- 1Microbial Horticulture, Department of Biosystems and Technology, Swedish University of Agricultural Sciences, Uppsala, Sweden
- 2Horticultural Crop Physiology, Department of Biosystems and Technology, Swedish University of Agricultural Sciences, Uppsala, Sweden
- 3Department of Plant Breeding, Swedish University of Agricultural Sciences, Uppsala, Sweden
- 4Department of Plant Protection Biology, Swedish University of Agricultural Sciences, Uppsala, Sweden
Plants and crop stands are considered holobionts, colonized by both autotrophic and by non-phototrophic heterotrophic microbiota. The dilemma in the exisiting body of studies is that the focus is primarily directed towards environmental specificties relevant for phototrophic organisms (predominantly plants), but does not take into account non-phototrophs. By definition, non-phototrophic heterotrophic bacteria do not use light as an energy source. Light energy and wavelength are rather used as a signal that can provoke shifts in both their metabolism and microbial lifestyle. Reaction and recovery time can vary between organisms and is dependent on the organism’s physiological stage. The length of the lighting event affects the energy an organism is exposed to. We argue that to obtain a deeper and more distinct understanding of light exposure (irradiance, exposure length), quantity (light intensity), and quality (wavelength/spectral distribution, bandwidth at full-width half-maximum) related mechanisms on non-phototrophic bacteria in the phyllosphere, the light environment needs to be further strictly characterized. This includes information on the actual energy hitting planktonic or sessile non-phototrophic bacteria resident on and inside plants aboveground. Mapping the light environment in ecosystems aids in unraveling light-phyllosphere interactions and strengthens their transdisciplinary character. This issue is fundamental in order to revisit and repeat others’ experimental approaches and findings but also to be able to translate findings into further action.
1 Introduction
Based on premises rather than experimental evidence, the phyllosphere is often characterized using atmospheric rather than boundary layer conditions (mainly temperature and to some extent relative humidity) as a basis of its description (Alsanius et al., 2019). Light conditions are often incomplete in microbial studies, such as day length, crop stand, light spectrum, and light intensity, are often ignored despite the overruling influence of light on primary (photosynthesis) and secondary plant processes (biomass formation, crop development, secondary metabolisms) which could influence the plant microbiota.
In crop science, visible light, ranging from 380 nm (violet) to 750 nm (far-red light), is usually in focus. Given the plants’ ability to transform light into energy, light is usually expressed as photosynthetic active radiation (PAR; 400–700 nm) and photosynthetic photon flux density (PPFD; μmol m−2 s−1). PAR and PPFD units have been applied in studies of interactions between plants and UV- or infrared-light. Plants and crop stands are considered holobionts, colonized by both autotrophic and by non-phototrophic heterotrophic microbiota. Although the latter ones do not directly depend on light as an energy source, there is growing evidence that their metabolism uses light (light quality: light spectral distribution; wavelength, exposure dose that can affect the circadian rhythm) as signals (Losi and Gärtner, 2021; Kahl et al., 2022; Wollmuth and Angert, 2023).
The interdependence of light quality and aerial fungal plant pathogens is well known (see Alsanius et al., 2019, see Beattie et al., 2018 and references in both). Several studies indicate that spectral distribution induced shifts in bacterial lifestyles (planktonic, sessile), metabolic activity and environmental stress responses can occur when exposed to different wavelengths and intensities (Wu et al., 2013; Gharaie et al., 2017; Alsanius et al., 2019; Alsanius et al., 2021; Kahl et al., 2022; Hatfield et al., 2023). Moreover, the presence or absence of light can impact biofilm formation, as can light quality influence respiration of non-phototrophic bacteria (Kahl et al., 2022); redox stages in zones affected by different previous lighting stimuli could be endured upon changes in light conditions. Thus, irradiance based measures, such energy (W m−2) and exposure dose (W m−2 s−1; J m−2) rather than just photon density ought to be mentioned when describing the phyllosphere environment in relation to light exposure (see Box 1).
We postulate that
• To study light-phyllosphere interactions, non-phototrophic organisms’ perception of light (radiation) must be considered
• PAR based descriptions of the light environment, only focuses on plants’, and associated phototrophic organisms’ ability to utilize light.
Thus energy based information must be used to study the fate of non-phototrophic organisms in the phyllosphere.
