner, L., Massarweh, A., et al. (2018). ATF3 regulates the expression of AChE during stress. Front. Mol. Neurosci. 11, 88. doi:10.3389/fnmol.2018.00088
Holtzman, D. M., Herz, J., and Bu, G. (2012). Apolipoprotein E and apolipoprotein E receptors: normal biology and roles in Alzheimer disease. Cold Spring Harb. Perspect. Med. 2 (3), a006312. doi:10.1101/cshperspect.a006312
Homolak, J., et al. (2018). “Circadian rhythm and alzheimer’s disease,” in Medical sciences (Basel, Switzerland: NLM). doi:10.3390/medsci6030052
Hudson, N., Celkova, L., Hopkins, A., Greene, C., Storti, F., Ozaki, E., et al. (2019). Dysregulated claudin-5 cycling in the inner retina causes retinal pigment epithelial cell atrophy. JCI insight 4 (15), e130273. doi:10.1172/jci.insight.130273
Huffels, C. F. M., Osborn, L. M., Hulshof, L. A., Kooijman, L., Henning, L., Steinhäuser, C., et al. (2022). Amyloid-β plaques affect astrocyte Kir4.1 protein expression but not function in the dentate gyrus of APP/PS1 mice. GLIA 70 (4), 748–767. doi:10.1002/glia.24137
Iandiev, I., Wurm, A., Hollborn, M., Wiedemann, P., Grimm, C., Reme, C. E., et al. (2008). Muller cell response to blue light injury of the rat retina. Investigative Ophthalmol. Vis. Sci. 49 (8), 3559–3567. doi:10.1167/iovs.08-1723
Inman, D. M., and Horner, P. J. (2007). Reactive nonproliferative gliosis predominates in a chronic mouse model of glaucoma. Glia 55 (9), 942–953. doi:10.1002/glia.20516
Ivens, S., Kaufer, D., Flores, L. P., Bechmann, I., Zumsteg, D., Tomkins, O., et al. (2007). TGF- receptor-mediated albumin uptake into astrocytes is involved in neocortical epileptogenesis. Brain 130 (2), 535–547. doi:10.1093/brain/awl317
Jáñez-Escalada, L., Jáñez-García, L., Salobrar-García, E., Santos-Mayo, A., de Hoz, R., Yubero, R., et al. (2019). Spatial analysis of thickness changes in ten retinal layers of Alzheimer’s disease patients based on optical coherence tomography. Sci. Rep. 9 (1), 13000. doi:10.1038/s41598-019-49353-0
Janota, C. S., Brites, D., Lemere, C. A., and Brito, M. A. (2015). Glio-vascular changes during ageing in wild-type and Alzheimer’s disease-like APP/PS1 mice. Brain Res. 1620, 153–168. doi:10.1016/j.brainres.2015.04.056
Jia, J., Yin, J., Zhang, Y., Xu, G., Wang, M., Jiang, H., et al. (2023). Thioredoxin-1 promotes mitochondrial biogenesis through regulating AMPK/Sirt1/PGC1α pathway in alzheimer’s disease. ASN Neuro 15, 175909142311592. doi:10.1177/17590914231159226
Joly-Amado, A., Hunter, J., Quadri, Z., Zamudio, F., Rocha-Rangel, P. V., Chan, D., et al. (2020). CCL2 overexpression in the brain promotes glial activation and accelerates tau pathology in a mouse model of tauopathy. Front. Immunol. 11, 997. doi:10.3389/fimmu.2020.00997
Jorge, L., Canário, N., Martins, R., Santiago, B., Santana, I., Quental, H., et al. (2020). The retinal inner plexiform synaptic layer mirrors grey matter thickness of primary visual cortex with increased amyloid β load in early alzheimer’s disease. Neural Plast. 2020, 1–11. doi:10.1155/2020/8826087
Hoh Kam, J., Lenassi, E., and Jeffery, G. (2010). Viewing ageing eyes: diverse sites of amyloid Beta accumulation in the ageing mouse retina and the up-regulation of macrophages. PloS one 5 (10), e13127. doi:10.1371/journal.pone.0013127
Kaneshwaran, K., Olah, M., Tasaki, S., Yu, L., Bradshaw, E. M., Schneider, J. A., et al. (2019). Sleep fragmentation, microglial aging, and cognitive impairment in adults with and without Alzheimer’s dementia. Sci. Adv. 5, eaax7331. doi:10.1126/sciadv.aax7331
Kang, S., Larbi, D., Andrade, M., Reardon, S., Reh, T. A., and Wohl, S. G. (2021). A comparative analysis of reactive müller glia gene expression after light damage and microRNA-depleted müller glia—focus on microRNAs. Front. Cell. Dev. Biol. 8, 620459. doi:10.3389/fcell.2020.620459
Keaney, J., Walsh, D. M., O’Malley, T., Hudson, N., Crosbie, D. E., Loftus, T., et al. (2015). Autoregulated paracellular clearance of amyloid-β across the blood-brain barrier. Sci. Adv. 1 (8), e1500472. doi:10.1126/sciadv.1500472
