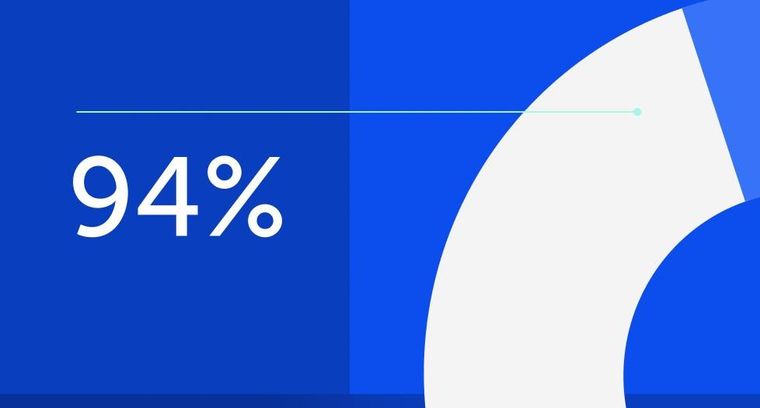
94% of researchers rate our articles as excellent or good
Learn more about the work of our research integrity team to safeguard the quality of each article we publish.
Find out more
REVIEW article
Front. Pharmacol., 01 April 2025
Sec. Inflammation Pharmacology
Volume 16 - 2025 | https://doi.org/10.3389/fphar.2025.1579507
Intervertebral disc degeneration (IVDD) is a prevalent condition contributing to various spinal disorders, posing a significant global health burden. Mitophagy plays a crucial role in maintaining mitochondrial quantity and quality and is closely associated with the onset and progression of IVDD. Well-documented region-specific mitophagy mechanisms in IVDD are guiding the development of therapeutic strategies. In the nucleus pulposus (NP), impaired mitochondria lead to apoptosis, oxidative stress, senescence, extracellular matrix degradation and synthesis, excessive autophagy, inflammation, mitochondrial instability, and pyroptosis, with key regulatory targets including AMPK, PGC-1α, SIRT1, SIRT3, Progerin, p65, Mfn2, FOXO3, NDUFA4L2, SLC39A7, ITGα5/β1, Nrf2, and NLRP3 inflammasome. In the annulus fibrosus (AF), mitochondrial damage induces apoptosis and oxidative stress mediated by PGC-1α, while in the cartilage endplate (CEP), mitochondrial dysfunction similarly triggers apoptosis and oxidative stress. These mechanistic insights highlight therapeutic strategies such as activating Parkin-dependent and Ub-independent mitophagy pathways for NP, enhancing Parkin-dependent mitophagy for AF, and targeting Parkin-mediated mitophagy for CEP. These strategies include the use of natural ingredients, hormonal modulation, gene editing technologies, targeted compounds, and manipulation of related proteins. This review summarizes the mechanisms of mitophagy in different regions of the intervertebral disc and highlights therapeutic approaches using mitophagy modulators to ameliorate IVDD. It discusses the complex mechanisms of mitophagy and underscores its potential as a therapeutic target. The objective is to provide valuable insights and a scientific basis for the development of mitochondrial-targeted drugs for anti-IVDD.
The intervertebral disc, a fibrocartilaginous tissue between adjacent vertebral bodies, consists of the nucleus pulposus (NP), annulus fibrosus (AF) and cartilage endplate (CEP) (Vergroesen et al., 2015). It is a unique structure that provides segmental mobility and is simultaneously responsible for the mechanical stability of the spinal column (Wise et al., 2020). Specifically, the NP resides centrally within the intervertebral disc, fulfilling the role of enduring mechanical impacts (Kepler et al., 2013). Surrounding it intactly, the AF is associated with the regulation of the occurrence of intervertebral disc herniation (Gorth et al., 2020), while the CEP interfaces with the vertebral bones and plays a crucial part in regulating the transport of nutrients (Habib et al., 2023). Residing in a naturally harsh microenvironment of hypoxia, acidic pH, low nutrition and high mechanical loading, disc have limited capacity for self-repair and are vulnerable to damage (Huang et al., 2013).
Abnormal mechanical stresses, nutritional deficiencies, and the aging process are recognized as inductive factors for regulated cell death (RCD) in intervertebral disc cells (Vergroesen et al., 2015; Yang et al., 2022; Yang et al., 2019; Kang et al., 2020a). This cellular demise leads to the disruption of the normal architectural and physiological functions of the disc, ultimately progressing to intervertebral disc degeneration (IVDD) (Kepler et al., 2013; Walter et al., 2011). IVDD further serves as a primary contributor to a multitude of spinal disorders that pose a significant global health burden (Liebsch and Wilke, 2022; Binch et al., 2021). The escalating incidence of IVDD is poised to exacerbate the global prevalence of pain, disability, and the associated economic strain on healthcare systems (Collaborators, 2021; GBD, 2019 Diseases and Injuries Collaborators, 2020; GBD 2017 Disease and Injury Incidence and Prevalence Collaborators, 2018).
Mitochondria serve as the “powerhouse” of cells, generating energy in the form of ATP and participating in various vital cellular processes (Dc, 2013). However, mitochondria are prone to damage, leading to mitochondrial dysfunction and imbalance of cell homeostasis, which are closely associated with the occurrence of various diseases (Doblado et al., 2021). Thus, maintaining mitochondrial homeostasis is of vital importance. Mitophagy selectively eliminates damaged mitochondria and maintains their quality stability. On one hand, mitophagy is capable of selectively identifying and removing damaged mitochondria to prevent them from causing further harm to cells. On the other hand, through mitophagy, cells can adjust the quantity and quality of mitochondria to adapt to different metabolic requirements and microenvironmental changes (Pickles et al., 2018). This contributes to ensuring that cells function optimally in different physiological and pathological conditions.
Mitochondria play a crucial regulatory role in skeletal muscle physiology, demonstrating stimulus-responsive alterations in quantity, configuration, and performance under external stress conditions (Wu et al., 2024). The metabolic functions of mitochondria in hypoxic intervertebral disc environments have been largely overlooked (Madhu et al., 2020). It is only in recent years that steady advancements have been made in understanding the association between mitophagy and IVDD, revealing a close correlation between maintaining a healthy mitochondrial pool and preventing IVDD (Lin et al., 2023). Emerging research findings have facilitated the development of novel diagnostic protocols and more targeted interventions (Vlaeyen et al., 2018). Due to the distinct structures, physiological functions, and microenvironments of the NP, AF, and CEP, the pathological processes and repair mechanisms following injuries to these regions exhibit region-specific characteristics (Kepler et al., 2013; Xu et al., 2024). This heterogeneity profoundly impacts therapeutic targeting, as each subregion faces unique mitochondrial challenges, necessitating tailored strategies. In this review, we will summarize the mechanisms of mitophagy in distinct regions of the intervertebral disc, and discuss therapeutic strategies employing mitophagy modulators to delay IVDD. The goal is to provide significant insights that are broadly pertinent to enhancing human health and quality of life for patients suffering from related conditions.
A literature search was conducted in the PubMed from inception to March 2025. The keywords “nucleus pulposus” (6,796), “annulus fibrosus” (2,366), “cartilage endplate” (1,671), “intervertebral disc” (43,493), and “intervertebral disc degeneration” (13,699) were independently searched and then combined with the terms “mitophagy” (Liebsch and Wilke, 2022; Li et al., 2019), “mitochondria” (268,773), “mitochondrial homeostasis” (27,901), and “mitochondrial dysfunction” (103,290). Specifically, the combined searches retrieved 225 results. The reference lists of relevant studies were additionally screened to identify potentially eligible articles. The potentially eligible studies were then screened by three independent authors (C.F., Z.H., and M.Z.). The screenings were cross-checked, and any discrepancies were resolved through discussion with a senior reviewer (X.F.). After this process, 23 articles were ultimately included.
Studies were selected according to the following criteria.
Inclusion criteria: 1) Original research articles investigating NP, AF, or CEP in vitro or in vivo models; 2) Studies that explicitly assessed mitophagy phenotypes.
Exclusion criteria: 1) Duplicate publications or studies with overlapping datasets; 2) Articles lacking direct experimental evidence on NP, AF, or CEP.
During the past few decades, the research on mitophagy has demonstrated consistent progress, and its exploration in IVDD has exhibited a notable development trend over the recent years (Figure 1).
Figure 1. The development timeline of mitophagy in IVDD. PTEN, phosphatase and tensin homologue; PINK1, PTEN-induced putative kinase 1; BNIP3L, BCL2-interacting protein 3-like; IVDD, intervertebral disc degeneration; NP, nucleus pulposus; AF, annulus fibrosus; CEP, cartilage endplate.
In the 1960s, based on the findings of electron microscope studies, sufficient evidence existed to demonstrate the degradation of mitochondria and other intracellular structures in lysosomes within mammalian cells (Duve and Wattiaux, 1966). The term “mitophagy” was initially proposed to delineate the process of selective autophagy of mitochondria in 2005, highlighting its role as a targeted defense mechanism (Lemasters, 2005). As the research advanced, the synergistic regulation of mitophagy by PTEN induced putative kinase 1 (PINK1) and Parkin was first elucidated in the experiment, and studies suggest that PINK1 may be upstream of Parkin in the regulatory pathway (Clark et al., 2006). Additionally, the BCL2-interacting protein 3-like (BNIP3L) receptor, also known as Nip3-like protein X (NIX) receptor, has also been proven to play a crucial role in the selective elimination of mitochondria (Schweers et al., 2007). By the 2010s, research into mitophagy mechanisms had advanced significantly. Researchers have not only revealed a strong link between mitochondrial fission, fusion mechanisms, and mitophagy (Ding et al., 2010), but also, in 2014, identified that PINK1 possesses additional phosphorylation sites, which are capable of compensating for the function of Parkin in mitophagy (Dave et al., 2014; Lai et al., 2015; Villa et al., 2017). Over the following years, multiple PINK1-mediated mitophagy processes that are independent of Parkin have been further validated and studied. With advancements in gene editing and high-throughput sequencing technologies, novel mitophagy-related genes have been identified (Xie et al., 2019). Particularly, CRISPR-Cas9-mediated mitochondrial genome editing has enabled successful mtDNA modification while systematically identifying key regulators of mitophagy (Bi et al., 2022). Recent research has demonstrated the existence of machinery capable of delivering mitochondrial constituents and membranes to lysosomes in the absence of autophagy. For instance, inner mitochondrial membrane (IMM)-mediated mitophagy and mitochondrial extracellular vesicles (mitoEVs) are recognized as mechanisms for delivering mitochondrial components to lysosomes, thereby compensating for the deficiencies in canonical mitophagy (Konig et al., 2021; Saunders et al., 2024; Iorio et al., 2024).