2 Plant-light interactions
Plants use light as a primary energy source via photosynthesis, but light also informs the plant about the time of day, time of year, and about its surroundings (e.g., if the plant is shaded by other plants) (Wassink and Stolwijk, 1956). The photosynthetically active spectrum is normally generalized to 400–700 nm in wavelength, the range of 380–710 nm has also been suggested (McCree, 1972). However, wavelengths shorter (Ultraviolet light, UV) and longer (Far red, FR, Infra-red, IR) than the photosynthetically active wavelengths will further affect the plant in several ways. For measuring photosynthetic light, special PAR-sensors (quantum sensors) have been developed, giving values in the unit μmol m−2 s−1 (Ryer, 1997). Information about light intensity in combination with daily photoperiod can be integrated into a daily light integral (DLI), expressed in mol m−2 day−1, which is a commonly used unit to quantify photosynthetic light. Spectral distribution within the photosynthetic light, and the amounts of UV-, FR- and IR-light can be measured using a spectroradiometer (Ryer, 1997), informing of the spectral irradiance for each wavelength in the units μmol m−2 s−1 or mW m−2.
However, light measurements in growing systems are normally performed above the canopy. As soon as the light enters the canopy, the intensity and spectral distribution is altered due to absorption and reflection; this is a fact that is often overlooked when discussing the full lighting effect on and within plants (Figure 1).
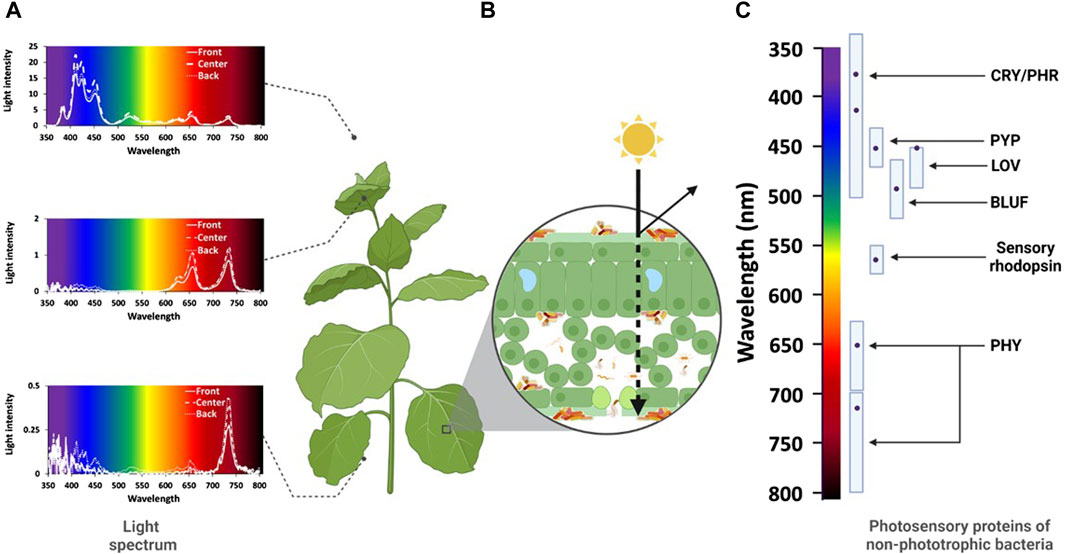
Figure 1. The plant holobiont as affected by light. (A) Light quantity (light intensity, irradiance) and light quality (spectral distribution) of white LEDs in the canopy of a Poinsettia (Euphorbia pulcherima) crop under greenhouse conditions. Light quantity and quality parameters were measured above the canopy as well as beneath the apical and basal leaves of Poinsettia plants placed directly under the LED (centre), or at 20 cm distance from the central plant (front, back). Light quantity decreases from the top to the bottom (note deviating y-axes). Differences also occurred with respect to the position of the plant in the crop stand. With respect to light quality, the light spectrum is distorted from high relative abundance of blue light above the canopy to higher relative abundance of red and far-red or just far-red bands beneath the apical and basal leaves, respectively. (B) Light quantity and quality hitting a leaf surface, light may be absorbed, refracted, or reflected. The leaf surface structure, but also the morphology and thickness of different leaf layers, the nature of pigments and their distribution play a key role for the transmittance of light through the leaf. (C) Spectral bands absorbed by different photosensory proteins (cryptochrome/photolyase, CRY/PHR; photosensory yellow protein, PYP; light-oxygen-voltage, LOV; sensor of blue light utilizing flavin adenine nucleotide, BLUF; Sensory rhodopsin; bacteriophotochrome, PHY) of non-phototrophic bacteria. The dots indicate the peak absorption within each wavelength spectrum for each photosensory protein. Recent results indicate that PHY also may act within the blue spectrum (Hatfield et al., 2023). (Illustration: B. Alsanius and M. Hellström, supported by Biorender.com).
