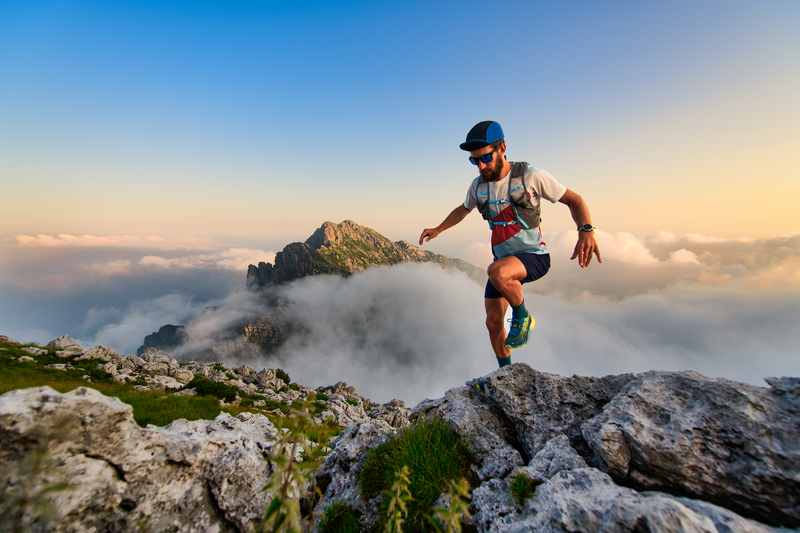
95% of researchers rate our articles as excellent or good
Learn more about the work of our research integrity team to safeguard the quality of each article we publish.
Find out more
MINI REVIEW article
Front. Photobiol. , 02 April 2025
Sec. Light Reactions of Photosynthesis
Volume 3 - 2025 | https://doi.org/10.3389/fphbi.2025.1571863
This article is part of the Research Topic Heterogeneity in Photobiology of Phototrophs – Emerging Questions and Microscopic Methods View all 3 articles
Diatoms, one of the most ubiquitous phytoplankton in the oceans, have evolved a pyrenoid-based CO2-concentrating mechanism (CCM) to utilize limited CO2 in seawater for photosynthesis. Recent proteomics analyses and molecular biological tools have deepened our understanding of the molecular mechanisms involved in diatom chloroplast architecture and the CCM. Here, we provide an update to our knowledge of the processes involved in high affinity photosynthesis for dissolved inorganic carbon (DIC) in diatoms. Based on the phenotype of genome-edited mutants, we propose a model of the diatom CCM composed of four phases of CO2-dependent photosynthesis at (I) less than 0.1 mM, (II) 0.1–2 mM, (III) 2–10 mM, and (IV) more than 10 mM of DIC concentrations, in which the rate-determining steps are the capture of unfixed CO2 in the chloroplast stroma at Phases I and II, the evolution of CO2 in the pyrenoid-penetrating thylakoid lumen at Phase III, and DIC transport to the stroma at Phase IV. Under natural seawater containing 2 mM DIC mainly in the form of HCO3−, the photosynthesis of marine diatoms is likely primarily in Phase III, shifting to Phase II when available CO2 is limited.
Marine diatoms are widely distributed and highly diversified phytoplankton group in the global oceans. They are among the most productive phytoplankton groups, with their photosynthesis contributing nearly half of oceanic primary production (Falkowski et al., 1998). Since dissolved inorganic carbon (DIC) mainly exists in the ionic form in natural seawater (ca. 2 mM of HCO3− >> ca. 16 μM of CO2 at 20°C), marine diatoms have evolved a system to utilize this large HCO3− pool for photosynthesis, the so-called biophysical CO2-concentrating mechanism (CCM) (Kaplan et al., 1980; Matsuda et al., 2001). The pennate diatom Phaeodactylum tricornutum and the centric Thalassiosira pseudonana are model species for which a variety of genetic engineering techniques have been developed. To date, molecular studies of these species have revealed the functions of many CCM components (Figure 1). In both species, extracellular CO2 is directly taken up by diatom cells. In P. tricornutum, external HCO3− is also directly taken up to the cytoplasm by active transporters such as solute carrier four family proteins (SLC4-1, SLC4-2, and SLC4-4) (Nakajima et al., 2013; Nawaly et al., 2023a), while T. pseudonana in contrast indirectly uptakes external HCO3− via dehydration by extracellular carbonic anhydrases (CA) (Tsuji et al., 2017a). Cytosolic pH was estimated to be 7.4 in Thalassiosira weissflogii (Hervé et al., 2012) and 7.9 in P. tricornutum (Shimakawa et al., 2023b), and at this pH DIC should be kept mainly as HCO3− in the cytosol. Diatom chloroplasts are comprised of four-layered membranes (Keeling, 2010; Flori et al., 2016; Cavalier-Smith, 2018). Chloroplast intermembrane CAs have been found in both P. tricornutum and T. pseudonana (Tachibana et al., 2011; Jensen et al., 2019). The periplastidal compartment (PPC) between the two outer and two inner membranes is maintained at an acidic pH, implying that cytosolic HCO3− is actively transported into PPC and then converted to CO2 by intermembrane CAs to be passively diffused across the two inner chloroplast envelopes to the stroma (Shimakawa et al., 2023b). Meanwhile, it is also possible that intermembrane CAs rather convert CO2 to HCO3− to suppress CO2 leakage from the chloroplast stroma (Tsuji et al., 2017b; Jensen et al., 2019). Assuming the four-layer membrane system is beneficial to the diatom CCM, the CA-dependent CO2 diffusion barrier would be located between the cytosol and PPC, i.e., chloroplast endoplasmic reticulum (CER) lumen, although this model of DIC dynamics across the chloroplast membranes still remains to be demonstrated (Figure 1). Stromal pH is 8.0 or higher (Anning et al., 1996; Shimakawa et al., 2023b), and CO2 is trapped there in the form of HCO3−, presumably via hydration by stromal CAs (Tsuji et al., 2017b; Nawaly et al., 2023b). Overall, extracellular DIC in alkaline seawater is taken up across the plasma membrane with a strategy either extracellular-CA mediated CO2 diffusion or direct HCO3− transport, and they are finally pumped into the chloroplast stroma mainly via active transport across chloroplast membranes (Hopkinson et al., 2011). The HCO3− pumping process across chloroplast membranes is likely to be fast enough to maintain the cytosolic CO2 lower than external CO2, thus enabling the influx of external CO2 even without plasma membrane HCO3− transporter (Hopkinson et al., 2011). In either case, external DIC acquisition across the plasma membrane is, in principle, not the rate-determining step for diatom photosynthesis.