In the research on mitophagy and IVDD, a study in 2017 pointed out that mitochondrial dysfunction is associated with the apoptosis of NP cells, suggesting that improving mitochondrial dysfunction could be a new way for effectively protecting NP cells (Xu et al., 2017). Subsequently, scientists discovered that Parkin is involved in the pathogenesis of IVDD and may serve as a potential therapeutic target for IVDD (Zhang et al., 2018). The crucial role of PINK1 in eliminating damaged mitochondria and alleviating the senescence of NP cells through the mitophagy pathway was also revealed (Y et al., 2018). In 2019, studies focused on the role of mitochondrial function in the pathogenesis of AF-related IVDD (Wu et al., 2021; Xu W-N. et al., 2019). In 2020, the importance of Parkin-mediated mitophagy in the survival of CEP cells under pathological conditions was unveiled (Kang et al., 2020b). In 2023, the role of the NLRX1-SLC39A7 complex in orchestrating mitochondrial dynamics and mitophagy to rejuvenate intervertebral disc through modulation of mitochondrial Zn2+ trafficking was unveiled (Song et al., 2024). These findings have provided new insights into understanding the pathological mechanisms and potential therapeutic approaches for IVDD. Concurrently, certain medications have exhibited potential in addressing IVDD by regulating mitophagy levels, bringing new therapeutic options and directions insights for future research and treatment (Kang et al., 2020a; Lin et al., 2020).
This primarily procedure of mitophagy consists of several sequential events: Firstly, damaged mitochondria depolarize and lose membrane potential. Secondly, mitochondria are wrapped by autophagosomes to form mitochondrial autophagosomes. Thirdly, these mitochondrial autophagosomes fuse with lysosomes. Lastly, the contents of the mitochondria are degraded by lysosomes (Xu et al., 2020).
In diverse cellular environments, various stimuli can induce mitophagy through multiple signaling cascades (Palikaras et al., 2017). Mitophagy operates through distinct yet interrelated mechanisms. These mechanisms can generally be categorized into ubiquitin (Ub)-dependent and Ub-independent pathways (Khaminets et al., 2016). The Ub-dependent pathways are further divided into the Parkin-dependent and Parkin-independent pathways (Birgisdottir et al., 2013; Chen G. et al., 2020) (Figure 2).
Figure 2. The overview of the mitophagy mechanisms. Note: Mitophagy can be categorized into Ub-dependent pathways (highlighted in yellow) and Ub-independent pathways (highlighted in purple). Atg8yl-Mach, Atg8ylation machinery; OPTN, optineurin; NDP52, nuclear dot protein 52; LC3, microtubule-associated protein1 light chain 3.
The Ub-dependent pathways rely on extensive ubiquitination of damaged mitochondrial surface proteins to promote mitophagy. In the field of Ub-dependent mitophagy, the Parkin-dependent pathway, mediated by the kinase PINK1 and the E3 ubiquitin ligase Parkin, is the most extensively studied mechanism (Clark et al., 2006; Ashrafi and Schwarz, 2013). PINK1, a highly conserved mitochondrial protein encoded by the PARK6 gene, is involved in regulating various cellular physiological processes, particularly crucial for the mitochondrial function (Wang N. et al., 2020). In healthy mitochondria, PINK1 is continuously imported into the IMM and degraded, maintaining low expression levels (Narendra et al., 2010; Jin et al., 2010; Yamano and Youle, 2013). However, when mitochondrial membrane potential (MMP) is compromised, leading to mitochondrial dysfunction, the import channel for PINK1 into the IMM is blocked, causing its accumulation at the translocase complex on the outer mitochondrial membrane (OMM) of the damaged mitochondria [45, 46]. At this location, PINK1 undergoes dimerization, triggering autophosphorylation and activation (Gan et al., 2022; Rasool et al., 2022). The activated PINK1 subsequently phosphorylates serine 65 of Ub and the Ub-like domain of Parkin, resulting in the further categorization of Ub-dependent mitophagy into Parkin-dependent and Parkin-independent pathways (Uoselis et al., 2023). In the Parkin-dependent pathway, Parkin, encoded by the PARK2 gene, is responsible for conjugating Ub-molecules to substrates (Riley et al., 2013). Autophagy receptor proteins, such as optineurin (OPTN) and nuclear dot protein 52 (NDP52), play a pivotal role in the PINK1/Parkin pathway (Lazarou et al., 2015; Heo et al., 2015; Wong and Holzbaur, 2014). These autophagy receptor proteins bind to ubiquitinated substrates and associate with ATG8 family members, facilitating the capture of damaged mitochondria by autophagosomes through the Atg8ylation machinery (Atg8yl-Mach). The Atg8yl-Mach is composed of the ATG12-ATG5-ATG16L1 complex, which anchors ATG8 to the autophagosome membrane by promoting its conjugation to phosphatidylethanolamine, thereby promoting the formation and expansion of autophagosomes on the surface of damaged mitochondria. Members of the ATG8 family are divided into the microtubule-associated protein one light chain 3 (LC3) and GABA receptor-associated protein (GABARAP) subgroups. During the downstream process of mitophagy initiation, ATG8 family members play a crucial role in the fusion of autophagosomes with lysosomes for the degradation of mitochondrial substrates, a process primarily driven by the GABARAP subgroup (Nguyen et al., 2016; Vaites et al., 2017).
In addition to the classical PINK1/Parkin pathway, there exist other Ub-dependent pathways that are independent of Parkin. In Parkin-independent mitophagy, activated PINK1 can directly recruit autophagy receptor proteins to mitochondria by phosphorylation of Ub at the serine 65 site. Next, the Ub chains generated on OMM substrates serve as recruitment platforms for Ub-binding autophagy receptor proteins, including sequestosome one/p62 (SQSTM1/p62), neighbor of BRCA1 (NBR1), OPTN, NDP52, and Tax1-binding protein 1 (TAX1BP1). These receptor proteins function by initiating the formation of autophagosomes (Lazarou et al., 2015; Richter et al., 2016). The aforementioned OMM autophagy-related proteins contain both LC3-interacting region (LIR) and Ub-binding domain (UBD), thereby mediating the binding of LC3 to Ub chains on targeted mitochondria. As a result, these proteins anchor ubiquitinated mitochondria to autophagosomes (Fan et al., 2021).
Ub chains are not the only factor recruiting autophagy receptor proteins. The autophagy receptor proteins on the OMM inherently possess LIR. These autophagy receptors can directly bind to LC3 without ubiquitination, thus initiating mitophagy. In mammals, such receptors primarily include NIX receptor, BCL2-interacting protein 3 (BNIP3) receptor, and FUN14 domain-containing protein 1 (FUNDC1) receptor, among others (Lu et al., 2023) (Figure 2).
NIX and BNIP3 share 56% homology and both contain the BCL2 homology 3 (BH3) domain, which allows them to directly bind to LC3 through their BH3 domains and induce mitophagy (Novak et al., 2010). FUNDC1 can interact with LC3 to induce Parkin-independent mitophagy under hypoxic conditions (Liu et al., 2012).
Non-canonical autophagy, independent of autophagosome formation, represents an endosomal-dependent mitophagy pathway activated under specific stress conditions. Specifically, upon mitochondrial DNA damage, mitochondrial nucleoids are eliminated via the endosome-mitophagy pathway. MitoEVs that bud from mitochondrial networks have been implicated as a means of delivering mitochondrial components to lysosomes (Konig et al., 2021; Iorio et al., 2024; Soubannier et al., 2012), thereby compensating for deficiencies in canonical mitophagy (Towers et al., 2021). In addition to mitoEVs-mediated mitophagy, mitochondrial herniation leads to the exposure and ubiquitination of the IMM, initiating the induction of an apoptotic mitophagy sequestration pathway. IMM-mitophagy has the potential to capture herniating mitochondria, theoretically preventing mtDNA release into the cytosol at an earlier stage of the process before any transcriptional response can be triggered. Using proximity proteomics, researchers have also identified the protein required for the clearance of mutated mitochondrial nucleoids from the mitochondrial matrix. Among these, ATAD3 and SAMM50 regulate both the architecture of mitochondrial cristae and nucleoid interactions. SAMM50 cooperates with the retromer complex protein VPS35 to sequester mitochondrial DNA within endosomes, thereby preventing excessive immune response (Sen et al., 2023).
The complex multi-tissue structure of the intervertebral disc allows it to absorb and distribute mechanical stresses during physical activities (Konig et al., 2021). Specifically, the NP is primarily composed of NP cells and extracellular matrix (ECM), with the ECM of the NP consisting of type II collagen and proteoglycans (Risbud et al., 2015). NP cells maintain the biomechanical homeostasis of the NP by synthesizing and secreting ECM (Vamvakas et al., 2017; Lin J. et al., 2019; Silagi et al., 2018). Nutrients and metabolites enter and exit the disc through diffusion within the dense ECM (Wise et al., 2020). Evidence suggests that IVDD initially occurs in the NP region of the disc (Guerrero et al., 2021), and research on the mechanisms and therapeutic strategies of IVDD has predominantly focused on NP cells (Xin et al., 2022; Wu et al., 2022; Zhang et al., 2021; Sun et al., 2022). Currently, the etiology of RCD induced by mitochondrial dysfunction in NP cells involves multiple factors, including inflammation, oxidative stress, nutrient deficiency, compression, and hyperlipidemia (Figure 3).
Figure 3. The mitophagy in distinct regions of IVDD NP, nucleus pulposus; AF, annulus fibrosus; CEP, cartilage endplate; ECM, extracellular matrix.
Firstly, inflammation is considered a significant pathogenic factor. Pro-inflammatory cytokines, such as interleukin-1β (IL-1β), tumor necrosis factor-α (TNF-α), and interleukin-6 (IL-6), collectively accelerate the progression of IVDD by promoting ECM degradation, chemokine production, immune cell recruitment, and phenotypic changes in disc cells (Xu et al., 2017; Zhang et al., 2018; Risbud and Shapiro, 2014; Peng et al., 2022). The relationship between inflammation induction and mitophagy has garnered attention from Zhang and his team (Zhang et al., 2018). They made pioneering observations that Parkin expression is not only elevated in degenerated human NP but also increased in rat NP stimulated with TNF-α. Meanwhile, TNF-α stimulates NP cells to produce more reactive oxygen species (ROS), subsequently activating autophagy and apoptosis processes. During this process, despite increased expression levels of LC3 and Beclin-1, p62 levels also rise in NP cells, indicating impaired autophagy flux. Dysfunction in Parkin-dependent mitophagy has been confirmed as a pivotal cause. Further research has found that the NF-κB signaling pathway is a potential mechanism through which pro-inflammatory cytokines exert their effects. Studies by Zhao et al. (2020) and Yu et al. (2021) demonstrated that the inflammation induced by TNF-α can affect mitochondrial function in NP cells through the NF-κB pathway, which in turn triggers a series of secondary phenotypic changes, including exacerbated inflammation, oxidative stress, and pyroptosis. Among these, infiltration and activation of immune cells further amplify the inflammatory cascade, leading to aggravated inflammation (Risbud and Shapiro, 2014). Cytokines induce oxidative stress by increasing ROS accumulation (Yang et al., 2023). Furthermore, pyroptosis is dependent on inflammasome activation and is accompanied by the massive release of inflammatory cytokines. Evidence suggests that activated NLRP3 inflammasomes aggregate around mitochondria, and their potential detrimental effects in IVDD have attracted widespread attention (Zhou et al., 2011; Xia et al., 2019). NLRP3 inflammasome induces pyroptosis and release inflammatory cytokines in NP cells, a process that promotes the secretion of metalloproteinases and leads to NP degradation (Song et al., 2017; A et al., 2020), thereby accelerating the pathological progression of IVDD (Tang et al., 2021). Research by Peng et al. (Peng et al., 2022) found that in a lipopolysaccharide (LPS) induced inflammation model, activation of the NLRP3 inflammasome promotes ROS production and inhibits mitophagy flux. This leads to pyroptosis and apoptosis of NP cells, resulting in accelerated degeneration of the intervertebral disc NP.
