- Department of Bioengineering, Northeastern University, Boston, MA, United States
Our team recently developed “Diffuse in vivo Flow Cytometry” (DiFC) for detection and enumeration rare circulating tumor cells (CTCs) in mice with highly-scattered fluorescent light. We have used DiFC to study dissemination of CTCs in a number of mouse models of metastasis with fluorescent protein expressing cells. Because DiFC uses diffuse light and interrogates large blood vessels in relatively deep tissue, in principle it could be translated to larger limbs, species, and even humans clinically. In this perspective, we discuss the technical challenges of human translation of DiFC in the context of the current state of the technology, as well as potential strategies for labeling of CTCs with targeted fluorescent molecular probes. We also discuss potential advantages and disadvantages of DiFC as a clinical tool. In principle, DiFC could represent a powerful complementary technique (to liquid biopsy blood draws) for accurate and sensitive measurement of changes in CTC numbers over time.
Introduction: Circulating Tumor Cells and Diffuse in Vivo Flow Cytometry
Although major efforts in biophotonics in oncology have historically focused on imaging of primary tumors, metastasis to distant organs and tissues is responsible for the majority of cancer-related deaths (Chaffer and Weinberg, 2011). One of the main pathways is hematogenous metastasis, wherein circulating tumor cells (CTCs) and multicellular CTC clusters (CTCCs) migrate from the primary tumor into the peripheral blood (PB) and form secondary sites (Steeg and Theodorescu, 2008; Hanahan and Weinberg, 2011). Because of this, CTCs have been a major focus of clinical and pre-clinical research outside of the biophotonics field (Joosse et al., 2015; Pantel and Speicher, 2016). In 2021 alone there were over 1900 journal publications on CTCs cataloged on Pubmed.gov.
There is a well-documented association between the number of CTCs in PB and the development of metastases, overall survival, and response to treatment for many cancers including breast, prostate and colorectal (Moreno et al., 2005; Cohen et al., 2008; Boutrus et al., 2013). CTCs are extremely rare. Fewer than one CTC per mL of PB is associated with reduced overall survival (Cristofanilli et al., 2004; Moreno et al., 2005). Clinical and pre-clinical interest in CTCs has driven major efforts in the field of “liquid biopsy”, wherein blood samples are drawn from a patient (or mouse) and analyzed (Hong and Zu, 2013). Typically, CTC isolation is a multi-step process involving red blood cell depletion followed by CTC selection based on epithelial cell surface markers or size (Alix-Panabières and Pantel, 2013). The only FDA cleared clinical test for CTCs is currently CellSearch (Raimondi et al., 2014), although there are many other platforms in development or in pre-clinical use.
In the context of pre-clinical research, there have been significant efforts in the last decade to develop and use specialized optical microscopy and photoacoustic methods for enumerating CTCs directly in vivo in mouse tumor models (Suo et al., 2020; Zharov et al., 2006; Hartmann et al., 2017). ‘Diffuse in vivo Flow Cytometry’ (DiFC) (Patil et al., 2019; Tan et al., 2019; Fitzgerald et al., 2020) is an emerging technique for enumeration of rare CTCs directly in the bloodstream of small animals. The concept is shown in Figure 1. DiFC uses diffuse light to sample hundreds of μL per minute of PB flowing in large arteries in a mouse hindleg or tail of a mouse. DiFC permitted highly sensitive detection of rare CTCs, particularly at early stages of disease progression (Patil et al., 2019; Fitzgerald et al., 2020).
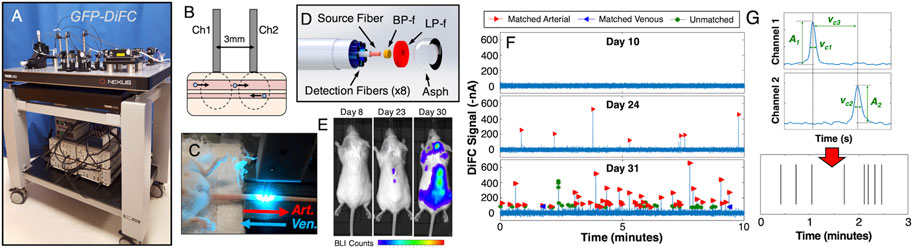
FIGURE 1. (A) Photograph of the blue-green DiFC instrument that was described in detail previously (Patil et al., 2019; Tan et al., 2019). DiFC allows detection of (B) cells traveling in large arteries and veins in bulk tissue large limbs of mice, typically either the (C) tail or leg. (D) We developed a specialized fiber probe design with integrated filters and lenses which allows detection of weak signals from single fluorescently labeled CTCs. DiFC can be used to monitor CTCs in circulation during tumor growth. For example, we used DiFC to non-invasively monitor rare CTC dissemination in a (E) multiple myeloma disseminated xenograft mouse model (Patil et al., 2019). (F) As CTCs appear in circulation, these are detected with DiFC as transient fluorescent peaks. (G) Using amplitude and temporal data from the two fiber probes, we are able to determine the number and direction of CTCs flowing in the blood vessel. These may be displaed as (G) raster plots, where each line vertical represents (in this case) the detection of a forward matched (arterial) CTC. Figure adapted with permission from (Patil et al., 2019).
We have built red (Tan et al., 2019), and blue-green (Patil et al., 2019) (Figure 1A) fluorescence versions of DiFC for use with common fluorophores and fluorescent proteins, including Cy5.5, Vybrant DiD, FiTC and GFP. DiFC uses a specially-designed fiber optic probes (Pera et al., 2017) in epi-reflectance geometry placed over a vessel in the limb (Figures 1B,C). As fluorescently-labeled CTCs pass through the DiFC field-of-view, transient “peaks” are detected on each probe. Diffusely emitted fluorescent light from moving cells is inherently very weak, and laser illumination of bulk biological tissue generates substantial non-specific tissue autofluorescence. As such we previously evaluated multiple illumination and detection designs for DiFC (Zettergren et al., 2012a; Zettergren et al., 2012b; Pestana et al., 2013) to maximize the detection signal-to-noise ratio (SNR) from a single cell. Most recently we developed specialized integrated fiber optic probes (Figure 1D) which were described in detail previously (Pera et al., 2017; Tan et al., 2019). Briefly, these use micromachined bandpass laser “cleanup” and long-pass fluorescence collection filters directly on the probe tip to minimize autofluorescence in the fiber assembly. The integrated collection lens also maximized geometric collection of fluorescent light from the region below the probe tip. We found that a short, 0.3 mm center-to-center source and detector fiber separation yielded the best SNR for cells moving in blood vessels less than 1 mm in depth.