Kim, J.Il, and Kang, B. H. (2019). Decreased retinal thickness in patients with Alzheimer’s disease is correlated with disease severity. PLoS ONE 14 (11), e0224180. doi:10.1371/journal.pone.0224180
Kim, S. Y., Kambhampati, S. P., Bhutto, I. A., McLeod, D. S., Lutty, G. A., and Kannan, R. M. (2021). Evolution of oxidative stress, inflammation and neovascularization in the choroid and retina in a subretinal lipid induced age-related macular degeneration model. Exp. Eye Res. 203, 108391. doi:10.1016/j.exer.2020.108391
Knier, B., et al. (2016). Retinal inner nuclear layer volume reflects response to immunotherapy in multiple sclerosis. doi:10.1093/aww239
Ko, G. Y. P. (2020). “Circadian regulation in the retina: from molecules to network,” in European journal of neuroscience (Oxford, United Kingdom: Blackwell Publishing Ltd), 194–216. doi:10.1111/ejn.14185
Kole, C., Brommer, B., Nakaya, N., Sengupta, M., Bonet-Ponce, L., Zhao, T., et al. (2020). Activating transcription factor 3 (ATF3) protects retinal ganglion cells and promotes functional preservation after optic nerve crush. Investigative Ophthalmol. Vis. Sci. 61 (2), 31. doi:10.1167/iovs.61.2.31
Kong, L., and Zhang, Z. (2021). Artemisinin ameliorates diabetic retinopathy by upregulating casc2/mir-155/sirt1 axis. Trop. J. Pharm. Res. 20 (10), 2023–2028. doi:10.4314/tjpr.v20i10.2
Koronyo, Y., Biggs, D., Barron, E., Boyer, D. S., Pearlman, J. A., Au, W. J., et al. (2017). Retinal amyloid pathology and proof-of-concept imaging trial in Alzheimer’s disease. JCI Insight 2 (16), e93621. doi:10.1172/JCI.INSIGHT.93621
Kowluru, R. A. (2010). Role of matrix metalloproteinase-9 in the development of diabetic retinopathy and its regulation by H-Ras. Investigative Ophthalmol. Vis. Sci. 51 (8), 4320–4326. doi:10.1167/iovs.09-4851
Kress, G. J., Liao, F., Dimitry, J., Cedeno, M. R., FitzGerald, G. A., Holtzman, D. M., et al. (2018). Regulation of amyloid-β dynamics and pathology by the circadian clock. J. Exp. Med. 215 (4), 1059–1068. doi:10.1084/jem.20172347
Križaj, D., Cordeiro, S., and Strauß, O. (2023). Retinal TRP channels: cell-type-specific regulators of retinal homeostasis and multimodal integration. Prog. Retin. eye Res. 92, 101114. doi:10.1016/j.preteyeres.2022.101114
Krügel, K., Wurm, A., Pannicke, T., Hollborn, M., Karl, A., Wiedemann, P., et al. (2011). Involvement of oxidative stress and mitochondrial dysfunction in the osmotic swelling of retinal glial cells from diabetic rats. Exp. Eye Res. 92 (1), 87–93. doi:10.1016/j.exer.2010.11.007
Kshirsagar, S., Sawant, N., Morton, H., Reddy, A. P., and Reddy, P. H. (2022). Protective effects of mitophagy enhancers against amyloid beta-induced mitochondrial and synaptic toxicities in Alzheimer disease. Hum. Mol. Genet. 31 (3), 423–439. doi:10.1093/hmg/ddab262
Kumar, A., et al. (2013). Müller Glia in Retinal Innate Immunity: a perspective on their roles in endophthalmitis.
Labin, A. M., and Ribak, E. N. (2010). Retinal glial cells enhance human vision acuity. Phys. Rev. Lett. 104 (15), 158102. doi:10.1103/physrevlett.104.158102
Labin, A. M., Safuri, S. K., Ribak, E. N., and Perlman, I. (2014). Müller cells separate between wavelengths to improve day vision with minimal effect upon night vision. Nat. Commun. 5 (1), 4319. doi:10.1038/ncomms5319
Lamoke, F., Stampley, C., Jahng, W. J., Baban, B., and Bartoli, M. (2012). Regulation of Thioredoxin Interacting Protein (TXNIP) activity by PKC delta in the diabetic retina. Investigative Ophthalmol. Vis. Sci. 53 (14), 5418.
la Morgia, C., Ross-Cisneros, F. N., Koronyo, Y., Hannibal, J., Gallassi, R., Cantalupo, G., et al. (2016). Melanopsin retinal ganglion cell loss in Alzheimer disease. Ann. Neurology 79 (1), 90–109. doi:10.1002/ana.24548
Lauwen, S., Lefeber, D. J., Fauser, S., Hoyng, C. B., and Anneke, I. den H. (2021). Increased pro-MMP9 plasma levels are associated with neovascular age-related macular degeneration and with the risk allele of rs142450006 near MMP9. Mol. Vis. 27, 142–150.