Secondly, mitochondria are both the primary source of cellular ROS and highly susceptible to oxidative stress damage, leading to dysfunction (Hm et al., 2015). Impaired mitophagy will result in mitochondrial dysfunction and ROS accumulation (Zhang et al., 2018; Lin Q. et al., 2019). ROS accumulation, in turn, leads to exaggerated inflammation, disordered metabolism, and enhanced apoptosis in cells (Zhou et al., 2011). Among the molecules triggered by mitochondrial ROS, the NLRP3 inflammasome has been extensively studied for its detrimental role in IVDD (Zhou et al., 2011; Xia et al., 2019). Activation of the NLRP3 inflammasome can elevate the production of IL-1β, which facilitates the secretion of metalloproteinases and subsequently causes degradation of NP tissue (Song et al., 2017; A et al., 2020). Furthermore, NLRP3 is linked to the mitochondrial apoptosis pathway, programmed cell death, and apoptosis through several mechanisms in NP cells (Wn et al., 2019). In Wang et al.‘s (Y et al., 2018) study, treatment of human NP cells with H2O2 led to impaired mitophagy, manifesting as ROS accumulation, decreased ECM synthesis, and accelerated senescence. These alterations collectively contributed to the degeneration of NP cells. Additionally, tert-butyl hydroperoxide (TBHP) is also widely used to simulate oxidative stress environments. Various scholars (Xie et al., 2019; Lin et al., 2020; Wang et al., 2018; Wn et al., 2019; Chen et al., 2019; Chen Y. et al., 2020) have explored the role of mitophagy in TBHP-induced oxidative stress using different experimental models, finding that TBHP treatment results in impaired mitophagy in NP cells of the intervertebral disc. This includes both the Ub-dependent classical pathway, characterized by decreased expression levels of PINK1 and Parkin proteins, reduced LC3 II/I ratio, and decreased MMP and ATP levels, as well as BNIP3-mediated Ub-independent mitophagy (Wang et al., 2018). BNIP3, initially identified as a pro-apoptotic protein, features an atypical BH3 domain localized to the OMM. Induction of BNIP3 triggers the translocation and activation of BCL2-antagonist/killer 1 (BAK1) and Bcl-2 Associated X Protein (BAX) to mitochondria, leading to increased mitochondrial membrane permeability, subsequent release of cytochrome C from mitochondria to the cytosol, and ultimately initiating the caspase cascade of apoptosis. Emerging research has found that BNIP3 also serves as a key receptor for mitophagy, playing a role in promoting cell survival (Madhu et al., 2020). The bidirectional functions of BNIP3 in apoptosis and mitophagy suggest that it may be a critical regulator of cell fate (Madhu et al., 2023). The aforementioned changes in mitophagy will further affect processes such as apoptosis, senescence, ROS generation, and ECM degradation, ultimately influencing the degeneration of the NP of the intervertebral disc.
In addition to inflammation and oxidative stress, nutrient deficiency and abnormal mechanical loads are also key risk factors for IVDD. Wang et al. (2020b) found that nutrient deficiency and aging can downregulate FOXO3, leading to mitochondrial dysfunction and inhibited mitophagy, resulting in increased NP cell apoptosis and ECM degradation. Abnormal compressive forces, tensile forces, and increased matrix stiffness all exert detrimental effects on disc cells (Wang D. et al., 2022; Xiao et al., 2022; Wang et al., 2021). Further research has shown that excessive mechanical stress applied to NP cells can also lead to oxidative stress and mitochondrial dysfunction (Kang et al., 2020a; Hu et al., 2022). Kang et al. (Kang et al., 2020a) found that mechanical compression can cause mitochondrial dysfunction in NP cells of the intervertebral disc, with increased ROS production, exacerbated mitochondrial dysfunction, and increased apoptosis, thereby promoting NP cell degeneration. Mitochondrial dysfunction can further increase ROS production, leading to a vicious cycle between mitochondrial dysfunction and ROS accumulation, causing sustained oxidative damage (Chen et al., 2018).
Combining the above evidence, mitochondrial damage leads to pathological phenotypes in NP cells, including apoptosis, oxidative stress, senescence, ECM degradation and synthesis, excessive autophagy, exacerbated inflammation, and pyroptosis (Table 1).
As the outer structure of the intervertebral disc, the primary physiological function of the AF lies in its ability to effectively encapsulate the NP, preventing its herniation through its unique hydraulic sealing properties, and evenly distributing the various pressures acting on the disc (Moore, 2006). The AF comprises two distinct components: the inner AF, which is adjacent to the NP and consists of chondrocytes with an ECM primarily composed of type II collagen; and the outer AF, which is mainly composed of fibroblast-like cells with an ECM primarily composed of type I collagen. This lamellar structure provides the intervertebral disc with high flexibility and adaptability across multiple planes of motion, ensuring the stability and normal function of the disc structure (Smith et al., 2011; Roughley, 2004). The outer AF receives nutritional support from capillaries within the surrounding soft tissues, while the rest of the AF exchanges nutrients and metabolic waste through a capillary network at the CEP via diffusion (Wise et al., 2020) (Figure 3). Due to the unique structure and physiological function of the AF, the pathological repair process following AF injury exhibits distinct characteristics (Bailey et al., 2013). Clinically, both acute trauma and chronic degeneration of the AF can exacerbate IVDD. Studies have demonstrated that AF injury contributes to disc instability and disrupts the intradiscal microenvironment. Meanwhile, AF injuries often persist due to insufficient endogenous repair capacity [105]. Histologically, AF scar healing is predominantly characterized by disorganized type III collagen deposition, with sparse type I collagen bundles observed in the outer layer. The key subsequent effects include reduced resistance to small molecule permeation, decreased tensile strength, diminished disc height, NP fibrosis, and CEP subchondral ossification (Kuivaniemi and Tromp, 2019). These findings underscore the necessity of elucidating AF repair mechanisms and their systemic impact on disc homeostasis.
Oxidative stress and abnormal lipid metabolism are currently recognized as significant factors inducing IVDD through mitophagy-mediated AF damage. Xu et al. (Konig et al., 2021) demonstrated that TBHP-induced oxidative stress can lead to mitochondrial dysfunction accompanied by downregulation of mitophagy levels. When mitophagy function is impaired, oxidative stress and apoptosis levels significantly increase, accelerating the occurrence and progression of IVDD. Further research revealed that SIRT2, upstream of this pathway, is a key target that influences the expression levels of peroxisome proliferator-activated receptor γ coactivator 1α (PGC-1α), Parkin, and LC3 II, thereby regulating mitophagy. Wu et al. (Wu et al., 2021) found that oxidized low-density lipoprotein (oxLDL) can promote mitochondrial fission, further exacerbating mitochondrial dysfunction and increasing AF cell apoptosis, thereby accelerating the pathological process of IVDD.
In brief, oxidative stress and abnormal lipid metabolism are crucial factors inducing IVDD through mitophagy-mediated AF damage. The pathological phenotypes of AF cells resulting from mitochondrial damage include apoptosis and oxidative stress (Table 1).
The CEP consists of a biological tissue layer rich in type II collagen and chondrocytes, situated between the intervertebral disc and adjacent vertebral body. It is similar to other articular tissues in the body, and it exhibits the highest cellular density among all structures of the intervertebral disc (Kirnaz et al., 2022). The CEP serves as the primary pathway for nutrient delivery from vertebral body capillaries to the disc, as well as for the excretion of waste products from the disc. Degeneration of the CEP can hinder the nutrition and waste exchange of the intervertebral disc, leading to the disruption of its homeostasis and the initiation of IVDD (Wong et al., 2019). Kang et al. (Kang et al., 2020b) investigated the regulatory role of oxidative stress on mitophagy mechanisms in CEP cells. Their study induced an oxidative stress state with H2O2, resulting in mitochondrial dysfunction manifested as decreased MMP, reduced ATP synthesis, increased ROS levels, and opening of the mitochondrial permeability transition pore (mPTP). Concurrently, mitophagy was inhibited, further exacerbating cellular apoptosis and ultimately accelerating the progression of IVDD (Figure 3).
In summary, impaired mitophagy function leads to a series of pathological phenotypes in CEP cells, including enhanced apoptosis and oxidative stress. Additionally, this mechanism has been extensively studied in other articular cartilage tissues structurally similar to CEP cells and has been shown to be closely related to the pathological processes of these tissues (Sun et al., 2021) (Table 1).
Mitophagy, as an important mechanism for cellular self-renewal and homeostasis maintenance, exhibits significant correlations with various forms of RCD (Sun et al., 2018; Sun et al., 2019). It is important to note that mitophagy is a double-edged sword. Moderate mitophagy can protect cells from various external stimuli, whereas excessive mitophagy can also accelerate cellular apoptosis and the progression of IVDD (Kang et al., 2020a; Xu W-N. et al., 2019).
Intervertebral disc cells reside in a physically avascular and hypoxic microenvironment, primarily relying on anaerobic glycolysis for energy production (Urban et al., 2004). Based on this observation, it was once widely accepted in the academic community that, compared to cells dependent on aerobic metabolism, intervertebral disc cells contain fewer functional mitochondria (Madhu et al., 2020; Gan et al., 2003). However, subsequent studies have revealed the presence of a functional mitochondrial network within NP cells, capable of adjusting mitochondrial quantity through active mitochondrial flux to match metabolic demands. Furthermore, intervertebral disc metabolism is relatively active, and its internal cells, due to oxygen scarcity, have developed compensatory mechanisms to counteract relative hypoxia, including upregulation of HIF-1α and others (Risbud et al., 2010; Theodore, 2020). Additionally, mitochondrial dysfunction and abnormal mitochondrial morphology can be observed in degenerated intervertebral disc cells (Hu et al., 2022; Song et al., 2018). Consequently, therapeutic strategies aimed to ameliorate IVDD through modulation of mitophagy have emerged as a focal point of current research, increasingly gaining attention and recognition from scholars in the field.