Interrogation of large blood vessels allow DiFC to sample the entire circulating peripheral blood (PB) volume of a mouse in about 15 min. We showed that DiFC allowed detection of early CTC dissemination in multiple myeloma bearing mice (Figure 1E) with calibrated CTC numbers on the order of one cell per mL of PB (Figure 1F) (Patil et al., 2019). Use of two probes also allowed us to algorithmically (Figure 1G) identify cell direction, speed, and better reject motion artifacts and operate DiFC with very low false alarm rates (Tan et al., 2019). DiFC also allowed detection of CTC multicellular clusters by analysis of the amplitude and temporal width of detected peaks. This is very significant because CTCCs are known to have 50–100 times higher likelihood of forming metastasis than individual CTCs (Duda et al., 2010; Hong et al., 2016). In addition, because DiFC is non-invasive, it can be performed for extended periods, and repeatedly over time.
Could Diffuse in Vivo Flow Cytometry Be Translated to Humans?
Although our prior research efforts have been concentrated on studying mouse models of metastasis, an evident question is whether DiFC could ever be used in humans. In this section, we discuss the technical challenges of doing so in the context of the present state of the technology. The parallel (and equally important) issue of the potential value of DiFC as a clinical tool is discussed in detail in section 3 below. We also note that there are other optical approaches to in vivo detection of circulating cells under development. We do not discuss these in detail here for brevity, but these include, 1) photoacoustic methods which permit detection of highly pigmented CTCs such as melanoma cells (Hai et al., 2017; Galanzha et al., 2019). Photoacoustic methods are generally advantageous because they do not require exogenous contrast agents and can operate in deep tissue, 2) fluorescence confocal microscopy in vivo flow cytometry (Georgakoudi et al., 2004; Suo et al., 2020) methods, which are generally intended for use in small animal studies because they interrogate small, superficial blood vessels in the mouse ear, or 3) intrinsic contrast microscopy of circulating blood cells in the capillary bed (Li et al., 2010; Golan et al., 2012; McKay et al., 2021), which are advantageous because they permit detection of abundant blood cells without the use of contrast agents.
Tissue Optics
DiFC is inherently scalable to larger limbs and species because it uses highly scattered light, and because the probe is placed on the limb surface with the source and detector on the same side of the tissue, i.e., there is no requirement for trans-illumination of a limb. The abundant prior work in the diffuse optical tomography (DOT) and functional near infrared spectroscopy (fNIRS) fields indicate that optical interrogation of tissue depths on the order of centimeters are feasible (Jacques, 2013). Therefore, this is (in principle) an advantage of DiFC compared to fluorescence confocal microscopy methods, which are limited to relatively superficial depths (Hartmann et al., 2017). Moreover, DiFC uses laser powers of approximately 10–20 mW at the skin surface which is distributed over the 3 mm diameter probe tip. This exposure is below that at which skin heating is observed and would be in accordance with International Electrotechnical Commission (IEC) laser safety standards, which permits maximum exposures of approximately 300 mW per cm2 in the near infrared (NIR) range.
In our previous work in pre-clinical mouse models, we showed that DiFC detects cells traveling in blood vessels approximately 0.75–1 mm in depth (Patil et al., 2019; Tan et al., 2019). However, because of the inherently lower optical attenuation in biological tissues (Goldstein and Gupta, 2003), use of red or NIR fluorophores (as opposed to GFP) are better suited to interrogation of deeper-seated blood vessels as would be required in humans. There are several major candidate blood vessels in the human anatomy that are 2–4 mm deep that carry large blood volumes that could be accessible with DiFC such as the radial artery in the wrist (Goldstein and Gupta, 2003; Masengu et al., 2016). We recently performed an in-depth analysis of the optimal source-and-detector separation for potential use of DiFC in humans (Ivich et al., 2022). In brief, we showed that use of NIR fluorophores and a 3 mm source and detector separation should permit detection of labeled CTCs with SNR of at least 14 dB at depths of 2–4 mm. DiFC instrument development is an ongoing area of research in our group.
Contrast Agents
In contrast to mouse studies where cultured cancer cells can be readily transfected to express GFP prior to inoculation of tumors, any use of DiFC in humans would require the use of specific and bright fluorescent molecular contrast agents to label CTCs. Therefore, this is a significant technical and regulatory challenge for any potential use in humans. However, specific labeling of circulating cells with receptor-targeted probes has been achieved previously in small animals (Novak et al., 2004; Wei et al., 2005; He et al., 2007; Pitsillides et al., 2011; Patil et al., 2020) illustrating the general feasibility of the molecularly-targeted CTC labeling approach. As we have shown previously (Hartmann et al., 2017), because DiFC relies on detection of CTCs with diffuse light on a non-specific tissue background, the labeling requirements—in terms of fluorophore brightness and number of fluorophores per cell—are expected to be higher than confocal microscopy methods.
With respect to regulatory acceptance (i.e., FDA approval in the United States), the use of targeted fluorescent contrast agents in humans is rapidly gaining clinical traction, primarily for intraoperative fluorescence guided surgery (FGS) (Lee et al., 2019; Barth and Gibbs, 2020). If available FGS contrast agents have sufficient sensitivity, specificity, and brightness for CTCs, this suggests that human translation of DiFC could be expedited by “piggy backing” on this clinical progress.