Lax, P., Ortuño-Lizarán, I., Maneu, V., Vidal-Sanz, M., and Cuenca, N. (2019). Photosensitive melanopsin-containing retinal ganglion cells in health and disease: implications for circadian rhythms. Int. J. Mol. Sci. 20 (13), 3164. doi:10.3390/ijms20133164
Lax, P., Otalora, B. B., Esquiva, G., Rol, M. d. l. Á., Madrid, J. A., and Cuenca, N. (2011). Circadian dysfunction in P23H rhodopsin transgenic rats: effects of exogenous melatonin. J. pineal Res. 50 (2), 183–191. doi:10.1111/j.1600-079x.2010.00827.x
Lee, S., Jiang, K., McIlmoyle, B., To, E., Xu, Q., Hirsch-Reinshagen, V., et al. (2020). Amyloid beta immunoreactivity in the retinal ganglion cell layer of the Alzheimer’s eye. Front. Neurosci. 14, 758. doi:10.3389/fnins.2020.00758
Lee, Y., Lee, J., and Jo, D. (2023). A novel function of CD44 in the pathogenesis of Alzheimer’s disease. Alzheimer’s Dementia 19 (S21). doi:10.1002/alz.076285
Lefevere, E., Toft-Kehler, A. K., Vohra, R., Kolko, M., Moons, L., and Van Hove, I. (2017). Mitochondrial dysfunction underlying outer retinal diseases. Mitochondrion 36, 66–76. doi:10.1016/j.mito.2017.03.006
Lenkowski, J. R., and Raymond, P. A. (2014). Müller glia: stem cells for generation and regeneration of retinal neurons in teleost fish. Prog. Retin. Eye Res. 40, 94–123. doi:10.1016/j.preteyeres.2013.12.007
Leong, I. (2018). Metabolism: DRP1 links mitochondrial dynamics to the clock. Nat. Rev. Endocrinol. 14 (5), 252–253. doi:10.1038/nrendo.2018.32
Li, M.-Z., Zheng, L.-J., Shen, J., Li, X.-Ya, Qi, Z., Bai, X., et al. (2018). SIRT1 facilitates amyloid beta peptide degradation by upregulating lysosome number in primary astrocytes. Neural Regen. Res. 13 (11), 2005–2013. doi:10.4103/1673-5374.239449
Li, Y., Zhang, J., Wan, J., Liu, A., and Sun, J. (2020). Melatonin regulates Aβ production/clearance balance and Aβ neurotoxicity: a potential therapeutic molecule for Alzheimer’s disease. Biomed. Pharmacother. 132, 110887. doi:10.1016/j.biopha.2020.110887
Lindqvist, N., Liu, Q., Zajadacz, J., Franze, K., and Reichenbach, A. (2010). Retinal glial (müller) cells: sensing and responding to tissue stretch. Investigative Ophthalmol. Vis. Sci. 51 (3), 1683–1690. doi:10.1167/iovs.09-4159
Liu, R. T., Gao, J., Cao, S., Sandhu, N., Cui, J. Z., Chou, C. L., et al. (2013). Inflammatory mediators induced by amyloid-beta in the retina and RPE in vivo: implications for inflammasome activation in age-related macular degeneration. Investigative Ophthalmol. Vis. Sci. 54 (3), 2225–2237. doi:10.1167/iovs.12-10849
Liu, X. Q., Kobayashi, H., Jin, Z. B., Wada, A., and Nao-i, N. (2007). Differential expression of Kir4.1 and aquaporin 4 in the retina from endotoxin-induced uveitis rat. Mol. Vis. 13, 309–317.