3 Photoreception of non-phototrophic bacteria
Photosynthetic prokaryotes and some non-phototrophic bacteria are equipped with photosensory proteins. For non-phototrophic bacteria, in total, six different photosensory proteins have been identified with an array of absorption spectra within the visible and non-visible light spectrum (Figure 1). These include phytochromes (PHY) (Auldridge and Forest, 2011) absorbing red and far-red light, photoactive yellow proteins (PYP) (Kumauchi et al., 2008; Meyer et al., 2012), rhodopsins which are retinal binding receptors and provide light dependent ion transport (Ernst et al., 2014), blue light absorbing proteins cryptochrome/photolyase (Cry/PHR), blue light using flavin adenine dinucleotide (BLUF-FAD) and light oxygen voltage (LOV) domain (Gomelsky and Hoff, 2011; Wu et al., 2013).
The typical phytochrome architecture consists of three conserved domains: PAS (Per-ARNT.Sim), -GAF (cGMP phosphodiesterase/adenylate) and PHY (Phytochrome specific). In bacteria, biliverdin is used as a chromophore (Bhoo et al., 2001). The structure of PYP has a typical PAS domain and is often referred to as the prototype of proteins in this domain (Imamoto and Kataoka, 2007). The BLUF domain proteins can both be standalone BLUF domains or be coupled to phosphodiesterase (EAL) domains. Almost 70% of BLUF domains are not connected to EAL. The second most common structure of the BLUF domain is a BLUF-EAL combination (Kanazawa et al., 2010). The small photosensory protein LOV belongs to the PAS domain and is linked to histidine protein kinase (HisKa), di-guanylate cyclase (GGDEF) and EAL domains (Van der Horst et al., 2007).
Photosensing in bacteria can cause a cascade of reactions inside the cell. Signalling molecules and regulatory proteins can result in a change of gene regulation that can alter the behaviour and lifestyle of the bacteria involved. LOV, PYP and BLUF have under different light conditions shown to control the transition between planktonic single cell lifestyle into a sessile multicellular lifestyle. The key player in this is the second messenger c-di-GMP with the help by GGDEF and EAL, which is responsible in the synthesis and the hydrolysis of c-di-GMP (Hengge, 2009). If the concentration of c-di-GMP increases in the cell, the motility of the flagella is inhibited and thus the synthesis and excretion of the biofilm component is stimulated. There is also evidence that both LOV and PHY regulates swarming motility in the pathogen Pseudomonas syringae and that there is cross talk between these two proteins (Wu et al., 2013). In Escherichia coli, the BLUF-EAL protein YcgF does not hydrolyse c-di-GMP but instead binds to the repressor YcgE during blue light exposure. This leads to activation of both biofilm matrix production and of acid resistance genes and downregulation of adhesive curli fimbriae. Moreover, the expression of YcgF and YcgE was activated strongly at low temperatures (Tschowri et al., 2009).
Light can also change the utilization patterns of nutrients in bacteria and thus affect several metabolic pathways when bacteria are exposed to different light spectra (Müller et al., 2017; Alsanius et al., 2021; Karlsson et al., 2023). Recent results on P. syringae pv. syringae demonstrate that non-phototrophic bacteria employ light information to sense and prepare for environmental changes, such as water stress (Hatfield et al., 2023).
4 Discussion
In non-phototrophic bacteria, photoreceptors are globally regulating metabolic functional activities (Hatfield et al., 2023). Photoreceptors are thus high on the regulatory pyramid. Reaction and recovery time to light exposure can vary between seconds to minutes and even hours (Ernst et al., 2014; Liu et al., 2018). They deviate between different photoreceptor proteins and wavelengths, as well as on the intensity of emitted light (Figure 2). Furthermore, the light exposure and interval have been shown to affect the circadian rhythm in various bacteria (Kahl et al., 2022; Wollmuth and Angert, 2023), which have led to permanent metabolic changes. By not defining all possible driving factors, such as light intensity, wavelength and exposure time, a dilemma occurs with respect to understanding how they can affect microbial mechanisms, in particular of non-phototrophic bacteria within the phyllosphere. For example, when comparing the two wavelengths of 660 nm and 400 nm even though they have the same intensity (500 μmol m−2 s−1) the total exposure dose is much higher in the latter of the two (see example shown in Supplementary Material S1). Some studies either state the wavelength used in treatment, e.g., 420 nm, or just the colour perceived by the human eye, e.g., blue, and the intensity at which it was used for (PPFD) (Huché-Thélier et al., 2016). This not only affects the reproducibility of experiments and results, comparability, generalization of data but also their translation to any applied setting. Thus, in the case of studying light-microbe and phyllosphere interactions a defined exposure dose is necessary.