For example, folate receptor (FR)-α is a frequently-used therapeutic and diagnostic target for cancer. FRα is over-expressed in many epithelial cancers (Parker et al., 2005; Leamon, 2008; Vlahov and Leamon, 2012) including ovarian (Vergote et al., 2015), breast (O'Shannessy et al., 2012), and non-small cell lung carcinoma (Shi et al., 2015). FRα also has very low expression in normal tissues and blood cells and therefore presents a relatively low background signal. One of the seminal papers in FGS by van Dam et. al. (van Dam et al., 2011) used a visible (FITC-based) FRα-targeted probe called EC17. Moreover, Low et. al. showed that EC17 had high affinity for ovarian and prostate cancer CTCs from patient blood samples and cultured cell lines, with better labeling efficiency than larger molecular weight antibody-based designs (He et al., 2008). In the context of CTC labeling in blood, non-specific uptake (e.g., by macrophages) is a serious concern, but the small-molecule design exhibited little uptake by white blood cells, with specificity for FR + CTCs by factor of at least 20 (He et al., 2008). More recently, our team (Patil et al., 2020) also confirmed this specificity using FR + CTCs in mouse blood with EC17 and found similar specificity (Figures 2A–F; see figure caption for details). Moreover, in the same study we showed that L1210A cells labeled with EC17 either prior to injection, or while in circulation (i.e., EC17 and L1210A cells were injected separately) were detectable with our existing blue-green DiFC system (Figures 2G–I).
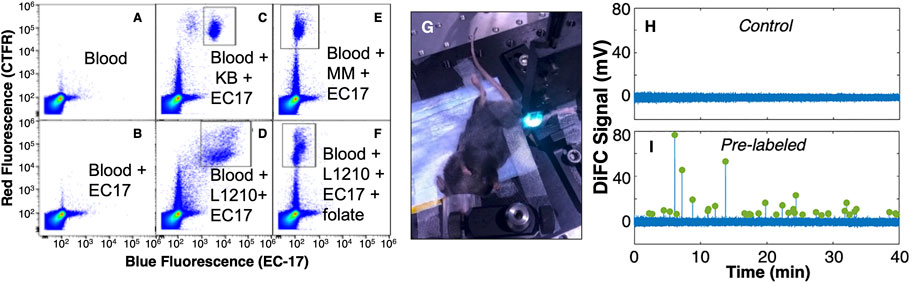
FIGURE 2. (A–F) Flow cytometry analysis of CTC labeling in whole blood with a FR-targeted molecular probe. Cells were pre-labeled with cell trace far red (CTFR; red y-axes) stain and added to whole blood, and EC-17 (blue; x-axes) added separately. (A) whole blood only, (B) whole blood with EC-17 added, showing minimal non-specific uptake of blood cells, blood with FR+ (C) KB, and (D) L1210 cells added, showing the population (square area) of EC-17 labeled CTCs. Likewis (E) FR- MM cells were not labeled by EC-17, and (F) addition of free-folate blocked binding in FR + L1210A cells. We also showed that these cells were detectable with DiFC in (G) C57BL/6 mice. (H) Un-injected control mice, and (I) mice with circulating EC-17 labeled L1210 cells. Peaks indicate example CTC detections. Figure adapted with permission from (Patil et al., 2020)
A near-infrared (NIR) analog of EC17 called OTL38 was recently approved for clinical use in ovarian and lung cancer. OTL-38 is also in phase-II trials for endometrial, brain, and kidney cancers. Because OTL38 is a longer wavelength contrast agent (780 nm excitation, 800 nm emission) than EC-17, as above it is in principle better suited significantly for deeper-tissue light penetration as would be necessary for use of DiFC in humans.
In addition to folate-targeted fluorescent probes, there are dozens of FGS molecular probes in various stages of clinical development. These efforts have been reviewed in detail elsewhere (Lee et al., 2019; Barth and Gibbs, 2020). Briefly, these include cancer associated molecular targets such as EGFR, Her2, αvβ3, integrin, Cathepsins, heat shock proteins (HSPs), and others. Multiple targeting strategies are being evaluated including small molecule, anti-body targeted, peptide, “activatable” (fluorogenic), and other nano-particle delivery systems. Many of these also have red and NIR spectral properties and therefore may be amenable to use with DiFC. Hence there are many potential CTC contrast agents that could be evaluated for DiFC in the future. In principle it could be possible to use a cocktail of contrast agents with different fluorophores to permit multiplexed profiling of CTCs, for example, to distinguish and enumerate epithelial and mesenchymal CTCs directly in vivo (Chaffer and Weinberg, 2011).
Rationale for Diffuse in Vivo Flow Cytometry Translation to Humans
Aside from technical and regulatory feasibility, it is important to consider how DiFC could be of potential value as a clinical tool. In particular: what potential advantages would DiFC offer given that there are already FDA-cleared microfluidic devices available that can isolate and enumerate CTCs from drawn patient blood samples? In this section we discuss some of the theoretical advantages and limitations of human translation of DiFC.
Analysis of Large Blood Volumes and More Accurate Enumeration of Circulating Tumor Cells
Although CTCs are crucial in metastasis formation, and there is clear established link between CTC numbers and metastasis in population studies, the true clinical value of CTC liquid biopsy in guiding individual patient management remains unclear (Raimondi et al., 2014). For example, in the well-known SWOGS0500 trial, CTC enumeration failed to demonstrate predictive value in guiding frontline chemotherapy for breast cancer (Smerage et al., 2014). One widely-held explanation for this is CTC heterogeneity (Polzer et al., 2014): because most CTCs do not form metastases, it is believed that specific CTC phenotypes may have higher likelihood of doing so (“metastatic potential”). This hypothesis has contributed to major efforts in development of next-generation liquid biopsy technologies that allow detailed genotypic and phenotypic characterization of individual CTCs (Bardia and Haber, 2014).