Liutkeviciene, R., Lesauskaite, V., Sinkunaite-Marsalkiene, G., Zaliuniene, D., Zaliaduonyte-Peksiene, D., Mizariene, V., et al. (2015). The role of matrix metalloproteinases polymorphisms in age-related macular degeneration. Ophthalmic Genet. 36 (2), 149–155. doi:10.3109/13816810.2013.838274
Löffler, S., Wurm, A., Kutzera, F., Pannicke, T., Krügel, K., Linnertz, R., et al. (2010). Serum albumin induces osmotic swelling of rat retinal glial cells. Brain Res. 1317, 268–276. doi:10.1016/j.brainres.2009.12.067
Lucey, B. P. (2020). It's complicated: the relationship between sleep and alzheimer's disease in humans. Neurobiol. Dis. 144, 105031. doi:10.1016/j.nbd.2020.105031
Lujan, B. J., and Horton, J. C. (2013). Microcysts in the inner nuclear layer from optic atrophy are caused by retrograde trans-synaptic degeneration combined with vitreous traction on the retinal surface. Brain 136 (11), e260. doi:10.1093/brain/awt154
Luo, Q., Xiao, Y., Cummins, T. R., and Bhatwadekar, A. D. (2019). The diurnal rhythm of insulin receptor substrate-1 (IRS-1) and Kir4.1 in diabetes: implications for a clock gene Bmal1. Investigative Ophthalmol. Vis. Sci. 60 (6), 1928–1936. doi:10.1167/iovs.18-26045
Lustbader, J. W., Cirilli, M., Lin, C., Xu, H. W., Takuma, K., Wang, N., et al. (2004). ABAD directly links aß to mitochondrial toxicity in alzheimer's disease. Science 304 (5669), 448–452. doi:10.1126/science.1091230
Madigan, M., Diep, M., Junghans, B., and Valter, K. (2014). Aquaporin-4 and potassium channel kir4. 1 in AMD and serous retinal detachment secondary to choroidal melanoma. Acta Ophthalmol. 92, 0. doi:10.1111/j.1755-3768.2014.3644.x
Mahoney, H. L., and Schmidt, T. M. (2024). The cognitive impact of light: illuminating ipRGC circuit mechanisms. Nat. Rev. Neurosci. 25, 159–175. doi:10.1038/s41583-023-00788-5
Maran, J. J., Adesina, M. M., Green, C. R., Kwakowsky, A., and Mugisho, O. O. (2023). Retinal inner nuclear layer thickness in the diagnosis of cognitive impairment explored using a C57BL/6J mouse model. Sci. Rep. 13 (1), 8150. doi:10.1038/s41598-023-35229-x
Marchese, N. A., Ríos, M. N., and Guido, M. E. (2023). Müller glial cell photosensitivity: a novel function bringing higher complexity to vertebrate retinal physiology. J. Photochem. Photobiol. 13, 100162. doi:10.1016/j.jpap.2023.100162
Medina-Flores, F., Hurtado-Alvarado, G., Contis-Montes de Oca, A., López-Cervantes, S. P., Konigsberg, M., Deli, M. A., et al. (2020). Sleep loss disrupts pericyte-brain endothelial cell interactions impairing blood-brain barrier function. Brain, Behav. Immun. 89, 118–132. doi:10.1016/j.bbi.2020.05.077
Milton, M., and Smith, P. D. (2018). “It’s all about timing: the involvement of kir4.1 channel regulation in acute ischemic stroke pathology,” in Frontiers in cellular neuroscience (Lausanne, Switzerland: Frontiers Media S.A). doi:10.3389/fncel.2018.00036
Min, S.-W., Sohn, P. D., Li, Y., Devidze, N., Johnson, J. R., Krogan, N. J., et al. (2018). SIRT1 deacetylates tau and reduces pathogenic tau spread in a mouse model of tauopathy. J. Neurosci. 38 (15), 3680–3688. doi:10.1523/jneurosci.2369-17.2018
Miners, J. S., Schulz, I., and Love, S. (2018). Differing associations between Aβ accumulation, hypoperfusion, blood–brain barrier dysfunction and loss of PDGFRB pericyte marker in the precuneus and parietal white matter in Alzheimer's disease. J. Cereb. Blood Flow Metabolism 38 (1), 103–115. doi:10.1177/0271678X17690761
Mishima, K., Okawa, M., Hishikawa, Y., Hozumi, S., Hori, H., and Takahashi, K. (1994). Morning bright light therapy for sleep and behavior disorders in elderly patients with dementia. Acta Psychiatr. Scand. 89 (1), 1–7. doi:10.1111/j.1600-0447.1994.tb01477.x
Molina-Fernández, R., Picón-Pagès, P., Barranco-Almohalla, A., Crepin, G., Herrera-Fernández, V., García-Elías, A., et al. (2022). Differential regulation of insulin signalling by monomeric and oligomeric amyloid beta-peptide. Brain Commun. 4 (5), fcac243. doi:10.1093/braincomms/fcac243
Most, E. I. S., Scheltens, P., and Van Someren, E. J. W. (2012). Increased skin temperature in alzheimer’s disease is associated with sleepiness. J. Neural Transm. 119 (10), 1185–1194. doi:10.1007/s00702-012-0864-1
Mure, L. S. (2021). Intrinsically photosensitive retinal ganglion cells of the human retina. Front. neurology 12, 636330. Available at. doi:10.3389/fneur.2021.636330
Nasoohi, S., Parveen, K., and Ishrat, T. (2018). Metabolic syndrome, brain insulin resistance, and Alzheimer’s disease: thioredoxin Interacting Protein (TXNIP) and inflammasome as core amplifiers. J. Alzheimer's Dis. 66 (3), 857–885. doi:10.3233/jad-180735