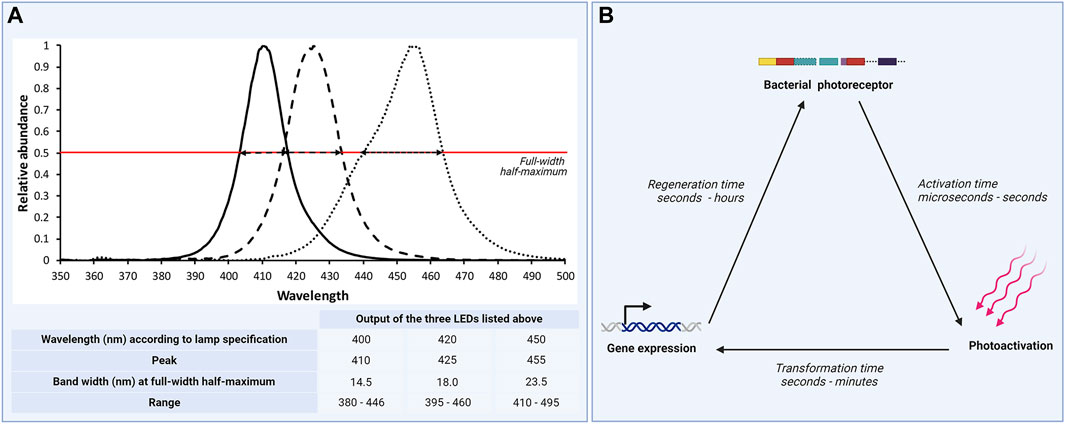
Figure 2. Exposure dose information - a fundamental detail in studies on light response of non-phototrophic bacteria. (A) Outputs of three LED devices within the violet-blue spectrum. Wavelengths specified for the LEDs were 400 nm, 420 nm, and 450 nm, respectively. Regauging the three LEDs displayed that peak relative abundance deviated from the specifications and that spectral width characteristics varied considerably between the three LEDs as expressed by bandwidth at full-width half-maximum (indicated by the red line) and spectrum range output. To calculate the exposure dose, these characteristics need to be described. (B) Response to electromagnetic radiation differs between different photoreceptors, bacterial organisms and strains, but also between different phases within. Activation time might range between microseconds to seconds, transition from photoactivation to gene expression (transition time) between seconds to minutes and regeneration time between seconds to hours. (Illustration: B. Alsanius and M. Hellström, supported by biorender.com).
Information about the light environment varies substantially in several published studies. A Scopus literature survey, spanning over a ten-year-period (2013–2023) and based on the search terms (“bacteria” OR “biocontrol*") AND (“light” OR “irradiation” OR “light quality”) AND (“phyllosphere” OR “leaf”) produced 21 eligible references and 29 individually assessed experiments (Alsanius et al., 2024). Most of the studies were conducted in vitro. A majority, but not all studies, stated light quantity. Either light intensity (37.9%) or irradiance (48.3%) was used as a measure; no information was given on the intensity distribution (as displayed in Hoenes et al., 2015). Light quantity statements considered outputs from the light source but did not take material properties of vials or containers light transmittance into account, used for housing the organisms during the experiment (as displayed in Gharaie et al., 2017). The studies included various light sources delivering either mono- or polychromatic (white) light. Light quality parameters were poorly displayed and information on the spectral distribution was mostly lacking. In studies including monochromatic light, the peak value, but not the bandwidth, was often mentioned. The spectral composition in polychromatic light varies and information on the spectral range was not always sufficient. Most studies indicated exposure time. However, as light quantity and quality parameters were mostly poorly described and thus the recalculation of exposure dose is rather difficult.