A complementary hypothesis is simply that enumerating CTCs from small blood samples (as in liquid biopsy) is inaccurate. Most CTC enumeration methods rely on analysis of 2—10 ml of PB, which fractionally represents 0.04—0.2% of the 5 L human PB volume. For CellSearch, CTC-positive patients are defined as having at least 5 [breast (Cristofanilli et al., 2004), prostate (Moreno et al., 2005)], or three CTCs [colorectal (Cohen et al., 2008)] per 7.5 ml sample (Miller et al., 2010), i.e. fewer than one CTC per mL blood. Even if the numbers of CTCs in a random blood draw follows a Poisson distribution (and, as we discuss below this is not necessarily true), it has been shown that fractionally small blood samples yield inaccurate quantitative estimates of CTCs (Allan and Keeney, 2010; Mishra et al., 2020).
We recently showed that this by analysis of DiFC data in mice (Williams et al., 2020). Specifically, we analyzed hundreds of hours of DiFC data from xenograft models. This allowed us to study the effect of blood sample size on CTC detection accuracy and sensitivity. For example, in Figure 3A each vertical line represents detection of a CTC in multiple myeloma (MM) bearing mouse in a 35 min scan as reported in Patil et al. (Patil et al., 2019). Our analysis showed that in general, because CTCs are rare and discreet (not continuous) detection events, a random small blood sample is likely to give a very inaccurate estimate of CTC burden: 1% of the PBV (Figures 3B,D) yielded large, short-term fluctuations in CTC count rate, and produced a correct estimate of CTC numbers (within 50%) only about 25% of the time on average. Sampling larger PBVs (e.g., 20%, Figures 3C,E) was more stable and allowed correct estimates of CTC burden > 90% of the time. Likewise, small blood samples frequently missed detection of rare CTCs entirely (Fitzgerald et al., 2020), whereas larger samples yielded more frequent detections (Figures 3F,G). In summary, larger blood volumes resulted in more sensitive and accurate enumeration of CTCs.
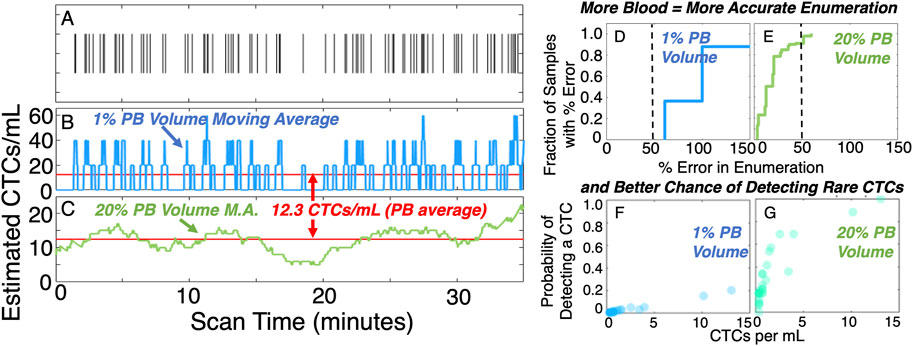
FIGURE 3. In general, analysis of larger blood samples gives more accurate and sensitive quantification of CTCs than smaller samples. (A) example raster plot showing CTC detections in a DiFC scan. The CTC count rate may vary dramatically in the timescale of a 35-minute scan. (B) Considering a moving average equivalent to 1% peripheral blood volume gives wide estimates of CTC numbers whereas, (C) moving averages equivalent to 20% peripheral blood volumes yield less noise. This leads to (D,E) more accurate quantification of CTC numbers, and (F,G) higher probability of detecting rare cells. Figure adapted with permission from (Williams et al., 2020).
In terms of candidate anatomical sites for DiFC the radial artery in the wrist or cephalic vein in the forearm carry more than 100 ml of blood per min (Goldstein and Gupta, 2003; Masengu et al., 2016). Optical sampling of these vessels would therefore sample 1 L of blood (∼20% of PBV) in minutes. Hence, in principle DiFC could yield more quantitatively accurate enumeration of CTCs which may better support their clinical utility.
Circulating Tumor Cells Numbers May be Dynamic Over the Timescale of Minutes, Hours, and Weeks
Furthermore, the assumption that CTC numbers in a blood sample should follow a Poisson distribution implicitly rests on the assumption that the number of CTCs in circulation do not change significantly in the minutes or hours surrounding a blood draw. Available pre-clinical and clinical evidence suggests that CTC numbers are more dynamic than is generally appreciated and may fluctuate over relatively short timescales (Juratli et al., 2014). CTC numbers and have been shown to follow circadian (diurnal) cycles in both mouse models (Zhu et al., 2021) and in humans (Paiva et al., 2013). Because they rely on drawing of a blood sample at a single time point, liquid biopsy methods are generally insensitive to these natural fluctuations that may occur.
Analysis of previously acquired DiFC data also indicates that this is true. Even considering 30–60 min scans (Figures 3A–C), the mean number of CTCs detected by DiFC frequently fluctuated with variability beyond that predicted by Poisson statistics or operator variability (Williams et al., 2020). Measurements taken over 24-hour cycles (Figures 4A–C), often showed more than an order-of-magnitude change in the mean MM CTC numbers (Williams et al., 2020). Similar fluctuations were observed day-to-day even without any obvious perturbations. In general, tumor volume does not necessarily correlate with tumor volume (Figure 5).
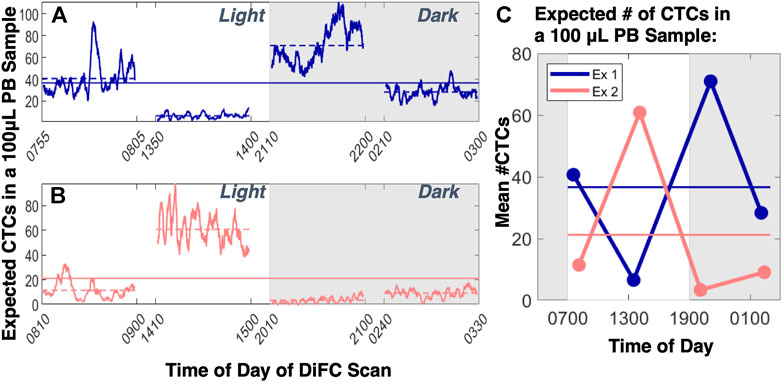
FIGURE 4. When DiFC was performed over 24-hour cycles in MM-bearing mice (two examples are shown in (A,B), large diurnal fluctuations in CTCs numbers were observed which would lead to (C) large differences in the mean number of CTCs that would be expected in a blood sample. This variability also far exceeded that expected from Poisson statistics or from operator variability. Figure adapted with permission from (Williams et al., 2020).