Newman, E. A. (2006) “A purinergic dialogue between glia and neurons in the retina,”in Purinergic signalling in neuron–glia interactions: novartis foundation symposium, 276. Chichester, UK: John Wiley and Sons, Ltd, 193–281. doi:10.1002/9780470032244.ch15
Ni, J., Wu, Z., Meng, J., Saito, T., Saido, T. C., Qing, H., et al. (2019). An impaired intrinsic microglial clock system induces neuroinflammatory alterations in the early stage of amyloid precursor protein knock-in mouse brain. J. Neuroinflammation 16 (1), 173. doi:10.1186/s12974-019-1562-9
Olafsdottir, O. B., Saevarsdottir, H. S., Hardarson, S. H., Hannesdottir, K. H., Traustadottir, V. D., Karlsson, R. A., et al. (2018). Retinal oxygen metabolism in patients with mild cognitive impairment. Alzheimer’s Dementia Diagnosis, Assess. Dis. Monit. 10, 340–345. doi:10.1016/j.dadm.2018.03.002
Ou, K., Mertsch, S., Theodoropoulou, S., Wu, J., Liu, J., Copland, D. A., et al. (2019). Müller cells stabilize microvasculature through hypoxic preconditioning. Cell. Physiology Biochem. 52 (4), 668–680. doi:10.33594/000000047
Panes, J. D., Godoy, P. A., Silva-Grecchi, T., Celis, M. T., Ramirez-Molina, O., Gavilan, J., et al. (2020). Changes in PGC-1α/SIRT1 signaling impact on mitochondrial homeostasis in amyloid-beta peptide toxicity model. Front. Pharmacol. 11, 709. doi:10.3389/fphar.2020.00709
Pannicke, T., Iandiev, I., Wurm, A., Uckermann, O., vom Hagen, F., Reichenbach, A., et al. (2006). Diabetes alters osmotic swelling characteristics and membrane conductance of glial cells in rat retina 55, 633–639. doi:10.2337/diabetes.55.03.06.db05-1349
Park, J., Suh, S. W., Kim, G. E., Lee, S., Kim, J. S., Kim, H. S., et al. (2020). Smaller pineal gland is associated with rapid eye movement sleep behavior disorder in alzheimer’s disease. Alzheimer's Res. Ther. 12, 157–158. doi:10.1186/s13195-020-00725-z
Perez de Lara, M. J., and Jesús, P. (2015). Presence and release of ATP from the retina in an Alzheimer's disease model. J. Alzheimer's Dis. 43 (1), 177–181. doi:10.3233/jad-141005
Pilotto, E., Midena, E., Longhin, E., Parrozzani, R., Frisina, R., and Frizziero, L. (2019). Müller cells and choriocapillaris in the pathogenesis of geographic atrophy secondary to age-related macular degeneration. Graefe’s Archive Clin. Exp. Ophthalmol. 257, 1159–1167. doi:10.1007/s00417-019-04289-z
Prinz, P. N., Peskind, E. R., Vitaliano, P. P., Raskind, M. A., Eisdorfer, C., Zemcuznikov, H. N., et al. (1982). Changes in the sleep and waking EEGs of nondemented and demented elderly subjects. J. Am. Geriatrics Soc. 30 (2), 86–92. doi:10.1111/j.1532-5415.1982.tb01279.x
Pyun, J. M., Kang, M. J., Yun, Y., Park, Y. H., and Kim, S. (2019). APOE ɛ4 and REM sleep behavior disorder as risk factors for sundown syndrome in alzheimer’s disease. J. Alzheimer's Dis. 69 (2), 521–528. doi:10.3233/jad-190032
Ramsey, D. J., and Arden, G. B. (2015). “Hypoxia and dark adaptation in diabetic retinopathy: interactions, consequences, and therapy,” in Current diabetes reports (Philadelphia, PA, United States: Current Medicine Group LLC). doi:10.1007/s11892-015-0686-2
Ratnayaka, J. A., Serpell, L. C., and Lotery, A. J. (2015). Dementia of the eye: the role of amyloid beta in retinal degeneration. Eye (Basingstoke) 29 (8), 1013–1026. doi:10.1038/eye.2015.100
Reda, F., Gorgoni, M., Lauri, G., Truglia, I., Cordone, S., Scarpelli, S., et al. (2017). In search of sleep biomarkers of alzheimer’s disease: K-complexes do not discriminate between patients with mild cognitive impairment and healthy controls. Brain Sci. 7 (5), 51. doi:10.3390/brainsci7050051
Rezai, A. R., et al. (2020). Noninvasive hippocampal blood−brain barrier opening in Alzheimer’s disease with focused ultrasound. doi:10.1073/pnas.2002571117/-/DCSupplemental
Roy, U., Heredia-Muñoz, M. T., Stute, L., Höfling, C., Matysik, J., Meijer, J. H., et al. (2019). Degeneration of the suprachiasmatic nucleus in an alzheimer’s disease mouse model monitored by in vivo magnetic resonance relaxation measurements and immunohistochemistry. J. Alzheimer’s Dis. 69 (2), 363–375. doi:10.3233/JAD-190037
Ruan, G. X., Gamble, K. L., Risner, M. L., Young, L. A., and McMahon, D. G. (2012). Divergent roles of clock genes in retinal and suprachiasmatic nucleus circadian oscillators. PLoS ONE 7 (6), e38985. doi:10.1371/journal.pone.0038985
Sambamurti, K., Venugopal, C., Suram, A., Pappolla, M., Rohrer, B., and Annamalai, P. (2007). Amyloid precursor protein metabolism in retinal degeneration. Investigative Ophthalmol. Vis. Sci. 48 (13), 26.