As any environmental cue, light quality, exposure and dose can have large effects on the plant as a holobiont. To direct and manage photodependent responses in non-phototrophic plant colonizing bacteria, deeper insights are required into the pace of action caused by light within the crop stand. Reception, scattering and absorption of light differs greatly within the canopy as demonstrated by Schipper et al. (2023), but also within the plant organs, e.g., leaf (Vogelmann and Björn, 1986; Vogelmann and Gorton, 2014; Müller et al., 2016) (Figure 1).
Leaves tend to absorb approximately 80% of the light they receive. Within this percentage, some of it is reflected due to light scattering within the intercellular air pockets inside a leaf. Further, several leaf organs can alter the spectral quality of the received light such as chlorophylls and carotenoids due to absorption. This alteration causes steep internal gradients within the leaf tissue and thus at different depths there are diverse light environments for chloroplasts. One other factor that can cause a light gradient is the leaf angle itself, this as light direction and quality is affected greatly by it (Vogelmann and Gorton, 2014) (Figure 1).
A majority of studies on light-non-phototrophic bacteria-phyllosphere interactions have only been conducted under in vitro conditions. Substantially fewer studies involve plants and crop stands. However, the experimental conditions (light intensity, wavelength, photoperiod, exposure dose, humidity, temperature) are not always stated (Alsanius et al., 2024). To apply and follow up photo-dependent bacterial mechanisms in crop stands, distinct characterization of plant canopy conditions are necessary. This is needed to better understand the plant holobiont and to foresee light related events in both natural and cultured crop stands. Thus, it is vital to re-evaluate findings presented in the literature from the perspective of light exposure dose and related parameters to get a deeper and more distinct understanding of the effect of light in light-microbe interactions, especially in the phyllosphere. This would lead to a clearer characterization of the ecosystems studied and allow for a richer understanding of why light quality and quantity can have the effect observed within the microbiota present or introduced to on a plant.
Data availability statement
The original contributions presented in the study are included in the article/Supplementary Material, further inquiries can be directed to the corresponding author.
Author contributions
BA: Conceptualization, Funding acquisition, Investigation, Project administration, Resources, Supervision, Visualization, Writing–original draft, Writing–review and editing. MH: Investigation, Visualization, Writing–original draft, Writing–review and editing. K-JB: Investigation, Writing–original draft, Writing–review and editing. RV: Supervision, Writing–original draft, Writing–review and editing. PB: Supervision, Writing–original draft, Writing–review and editing. MK: Investigation, Supervision, Writing–original draft, Writing–review and editing.
Funding
The author(s) declare that financial support was received for the research, authorship, and/or publication of this article. This perspective paper is funded by a joined grant (R-18-25-006) from the Swedish research council Formas and the Swedish Farmers’ Foundation, both Stockholm, Sweden.
Acknowledgments
Our funding agencies, the Swedish research council Formas and the Swedish Farmers’ Foundation are gratefully acknowledged.
Conflict of interest
The authors declare that the research was conducted in the absence of any commercial or financial relationships that could be construed as a potential conflict of interest.
Publisher’s note
All claims expressed in this article are solely those of the authors and do not necessarily represent those of their affiliated organizations, or those of the publisher, the editors and the reviewers. Any product that may be evaluated in this article, or claim that may be made by its manufacturer, is not guaranteed or endorsed by the publisher.