Figure 1. Multiphase CO2-dependent photosynthesis in the marine diatom Phaeodactylum tricornutum. Cytoplasmic membranes (grey line), chloroplast endoplasmic reticulum (CER) envelopes (wine red dashed line), periplastidal membranes (red line), outer and inner plastid membranes (dashed and solid green lines), thylakoid membranes (brown line), and pyrenoid shells (blue dotted line) are drawn to separate each compartment. P. tricornutum possesses carbonic anhydrases (CAs) in the cytosol (θ-type), periplastidal compartment (PPC; α- and possibly ℩-types), stroma (β-type), and the lumen of pyrenoid-penetrating thylakoid membranes (θ-type; Tachibana et al., 2011; Kikutani et al., 2016; Tsuji et al., 2017b). Solute carrier four family proteins (SLC4), stromal β-CAs, and the major bestrophin-like protein (BST1) are highly expressed in low dissolved inorganic carbon (DIC) concentrations. Thickness of each arrow roughly indicates the flux of diffusion, transport, and reaction. Phase I could be illustrated similarly to Phase II. Dynamics of DIC across four-layer chloroplast membranes (sky blue shadings) still remains to be demonstrated.
In diatom chloroplasts, there is a triple-layered thylakoid membrane called the girdle lamella with a layered stromal thylakoid in its interior, where the photosynthetic electron transport chain produces NADPH and ATP for CO2 assimilation in the Calvin-Benson-Bassham cycle. In this process, H2O is oxidized with O2 evolution at the luminal side of photosystem II (PSII), and the electrons passed to the PSII reaction center are transported to photosystem I (PSI) through plastoquinone, cytochrome (Cyt) b6f complex, and Cyt c6. This linear electron transport process is accompanied by the generation of a proton concentration gradient (ΔpH) and an electric field gradient (ΔΨ) across the thylakoid membranes, which both function in the proton motive force driving ATP production by chloroplast ATP synthase (Witt, 1979). In P. tricornutum, the bestrophin-like protein (BST) is widely distributed throughout the stroma thylakoid, playing a role in transporting HCO3− into the lumen (Nigishi et al., 2024). In the middle of the stroma, there is a phase-separated proteinaceous body called the pyrenoid (Bedoshvili et al., 2009), which is a condensate body of ribulose 1,5-bisphosphate carboxylase/oxygenase (Rubisco) interlinked by a intrisically disorder protein, PYCO1 (Oh et al., 2023). Two layered thylakoid membranes traverse along with the long axis of the oval shaped pyrenoid (Flori et al., 2016), which are termed the pyrenoid-penetrating thylakoid (PPT) membrane (Nigishi et al., 2024). The θ-type CA exists on the luminal side of PPT to convert HCO3− to CO2 in the low pH of the PPT lumen under illumination (Kikutani et al., 2016; Shimakawa et al., 2023a). Due to the high membrane permeability of CO2, evolved luminal CO2 quickly effluxes to the pyrenoid and is fixed by Rubisco. This system was previously defined as the “CO2-evolving machinery” (Shimakawa et al., 2023a). Further, unfixed CO2 should be converted back to HCO3− by stromal CAs and transported again into the lumen of stroma thylakoid membranes by BST. It has been shown recently that the pyrenoid is surrounded by a sheet composed of a lattice-like protein sheath named Pyrenoid-Shell (PyShell) (Shimakawa et al., 2024a). It is possible that the PyShell sheet functions as a part of CO2 diffusion barrier between the chloroplast stroma and the pyrenoid matrix. The strategy to utilize external DIC for photosynthesis should be diversified among diatom species dependent on their ecological niche. Nevertheless, central CCM components in the chloroplast, including stromal/luminal CA, BST, and PyShell are widely conserved in diatoms at the genetic level. Indeed, the centric diatom T. pseudonana also possesses stromal CA, thylakoid-luminal CA, thylakoid-localized BST, and PyShell proteins (Nawaly et al., 2023b; Nam et al., 2024; Nigishi et al., 2024). Overall, we speculate that the “CO2-evolving machinery” we modeled in P. tricornutum and T. pseudonana is a central and evolutionary-conserved system of the diatom CCM.