Savaskan, E., Jockers, R., Ayoub, M., Angeloni, D., Fraschini, F., Flammer, J., et al. (2007). The MT2 melatonin receptor subtype is present in human retina and decreases in Alzheimer's disease. Curr. Alzheimer Res. 4 (1), 47–51. doi:10.2174/156720507779939823
Schultz, H., Song, Y., Kapphahn, R. J., Rocio Montezuma, S., Ferrington, D. A., and Dunaief, J. L. (2019). Blood retinal barrier disruption in non-exudative AMD. Investigative Ophthalmol. Vis. Sci. 60 (9), 4890.
Shiiki, T., Ohtsuki, S., Kurihara, A., Naganuma, H., Nishimura, K., Tachikawa, M., et al. (2004). Brain insulin impairs amyloid-β(1-40) clearance from the brain. J. Neurosci. 24 (43), 9632–9637. doi:10.1523/jneurosci.2236-04.2004
Sigler, E. J. (2014). Microcysts in the inner nuclear layer, a nonspecific SD-OCT sign of cystoid macular edema. Investigative Ophthalmol. Vis. Sci. 55 (5), 3282–3284. doi:10.1167/iovs.14-14056
Smilnak, G. J., Deans, J. R., Doraiswamy, P. M., Stinnett, S., Whitson, H. E., and Lad, E. M. (2019). Comorbidity of age-related macular degeneration with Alzheimer’s disease: a histopathologic case-control study. PLoS ONE 14 (9), e0223199. doi:10.1371/journal.pone.0223199
Smyth, L. C. D., Highet, B., Jansson, D., Wu, J., Rustenhoven, J., Aalderink, M., et al. (2022). Characterisation of PDGF-BB:PDGFRβ signalling pathways in human brain pericytes: evidence of disruption in Alzheimer’s disease. Commun. Biol. 5 (1), 235. doi:10.1038/s42003-022-03180-8
Stenvers, D. J., et al. (2019). “Circadian clocks and insulin resistance,” in Nature reviews endocrinology (London, United Kingdom: Nature Publishing Group), 75–89. doi:10.1038/s41574-018-0122-1
Stopa, E. G., Volicer, L., Kuo-Leblanc, V., Harper, D., Lathi, D., Tate, B., et al. (1999). Pathologic evaluation of the human suprachiasmatic nucleus in severe dementia. J. Neuropathology Exp. Neurology 58 (1), 29–39. doi:10.1097/00005072-199901000-00004
Sun, J., Wu, J., Hua, F., Chen, Y., Zhan, F., and Xu, G. (2020). Sleep deprivation induces cognitive impairment by increasing blood-brain barrier permeability via CD44. Front. Neurology 11, 563916. doi:10.3389/fneur.2020.563916
Szabó, L., Erdei, G., and Maák, P. A. (2022). Optical analysis of müller glia cells as light transporters through the retina. Biomed. Opt. Express 13 (12), 6335–6356. doi:10.1364/boe.462568
Vecino, E., et al. (2016). “Glia-neuron interactions in the mammalian retina,” in Progress in retinal and eye research (Elsevier Ltd), 1–40. doi:10.1016/j.preteyeres.2015.06.003
Ventorp, F., Bay-Richter, C., Sauro, A., Janelidze, S., Matsson, V. S., Lipton, J., et al. (2016). The CD44 ligand hyaluronic acid is elevated in the cerebrospinal fluid of suicide attempters and is associated with increased blood-brain barrier permeability. J. Affect. Disord. 193, 349–354. doi:10.1016/j.jad.2015.12.069
Wahl, V., Vogler, S., Grosche, A., Pannicke, T., Ueffing, M., Wiedemann, P., et al. (2013). Osteopontin inhibits osmotic swelling of retinal glial (Müller) cells by inducing release of VEGF. Neuroscience 246, 59–72. doi:10.1016/j.neuroscience.2013.04.045
Walsh, D. T., Montero, R. M., Bresciani, L. G., Jen, A. Y., Leclercq, P. D., Saunders, D., et al. (2002). Amyloid-beta peptide is toxic to neurons in vivo via indirect mechanisms. Neurobiol. Dis. 10 (1), 20–27. doi:10.1006/nbdi.2002.0485