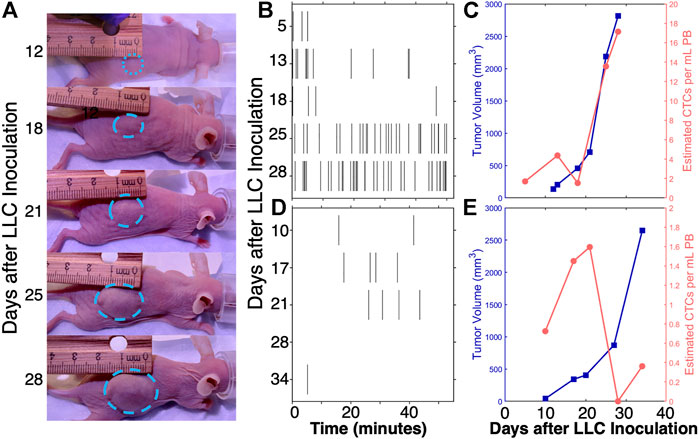
FIGURE 5. Consistent with the available literature, analysis of DiFC data shows that CTC numbers do not always vary directly with primary tumor size, and may flucuate substantially over the timescale of days. For example, in a (A) metastatic LLC tumor model (Fitzgerald et al., 2020). (B) CTC numbers generally increased as (C) tumors grew. However there was frequently significant heterogeneity (D,E) in this pattern. Figure adapted with permission from (Fitzgerald et al., 2020).
The overarching physical interpretation of these data is that CTCs undergo natural and fluctuations over time and are far from being in “steady state”. Practically, this means that the estimated number of CTCs from a blood sample will depend strongly on the timing of the blood draw. This should not be surprising: it is known that tumors shed CTCs at the rate of ∼106 C/g/day (Butler and Gullino, 1975), and that CTC half-life in circulation is only on the order of minutes (Georgakoudi et al., 2004; Stott et al., 2010). The issue is amplified for CTC clusters or subtypes, which are even more rare (Paiva et al., 2013).
In summary, human translation of DiFC could present a more accurate method of CTC enumeration, that is, less sensitive to temporal fluctuations in their numbers. Because little is known about whether and to what degree these fluctuations occur in humans, DiFC could represent a new research method to study these.
Limitations of Using Diffuse in Vivo Flow Cytometry in Humans
Finally, we briefly discuss limitations of any potential use of DiFC to humans. First, as above, DiFC would require administration of contrast agents. Although existing FGS contrast agents are purportedly well tolerated with only mild immune reactions reported in some cases (Lee et al., 2019), this presents a potential risk and regulatory barrier.
Second, DiFC would provide a real-time count of CTC and CTCC numbers. This would not directly allow—as is possible with liquid biopsy methods—isolation and detailed imaging, single-cell genomic, or phenotypic profiling of cells. Therefore, it is important to underscore that we do not view DiFC as a replacement for liquid biopsy methods—rather we view it as a potential complementary tool in the metastasis research toolbox. For example, use of DiFC could provide insight into early formation of metastasis, routine monitoring for recurrence of cancer, or possible mobilization of CTCs into circulation after anti-cancer therapies such as radiation therapy or surgery (Martin et al., 2017). Monitoring of CTC numbers could also be used to inform the timing of blood draws for subsequent analysis with liquid biopsy methods.
Summary
DiFC is an emerging method for non-invasive fluorescence enumeration of rare circulating cells directly in the bloodstream in vivo. Beyond small animal studies, translation of DiFC to humans could provide meaningful diagnostic information of cancer progression, recurrence, and response to therapy. The physics of near-infrared light propagation in biological tissue support the possibility of interrogating large blood vessels in human-scale geometries. A major outstanding challenging is identification of a clinically approved contrast agent for sensitive, specific, and bright labeling of CTCs. However, this may be achievable through major technical advances and investment in the field of fluorescence guided surgery.
Author Contributions
The author confirms being the sole contributor of this work and has approved it for publication.
Funding
This work was funded by the National Institutes of Health (R21CA246413 and R01EB024186).
Conflict of Interest
The authors declare that the research was conducted in the absence of any commercial or financial relationships that could be construed as a potential conflict of interest.
Publisher’s Note
All claims expressed in this article are solely those of the authors and do not necessarily represent those of their affiliated organizations, or those of the publisher, the editors and the reviewers. Any product that may be evaluated in this article, or claim that may be made by its manufacturer, is not guaranteed or endorsed by the publisher.
References
Alix-Panabières, C., and Pantel, K. (2013). Circulating Tumor Cells: Liquid Biopsy of Cancer. Clin. Chem. 59, 110–118. doi:10.1373/clinchem.2012.194258
Allan, A. L., and Keeney, M. (2010). Circulating Tumor Cell Analysis: Technical and Statistical Considerations for Application to the Clinic. J. Oncol. 2010, 426218. doi:10.1155/2010/426218
Bardia, A., and Haber, D. A. (2014). Solidifying Liquid Biopsies: Can Circulating Tumor Cell Monitoring Guide Treatment Selection in Breast Cancer? Jco 32, 3470–3471. doi:10.1200/jco.2014.57.1505
Barth, C. W., and Gibbs, S. L. (2020). Fluorescence Image-Guided Surgery - a Perspective on Contrast Agent Development. Proc. SPIE Int. Soc. Opt. Eng. 11222. doi:10.1117/12.2545292
Boutrus, R. R., Raad, R. F. A., Kuter, I., Ancukiewicz, M., Roberts, L., Solomon, N., et al. (2013). Circulating Tumor Cells as Predictors of Response and Failure in Breast Cancer Patients Treated with Preoperative Chemotherapy. Int. J. Biol. Markers 28, 17–23. doi:10.5301/jbm.2012.9580
Butler, T. P., and Gullino, P. M. (1975). Quantitation of Cell Shedding into Efferent Blood of Mammary Adenocarcinoma. Cancer Res. 35, 512–516.