The efficiency of the algal CCM can be evaluated by measuring the dependency of photosynthetic activity (O2 evolution rate) on total external DIC concentrations ([DIC]) reflected in the so-called “P-DIC curve” (Figure 2). The wild type P. tricornutum (PtWT) shows the maximum photosynthetic activity (Pmax) even at very low [DIC], and the [DIC] giving half of Pmax (K0.5) is ca. 0.01–0.05 mM (Matsuda et al., 2001; Shimakawa et al., 2023a; Nigishi et al., 2024). This order of K0.5 value is also observed in other marine diatoms fully acclimated to low CO2 conditions (Tsuji et al., 2017a; Tsuji et al., 2021; Shimakawa et al., 2024a). In P. tricornutum, several CCM components, including plasma membrane-localized SLC4s, stromal β-CAs, and BST1, are highly downregulated at the transcript levels in 1–5% CO2 conditions (high CO2) (Ohno et al., 2011; Nakajima et al., 2013; Nigishi et al., 2024), resulting in the higher K0.5 (approximately 0.2–0.8 mM) in PtWT when the cells are grown in high CO2 bubbling (Matsuda et al., 2001; Shimakawa et al., 2023a; Nigishi et al., 2024). This indicates that CCM is repressed by high CO2 growth conditions.
Figure 2. Photosynthetic affinity with dissolved inorganic carbon (DIC) in the wild type (PtWT) and the genome editing mutants (ΔPtBST1 and ΔPtθ-CA1) in the marine diatom Phaeodactylum tricornutum. The representative data in Shimakawa et al. (2023a) and Nigishi et al. (2024) are used after normalization of net O2 evolution rates (µmol mg−1 Chl a h−1) to Pmax as 100%. Grey circles, atmospheric CO2 (air)-grown PtWT; black stars, 1% (high) CO2-grown PtWT; light purple triangles, air-grown ΔPtBST1; purple inverted triangles, high CO2-grown ΔPtBST1; light green squares, air-grown ΔPtθ-CA1; green diamonds, high CO2-grown ΔPtθ-CA1.
Recent advances in molecular biology make it possible to isolate genome-edited mutants deficient in CCM components in P. tricornutum and T. pseudonana. We have found notable differences in P-DIC curve in two mutants defective of BST1 and PPT luminal θ-CA in P. tricornutum. The knock-out mutants of BST1 (ΔPtBST1) showed the higher K0.5 (around 0.2 mM) than that in PtWT (Figure 2). This value is close to K0.5 in high-CO2 grown PtWT, suggesting that the BST is the important component that contributes to the induced-level DIC affinity in PtWT grown in sub-atmospheric CO2 conditions. Meanwhile, the K0.5 in ΔPtBST1 slightly increased when the cells were grown under high CO2, indicating that other low CO2 inducible CCM components such as plasma membrane-localized SLC4s and stromal β-CAs also contribute to the CCM induction (Nigishi et al., 2024). In the knock-out mutants of the PPT luminal θ-CA (ΔPtθ-CA1), we observed the extremely low photosynthetic affinity for DIC than that in PtWT, giving K0.5 exceeding 2 mM in both air- and high CO2-grown cells of ΔPtθ-CA1 (Figure 2) (Shimakawa et al., 2023a), indicating that low CO2 inducible CCM components cannot rescue the deficit of the CA localized in the PPT lumen. Importantly, a very similar null CCM phenotype was also observed in the genome edited knock-out mutant of PyShells in T. pseudonana, a strain that fails to construct a proper pyrenoid structure with PPT at the core (Shimakawa et al., 2024a). We thus model that pyrenoid structure harboring the right arrangement of PPT is a critical factor for the CO2 evolving machinery and that PPT luminal CA is a pivotal component in this machinery operation.