Numerous natural products exhibit therapeutic potential for improving IVDD by modulating mitophagy levels. Hydrogen sulfide (H2S), along with nitric oxide and carbon monoxide, is regarded as one of three endogenously produced gaseous signaling molecules. These molecules possess diverse biological functions, including anti-inflammatory and anti-apoptotic effects, and exert impacts on multiple key mechanisms and pathways both in vivo and in vitro (Hu et al., 2007; Hu et al., 2009). Recent studies have demonstrated that H2S effectively improves mitochondrial function by closing the mPTP, enhancing MMP, and ATP levels, thereby reducing cellular apoptosis and showing therapeutic potential for IVDD (Xu et al., 2017). Salidroside, a phenylpropanoid glycoside extracted from Rhodiola, and Polydatin, a resveratrol glycoside extracted from the rhizomes of Polygonum cuspidatum, can both activate mitophagy through a Parkin-dependent pathway, upregulate Parkin protein expression, promote the reduction of ROS accumulation, and effectively inhibit cellular apoptosis, thereby ameliorating mitochondrial damage and apoptosis in NP and CEP cells, respectively (Zhang et al., 2018; Kang et al., 2020b). Notably, Polydatin can also simultaneously activate the Nrf2 pathway, upregulating Nrf2 protein expression and its nuclear translocation, further improving mitochondrial dysfunction (Kang et al., 2020b). Urolithin A, a metabolite of ellagitannins and ellagic acid abundant in pomegranates, strawberries, and other nuts (Cerdá et al., 2005), can specifically induce mitophagy both in vivo and in vitro (Ryu et al., 2016; Fang et al., 2019). Mechanistic studies have shown that Urolithin A inhibits NP cell apoptosis by activating mitophagy through the AMPK pathway, thereby slowing down the progression of IVDD (Lin et al., 2020). Honokiol, a natural flavonoid compound derived from the roots and bark of Magnolia officinalis, also exerts therapeutic effects by activating the AMPK pathway. Honokiol demonstrates multiple pharmacological effects such as antioxidant, anti-lipid peroxidation, anti-inflammatory, and neuroprotective activities, showing therapeutic potential in cartilage protection and IVDD (Chen et al., 2014; Chen et al., 2015). To explore the mechanism of Honokiol-induced SIRT3 expression enhancement, Wang et al. (Wang et al., 2018) examined the activation of two major energy sensor molecules, namely, AMPK and PGC-1α. By activating the AMPK pathway, upregulating PGC-1α and SIRT3, regulating mitochondrial dynamics, and enhancing mitophagy flux through the autophagy receptor BNIP3, Honokiol protects NP cells from oxidative stress damage, reversing the processes of aging and apoptosis (Wang et al., 2018). Furthermore, natural isothiocyanate compounds such as Sulforaphane, found in cruciferous vegetables, especially broccoli, have been shown to improve mitochondrial dysfunction, reduce mitochondrial morphological abnormalities, and enhance mitochondrial dynamics. The specific mechanisms involve upregulating PGC-1α expression and AMPK phosphorylation, reducing ROS accumulation, delaying aging, inhibiting apoptosis, and reducing ECM degradation (Xu X. et al., 2019). Mangiferin exhibits potent free radical scavenging activity, with mango trees serving as its primary and readily accessible source (Zhao et al., 2017). It possesses multiple pharmacological potentials, including antioxidant, anti-inflammatory, anti-diabetic, anti-hyperlipidemic, and anti-atherosclerotic properties, with mechanisms involving the counteraction of oxidative stress and mitochondrial dysfunction (Alberdi et al., 2018; Li et al., 2019). Opa1, Drp1, and TFAM are biomarkers of mitochondrial dynamics. Mangiferin not only downregulates Drp1 expression but also upregulates Opa1 and TFAM levels, thereby reducing inflammation, ECM degradation, oxidative stress, and apoptosis (Yu et al., 2021). Selenium can also reduce oxidative stress and cellular apoptosis by regulating mitochondrial dynamics and the expression of autophagy-related proteins (Wang P. et al., 2022) (Table 2).
Beyond natural products, certain hormones have also been identified as possessing the potential to regulate mitophagy. For instance, Melatonin, an endogenous molecule released by the pineal gland, has been proven to effectively delay oxidative stress, inflammatory responses, and apoptosis in osteoarthritis models (Pei et al., 2009; Liu et al., 2013; Lim et al., 2012), while also enhancing mitophagy levels in various tissues such as the brain and liver (Lin et al., 2016; Kang et al., 2016). Chen et al. (Chen et al., 2019) found that Melatonin can promote mitophagy by upregulating Parkin protein expression and the LC3 II/I ratio, thereby improving oxidative stress-induced mitochondrial dysfunction and apoptosis, and exhibiting potential therapeutic effects on IVDD. Cortistatin, a cyclic neuropeptide, is an appealing therapeutic candidate in the treatment of degenerative and inflammatory diseases (Gonzalez-Rey et al., 2007; Duran-Prado et al., 2013; Gruber et al., 2014), including its role in mitigating TNF-α-induced chondrocyte inflammation to counteract articular cartilage degeneration in osteoarthritis (Zhao et al., 2019). Zhao et al. (Zhao et al., 2020) discovered that Cortistatin inhibits apoptosis by suppressing the NF-κB pathway and regulating mitochondrial dynamics, thereby reducing ROS accumulation and NLRP3 inflammasome activation. Specifically, in this study, Cortistatin, by activating the AMPK/PGC-1α pathway, upregulated the expression levels of fusion-related markers Opa1, Mfn1, and Mfn2, while simultaneously downregulating the expression of fission marker Drp1. Inhibition of proteins involved in mitochondrial fission also demonstrated a positive therapeutic effect on IVDD (Wu et al., 2021) (Table 2).
Targeted strategies aimed at mitochondrial function also constitute effective avenues for regulating mitophagy and ameliorating IVDD. Studies have confirmed that oxidative products are significantly increased in IVDD, and inhibiting the excessive production of ROS while promoting their clearance has been demonstrated to effectively delay the progression of IVDD [136–138] (Kang et al., 2020a; Suzuki et al., 2015). Among these, activating the Nrf2 antioxidant defense system emerges as a potent therapeutic strategy for IVDD. Nrf2, a crucial redox-sensitive transcription factor, regulates the antioxidant system by activating the expression of cytoprotective genes in response to oxidative stress (Xiang et al., 2022). For instance, antioxidants such as MitoQ and Mito-TEMPO significantly mitigate oxidative stress and mitochondrial dysfunction by activating the Nrf2 pathway and upregulating PINK1/Parkin-mediated mitophagy (Kang et al., 2020a; Kang et al., 2020b).
With the advancement of gene editing technology, an increasing number of studies have begun to explore their potential in regulating mitophagy and improving IVDD. For example, knocking down PINK1 expression using sh-PINK1 can affect the mitophagy process, leading to accelerated aging and increased ROS accumulation (Y et al., 2018). Additionally, circERCC2 promotes PINK1/Parkin-mediated mitophagy by downregulating miR-182-5p and upregulating SIRT1, thereby reducing NP cell apoptosis, senescence, and ECM degradation (Xie et al., 2019). Additionally, overexpression of genes such as Mfn2 and FOXO3 has also shown positive effects on mitophagy and IVDD treatment (Chen Y. et al., 2020; Wang et al., 2020b).
During the development of IVDD, various risk factors have been found to induce mitochondrial damage by inhibiting mitophagy, ultimately leading to RCD of disc cells. Therefore, enhancing mitophagy is considered a potential therapeutic approach for alleviating IVDD (Wang et al., 2020c; Lan et al., 2022). However, not all activation of mitophagy is positively correlated with halting the progression of IVDD. Excessive mitochondrial fission and autophagy can directly contribute to the occurrence and development of IVDD (Lin et al., 2023). Prolonged duration of mechanical loading has been reported to result in excessive removal of mitochondria by mitophagy, thus exacerbating NP cell senescence, and inhibiting mitophagy can have a positive effect (Huang et al., 2020). Further research has found that overexpression of the NDUFA4L2 gene exerts a positive therapeutic effect on IVDD by inhibiting excessive mitophagy induced through the Parkin-dependent pathway (Wn et al., 2019). NLRX1, as the only Nod-like receptor located in mitochondria, plays a crucial role in sensing mitochondrial damage and regulating mitochondrial function (Zhang et al., 2019; Killackey et al., 2022; Killackey et al., 2023). Song et al. (Song et al., 2024) revealed that mitophagy is activated in both NLRX1-overexpressing and NLRX1-deficient NP cells exposed to oxidative stress. However, distinct biological outcomes were observed. When NLRX1 was overexpressed, pharmacological intervention targeting the NLRX1-SLC39A7 pathway showed great potential for promoting disc regeneration. Conversely, NLRX1 deficiency promoted PINK1/Parkin-mediated mitophagy, inducing excessive mitophagy and accelerating the progression of IVDD. Therefore, from a mechanistic perspective, the zinc transporter SLC39A7, a novel NLRX1-interacting protein, has been identified and proven to regulate mitochondrial dynamics and promote beneficial and synchronized mitophagy (Song et al., 2024) (Table 2).
Proteins such as HSP70 also mitigate oxidative stress and apoptosis by modulating mitochondrial dynamics and the expression of autophagy-related proteins (Hu et al., 2022). Furthermore, proteins including A20 and SIRT2 have been identified as possessing potential to regulate mitophagy (Xu W-N. et al., 2019; Peng et al., 2022). In the study by Peng et al. (Peng et al., 2022), following LPS treatment, the mitochondrial fission protein Drp1 translocated from the cytoplasm to mitochondria, while the expression of Mfn1 significantly decreased. A20 mitigated the LPS-induced changes and promoted the normalization of mitochondrial morphology. A20 significantly reduced the NLRP3 aggregation around mitochondria induced by LPS. Analysis revealed that A20 protected NP cells from LPS-induced mPTP collapse and massive ROS production. These findings suggest that A20 may exert protective effects by facilitating the elimination of ROS through mitophagy.
On the other hand, mitochondria are highly dynamic organelles undergoing continuous fission and fusion, a process termed mitochondrial dynamics (Westermann, 2010a). When cells undergo metabolic or environmental stress, the quantity and quality of mitochondria are regulated through continuous processes of fusion and fission (Pernas and Scorrano, 2016; Kraus and Ryan, 2017). Fusion aids in stress alleviation by mixing the contents of partially damaged mitochondria as a form of complementation. Fission is necessary for the generation of new mitochondria, but it also contributes to quality control by enabling the removal of damaged mitochondria and can facilitate apoptosis under high levels of cellular stress (Youle and van der Bliek, 2012). Mitochondrial fusion and fission are crucial for a wide range of cellular functions, including energy metabolism, development, aging, and cell death. Mitochondrial fusion and fission are crucial for a great variety of cellular functions, including energy metabolism, development, aging and cell death. The core mechanisms involved have been identified and analyzed in diverse model organisms (Westermann, 2010b). A delicate balance in mitochondrial dynamics is conducive to maintaining a healthy mitochondrial pool (Lee and Yoon, 2016). Disruption of this balance is associated with various human diseases, including cancer, type 2 diabetes, and osteoarthritis (Rovira-Llopis et al., 2017; Srinivasan et al., 2017; Yao et al., 2019). Mitophagy and mitochondrial dynamics are interrelated but distinct processes. During the process of mitochondrial fission, damaged daughter mitochondria are first segregated and subsequently targeted for elimination by lysosomes, thereby preventing their reintegration into the pool of active and healthy mitochondria through fusion (Kang et al., 2020a). Maintaining a healthy mitochondrial pool is crucial for disc cells in both physiological adaptation and pathological responses to external stimuli. Therefore, in addition to direct interventions targeting mitophagy, appropriate mitochondrial quality control also merits investigation.
IIVDD is closely associated with a range of spinal disorders, including lumbar disc herniation, spinal canal stenosis, degenerative spondylolisthesis, and scoliosis, which impose significant economic burdens on both individuals and society (Hartvigsen et al., 2018). During the progression of IVDD, various modes of RCD exist within the intervertebral disc, such as apoptosis, pyroptosis, senescence, and autophagy-dependent cell death, which can occur independently or in combination (Yang et al., 2022). To date, effective therapeutic strategies for IVDD are still under continuous exploration.