Chaffer, C. L., and Weinberg, R. A. (2011). A Perspective on Cancer Cell Metastasis. Science 331, 1559–1564. doi:10.1126/science.1203543
Cohen, S. J., Punt, C. J. A., Iannotti, N., Saidman, B. H., Sabbath, K. D., Gabrail, N. Y., et al. (2008). Relationship of Circulating Tumor Cells to Tumor Response, Progression-free Survival, and Overall Survival in Patients with Metastatic Colorectal Cancer. Jco 26, 3213–3221. doi:10.1200/jco.2007.15.8923
Cristofanilli, M., Budd, G. T., Ellis, M. J., Stopeck, A., Matera, J., Miller, M. C., et al. (2004). Circulating Tumor Cells, Disease Progression, and Survival in Metastatic Breast Cancer. N. Engl. J. Med. 351, 781–791. doi:10.1056/nejmoa040766
Duda, D. G., Duyverman, A. M. M. J., Kohno, M., Snuderl, M., Steller, E. J. A., Fukumura, D., et al. (2010). Malignant Cells Facilitate Lung Metastasis by Bringing Their Own Soil. Proc. Natl. Acad. Sci. U.S.A. 107, 21677–21682. doi:10.1073/pnas.1016234107
Fitzgerald, J. E., Byrd, B. K., Patil, R. A., Strawbridge, R. R., Davis, S. C., Bellini, C., et al. (2020). Heterogeneity of Circulating Tumor Cell Dissemination and Lung Metastases in a Subcutaneous Lewis Lung Carcinoma Model. Biomed. Opt. Express 11, 3633–3647. doi:10.1364/boe.395289
Galanzha, E. I., Menyaev, Y. A., Yadem, A. C., Sarimollaoglu, M., Juratli, M. A., Nedosekin, D. A., et al. (2019). Erratum for the Research Article: "In Vivo Liquid Biopsy Using Cytophone Platform for Photoacoustic Detection of Circulating Tumor Cells in Patients with Melanoma" by E. I. Galanzha, Y. A. Menyaev, A. C. Yadem, M. Sarimollaoglu, M. A. Juratli, D. A. Nedosekin, S. R. Foster, A. Jamshidi-Parsian, E. R. Siegel, I. Makhoul, L. F. Hutchins, J. Y. Suen, V. P. Zharov. Sci. Transl. Med. 11. doi:10.1126/scitranslmed.aaz4393
Georgakoudi, I., Solban, N., Novak, J., Rice, W. L., Wei, X., Hasan, T., et al. (2004). In VivoFlow Cytometry. Cancer Res. 64, 5044–5047. doi:10.1158/0008-5472.can-04-1058
Golan, L., Yeheskely-Hayon, D., Minai, L., Dann, E. J., and Yelin, D. (2012). Noninvasive Imaging of Flowing Blood Cells Using Label-free Spectrally Encoded Flow Cytometry. Biomed. Opt. Express 3, 1455–1464. doi:10.1364/boe.3.001455
Goldstein, L. J., and Gupta, S. (2003). Use of the Radial Artery for Hemodialysis Access. Arch. Surg. 138, 1130–1134. doi:10.1001/archsurg.138.10.1130
Hai, P., Zhou, Y., Zhang, R., Ma, J., Li, Y., Shao, J. Y., et al. (2017). Label-free High-Throughput Detection and Quantification of Circulating Melanoma Tumor Cell Clusters by Linear-Array-Based Photoacoustic Tomography. J. Biomed. Opt. 22, 41004. doi:10.1117/1.JBO.22.4.041004
Hanahan, D., and Weinberg, R. A. (2011). Hallmarks of Cancer: the Next Generation. Cell 144, 646–674. doi:10.1016/j.cell.2011.02.013
Hartmann, C., Patil, R., Lin, C. P., and Niedre, M. (2017). Fluorescence Detection, Enumeration and Characterization of Single Circulating Cellsin Vivo: Technology, Applications and Future Prospects. Phys. Med. Biol. 63, 01TR01. doi:10.1088/1361-6560/aa98f9
He, W., Kularatne, S. A., Kalli, K. R., Prendergast, F. G., Amato, R. J., Klee, G. G., et al. (2008). Quantitation of Circulating Tumor Cells in Blood Samples from Ovarian and Prostate Cancer Patients Using Tumor-specific Fluorescent Ligands. Int. J. Cancer 123, 1968–1973. doi:10.1002/ijc.23717
He, W., Wang, H., Hartmann, L. C., Cheng, J.-X., and Low, P. S. (2007). In Vivo quantitation of Rare Circulating Tumor Cells by Multiphoton Intravital Flow Cytometry. Proc. Natl. Acad. Sci. U.S.A. 104, 11760–11765. doi:10.1073/pnas.0703875104
Hong, B., and Zu, Y. (2013). Detecting Circulating Tumor Cells: Current Challenges and New Trends. Theranostics 3, 377–394. doi:10.7150/thno.5195
Hong, Y., Fang, F., and Zhang, Q. (2016). Circulating Tumor Cell Clusters: What We Know and what We Expect (Review). Int. J. Oncol. 49, 2206–2216. doi:10.3892/ijo.2016.3747
Ivich, J. P. F., Williams, A. L., Shumel, M., Fang, Q., and Niedre, M. (2022). Signal and Measurement Considerations for Human Translation of Diffuse in Vivo Flow Cytometry. biorxiv.org. 2022.03.23.485369.
Jacques, S. L. (2013). Optical Properties of Biological Tissues: a Review. Phys. Med. Biol. 58, R37–R61. doi:10.1088/0031-9155/58/11/r37
Joosse, S. A., Gorges, T. M., and Pantel, K. (2015). Biology, Detection, and Clinical Implications of Circulating Tumor Cells. EMBO Mol. Med. 7, 1–11. doi:10.15252/emmm.201303698
Juratli, M., Sarimollaoglu, M., Nedosekin, D., Melerzanov, A., Zharov, V., and Galanzha, E. (2014). Dynamic Fluctuation of Circulating Tumor Cells during Cancer Progression. Cancers 6, 128–142. doi:10.3390/cancers6010128
Leamon, C. P. (2008). Folate-targeted Drug Strategies for the Treatment of Cancer. Curr. Opin. Investig. Drugs 9, 1277–1286.