Based on the [DIC] required for Pmax in P-DIC curves shown in Figure 2, we defined several phases of CO2-dependent photosynthesis in diatoms: Phase I, less than 0.1 mM; Phase II, 0.1–2 mM; Phase III, 2–10 mM; and Phase IV, more than 10 mM of [DIC], which differ in their rate-determining steps of CO2 utilization for photosynthesis (Figure 1).
At Phase I, photosynthesis is limited by very low levels concentrations of DIC (∼0.1 mM), which are much smaller than the abundance of DIC in natural seawater (ca. 2 mM). Such a strong CO2 limitation may arise in natural tide pools and artificial culture tanks containing heavily concentrated cells. Less than 0.1 mM DIC in natural seawater contains less than 0.001 mM CO2 which is far lower than the Michaelis constant for the carboxylation reaction of Rubisco (0.052 mM and 0.036 mM with and without atmospheric O2) (Young et al., 2016). This indicates that CO2 in the pyrenoid matrix is higher than the external one. Thus, CO2 is literally “concentrated” by a factor way exceeding 50, perhaps approaching 100 times. In this situation, HCO3− is actively pumped first into the chloroplasts by chloroplast membrane transporters and finally to the thylakoid lumen, while CO2 leakage is strictly prevented in the chloroplast stroma. During the operation of the chloroplastic CCM, a steep drawdown of cytosolic DIC occurs, but it is replenished quickly by efficient HCO3− transport across plasma membrane from the environment. Overall, maximum photosynthesis is fulfilled by CCM components in PtWT grown in atmospheric CO2 conditions (Figure 1), and the range of [DIC] giving Phase I defines the exact limit of CCM. The rate-determining step of photosynthesis in diatom cells at Phase I is not yet evidenced, but it is presumably the same as that at Phase II (described below). We note that P. tricornutum has relatively higher photosynthetic affinity for DIC (lower K0.5) even among diatom species (Tsuji et al., 2017a), which could be due to the high ability to prevent CO2 leakage from chloroplasts.
The P-DIC curve in ΔPtBST1 (Figure 2) suggests that the transport of HCO3− from the chloroplast stroma into the lumen of thylakoid membranes is a major rate-determining step for cells in a DIC range between 0.1 and 2 mM. The HCO3− transport by BST should depend on (i) the permeability of HCO3−, (ii) the gradient of HCO3− concentrations, and (iii) ΔΨ across thylakoid membranes. Thylakoid HCO3− permeability is determined by the abundance of channels, corresponding to the balance of synthesis and degradation of BST. Indeed, PtWT grown under 1% CO2 showed little expression of BST1 and exhibited a similar affinity for DIC during CO2 assimilation to that in the ΔPtBST1 mutants (Nigishi et al., 2024). In P. tricornutum, the active DIC pumping into chloroplasts would be powerful enough to supply the chloroplast stroma with enough HCO3− to provide a saturating concentration of CO2 for Rubisco, at least, where the external [DIC] is more than 0.1 mM. Additionally, stromal CA is expected to play an important role in preventing CO2 leakage and maintaining adequate levels of stromal DIC. Without the leakage barrier, CO2 could quickly diffuse out of the chloroplast, reducing the efficiency of the CCM. In the CCM model in the green alga Chlamydomonas reinhardtii, the occurrence of a leakage barrier at the pyrenoid could account for their role of CCM in the cells grown in the atmospheric CO2 (Fei et al., 2022). Perhaps, the leakage barrier model could be applicable to the CCM at Phase II in diatoms. In these contexts, stromal CA would mainly play a CO2-leakage resistant role in diatom chloroplasts at Phase II. Furthermore, PyShell and the stroma-thylakoid membranes surrounding the pyrenoid could be a physical barrier against the diffusion of CO2. It should be noted that such physical barrier could also prevent O2 influx into the pyrenoid. In diatoms, there is also an additional system that could work as a leakage barrier of CO2 from the chloroplast; that is, the four-layered membrane system of chloroplast. This complicated membrane system comprises two additional matrices specific to chloroplasts derived from secondary endosymbiotic events (like diatoms) but absent in chloroplasts of primary endosymbionts, that is the CER lumen and PPC. Indeed, there are numerous CAs located in these membrane matrices even though the exact matrix that each CA is present in has not yet been determined (Tachibana et al., 2011; Samukawa et al., 2014; Jensen et al., 2019). The active HCO3− transporters on the plasma membrane and the chloroplast membranes are less critical in Phase II relative to Phase I, but still important to maintain DIC levels against the drawdown in the cytosol and the stroma. In thylakoid membranes, photosynthetic electron transport is driven by light energy to generate ΔΨ as a component of the proton motive force, implying that the HCO3− transport via PtBST1 is promoted under illumination. In nature, marine diatoms could be easily exposed to the low [DIC] characteristic of Phase II, either where the cell concentrations are high or where the DIC supply is limited.