By Delving into the mechanisms underlying the role of mitochondrial function in IVDD, the modulation of mitophagy has emerged as one of the important directions for improving therapeutic strategies for IVDD. Various interventions, including natural products, hormones, targeted compounds, gene editing technologies, and related proteins, have demonstrated positive effects on mitophagy, providing new directions and strategies for the treatment of IVDD. However, enhancing mitophagy is not always a favorable approach for treating IVDD, and treatment decisions should be based on a clear understanding of the molecular background. Therefore, whether mitophagy is beneficial or harmful to health depends on cellular and microenvironmental factors (Zhou et al., 2019). Meanwhile, both mitophagy and mitochondrial dynamics are key mechanisms for maintaining mitochondrial homeostasis (K et al., 2018).
Current research still faces numerous challenges and unknown areas, such as the interactions between different mechanisms and the long-term safety and efficacy of intervention methods. Therefore, future studies need to explore the specific mechanisms of these strategies in greater depth and validate and optimize them in clinical practice, aiming to provide more effective and safe treatment options for patients.
CF: Conceptualization, Writing – original draft. ZH: Data curation, Writing – original draft. MZ: Conceptualization, Writing – original draft. CL: Data curation, Writing – original draft. GL: Data curation, Writing – original draft. FY: Writing – review and editing. XF: Writing – review and editing.
The author(s) declare that financial support was received for the research and/or publication of this article. This work is supported by grants from Health Commission of Sichuan Province (CJY2025-501&CGB2024051), Sichuan Provincial Administration of Traditional Chinese Medicine (2024ZD025), and Clinical Medicine School of Chengdu University of Traditional Chinese Medicine (2023KCZ01).
The authors declare that the research was conducted in the absence of any commercial or financial relationships that could be construed as a potential conflict of interest.
The author(s) declare that no Generative AI was used in the creation of this manuscript.
All claims expressed in this article are solely those of the authors and do not necessarily represent those of their affiliated organizations, or those of the publisher, the editors and the reviewers. Any product that may be evaluated in this article, or claim that may be made by its manufacturer, is not guaranteed or endorsed by the publisher.
Alberdi, E., Sánchez-Gómez, M. V., Ruiz, A., Cavaliere, F., Ortiz-Sanz, C., Quintela-López, T., et al. (2018). Mangiferin and morin attenuate oxidative stress, mitochondrial dysfunction, and neurocytotoxicity, induced by amyloid beta oligomers. Oxid. Med. Cell Longev. 2018, 2856063. doi:10.1155/2018/2856063
A, M., A, D. C., C, P., C, A.-F., A, P., N, P., et al. (2020). A mouse model of MSU-induced acute inflammation in vivo suggests imiquimod-dependent targeting of Il-1β as relevant therapy for gout patients. Theranostics, 10. doi:10.7150/thno.40650
Ashrafi, G., and Schwarz, T. L. (2013). The pathways of mitophagy for quality control and clearance of mitochondria. Cell Death Differ. 20, 31–42. doi:10.1038/cdd.2012.81
Bailey, A., Araghi, A., Blumenthal, S., and Huffmon, G. V.Anular Repair Clinical Study Group (2013). Prospective, multicenter, randomized, controlled study of anular repair in lumbar discectomy: two-year follow-up. Spine 38, 1161–1169. doi:10.1097/BRS.0b013e31828b2e2f
Bi, R., Li, Y., Xu, M., Zheng, Q., Zhang, D.-F., Li, X., et al. (2022). Direct evidence of CRISPR-Cas9-mediated mitochondrial genome editing. Innovation 3, 100329. doi:10.1016/j.xinn.2022.100329
Binch, A. L. A., Fitzgerald, J. C., Growney, E. A., and Barry, F. (2021). Cell-based strategies for IVD repair: clinical progress and translational obstacles. Nat. Rev. Rheumatol. 17, 158–175. doi:10.1038/s41584-020-00568-w
Birgisdottir, Å. B., Lamark, T., and Johansen, T. (2013). The LIR motif - crucial for selective autophagy. J. Cell Sci. 126, 3237–3247. doi:10.1242/jcs.126128
Cerdá, B., Periago, P., Espín, J. C., and Tomás-Barberán, F. A. (2005). Identification of urolithin a as a metabolite produced by human colon microflora from ellagic acid and related compounds. J. Agric. Food Chem. 53, 5571–5576. doi:10.1021/jf050384i
Chen, C.-H., Chiang, C.-J., Wu, L.-C., Yang, C.-H., Kuo, Y.-J., and Tsai, T.-H. (2015). In vitro penetration and in vivo distribution of Honokiol into the intervertebral disc in rat. Anal. Sci. Int. J. Jpn. Soc. Anal. Chem. 31, 1297–1302. doi:10.2116/analsci.31.1297
Chen, G., Kroemer, G., and Kepp, O. (2020a). Mitophagy: an emerging role in aging and age-associated diseases. Front. Cell Dev. Biol. 8, 200. doi:10.3389/fcell.2020.00200
Chen, S., Lv, X., Hu, B., Zhao, L., Li, S., Li, Z., et al. (2018). Critical contribution of RIPK1 mediated mitochondrial dysfunction and oxidative stress to compression-induced rat nucleus pulposus cells necroptosis and apoptosis. Apoptosis Int. J. Program Cell Death 23, 299–313. doi:10.1007/s10495-018-1455-x
Chen, Y., Lin, J., Chen, J., Huang, C., Zhang, Z., Wang, J., et al. (2020b). Mfn2 is involved in intervertebral disc degeneration through autophagy modulation. Osteoarthr. Cartil. 28, 363–374. doi:10.1016/j.joca.2019.12.009
Chen, Y., Wu, Y., Shi, H., Wang, J., Zheng, Z., Chen, J., et al. (2019). Melatonin ameliorates intervertebral disc degeneration via the potential mechanisms of mitophagy induction and apoptosis inhibition. J. Cell Mol. Med. 23, 2136–2148. doi:10.1111/jcmm.14125
Chen, Y. J., Tsai, K. S., Chan, D. C., Lan, K. C., Chen, C. F., Yang, R. S., et al. (2014). Honokiol, a low molecular weight natural product, prevents inflammatory response and cartilage matrix degradation in human osteoarthritis chondrocytes. J. Orthop. Res. Off. Publ. Orthop. Res. Soc. 32, 573–580. doi:10.1002/jor.22577
Clark, I. E., Dodson, M. W., Jiang, C., Cao, J. H., Huh, J. R., Seol, J. H., et al. (2006). Drosophila pink1 is required for mitochondrial function and interacts genetically with parkin. Nature 441, 1162–1166. doi:10.1038/nature04779
Collaborators, G. (2021). Global, regional, and national burden of neck pain, 1990-2020, and projections to 2050: a systematic analysis of the Global Burden of Disease Study 2021. Lancet Rheumatol. 6, e142–e155. doi:10.1016/S2665-9913(23)00321-1
Dave, K. D., De Silva, S., Sheth, N. P., Ramboz, S., Beck, M. J., Quang, C., et al. (2014). Phenotypic characterization of recessive gene knockout rat models of Parkinson’s disease. Neurobiol. Dis. 70, 190–203. doi:10.1016/j.nbd.2014.06.009
Dc, W. (2013). A mitochondrial bioenergetic etiology of disease. J. Clin. Invest, 123. doi:10.1172/JCI61398
Ding, W.-X., Ni, H.-M., Li, M., Liao, Y., Chen, X., Stolz, D. B., et al. (2010). Nix is critical to two distinct phases of mitophagy, reactive oxygen species-mediated autophagy induction and Parkin-ubiquitin-p62-mediated mitochondrial priming. J. Biol. Chem. 285, 27879–27890. doi:10.1074/jbc.M110.119537
Doblado, L., Lueck, C., Rey, C., Samhan-Arias, A. K., Prieto, I., Stacchiotti, A., et al. (2021). Mitophagy in human diseases. Int. J. Mol. Sci. 22, 3903. doi:10.3390/ijms22083903
Duran-Prado, M., Morell, M., Delgado-Maroto, V., Castaño, J. P., Aneiros-Fernandez, J., de Lecea, L., et al. (2013). Cortistatin inhibits migration and proliferation of human vascular smooth muscle cells and decreases neointimal formation on carotid artery ligation. Circ. Res. 112, 1444–1455. doi:10.1161/CIRCRESAHA.112.300695
Duve, C. de, and Wattiaux, R. (1966). Functions of lysosomes. Annu. Rev. Physiol. 28, 435–492. doi:10.1146/annurev.ph.28.030166.002251
Fan, S., Yue, L., Wan, W., Zhang, Y., Zhang, B., Otomo, C., et al. (2021). Inhibition of autophagy by a small molecule through covalent modification of the LC3 protein. Angew. Chem. Int. Ed. Engl. 60, 26105–26114. doi:10.1002/anie.202109464
Fang, E. F., Hou, Y., Palikaras, K., Adriaanse, B. A., Kerr, J. S., Yang, B., et al. (2019). Mitophagy inhibits amyloid-β and tau pathology and reverses cognitive deficits in models of Alzheimer’s disease. Nat. Neurosci. 22, 401–412. doi:10.1038/s41593-018-0332-9
Gan, J. C., Ducheyne, P., Vresilovic, E. J., and Shapiro, I. M. (2003). Intervertebral disc tissue engineering II: cultures of nucleus pulposus cells. Clin. Orthop. 411, 315–324. doi:10.1097/01.blo.0000063797.98363.d3
Gan, Z. Y., Callegari, S., Cobbold, S. A., Cotton, T. R., Mlodzianoski, M. J., Schubert, A. F., et al. (2022). Activation mechanism of PINK1. Nature 602, 328–335. doi:10.1038/s41586-021-04340-2
GBD 2017 Disease and Injury Incidence and Prevalence Collaborators (2018). Global, regional, and national incidence, prevalence, and years lived with disability for 354 diseases and injuries for 195 countries and territories, 1990-2017: a systematic analysis for the Global Burden of Disease Study 2017. Lancet Lond Engl. 392:1789–1858. doi:10.1016/S0140-6736(18)32279-7