Lee, J. Y. K., Cho, S. S., Stummer, W., Tanyi, J. L., Vahrmeijer, A. L., Rosenthal, E., et al. (2019). Review of Clinical Trials in Intraoperative Molecular Imaging during Cancer Surgery. J. Biomed. Opt. 24, 1–8. doi:10.1117/1.jbo.24.12.120901
Li, C., Pastila, R. K., Pitsillides, C., Runnels, J. M., Puoris’haag, M., Côté, D., et al. (2010). Imaging Leukocyte Trafficking In Vivo with Two-Photon-Excited Endogenous Tryptophan Fluorescence. Opt. Express 18, 988–999. doi:10.1364/oe.18.000988
Martin, O. A., Anderson, R. L., Narayan, K., and MacManus, M. P. (2017). Does the Mobilization of Circulating Tumour Cells during Cancer Therapy Cause Metastasis? Nat. Rev. Clin. Oncol. 14, 32–44. doi:10.1038/nrclinonc.2016.128
Masengu, A., McDaid, J., Maxwell, A. P., and Hanko, J. B. (2016). Preoperative Radial Artery Volume Flow Is Predictive of Arteriovenous Fistula Outcomes. J. Vasc. Surg. 63, 429–435. doi:10.1016/j.jvs.2015.08.106
McKay, G. N., Niemeier, R. C., Castro-González, C., and Durr, N. J. (2021). Scattering Oblique Plane Microscopy for In-Vivo Blood Cell Imaging. Biomed. Opt. Express 12, 2575–2585. doi:10.1364/boe.422993
Miller, M. C., Doyle, G. V., and Terstappen, L. W. (2010). Significance of Circulating Tumor Cells Detected by the CellSearch System in Patients with Metastatic Breast Colorectal and Prostate Cancer. J. Oncol. 2010, 617421. doi:10.1155/2010/617421
Mishra, A., Dubash, T. D., Edd, J. F., Jewett, M. K., Garre, S. G., Karabacak, N. M., et al. (2020). Ultrahigh-throughput Magnetic Sorting of Large Blood Volumes for Epitope-Agnostic Isolation of Circulating Tumor Cells. Proc. Natl. Acad. Sci. U.S.A. 117, 16839–16847. doi:10.1073/pnas.2006388117
Moreno, J. G., Miller, M. C., Gross, S., Allard, W. J., Gomella, L. G., and Terstappen, L. W. M. M. (2005). Circulating Tumor Cells Predict Survival in Patients with Metastatic Prostate Cancer. Urology 65, 713–718. doi:10.1016/j.urology.2004.11.006
Novak, J., Georgakoudi, I., Wei, X., Prossin, A., and Lin, C. P. (2004). In Vivo flow Cytometer for Real-Time Detection and Quantification of Circulating Cells. Opt. Lett. 29, 77–79. doi:10.1364/ol.29.000077
O'Shannessy, D. J., Somers, E. B., Maltzman, J., Smale, R., and Fu, Y. S. (2012). Folate Receptor Alpha (FRA) Expression in Breast Cancer: Identification of a New Molecular Subtype and Association with Triple Negative Disease. Springerplus 1, 22. doi:10.1186/2193-1801-1-22
Paiva, B., Paino, T., Sayagues, J.-M., Garayoa, M., San-Segundo, L., Martín, M., et al. (2013). Detailed Characterization of Multiple Myeloma Circulating Tumor Cells Shows Unique Phenotypic, Cytogenetic, Functional, and Circadian Distribution Profile. Blood 122, 3591–3598. doi:10.1182/blood-2013-06-510453
Pantel, K., and Speicher, M. R. (2016). The Biology of Circulating Tumor Cells. Oncogene 35, 1216–1224. doi:10.1038/onc.2015.192
Parker, N., Turk, M. J., Westrick, E., Lewis, J. D., Low, P. S., and Leamon, C. P. (2005). Folate Receptor Expression in Carcinomas and Normal Tissues Determined by a Quantitative Radioligand Binding Assay. Anal. Biochem. 338, 284–293. doi:10.1016/j.ab.2004.12.026
Patil, R. A., Srinivasarao, M., Amiji, M. M., Low, P. S., and Niedre, M. (2020). Fluorescence Labeling of Circulating Tumor Cells with a Folate Receptor-Targeted Molecular Probe for Diffuse In Vivo Flow Cytometry. Mol. Imaging Biol. 22, 1280–1289. doi:10.1007/s11307-020-01505-9
Patil, R., Tan, X., Bartosik, P., Detappe, A., Runnels, J. M., Ghobrial, I., et al. (2019). Fluorescence Monitoring of Rare Circulating Tumor Cell and Cluster Dissemination in a Multiple Myeloma Xenograft Model In Vivo. J. Biomed. Opt. 24, 1–11. doi:10.1117/1.jbo.24.8.085004
Pera, V., Tan, X., Runnels, J., Sardesai, N., Lin, C. P., and Niedre, M. (2017). Diffuse Fluorescence Fiber Probe for In Vivo Detection of Circulating Cells. J. Biomed. Opt. 22, 37004. doi:10.1117/1.JBO.22.3.037004
Pestana, N., Mortensen, L. J., Runnels, J. M., Vickers, D., Murthy, S. K., Lin, C. P., et al. (2013). Improved Diffuse Fluorescence Flow Cytometer Prototype for High Sensitivity Detection of Rare Circulating Cellsin Vivo. J. Biomed. Opt. 18, 077002. doi:10.1117/1.jbo.18.7.077002
Pitsillides, C. M., Runnels, J. M., Spencer, J. A., Zhi, L., Wu, M. X., and Lin, C. P. (2011). Cell Labeling Approaches for Fluorescence-Based In Vivo Flow Cytometry. Cytometry 79A, 758–765. doi:10.1002/cyto.a.21125
Polzer, B., Medoro, G., Pasch, S., Fontana, F., Zorzino, L., Pestka, A., et al. (2014). Molecular Profiling of Single Circulating Tumor Cells with Diagnostic Intention. EMBO Mol. Med. 6, 1371–1386. doi:10.15252/emmm.201404033