Here, we defined the [DIC] at Phase III as 2–10 mM, which is equal to or more than the [DIC] in natural seawater. In Phase III, ΔPtθ-CA1 does not achieve Pmax (Figure 2), indicating that the CO2-evolving machinery in PPT membranes is a rate-determining step for photosynthesis at these levels. In other words, CO2 is not supplied quickly enough to Rubisco in the pyrenoid matrix without the CO2-evolving machinery even at mM-order stromal [DIC]. The DIC equilibration should be largely shifted to HCO3− in the stroma (pH 8.0), resulting in 0.077 mM CO2 even for 10 mM total DIC at 20°C, in which the Rubisco carboxylation would show only 60–70% activity of Vmax (Young et al., 2016). Additionally, Rubisco is densely packed in the pyrenoid. Because of this, in the absence of the CO2 evolving machinery, CO2 diffusing into the pyrenoid from the surrounding stroma is quickly fixed by Rubisco on the periphery of the pyrenoid. This may be especially true in the case of P. tricornutum, where the pyrenoid is expected to be immobile (Oh et al., 2023). Nevertheless, the CO2-dependent photosynthesis in ΔPtθ-CA1 almost reaches Pmax at 10 mM DIC, indicating that the stromal [DIC] should be maintained much higher than extracellular [DIC] in P. tricornutum also at Phase III owing to the function of CO2-evolving machinery. The difference in K0.5 between atmospheric and high CO2 grown ΔPtθ-CA1 (Figure 2) (Shimakawa et al., 2023a) would be derived from low CO2 inducible components for the HCO3− transport (Nakajima et al., 2013). We mention again that neither the PPT luminal CA nor the proper pyrenoid structure with PPT are dispensable for marine diatoms to achieve Pmax within 10 mM of [DIC] (Shimakawa et al., 2023a; Shimakawa et al., 2024a). Overall, under DIC conditions generally found in nature, the CO2 substrate for diatom Rubisco is dominantly supplied from the lumen of PPT membranes at the pyrenoid core.
At Phase IV (more than 10 mM external [DIC]), extracellular CO2 should be directly taken up to the cells and passively diffused to the chloroplast stroma. Additionally, external HCO3− is actively pumped into chloroplasts to keep the stromal [DIC] higher than the outside of cells. We note that the CO2-evolving machinery is dispensable at Phase IV, and CO2 directly delivered from the stroma to Rubisco in the pyrenoid matrix is enough to produce Pmax in P. tricornutum. Such extremely high DIC concentration likely does not occur in much of the present ocean environment, but it does describe some unique situations such as an artificial culture tank bubbled with high CO2 gas and (potentially) a future high CO2 world of more than 5 times current pCO2. Approximately 0.3 mM CO2 can fulfill the maximum turnover of Rubisco carboxylation (Young et al., 2016), which corresponds to 40 mM total DIC at pH 8.0. In such an absolutely unnatural situation, CO2 could be supplied via passive diffusion, and marine diatoms would not require the use of a CCM. Nevertheless, the pyrenoid structure is maintained even when bubbling in 1% CO2 in P. tricornutum (Kikutani et al., 2016).
Here, we defined multiple phases of CO2-dependent photosynthesis in marine diatoms, based on the phenotype of genome-edited mutants deficient in pyrenoid structure and CO2-evolving machinery. In natural marine environments containing 2 mM DIC, Phase II and Phase III are, respectively, assumed to be the conditions with and without the limitation of CO2 availability, in which the incorporation of HCO3− into the thylakoid lumen and the PPT architecture mainly support photosynthetic CO2 assimilation. The CO2-evolving machinery should be functionally coupled with photosynthetic electron transport on thylakoid membranes, because lumen acidification is theoretically essential for the conversion of HCO3− to CO2. Indeed, it has been demonstrated that alternative electron transports promote the formation of ΔpH to support CCM in C. reinhardtii (Burlacot et al., 2022), although it is unclear if this occurs in diatoms. On the contrary, the HCO3− transport into the thylakoid lumen may indirectly decrease ΔpH to relieve heat dissipation of excess light energy (the so-called qE quenching), which is a possible combination of light and CO2 utilization by diatom cells (Nigishi et al., 2024). It should be also noted that diatom cells evolve CO2 also at tricarboxylic acid cycle and urea cycle in mitochondria (Allen et al., 2011). However, restoration of DIC from mitochondria to chloroplasts is still elusive in diatoms (Shimakawa et al., 2024b).