Wang, C. Y., Xie, J. W., Wang, T., Xu, Y., Cai, J. H., Wang, X., et al. (2013). Hypoxia-triggered m-calpain activation evokes endoplasmic reticulum stress and neuropathogenesis in a transgenic mouse model of alzheimer's disease. CNS Neurosci. Ther. 19 (10), 820–833. doi:10.1111/cns.12151
Wang, X., Lou, N., Eberhardt, A., Yang, Y., Kusk, P., Xu, Q., et al. (2020). An ocular glymphatic clearance system removes β-amyloid from the rodent eye. Sci. Transl. Med. 12, eaaw3210. doi:10.1126/scitranslmed.aaw3210
Wang, X., Su, B., Lee, H. g., Li, X., Perry, G., Smith, M. A., et al. (2009). Impaired balance of mitochondrial fission and fusion in Alzheimer’s disease. J. Neurosci. 29 (28), 9090–9103. doi:10.1523/JNEUROSCI.1357-09.2009
Wang, Y., Jin, S., Sonobe, Y., Cheng, Y., Horiuchi, H., Parajuli, B., et al. (2014). Interleukin-1β induces blood–brain barrier disruption by downregulating sonic hedgehog in astrocytes. PLoS ONE 9 (10), e110024. doi:10.1371/journal.pone.0110024
Wei, M., Sun, Y., Li, S., Chen, Y., Li, L., Fang, M., et al. (2023). Single-cell profiling reveals Müller glia coordinate retinal intercellular communication during light/dark adaptation via thyroid hormone signaling. Protein and Cell. 14 (8), 603–617. doi:10.1093/procel/pwad007
Wen, L. Y., Wan, L., Lai, J. N., Chen, C. S., Chen, J. J. Y., Wu, M. Y., et al. (2021). Increased risk of Alzheimer’s disease among patients with age-related macular degeneration: a nationwide population-based study. PLoS ONE 16 (May), e0250440. doi:10.1371/journal.pone.0250440
Wilcock, D. M., Vitek, M. P., and Colton, C. A. (2009). Vascular amyloid alters astrocytic water and potassium channels in mouse models and humans with Alzheimer’s disease. Neuroscience 159 (3), 1055–1069. doi:10.1016/j.neuroscience.2009.01.023
Williams, M. A., McKay, G. J., Carson, R., Craig, D., Silvestri, G., and Passmore, P. (2015). Age-related macular degeneration–associated genes in alzheimer disease. Am. J. Geriatric Psychiatry 23 (12), 1290–1296. doi:10.1016/j.jagp.2015.06.005
Winkler, C. W., Foster, S. C., Matsumoto, S. G., Preston, M. A., Xing, R., Bebo, B. F., et al. (2012). Hyaluronan anchored to activated CD44 on central nervous system vascular endothelial cells promotes lymphocyte extravasation in experimental autoimmune encephalomyelitis. J. Biol. Chem. 287 (40), 33237–33251. doi:10.1074/jbc.M112.356287
Wong, T. Y., and Sabanayagam, C. (2020). Strategies to tackle the global burden of diabetic retinopathy: from epidemiology to artificial intelligence. Ophthalmologica 243 (1), 9–20. doi:10.1159/000502387
Wu, Y., Tang, D., Liu, N., Xiong, W., Huang, H., Li, Y., et al. (2017). Reciprocal regulation between the circadian clock and hypoxia signaling at the genome level in mammals. Cell. Metab. 25 (1), 73–85. doi:10.1016/j.cmet.2016.09.009
Xie, L., et al. (2021). Sleep drives metabolite clearance from the adult brain. Available at: https://www.science.org.
Xie, Y., et al. (2019). “New insights into the circadian rhythm and its related diseases,” in Frontiers in physiology (Lausanne, Switzerland: Frontiers Media S.A). doi:10.3389/fphys.2019.00682
Xu, L., Ruan, G., Dai, H., Liu, A. C., Penn, J., and McMahon, D. G. (2016). Mammalian retinal Müller cells have circadian clock function. Mol. Vis. 22, 275–283. Available at: http://www.molvis.org/molvis/v22/275.