Supplementary material
The Supplementary Material for this article can be found online at: https://www.frontiersin.org/articles/10.3389/fphbi.2024.1432066/full#supplementary-material
References
Alsanius, B. W., Karlsson, M. E., Hellström, M., Bergstrand, K.-J., and Rosberg, A. K. (2024). Integrated greenhouse production in new light: prospects on improved LED-assisted biocontrol of foliar diseases. Acta Hortic., 293–296. accepted. doi:10.17660/actahortic.2020.1296.38
Alsanius, B. W., Karlsson, M. E., Rosberg, A. K., Dorais, M., Naznin, T., Khalil, S., et al. (2019). Light and microbial lifestyle: the impact of light quality on plant-microbe interactions in horticultural production systems - a review. Horticulturae 5, 41. doi:10.3390/horticulturae5020041
Alsanius, B. W., Vaas, L. A. I., Gharaie, S., Karlsson, M. E., Rosberg, A. K., Wohanka, W., et al. (2021). Dining in blue light impairs the appetite of some leaf epiphytes. Front. Microbiol. 12, 725021. doi:10.3389/fmicb.2021.725021
Auldridge, M. E., and Forest, K. T. (2011). Bacterial phytochromes: more than meets the light. Crit. Rev. Biochem. Mol. Biol. 46, 67–88. doi:10.3109/10409238.2010.546389
Beattie, G. A., Hatfield, B. M., Dong, H., and Mcgrane, R. S. (2018). Seeing the light: the roles of red- and blue-light sensing in plant microbes. Annu. Rev. Phytopathology 56, 41–66. doi:10.1146/annurev-phyto-080417-045931
Bhoo, S. H., Davis, S. J., Karniol, B., and Vierstra, R. D. (2001). Bacteriophytochromes are photochromic histidine kinases using a biliverdin chromophore. Nat. Rev. Microbiol. 414, 776–779. doi:10.1038/414776a
Ernst, O. P., Lodowski, D. T., Elstner, M., Hegemann, P., Brown, L. S., and Kandori, H. (2014). Microbial and animal rhodopsins: structures, functions, and molecular mechanisms. Chem. Rev. 114, 126–163. doi:10.1021/cr4003769
Gharaie, S., Vaas, L. A. I., Rosberg, A. K., Windstam, S., Karlsson, M. E., Bergstrand, K.-J., et al. (2017). Light spectrum modifies the utilization pattern of energy sources in Pseudomonas sp. DR 5-09. PLoS ONE 12, e0189862. doi:10.1371/journal.pone.0189862
Gomelsky, M., and Hoff, W. D. (2011). Light helps bacteria make important lifestyle decisions. Trends Microbiol. 19, 441–448. doi:10.1016/j.tim.2011.05.002
Goncalves Dos Reis, M., and Ribeiro, A. (2020). Conversion factors and general equations applied in agricultural and forest meteorology. Agrometeoros 27, 227–258. doi:10.31062/agrom.v27i2.26527
Hatfield, B. M., Lasarre, B., Liu, M., Dong, H., Nettleton, D., and Beattie, G. A. (2023). Light cues induce protective anticipation of environmental water loss in terrestrial bacteria. Proc. Natl. Acad. Sci. 120, e2309632120. doi:10.1073/pnas.2309632120
Hengge, R. (2009). Principles of c-di-GMP signalling in bacteria. Nat. Rev. Microbiol. 7, 263–273. doi:10.1038/nrmicro2109
Hoenes, K., Stangl, F., Stift, M., and Hessling, M. (2015). “Visible optical radiation generates bactericidal effect applicable for inactivation of health care associated germs demonstrated by inactivation of E. coli and B. subtilis using 405 nm and 460 nm light emitting diodes,” in Novel biophotonics techniques and applications III. Editors A. AMELINK, and I. A. VITKIN 95400T.
Huché-Thélier, L., Crespel, L., Gourrierec, J. L., Morel, P., Sakr, S., and Leduc, N. (2016). Light signaling and plant responses to blue and UV radiations—perspectives for applications in horticulture. Environ. Exp. Bot. 121, 22–38. doi:10.1016/j.envexpbot.2015.06.009
Imamoto, Y., and Kataoka, M. (2007). Structure and photoreaction of photoactive yellow protein, a structural prototype of the PAS domain superfamily. Photochem. Photobiol. 83, 40–49. doi:10.1562/2006-02-28-IR-827
Kahl, L. J., Eckartt, K. N., Morales, D. K., Price-Whelan, A., and Dietrich, L. E. P. (2022). Light/dark and temperature cycling modulate metabolic electron flow in Pseudomonas aeruginosa biofilms. mBio 13, e0140722. doi:10.1128/mbio.01407-22
Kanazawa, T., Ren, S., Maekawa, M., Hasegawa, K., Arisaka, F., Hyodo, M., et al. (2010). Biochemical and physiological characterization of a BLUF protein-EAL protein complex involved in blue light-dependent degradation of cyclic diguanylate in the purple bacterium Rhodopseudomonas palustris. Biochemistry 49, 10647–10655. doi:10.1021/bi101448t