The green alga C. reinhardtii, another well studied algal species in CCM research, shows some similarities to the diatom CCM in its DIC utilization strategy for photosynthesis, which suggests convergent evolution of the pyrenoid-based CCM in a variety of algae. For example, the LCIB-LCIC complex, which is a most probably θ-type CA complex, and BST function in blocking leakage and recycling the unfixed CO2 in chloroplasts (Yamano et al., 2010; Mukherjee et al., 2019), and the α-type CA evolves CO2 in the lumen of pyrenoid tubules (Raven, 1997), which can be defined as functional analogs to the essential components for Phase II and Phase III in the photosynthesis of P. tricornutum. In C. reinhardtii, the pyrenoid disperses at Phase IV, which may have the advantage for Rubisco to utilize CO2 in the stroma. Importantly, the chloroplast and pyrenoid architecture are likely to be largely different among different eukaryotic algal species (He et al., 2023). Pyrenoids lacking thylakoid-penetrating membranes are observed in many algae, and the existence of genes encoding CA and BST is also varied. Although the multiphase model of CCM fits to P. tricornutum, T. pseudonana, and presumably C. reinhardtii, it still remains to be seen if it accurately describes the CO2-concentrating and -evolving strategies of other phytoplankton on the earth.
GS: Writing–original draft, Writing–review and editing. YM: Writing–original draft, Writing–review and editing.
The author(s) declare that financial support was received for the research and/or publication of this article. This work was supported by JSPS KAKENHI (24H02102 to GS; 19H01153 to YM) and by JST CREST “Cell dynamics” (JPMJCR20E1 to YM).
We would like to thank Dr. Matthew B. Brown (Kwansei Gakuin University) for kindly proofreading our English writing.
The authors declare that the research was conducted in the absence of any commercial or financial relationships that could be construed as a potential conflict of interest.
The author(s) declare that no Generative AI was used in the creation of this manuscript.
All claims expressed in this article are solely those of the authors and do not necessarily represent those of their affiliated organizations, or those of the publisher, the editors and the reviewers. Any product that may be evaluated in this article, or claim that may be made by its manufacturer, is not guaranteed or endorsed by the publisher.
Allen, A. E., Dupont, C. L., Oborník, M., Horák, A., Nunes-Nesi, A., McCrow, J. P., et al. (2011). Evolution and metabolic significance of the urea cycle in photosynthetic diatoms. Nature 473, 203–207. doi:10.1038/nature10074
Anning, T., Nimer, N., Merrett, M. J., and Brownlee, C. (1996). Costs and benefits of calcification in coccolithophorids. J. Mar. Syst. 9, 45–56. doi:10.1016/0924-7963(96)00015-2
Bedoshvili, Y. D., Popkova, T. P., and Likhoshway, Y. V. (2009). Chloroplast structure of diatoms of different classes. Cell Tissue Biol. 3, 297–310. doi:10.1134/S1990519X09030122
Burlacot, A., Dao, O., Auroy, P., Cuiné, S., Li-Beisson, Y., and Peltier, G. (2022). Alternative photosynthesis pathways drive the algal CO2-concentrating mechanism. Nature 605, 366–371. doi:10.1038/s41586-022-04662-9
Cavalier-Smith, T. (2018). Kingdom Chromista and its eight phyla: a new synthesis emphasising periplastid protein targeting, cytoskeletal and periplastid evolution, and ancient divergences. Protoplasma 255, 297–357. doi:10.1007/s00709-017-1147-3
Falkowski, P. G., Barber, R. T., and Smetacek, V. (1998). Biogeochemical controls and feedbacks on ocean primary production. Science 281, 200–207. doi:10.1126/science.281.5374.200
Fei, C., Wilson, A. T., Mangan, N. M., Wingreen, N. S., and Jonikas, M. C. (2022). Modelling the pyrenoid-based CO2-concentrating mechanism provides insights into its operating principles and a roadmap for its engineering into crops. Nat. Plants 8, 583–595. doi:10.1038/s41477-022-01153-7
Flori, S., Jouneau, P.-H., Finazzi, G., Maréchal, E., and Falconet, D. (2016). Ultrastructure of the periplastidial compartment of the diatom Phaeodactylum tricornutum. Protist 167, 254–267. doi:10.1016/j.protis.2016.04.001