Xue, Y., Xie, Y., Xue, B., Hu, N., Zhang, G., Guan, H., et al. (2016). Activated müller cells involved in ATP-induced upregulation of P2X7 receptor expression and retinal ganglion cell death. BioMed Res. Int. 2016, 1–9. doi:10.1155/2016/9020715
Yan, Z., Liao, H., Chen, H., Deng, S., Jia, Y., Deng, C., et al. (2017). Elevated intraocular pressure induces amyloid-β deposition and tauopathy in the lateral geniculate nucleus in a monkey model of glaucoma. Investigative Ophthalmol. Vis. Sci. 58 (12), 5434–5443. doi:10.1167/iovs.17-22312
Yang, T., Zhang, Y., Chen, L., Thomas, E. R., Yu, W., Cheng, Bo, et al. (2023). The potential roles of ATF family in the treatment of Alzheimer's disease. Biomed. Pharmacother. 161, 114544. doi:10.1016/j.biopha.2023.114544
Yin, X., Li, Y., Fan, X., Huang, F., Qiu, Y., Zhao, C., et al. (2022). SIRT1 deficiency increases O-GlcNAcylation of tau, mediating synaptic tauopathy. Mol. Psychiatry 27 (10), 4323–4334. doi:10.1038/s41380-022-01689-2
Zhang, D., Liu, Y., Tang, Y., Wang, X., Li, Z., Li, R., et al. (2018). Increased mitochondrial fission is critical for hypoxia-induced pancreatic beta cell death. PLoS ONE 13 (5), e0197266. doi:10.1371/journal.pone.0197266
Zhang, X., Li, A., Ge, J., Reigada, D., Laties, A. M., and Mitchell, C. H. (2007). Acute increase of intraocular pressure releases ATP into the anterior chamber. Exp. Eye Res. 85 (5), 637–643. doi:10.1016/j.exer.2007.07.016
Zhao, H., Chang, R., Che, H., Wang, J., Yang, L., Fang, W., et al. (2013). Hyperphosphorylation of tau protein by calpain regulation in retina of Alzheimer’s disease transgenic mouse. Neurosci. Lett. 551, 12–16. doi:10.1016/j.neulet.2013.06.026
Zhao, W.-Q., De Felice, F. G., Fernandez, S., Chen, H., Lambert, M. P., Quon, M. J., et al. (2008). Amyloid beta oligomers induce impairment of neuronal insulin receptors. FASEB J. 22 (1), 246–260. doi:10.1096/fj.06-7703com
Zhou, L., Gao, Q., Nie, M., Gu, J. L., Hao, W., Wang, L., et al. (2016). Degeneration and energy shortage in the suprachiasmatic nucleus underlies the circadian rhythm disturbance in ApoE-/- mice: implications for Alzheimer’s disease. Sci. Rep. 6, 36335. doi:10.1038/srep36335
Zhou, L. T., Liu, D., Kang, H. C., Lu, L., Huang, H. Z., Ai, W. Q., et al. (2023). Tau pathology epigenetically remodels the neuron-glial cross-talk in Alzheimer’s disease. Sci. Adv. 9 (16), eabq7105. doi:10.1126/sciadv.abq7105
Zhu, N., Wei, M., Yuan, L., He, X., Chen, C., Ji, A., et al. (2022). Claudin-5 relieves cognitive decline in Alzheimer's disease mice through suppression of inhibitory GABAergic neurotransmission. Aging 14 (8), 3554–3568. doi:10.18632/aging.204029
Zielinski, M. R., Kim, Y., Karpova, S. A., McCarley, R. W., Strecker, R. E., and Gerashchenko, D. (2014). Chronic sleep restriction elevates brain interleukin-1 beta and tumor necrosis factor-alpha and attenuates brain-derived neurotrophic factor expression. Neurosci. Lett. 580, 27–31. doi:10.1016/j.neulet.2014.07.043
Glossary
Keywords: Alzheimer’s disease, retinal neurodegeneration, circadian rhythm, glymphatic clearance, Muller glia, optical coherence tomography (OCT)
Citation: Das G and Milner TE (2024) Association of circadian dysregulation with retinal degeneration and Alzheimer’s disease: a special focus on Muller glial cells. Front. Photonics 5:1389683. doi: 10.3389/fphot.2024.1389683
Received: 22 February 2024; Accepted: 13 June 2024;
Published: 23 July 2024.
Edited by:
Stefan G. Stanciu, Polytechnic University of Bucharest, RomaniaReviewed by:
Gema Esquiva, University of Alicante, SpainLuz Maria Lopez-Marin, National Autonomous University of Mexico, Mexico
Copyright © 2024 Das and Milner. This is an open-access article distributed under the terms of the Creative Commons Attribution License (CC BY). The use, distribution or reproduction in other forums is permitted, provided the original author(s) and the copyright owner(s) are credited and that the original publication in this journal is cited, in accordance with accepted academic practice. No use, distribution or reproduction is permitted which does not comply with these terms.
*Correspondence: Glori Das, Z2RhczJAaG91c3Rvbm1ldGhvZGlzdC5vcmc=