GBD 2019 Diseases and Injuries Collaborators (2020). Global burden of 369 diseases and injuries in 204 countries and territories, 1990-2019: a systematic analysis for the Global Burden of Disease Study 2019. Lancet Lond Engl. 396, 1204–1222. doi:10.1016/S0140-6736(20)30925-9
Gonzalez-Rey, E., Chorny, A., Del Moral, R. G., Varela, N., and Delgado, M. (2007). Therapeutic effect of cortistatin on experimental arthritis by downregulating inflammatory and Th1 responses. Ann. Rheum. Dis. 66, 582–588. doi:10.1136/ard.2006.062703
Gorth, D. J., Ottone, O. K., Shapiro, I. M., and Risbud, M. V. (2020). Differential effect of long-term systemic exposure of TNFα on health of the annulus fibrosus and nucleus pulposus of the intervertebral disc. J. Bone Min. Res. Off. J. Am. Soc. Bone Min. Res. 35, 725–737. doi:10.1002/jbmr.3931
Gruber, H. E., Hoelscher, G. L., Bethea, S. F., Menscher, E. A., Ingram, J. A., Templin, M. A., et al. (2014). Cortistatin is endogenous to the human intervertebral disc and exerts in vitro mitogenic effects on annulus cells and a downregulatory effect on TNF-α expression. Spine J. Off. J. North Am. Spine Soc. 14, 2995–3001. doi:10.1016/j.spinee.2014.06.002
Gu, H., Li, Q., Liu, Z., Li, Y., Liu, K., Kong, X., et al. (2024). SPP1-ITGα5/β1 accelerates calcification of nucleus pulposus cells by inhibiting mitophagy via ubiquitin-dependent PINK1/PARKIN pathway blockade. Adv. Sci. Weinh Baden-Wurtt Ger. 12, e2411162. doi:10.1002/advs.202411162
Guerrero, J., Häckel, S., Croft, A. S., Hoppe, S., Albers, C. E., and Gantenbein, B. (2021). The nucleus pulposus microenvironment in the intervertebral disc: the fountain of youth? Eur. Cell Mater 41, 707–738. doi:10.22203/eCM.v041a46
Habib, M., Hussien, S., Jeon, O., Lotz, J. C., Wu, P. I.-K., Alsberg, E., et al. (2023). Intradiscal treatment of the cartilage endplate for improving solute transport and disc nutrition. Front. Bioeng. Biotechnol. 11, 1111356. doi:10.3389/fbioe.2023.1111356
Hartvigsen, J., Hancock, M. J., Kongsted, A., Louw, Q., Ferreira, M. L., Genevay, S., et al. (2018). What low back pain is and why we need to pay attention. Lancet Lond Engl. 391, 2356–2367. doi:10.1016/S0140-6736(18)30480-X
Heo, J.-M., Ordureau, A., Paulo, J. A., Rinehart, J., and Harper, J. W. (2015). The PINK1-PARKIN mitochondrial ubiquitylation pathway drives a program of OPTN/NDP52 recruitment and TBK1 activation to promote mitophagy. Mol. Cell 60, 7–20. doi:10.1016/j.molcel.2015.08.016
Hm, N., Ja, W., and Wx, D. (2015). Mitochondrial dynamics and mitochondrial quality control. Redox Biol. 4, 6–13. doi:10.1016/j.redox.2014.11.006
Hu, B., Wang, P., Zhang, S., Liu, W., Lv, X., Shi, D., et al. (2022). HSP70 attenuates compression-induced apoptosis of nucleus pulposus cells by suppressing mitochondrial fission via upregulating the expression of SIRT3. Exp. Mol. Med. 54, 309–323. doi:10.1038/s12276-022-00745-9
Hu, L.-F., Lu, M., Wu, Z.-Y., Wong, P. T.-H., and Bian, J.-S. (2009). Hydrogen sulfide inhibits rotenone-induced apoptosis via preservation of mitochondrial function. Mol. Pharmacol. 75, 27–34. doi:10.1124/mol.108.047985
Hu, L.-F., Wong, P. T.-H., Moore, P. K., and Bian, J.-S. (2007). Hydrogen sulfide attenuates lipopolysaccharide-induced inflammation by inhibition of p38 mitogen-activated protein kinase in microglia. J. Neurochem. 100, 1121–1128. doi:10.1111/j.1471-4159.2006.04283.x
Huang, D., Peng, Y., Li, Z., Chen, S., Deng, X., Shao, Z., et al. (2020). Compression-induced senescence of nucleus pulposus cells by promoting mitophagy activation via the PINK1/PARKIN pathway. J. Cell Mol. Med. 24, 5850–5864. doi:10.1111/jcmm.15256
Huang, Y.-C., Leung, V. Y. L., Lu, W. W., and Luk, K. D. K. (2013). The effects of microenvironment in mesenchymal stem cell-based regeneration of intervertebral disc. Spine J. Off. J. North Am. Spine Soc. 13, 352–362. doi:10.1016/j.spinee.2012.12.005
Iorio, R., Petricca, S., Di Emidio, G., Falone, S., and Tatone, C. (2024). Mitochondrial Extracellular Vesicles (mitoEVs): emerging mediators of cell-to-cell communication in health, aging and age-related diseases. Ageing Res. Rev. 101, 102522. doi:10.1016/j.arr.2024.102522
Jin, S. M., Lazarou, M., Wang, C., Kane, L. A., Narendra, D. P., and Youle, R. J. (2010). Mitochondrial membrane potential regulates PINK1 import and proteolytic destabilization by PARL. J. Cell Biol. 191, 933–942. doi:10.1083/jcb.201008084
Kang, J.-W., Hong, J.-M., and Lee, S.-M. (2016). Melatonin enhances mitophagy and mitochondrial biogenesis in rats with carbon tetrachloride-induced liver fibrosis. J. Pineal Res. 60, 383–393. doi:10.1111/jpi.12319
Kang, L., Liu, S., Li, J., Tian, Y., Xue, Y., and Liu, X. (2020a). The mitochondria-targeted anti-oxidant MitoQ protects against intervertebral disc degeneration by ameliorating mitochondrial dysfunction and redox imbalance. Cell Prolif. 53, e12779. doi:10.1111/cpr.12779
Kang, L., Liu, S., Li, J., Tian, Y., Xue, Y., and Liu, X. (2020b). Parkin and Nrf2 prevent oxidative stress-induced apoptosis in intervertebral endplate chondrocytes via inducing mitophagy and anti-oxidant defenses. Life Sci. 243, 117244. doi:10.1016/j.lfs.2019.117244
Kepler, C. K., Ponnappan, R. K., Tannoury, C. A., Risbud, M. V., and Anderson, D. G. (2013). The molecular basis of intervertebral disc degeneration. Spine J. Off. J. North Am. Spine Soc. 13, 318–330. doi:10.1016/j.spinee.2012.12.003
Khaminets, A., Behl, C., and Dikic, I. (2016). Ubiquitin-dependent and independent signals in selective autophagy. Trends Cell Biol. 26, 6–16. doi:10.1016/j.tcb.2015.08.010
Killackey, S. A., Bi, Y., Philpott, D. J., Arnoult, D., and Girardin, S. E. (2023). Mitochondria-ER cooperation: NLRX1 detects mitochondrial protein import stress and promotes mitophagy through the ER protein RRBP1. Autophagy 19, 1601–1603. doi:10.1080/15548627.2022.2129763
Killackey, S. A., Bi, Y., Soares, F., Hammi, I., Winsor, N. J., Abdul-Sater, A. A., et al. (2022). Mitochondrial protein import stress regulates the LC3 lipidation step of mitophagy through NLRX1 and RRBP1. Mol. Cell 82, 2815–2831.e5. doi:10.1016/j.molcel.2022.06.004
Kirnaz, S., Capadona, C., Wong, T., Goldberg, J. L., Medary, B., Sommer, F., et al. (2022). Fundamentals of intervertebral disc degeneration. World Neurosurg. 157, 264–273. doi:10.1016/j.wneu.2021.09.066
Konig, T., Nolte, H., Aaltonen, M. J., Tatsuta, T., Krols, M., Stroh, T., et al. (2021). MIROs and DRP1 drive mitochondrial-derived vesicle biogenesis and promote quality control. Nat. Cell Biol. 23, 1271–1286. doi:10.1038/s41556-021-00798-4
K, P., E, L., and N, T. (2018). Mechanisms of mitophagy in cellular homeostasis, physiology and pathology. Nat. Cell Biol., 20. doi:10.1038/s41556-018-0176-2
Kraus, F., and Ryan, M. T. (2017). The constriction and scission machineries involved in mitochondrial fission. J. Cell Sci. 130, 2953–2960. doi:10.1242/jcs.199562
Kuivaniemi, H., and Tromp, G. (2019). Type III collagen (COL3A1): gene and protein structure, tissue distribution, and associated diseases. Gene 707, 151–171. doi:10.1016/j.gene.2019.05.003
Lai, Y.-C., Kondapalli, C., Lehneck, R., Procter, J. B., Dill, B. D., Woodroof, H. I., et al. (2015). Phosphoproteomic screening identifies Rab GTPases as novel downstream targets of PINK1. EMBO J. 34, 2840–2861. doi:10.15252/embj.201591593
Lan, T., Yan, B., Guo, W., Shen, Z., and Chen, J. (2022). VDR promotes nucleus pulposus cell mitophagy as a protective mechanism against oxidative stress injury. Free Radic. Res. 56, 316–327. doi:10.1080/10715762.2022.2094791
Lazarou, M., Sliter, D. A., Kane, L. A., Sarraf, S. A., Wang, C., Burman, J. L., et al. (2015). The ubiquitin kinase PINK1 recruits autophagy receptors to induce mitophagy. Nature 524, 309–314. doi:10.1038/nature14893
Lee, H., and Yoon, Y. (2016). Mitochondrial fission and fusion. Biochem. Soc. Trans. 44, 1725–1735. doi:10.1042/BST20160129
Lemasters, J. J. (2005). Selective mitochondrial autophagy, or mitophagy, as a targeted defense against oxidative stress, mitochondrial dysfunction, and aging. Rejuvenation Res. 8, 3–5. doi:10.1089/rej.2005.8.3
Li, M., Wu, C., Guo, H., Chu, C., Hu, M., and Zhou, C. (2019). Mangiferin improves hepatic damage-associated molecular patterns, lipid metabolic disorder and mitochondrial dysfunction in alcohol hepatitis rats. Food Funct. 10, 3514–3534. doi:10.1039/c9fo00153k
Liebsch, C., and Wilke, H.-J. (2022). Even mild intervertebral disc degeneration reduces the flexibility of the thoracic spine: an experimental study on 95 human specimens. Spine J. 22, 1913–1921. doi:10.1016/j.spinee.2022.06.010
Lim, H.-D., Kim, Y.-S., Ko, S.-H., Yoon, I.-J., Cho, S.-G., Chun, Y.-H., et al. (2012). Cytoprotective and anti-inflammatory effects of melatonin in hydrogen peroxide-stimulated CHON-001 human chondrocyte cell line and rabbit model of osteoarthritis via the SIRT1 pathway. J. Pineal Res. 53, 225–237. doi:10.1111/j.1600-079X.2012.00991.x
Lin, C., Chao, H., Li, Z., Xu, X., Liu, Y., Hou, L., et al. (2016). Melatonin attenuates traumatic brain injury-induced inflammation: a possible role for mitophagy. J. Pineal Res. 61, 177–186. doi:10.1111/jpi.12337