He, S., Crans, V. L., and Jonikas, M. C. (2023). The pyrenoid: the eukaryotic CO2-concentrating organelle. Plant Cell 35, 3236–3259. doi:10.1093/plcell/koad157
Hervé, V., Derr, J., Douady, S., Quinet, M., Moisan, L., and Lopez, P. J. (2012). Multiparametric analyses reveal the pH-dependence of silicon biomineralization in diatoms. PLoS One 7, e46722. doi:10.1371/journal.pone.0046722
Hopkinson, B. M., Dupont, C. L., Allen, A. E., and Morel, F. M. M. (2011). Efficiency of the CO2-concentrating mechanism of diatoms. Proc. Natl. Acad. Sci. U.S.A. 108, 3830–3837. doi:10.1073/pnas.1018062108
Jensen, E. L., Clement, R., Kosta, A., Maberly, S. C., and Gontero, B. (2019). A new widespread subclass of carbonic anhydrase in marine phytoplankton. ISME J. 13, 2094–2106. doi:10.1038/s41396-019-0426-8
Kaplan, A., Badger, M. R., and Berry, J. A. (1980). Photosynthesis and the intracellular inorganic carbon pool in the blueren alga Anabaena variabilis: response to external CO2 concentration. Planta 149, 219–226. doi:10.1007/BF00384557
Keeling, P. J. (2010). The endosymbiotic origin, diversification and fate of plastids. Philos. Trans. R. Soc. B Biol. Sci. 365, 729–748. doi:10.1098/rstb.2009.0103
Kikutani, S., Nakajima, K., Nagasato, C., Tsuji, Y., Miyatake, A., and Matsuda, Y. (2016). Thylakoid luminal θ-carbonic anhydrase critical for growth and photosynthesis in the marine diatom Phaeodactylum tricornutum. Proc. Natl. Acad. Sci. U.S.A. 113, 9828–9833. doi:10.1073/pnas.1603112113
Matsuda, Y., Hara, T., and Colman, B. (2001). Regulation of the induction of bicarbonate uptake by dissolved CO2 in the marine diatom, Phaeodactylum tricornutum. Plant Cell Environ. 24, 611–620. doi:10.1046/j.1365-3040.2001.00702.x
Mukherjee, A., Lau, C. S., Walker, C. E., Rai, A. K., Prejean, C. I., Yates, G., et al. (2019). Thylakoid localized bestrophin-like proteins are essential for the CO2 concentrating mechanism of Chlamydomonas reinhardtii. Proc. Natl. Acad. Sci. U.S.A. 116, 16915–16920. doi:10.1073/pnas.1909706116
Nakajima, K., Tanaka, A., and Matsuda, Y. (2013). SLC4 family transporters in a marine diatom directly pump bicarbonate from seawater. Proc. Natl. Acad. Sci. U.S.A. 110, 1767–1772. doi:10.1073/pnas.1216234110
Nam, O., Musiał, S., Demulder, M., McKenzie, C., Dowle, A., Dowson, M., et al. (2024). A protein blueprint of the diatom CO2-fixing organelle. Cell 187, 5935–5950.e18. doi:10.1016/j.cell.2024.09.025
Nawaly, H., Matsui, H., Tsuji, Y., Iwayama, K., Ohashi, H., Nakajima, K., et al. (2023a). Multiple plasma membrane SLC4s contribute to external HCO3– acquisition during CO2 starvation in the marine diatom Phaeodactylum tricornutum. J. Exp. Bot. 74, 296–307. doi:10.1093/jxb/erac380
Nawaly, H., Tanaka, A., Toyoshima, Y., Tsuji, Y., and Matsuda, Y. (2023b). Localization and characterization θ carbonic anhydrases in Thalassiosira pseudonana. Photosynth. Res. 156, 217–229. doi:10.1007/s11120-023-01007-z
Nigishi, M., Shimakawa, G., Yamagishi, K., Amano, R., Ito, S., Tsuji, Y., et al. (2024). Low-CO2-inducible bestrophins outside the pyrenoid sustain high photosynthetic efficacy in diatoms. Plant Physiol. 195, 1432–1445. doi:10.1093/plphys/kiae137
Oh, Z. G., Ang, W. S. L., Poh, C. W., Lai, S.-K., Sze, S. K., Li, H.-Y., et al. (2023). A linker protein from a red-type pyrenoid phase separates with Rubisco via oligomerizing sticker motifs. Proc. Natl. Acad. Sci. U.S.A. 120, e2304833120. doi:10.1073/pnas.2304833120
Ohno, N., Inoue, T., Yamashiki, R., Nakajima, K., Kitahara, Y., Ishibashi, M., et al. (2011). CO2-cAMP-responsive cis-elements targeted by a transcription factor with CREB/ATF-like basic zipper domain in the marine diatom Phaeodactylum tricornutum Plant Physiol. Plant Physiol. 158, 499–513. doi:10.1104/pp.111.190249
Raven, J. A. (1997). CO2-concentrating mechanisms: a direct role for thylakoid lumen acidification? Plant Cell Environ. 20, 147–154. doi:10.1046/j.1365-3040.1997.d01-67.x
Samukawa, M., Shen, C., Hopkinson, B. M., and Matsuda, Y. (2014). Localization of putative carbonic anhydrases in the marine diatom, Thalassiosira pseudonana. Photosynth. Res. 121, 235–249. doi:10.1007/s11120-014-9967-x