Raimondi, C., Gradilone, A., Naso, G., Cortesi, E., and Gazzaniga, P. (2014). Clinical Utility of Circulating Tumor Cell Counting through CellSearch(®): the Dilemma of a Concept Suspended in Limbo. Onco Targets Ther. 7, 619–625. doi:10.2147/OTT.S46200
Shi, H., Guo, J., Li, C., and Wang, Z. (2015). A Current Review of Folate Receptor Alpha as a Potential Tumor Target in Non-small-cell Lung Cancer. Drug Des. Devel Ther. 9, 4989–4996. doi:10.2147/DDDT.S90670
Smerage, J. B., Barlow, W. E., Hortobagyi, G. N., Winer, E. P., Leyland-Jones, B., Srkalovic, G., et al. (2014). Circulating Tumor Cells and Response to Chemotherapy in Metastatic Breast Cancer: SWOG S0500. Jco 32, 3483–3489. doi:10.1200/jco.2014.56.2561
Steeg, P. S., and Theodorescu, D. (2008). Metastasis: a Therapeutic Target for Cancer. Nat. Rev. Clin. Oncol. 5, 206–219. doi:10.1038/ncponc1066
Stott, S. L., Hsu, C.-H., Tsukrov, D. I., Yu, M., Miyamoto, D. T., Waltman, B. A., et al. (2010). Isolation of Circulating Tumor Cells Using a Microvortex-Generating Herringbone-Chip. Proc. Natl. Acad. Sci. U.S.A. 107, 18392–18397. doi:10.1073/pnas.1012539107
Suo, Y., Gu, Z., and Wei, X. (2020). Advances of In Vivo Flow Cytometry on Cancer Studies. Cytometry 97, 15–23. doi:10.1002/cyto.a.23851
Tan, X., Patil, R., Bartosik, P., Runnels, J. M., Lin, C. P., and Niedre, M. (2019). In Vivo Flow Cytometry of Extremely Rare Circulating Cells. Sci. Rep. 9, 3366. doi:10.1038/s41598-019-40143-2
van Dam, G. M., Themelis, G., Crane, L. M. A., Harlaar, N. J., Pleijhuis, R. G., Kelder, W., et al. (2011). Intraoperative Tumor-specific Fluorescence Imaging in Ovarian Cancer by Folate Receptor-α Targeting: First In-Human Results. Nat. Med. 17, 1315–1319. doi:10.1038/nm.2472
Vergote, I. B., Marth, C., and Coleman, R. L. (2015). Role of the Folate Receptor in Ovarian Cancer Treatment: Evidence, Mechanism, and Clinical Implications. Cancer Metastasis Rev. 34, 41–52. doi:10.1007/s10555-014-9539-8
Vlahov, I. R., and Leamon, C. P. (2012). Engineering Folate-Drug Conjugates to Target Cancer: from Chemistry to Clinic. Bioconjugate Chem. 23, 1357–1369. doi:10.1021/bc2005522
Wei, X., Sipkins, D. A., Pitsillides, C. M., Novak, J., Georgakoudi, I., and Lin, C. P. (2005). Real-time Detection of Circulating Apoptotic Cells by In Vivo Flow Cytometry. Mol. Imaging 4, 415–416. Oct-Dec. doi:10.2310/7290.2005.05148
Williams, A. L., Fitzgerald, J. E., Ivich, F., Sontag, E. D., and Niedre, M. (2020). Short-term Circulating Tumor Cell Dynamics in Mouse Xenograft Models and Implications for Liquid Biopsy. Front. Oncol. 10, 601085. doi:10.3389/fonc.2020.601085
Zettergren, E., Swamy, T., Runnels, J., Lin, C. P., and Niedre, M. (2012). Tomographic Sensing and Localization of Fluorescently Labeled Circulating Cells in Micein Vivo. Phys. Med. Biol. 57, 4627–4641. doi:10.1088/0031-9155/57/14/4627
Zettergren, E., Vickers, D., Runnels, J., Murthy, S. K., Lin, C. P., and Niedre, M. (2012). Instrument for Fluorescence Sensing of Circulating Cells with Diffuse Light in Mice In Vivo. J. Biomed. Opt. 17, 037001. doi:10.1117/1.jbo.17.3.037001
Zharov, V. P., Galanzha, E. I., Shashkov, E. V., Khlebtsov, N. G., and Tuchin, V. V. (2006). In Vivo photoacoustic Flow Cytometry for Monitoring of Circulating Single Cancer Cells and Contrast Agents. Opt. Lett. 31, 3623–3625. doi:10.1364/ol.31.003623
Keywords: optical devices, liquid biopsy, metastasis, fluorescence detection, circulating tumor cells, in vivo
Citation: Niedre M (2022) Prospects for Fluorescence Molecular In Vivo Liquid Biopsy of Circulating Tumor Cells in Humans. Front. Photonics 3:910035. doi: 10.3389/fphot.2022.910035
Received: 31 March 2022; Accepted: 22 April 2022;
Published: 09 May 2022.
Edited by:
Sylvain Gioux, Intuitive Surgical Sàrl, SwitzerlandReviewed by:
Aniruddha Ray, University of Toledo, United StatesQingqing Miao, Soochow University, China
Copyright © 2022 Niedre. This is an open-access article distributed under the terms of the Creative Commons Attribution License (CC BY). The use, distribution or reproduction in other forums is permitted, provided the original author(s) and the copyright owner(s) are credited and that the original publication in this journal is cited, in accordance with accepted academic practice. No use, distribution or reproduction is permitted which does not comply with these terms.
*Correspondence: Mark Niedre, bS5uaWVkcmVAbmV1LmVkdQ==