Lin, J., Chen, J., Zhang, Z., Xu, T., Shao, Z., Wang, X., et al. (2019a). Luteoloside inhibits IL-1β-induced apoptosis and catabolism in nucleus pulposus cells and ameliorates intervertebral disk degeneration. Front. Pharmacol. 10, 868. doi:10.3389/fphar.2019.00868
Lin, J., Zhuge, J., Zheng, X., Wu, Y., Zhang, Z., Xu, T., et al. (2020). Urolithin A-induced mitophagy suppresses apoptosis and attenuates intervertebral disc degeneration via the AMPK signaling pathway. Free Radic. Biol. Med. 150, 109–119. doi:10.1016/j.freeradbiomed.2020.02.024
Lin, Q., Li, S., Jiang, N., Shao, X., Zhang, M., Jin, H., et al. (2019b). PINK1-parkin pathway of mitophagy protects against contrast-induced acute kidney injury via decreasing mitochondrial ROS and NLRP3 inflammasome activation. Redox Biol. 26, 101254. doi:10.1016/j.redox.2019.101254
Lin, Z., Wang, H., Song, J., Xu, G., Lu, F., Ma, X., et al. (2023). The role of mitochondrial fission in intervertebral disc degeneration. Osteoarthr. Cartil. 31, 158–166. doi:10.1016/j.joca.2022.10.020
Liu, L., Feng, D., Chen, G., Chen, M., Zheng, Q., Song, P., et al. (2012). Mitochondrial outer-membrane protein FUNDC1 mediates hypoxia-induced mitophagy in mammalian cells. Nat. Cell Biol. 14, 177–185. doi:10.1038/ncb2422
Liu, X., Gong, Y., Xiong, K., Ye, Y., Xiong, Y., Zhuang, Z., et al. (2013). Melatonin mediates protective effects on inflammatory response induced by interleukin-1 beta in human mesenchymal stem cells. J. Pineal Res. 55, 14–25. doi:10.1111/jpi.12045
Lu, Y., Li, Z., Zhang, S., Zhang, T., Liu, Y., and Zhang, L. (2023). Cellular mitophagy: mechanism, roles in diseases and small molecule pharmacological regulation. Theranostics 13, 736–766. doi:10.7150/thno.79876
Madhu, V., Boneski, P. K., Silagi, E., Qiu, Y., Kurland, I., Guntur, A. R., et al. (2020). Hypoxic regulation of mitochondrial metabolism and mitophagy in nucleus pulposus cells is dependent on HIF-1α-BNIP3 Axis. J. Bone Min. Res. Off. J. Am. Soc. Bone Min. Res. 35, 1504–1524. doi:10.1002/jbmr.4019
Madhu, V., Hernandez-Meadows, M., Boneski, P. K., Qiu, Y., Guntur, A. R., Kurland, I. J., et al. (2023). The mitophagy receptor BNIP3 is critical for the regulation of metabolic homeostasis and mitochondrial function in the nucleus pulposus cells of the intervertebral disc. Autophagy 19, 1821–1843. doi:10.1080/15548627.2022.2162245
Moore, R. J. (2006). The vertebral endplate: disc degeneration, disc regeneration. Eur. Spine J. Off. Publ. Eur. Spine Soc. Eur. Spinal Deform. Soc. Eur. Sect. Cerv. Spine Res. Soc. 15 (Suppl. 3), S333–S337. doi:10.1007/s00586-006-0170-4
Narendra, D. P., Jin, S. M., Tanaka, A., Suen, D.-F., Gautier, C. A., Shen, J., et al. (2010). PINK1 is selectively stabilized on impaired mitochondria to activate parkin. PLOS Biol. 8, e1000298. doi:10.1371/journal.pbio.1000298
Nguyen, T. N., Padman, B. S., Usher, J., Oorschot, V., Ramm, G., and Lazarou, M. (2016). Atg8 family LC3/GABARAP proteins are crucial for autophagosome-lysosome fusion but not autophagosome formation during PINK1/Parkin mitophagy and starvation. J. Cell Biol. 215, 857–874. doi:10.1083/jcb.201607039
Novak, I., Kirkin, V., McEwan, D. G., Zhang, J., Wild, P., Rozenknop, A., et al. (2010). Nix is a selective autophagy receptor for mitochondrial clearance. EMBO Rep. 11, 45–51. doi:10.1038/embor.2009.256
Palikaras, K., Daskalaki, I., Markaki, M., and Tavernarakis, N. (2017). Mitophagy and age-related pathologies: development of new therapeutics by targeting mitochondrial turnover. Pharmacol. Ther. 178, 157–174. doi:10.1016/j.pharmthera.2017.04.005
Pei, M., He, F., Wei, L., and Rawson, A. (2009). Melatonin enhances cartilage matrix synthesis by porcine articular chondrocytes. J. Pineal Res. 46, 181–187. doi:10.1111/j.1600-079X.2008.00646.x
Peng, X., Zhang, C., Zhou, Z.-M., Wang, K., Gao, J.-W., Qian, Z.-Y., et al. (2022). A20 attenuates pyroptosis and apoptosis in nucleus pulposus cells via promoting mitophagy and stabilizing mitochondrial dynamics. Inflamm. Res. Off. J. Eur. Histamine Res. Soc. Al 71, 695–710. doi:10.1007/s00011-022-01570-6
Pernas, L., and Scorrano, L. (2016). Mito-morphosis: mitochondrial fusion, fission, and cristae remodeling as key mediators of cellular function. Annu. Rev. Physiol. 78, 505–531. doi:10.1146/annurev-physiol-021115-105011
Pickles, S., Vigié, P., and Youle, R. J. (2018). Mitophagy and quality control mechanisms in mitochondrial maintenance. Curr. Biol. CB 28 (28), R170–R185. doi:10.1016/j.cub.2018.01.004
Rasool, S., Veyron, S., Soya, N., Eldeeb, M. A., Lukacs, G. L., Fon, E. A., et al. (2022). Mechanism of PINK1 activation by autophosphorylation and insights into assembly on the TOM complex. Mol. Cell 82, 44–59.e6. doi:10.1016/j.molcel.2021.11.012
Richter, B., Sliter, D. A., Herhaus, L., Stolz, A., Wang, C., Beli, P., et al. (2016). Phosphorylation of OPTN by TBK1 enhances its binding to Ub chains and promotes selective autophagy of damaged mitochondria. Proc. Natl. Acad. Sci. U. S. A. 113, 4039–4044. doi:10.1073/pnas.1523926113
Riley, B. E., Lougheed, J. C., Callaway, K., Velasquez, M., Brecht, E., Nguyen, L., et al. (2013). Structure and function of Parkin E3 ubiquitin ligase reveals aspects of RING and HECT ligases. Nat. Commun. 4, 1982. doi:10.1038/ncomms2982
Risbud, M. V., Schipani, E., and Shapiro, I. M. (2010). Hypoxic regulation of nucleus pulposus cell survival: from niche to notch. Am. J. Pathol. 176, 1577–1583. doi:10.2353/ajpath.2010.090734
Risbud, M. V., Schoepflin, Z. R., Mwale, F., Kandel, R. A., Grad, S., Iatridis, J. C., et al. (2015). Defining the phenotype of young healthy nucleus pulposus cells: recommendations of the Spine Research Interest Group at the 2014 annual ORS meeting. J. Orthop. Res. Off. Publ. Orthop. Res. Soc. 33, 283–293. doi:10.1002/jor.22789
Risbud, M. V., and Shapiro, I. M. (2014). Role of cytokines in intervertebral disc degeneration: pain and disc content. Nat. Rev. Rheumatol. 10, 44–56. doi:10.1038/nrrheum.2013.160
Roughley, P. J. (2004). Biology of intervertebral disc aging and degeneration: involvement of the extracellular matrix. Spine 29, 2691–2699. doi:10.1097/01.brs.0000146101.53784.b1
Rovira-Llopis, S., Bañuls, C., Diaz-Morales, N., Hernandez-Mijares, A., Rocha, M., and Victor, V. M. (2017). Mitochondrial dynamics in type 2 diabetes: pathophysiological implications. Redox Biol. 11, 637–645. doi:10.1016/j.redox.2017.01.013
Ryu, D., Mouchiroud, L., Andreux, P. A., Katsyuba, E., Moullan, N., Nicolet-Dit-Félix, A. A., et al. (2016). Urolithin A induces mitophagy and prolongs lifespan in C. elegans and increases muscle function in rodents. Nat. Med. 22, 879–888. doi:10.1038/nm.4132
Saunders, T. L., Windley, S. P., Gervinskas, G., Balka, K. R., Rowe, C., Lane, R., et al. (2024). Exposure of the inner mitochondrial membrane triggers apoptotic mitophagy. Cell Death Differ. 31, 335–347. doi:10.1038/s41418-024-01260-2
Schweers, R. L., Zhang, J., Randall, M. S., Loyd, M. R., Li, W., Dorsey, F. C., et al. (2007). NIX is required for programmed mitochondrial clearance during reticulocyte maturation. Proc. Natl. Acad. Sci. U. S. A. 104, 19500–19505. doi:10.1073/pnas.0708818104
Sen, A., Boix, J., and Pla-Martín, D. (2023). Endosomal-dependent mitophagy coordinates mitochondrial nucleoid and mtDNA elimination. Autophagy 19, 2609–2610. doi:10.1080/15548627.2023.2170959
Silagi, E. S., Shapiro, I. M., and Risbud, M. V. (2018). Glycosaminoglycan synthesis in the nucleus pulposus: dysregulation and the pathogenesis of disc degeneration. Matrix Biol. 71–72, 368–379. doi:10.1016/j.matbio.2018.02.025
Smith, L. J., Nerurkar, N. L., Choi, K.-S., Harfe, B. D., and Elliott, D. M. (2011). Degeneration and regeneration of the intervertebral disc: lessons from development. Dis. Model Mech. 4, 31–41. doi:10.1242/dmm.006403
Song, Y., Li, S., Geng, W., Luo, R., Liu, W., Tu, J., et al. (2018). Sirtuin 3-dependent mitochondrial redox homeostasis protects against AGEs-induced intervertebral disc degeneration. Redox Biol. 19, 339–353. doi:10.1016/j.redox.2018.09.006
Song, Y., Liang, H., Li, G., Ma, L., Zhu, D., Zhang, W., et al. (2024). The NLRX1-SLC39A7 complex orchestrates mitochondrial dynamics and mitophagy to rejuvenate intervertebral disc by modulating mitochondrial Zn2+ trafficking. Autophagy 20, 809–829. doi:10.1080/15548627.2023.2274205
Song, Y., Wang, Y., Zhang, Y., Geng, W., Liu, W., Gao, Y., et al. (2017). Advanced glycation end products regulate anabolic and catabolic activities via NLRP3-inflammasome activation in human nucleus pulposus cells. J. Cell Mol. Med. 21, 1373–1387. doi:10.1111/jcmm.13067
Soubannier, V., McLelland, G.-L., Zunino, R., Braschi, E., Rippstein, P., Fon, E. A., et al. (2012). A vesicular transport pathway shuttles cargo from mitochondria to lysosomes. Curr. Biol. CB 22, 135–141. doi:10.1016/j.cub.2011.11.057
Srinivasan, S., Guha, M., Kashina, A., and Avadhani, N. G. (2017). Mitochondrial dysfunction and mitochondrial dynamics-The cancer connection. Biochim. Biophys. Acta Bioenerg. 1858, 602–614. doi:10.1016/j.bbabio.2017.01.004