Shimakawa, G., Demulder, M., Flori, S., Kawamoto, A., Tsuji, Y., Nawaly, H., et al. (2024a). Diatom pyrenoids are encased in a protein shell that enables efficient CO2 fixation. Cell 187, 5919–5934.e19. doi:10.1016/j.cell.2024.09.013
Shimakawa, G., Matsuda, Y., and Burlacot, A. (2024b). Crosstalk between photosynthesis and respiration in microbes. J. Biosci. 49, 45. doi:10.1007/s12038-023-00417-4
Shimakawa, G., Okuyama, A., Harada, H., Nakagaito, S., Toyoshima, Y., Nagata, K., et al. (2023a). Pyrenoid-core CO2-evolving machinery is essential for diatom photosynthesis in elevated CO2. Plant Physiol. 193, 2298–2305. doi:10.1093/plphys/kiad475
Shimakawa, G., Yashiro, E., and Matsuda, Y. (2023b). Mapping of subcellular local pH in the marine diatom Phaeodactylum tricornutum. Physiol. Plant. 175, e14086. doi:10.1111/ppl.14086
Tachibana, M., Allen, A. E., Kikutani, S., Endo, Y., Bowler, C., and Matsuda, Y. (2011). Localization of putative carbonic anhydrases in two marine diatoms, Phaeodactylum tricornutum and Thalassiosira pseudonana. Photosynth. Res. 109, 205–221. doi:10.1007/s11120-011-9634-4
Tsuji, Y., Kusi-Appiah, G., Kozai, N., Fukuda, Y., Yamano, T., and Fukuzawa, H. (2021). Characterization of a CO2-concentrating mechanism with low sodium dependency in the centric diatom Chaetoceros gracilis. Mar. Biotechnol. 23, 456–462. doi:10.1007/s10126-021-10037-4
Tsuji, Y., Mahardika, A., and Matsuda, Y. (2017a). Evolutionarily distinct strategies for the acquisition of inorganic carbon from seawater in marine diatoms. J. Exp. Bot. 68, 3949–3958. doi:10.1093/jxb/erx102
Tsuji, Y., Nakajima, K., and Matsuda, Y. (2017b). Molecular aspects of the biophysical CO2-concentrating mechanism and its regulation in marine diatoms. J. Exp. Bot. 68, 3763–3772. doi:10.1093/jxb/erx173
Witt, H. T. (1979). Energy conversion in the functional membrane of photosynthesis. Analysis by light pulse and electric pulse methods. The central role of the electric field. Biochim. Biophys. Acta Bioenerg. 505, 355–427. doi:10.1016/0304-4173(79)90008-9
Yamano, T., Tsujikawa, T., Hatano, K., Ozawa, S.-i., Takahashi, Y., and Fukuzawa, H. (2010). Light and low-CO2-dependent LCIB–LCIC complex localization in the chloroplast supports the carbon-concentrating mechanism in Chlamydomonas reinhardtii. Plant Cell Physiol. 51, 1453–1468. doi:10.1093/pcp/pcq105
Keywords: photosynthesis, pyrenoid, pyrenoid-penetrating thylakoid membranes, marine diatoms, CO2-concentrating mechanism
Citation: Shimakawa G and Matsuda Y (2025) Multiphase CO2-dependent photosynthesis in marine diatoms. Front. Photobiol. 3:1571863. doi: 10.3389/fphbi.2025.1571863
Received: 06 February 2025; Accepted: 18 March 2025;
Published: 02 April 2025.
Edited by:
Cristian Ilioaia, UMR9198 Institut de Biologie Intégrative de la Cellule (I2BC), FranceReviewed by:
Luke Colin Martin Mackinder, University of York, United KingdomCopyright © 2025 Shimakawa and Matsuda. This is an open-access article distributed under the terms of the Creative Commons Attribution License (CC BY). The use, distribution or reproduction in other forums is permitted, provided the original author(s) and the copyright owner(s) are credited and that the original publication in this journal is cited, in accordance with accepted academic practice. No use, distribution or reproduction is permitted which does not comply with these terms.
*Correspondence: Ginga Shimakawa, Z3NoaW1ha2F3YUBwYW5kYS5rb2JlLXUuYWMuanA=; Yusuke Matsuda, eXVzdWtlQGt3YW5zZWkuYWMuanA=
†ORCID: Ginga Shimakawa, orcid.org/0000-0002-8557-2096; Yusuke Matsuda, orcid.org/0000-0002-1892-4397
Disclaimer: All claims expressed in this article are solely those of the authors and do not necessarily represent those of their affiliated organizations, or those of the publisher, the editors and the reviewers. Any product that may be evaluated in this article or claim that may be made by its manufacturer is not guaranteed or endorsed by the publisher.
Research integrity at Frontiers
Learn more about the work of our research integrity team to safeguard the quality of each article we publish.