Karlsson, M. E., Hellström, M., Flöhr, A., Bergstrand, K.-J., and Alsanius, B. W. (2023). The power of light: impact on the performance of biocontrol agents under minimal nutrient conditions. Front. Microbiol. 14, 1087639. doi:10.3389/fmicb.2023.1087639
Kumauchi, M., Hara, M. T., Stalcup, P., Xie, A., and Hoff, W. D. (2008). Identification of six new photoactive yellow proteins--diversity and structure-function relationships in a bacterial blue light photoreceptor. Biochem. Photobiol. 84, 956–969. doi:10.1111/j.1751-1097.2008.00335.x
Liu, Z., Zhang, J., Jin, J., Geng, Z., Qi, Q., and Liang, Q. (2018). Programming bacteria with light—sensors and applications in synthetic biology. Front. Microbiol. 9, 2692. doi:10.3389/fmicb.2018.02692
Losi, A., and Gärtner, W. (2021). A light life together: photosensing in the plant microbiota. Photochem. Photobiological Sci. 20, 451–473. doi:10.1007/s43630-021-00029-7
Mccree, K. J. (1972). Test of current definitions of photosynthetically active radiation against leaf photosynthesis data. Agric. Meteorol. 10, 443–453. doi:10.1016/0002-1571(72)90045-3
Meyer, T. E., Kyndt, J. A., Memmi, S., Moser, T., Colón-Acevedo, B., Devreese, B., et al. (2012). The growing family of photoactive yellow proteins and their presumed functional roles. Photochem. Photobiological Sci. 11, 1495–1514. doi:10.1039/c2pp25090j
Müller, D. B., Vogel, C., Bai, Y., and Vorholt, J. A. (2016). The plant microbiota: systems-level insights and perspectives. Annu. Rev. Genet. 50, 211–234. doi:10.1146/annurev-genet-120215-034952
Müller, G. L., Tuttobene, M., Altilio, M., Martínez Amezaga, M., Nguyen, M., Cribb, P., et al. (2017). Light modulates metabolic pathways and other novel physiological traits in the human pathogen Acinetobacter baumannii. J. Bacteriol. 199, e00011-17–e00017. doi:10.1128/jb.00011-17
Ryer, A. D. (1997). The light measurement handbook. Peabody, MA USA: International Light Technologies.
Tschowri, N., Busse, S., and Hengge, R. (2009). The BLUF-EAL protein YcgF acts as a direct anti-repressor in a blue-light response of Escherichia coli. Genes Dev. 23, 522–534. doi:10.1101/gad.499409
Van Der Horst, M. A., Key, J., and Hellingwerf, K. J. (2007). Photosensing in chemotrophic, non-phototrophic bacteria: let there be light sensing too. Trends Microbiol. 15, 554–562. doi:10.1016/j.tim.2007.09.009
Vogelmann, T. C., and Björn, L. O. (1986). Plants as light traps. Physiol. Plant. 68, 704–708. doi:10.1111/j.1399-3054.1986.tb03421.x
Vogelmann, T. C., and Gorton, H. L. (2014). “Leaf: light capture in the photosynthetic organ,” in The structural basis of biological energy generation. Editor M. F. HOHMANN-MARRIOTT (Amsterdam: Springer).
Wassink, E., and Stolwijk, J. (1956). Effects of light quality on plant growth. Annu. Rev. Plant Physiology 7, 373–400. doi:10.1146/annurev.pp.07.060156.002105
Wollmuth, E. M., and Angert, E. R. (2023). Microbial circadian clocks: host-microbe interplay in diel cycles. BMC Microbiol. 123, 124. doi:10.1186/s12866-023-02839-4
Keywords: energy, exposure dose, irradiance, light intensity, non-phototrophic phyllosphere bacteria, phyllosphere environment
Citation: Alsanius BW, Hellström M, Bergstrand K-J, Vetukuri R, Becher P and Karlsson ME (2024) The power of light from a non-phototrophic perspective: a phyllosphere dilemma. Front. Photobiol. 2:1432066. doi: 10.3389/fphbi.2024.1432066
Received: 13 May 2024; Accepted: 28 May 2024;
Published: 13 June 2024.
Edited by:
Andrei Herdean, University of Technology Sydney, AustraliaReviewed by:
Iskander M. Ibrahim, Towson University, United StatesCopyright © 2024 Alsanius, Hellström, Bergstrand, Vetukuri, Becher and Karlsson. This is an open-access article distributed under the terms of the Creative Commons Attribution License (CC BY). The use, distribution or reproduction in other forums is permitted, provided the original author(s) and the copyright owner(s) are credited and that the original publication in this journal is cited, in accordance with accepted academic practice. No use, distribution or reproduction is permitted which does not comply with these terms.
*Correspondence: Beatrix W. Alsanius, YmVhdHJpeC5hbHNhbml1c0BzbHUuc2U=