Sun, F., Jiang, X., Wang, X., Bao, Y., Feng, G., Liu, H., et al. (2019). Vincristine ablation of Sirt2 induces cell apoptosis and mitophagy via Hsp70 acetylation in MDA-MB-231 cells. Biochem. Pharmacol. 162, 142–153. doi:10.1016/j.bcp.2018.10.021
Sun, K., Jing, X., Guo, J., Yao, X., and Guo, F. (2021). Mitophagy in degenerative joint diseases. Autophagy 17, 2082–2092. doi:10.1080/15548627.2020.1822097
Sun, M., Wang, S., Jiang, L., Bai, Y., Sun, X., Li, J., et al. (2018). Patulin induces autophagy-dependent apoptosis through lysosomal-mitochondrial Axis and impaired mitophagy in HepG2 cells. J. Agric. Food Chem. 66, 12376–12384. doi:10.1021/acs.jafc.8b03922
Sun, Y., Lyu, M., Lu, Q., Cheung, K., and Leung, V. (2022). Current perspectives on nucleus pulposus fibrosis in disc degeneration and repair. Int. J. Mol. Sci. 23, 6612. doi:10.3390/ijms23126612
Suzuki, S., Fujita, N., Hosogane, N., Watanabe, K., Ishii, K., Toyama, Y., et al. (2015). Excessive reactive oxygen species are therapeutic targets for intervertebral disc degeneration. Arthritis Res. Ther. 17, 316. doi:10.1186/s13075-015-0834-8
Tang, G., Han, X., Lin, Z., Qian, H., Chen, B., Zhou, C., et al. (2021). Propionibacterium acnes accelerates intervertebral disc degeneration by inducing pyroptosis of nucleus pulposus cells via the ROS-NLRP3 pathway. Oxid. Med. Cell Longev. 2021, 4657014. doi:10.1155/2021/4657014
Theodore, N. (2020). Degenerative cervical spondylosis. N. Engl. J. Med. 383, 159–168. doi:10.1056/NEJMra2003558
Towers, C. G., Wodetzki, D. K., Thorburn, J., Smith, K. R., Caino, M. C., and Thorburn, A. (2021). Mitochondrial-derived vesicles compensate for loss of LC3-mediated mitophagy. Dev. Cell 56, 2029–2042.e5. doi:10.1016/j.devcel.2021.06.003
Uoselis, L., Nguyen, T. N., and Lazarou, M. (2023). Mitochondrial degradation: mitophagy and beyond. Mol. Cell 83, 3404–3420. doi:10.1016/j.molcel.2023.08.021
Urban, J. P. G., Smith, S., and Fairbank, J. C. T. (2004). Nutrition of the intervertebral disc. Spine 29, 2700–2709. doi:10.1097/01.brs.0000146499.97948.52
Vaites, L. P., Paulo, J. A., Huttlin, E. L., and Harper, J. W. (2017). Systematic analysis of human cells lacking ATG8 proteins uncovers roles for GABARAPs and the CCZ1/Mon1 regulator C18orf8/RMC1 in macroautophagic and selective autophagic flux. Mol Cell Biol. 38, 003922–17. doi:10.1128/MCB.00392-17
Vamvakas, S.-S., Mavrogonatou, E., and Kletsas, D. (2017). Human nucleus pulposus intervertebral disc cells becoming senescent using different treatments exhibit a similar transcriptional profile of catabolic and inflammatory genes. Eur. Spine J. Off. Publ. Eur. Spine Soc. Eur. Spinal Deform. Soc. Eur. Sect. Cerv. Spine Res. Soc. 26, 2063–2071. doi:10.1007/s00586-017-5198-0
Vergroesen, P.-P. A., Kingma, I., Emanuel, K. S., Hoogendoorn, R. J. W., Welting, T. J., van Royen, B. J., et al. (2015). Mechanics and biology in intervertebral disc degeneration: a vicious circle. Osteoarthr. Cartil. 23, 1057–1070. doi:10.1016/j.joca.2015.03.028
Villa, E., Proïcs, E., Rubio-Patiño, C., Obba, S., Zunino, B., Bossowski, J. P., et al. (2017). Parkin-independent mitophagy controls chemotherapeutic response in cancer cells. Cell Rep. 20, 2846–2859. doi:10.1016/j.celrep.2017.08.087
Vlaeyen, J. W. S., Maher, C. G., Wiech, K., Van Zundert, J., Meloto, C. B., Diatchenko, L., et al. (2018). Low back pain. Nat. Rev. Dis. Primer 4, 52. doi:10.1038/s41572-018-0052-1
Walter, B. A., Korecki, C. L., Purmessur, D., Roughley, P. J., Michalek, A. J., and Iatridis, J. C. (2011). Complex loading affects intervertebral disc mechanics and biology. Osteoarthr. Cartil. 19, 1011–1018. doi:10.1016/j.joca.2011.04.005
Wang, B., Ke, W., Wang, K., Li, G., Ma, L., Lu, S., et al. (2021). Mechanosensitive ion channel Piezo1 activated by matrix stiffness regulates oxidative stress-induced senescence and apoptosis in human intervertebral disc degeneration. Oxid. Med. Cell Longev. 2021, 8884922. doi:10.1155/2021/8884922
Wang, D., Peng, P., Dudek, M., Hu, X., Xu, X., Shang, Q., et al. (2022a). Restoring the dampened expression of the core clock molecule BMAL1 protects against compression-induced intervertebral disc degeneration. Bone Res. 10, 20. doi:10.1038/s41413-022-00187-z
Wang, J., Nisar, M., Huang, C., Pan, X., Lin, D., Zheng, G., et al. (2018). Small molecule natural compound agonist of SIRT3 as a therapeutic target for the treatment of intervertebral disc degeneration. Exp. Mol. Med. 50, 1–14. doi:10.1038/s12276-018-0173-3
Wang, N., Zhu, P., Huang, R., Wang, C., Sun, L., Lan, B., et al. (2020a). PINK1: the guard of mitochondria. Life Sci. 259, 118247. doi:10.1016/j.lfs.2020.118247
Wang, P., Zhang, S., Liu, W., Chen, S., Lv, X., Hu, B., et al. (2022b). Selenium attenuates TBHP-induced apoptosis of nucleus pulposus cells by suppressing mitochondrial fission through activating nuclear factor erythroid 2-related factor 2. Oxid. Med. Cell Longev. 2022, 7531788. doi:10.1155/2022/7531788
Wang, Y., Wang, H., Zhuo, Y., Hu, Y., Zhang, Z., Ye, J., et al. (2020c). SIRT1 alleviates high-magnitude compression-induced senescence in nucleus pulposus cells via PINK1-dependent mitophagy. Aging 12, 16126–16141. doi:10.18632/aging.103587
Wang, Y., Yang, Y., Zuo, R., Wu, J., Zhang, C., Li, C., et al. (2020b). FOXO3 protects nucleus pulposus cells against apoptosis under nutrient deficiency via autophagy. Biochem. Biophys. Res. Commun. 524, 756–763. doi:10.1016/j.bbrc.2020.01.168
Westermann, B. (2010a). Mitochondrial fusion and fission in cell life and death. Nat. Rev. Mol. Cell Biol. 11, 872–884. doi:10.1038/nrm3013
Westermann, B. (2010b). Mitochondrial dynamics in model organisms: what yeasts, worms and flies have taught us about fusion and fission of mitochondria. Semin. Cell Dev. Biol. 21, 542–549. doi:10.1016/j.semcdb.2009.12.003
Wise, C. A., Sepich, D., Ushiki, A., Khanshour, A. M., Kidane, Y. H., Makki, N., et al. (2020). The cartilage matrisome in adolescent idiopathic scoliosis. Bone Res. 8, 13. doi:10.1038/s41413-020-0089-0
Wn, X., Hl, Z., Rz, Y., T, L., W, Y., Xf, Z., et al. (2019). Mitochondrial NDUFA4L2 attenuates the apoptosis of nucleus pulposus cells induced by oxidative stress via the inhibition of mitophagy. Exp. Mol. Med., 51. doi:10.1038/s12276-019-0331-2
Wong, J., Sampson, S. L., Bell-Briones, H., Ouyang, A., Lazar, A. A., Lotz, J. C., et al. (2019). Nutrient supply and nucleus pulposus cell function: effects of the transport properties of the cartilage endplate and potential implications for intradiscal biologic therapy. Osteoarthr. Cartil. 27, 956–964. doi:10.1016/j.joca.2019.01.013
Wong, Y. C., and Holzbaur, E. L. F. (2014). Optineurin is an autophagy receptor for damaged mitochondria in parkin-mediated mitophagy that is disrupted by an ALS-linked mutation. Proc. Natl. Acad. Sci. U. S. A. 111, E4439–E4448. doi:10.1073/pnas.1405752111
Wu, J., Chen, Y., Liao, Z., Liu, H., Zhang, S., Zhong, D., et al. (2022). Self-amplifying loop of NF-κB and periostin initiated by PIEZO1 accelerates mechano-induced senescence of nucleus pulposus cells and intervertebral disc degeneration. Mol. Ther. J. Am. Soc. Gene Ther. 30, 3241–3256. doi:10.1016/j.ymthe.2022.05.021
Wu, K., Shieh, J., Qin, L., and Guo, J. J. (2024). Mitochondrial mechanisms in the pathogenesis of chronic inflammatory musculoskeletal disorders. Cell Biosci. 14, 76. doi:10.1186/s13578-024-01259-9
Wu, W., Jing, D., Huang, X., Yang, W., and Shao, Z. (2021). Drp1-mediated mitochondrial fission is involved in oxidized low-density lipoprotein-induced AF cella poptosis. J. Orthop. Res. 39, 1496–1504. doi:10.1002/jor.24828
Xia, C., Zeng, Z., Fang, B., Tao, M., Gu, C., Zheng, L., et al. (2019). Mesenchymal stem cell-derived exosomes ameliorate intervertebral disc degeneration via anti-oxidant and anti-inflammatory effects. Free Radic. Biol. Med. 143, 1–15. doi:10.1016/j.freeradbiomed.2019.07.026
Xiang, Q., Zhao, Y., Lin, J., Jiang, S., and Li, W. (2022). The Nrf2 antioxidant defense system in intervertebral disc degeneration: molecular insights. Exp. Mol. Med. 54, 1067–1075. doi:10.1038/s12276-022-00829-6
Xiao, L., Hu, B., Ding, B., Zhao, Q., Liu, C., Öner, F. C., et al. (2022). N(6)-methyladenosine RNA methyltransferase like 3 inhibits extracellular matrix synthesis of endplate chondrocytes by downregulating sex-determining region Y-Box transcription factor 9 expression under tension. Osteoarthr. Cartil. 30, 613–625. doi:10.1016/j.joca.2022.01.002
Xie, L., Huang, W., Fang, Z., Ding, F., Zou, F., Ma, X., et al. (2019). CircERCC2 ameliorated intervertebral disc degeneration by regulating mitophagy and apoptosis through miR-182-5p/SIRT1 axis. Cell Death Dis. 10, 751. doi:10.1038/s41419-019-1978-2
Xin, J., Wang, Y., Zheng, Z., Wang, S., Na, S., and Zhang, S. (2022). Treatment of intervertebral disc degeneration. Orthop. Surg. 14, 1271–1280. doi:10.1111/os.13254
Xu, D., Jin, H., Wen, J., Chen, J., Chen, D., Cai, N., et al. (2017). Hydrogen sulfide protects against endoplasmic reticulum stress and mitochondrial injury in nucleus pulposus cells and ameliorates intervertebral disc degeneration. Pharmacol. Res. 117, 357–369. doi:10.1016/j.phrs.2017.01.005
Xu, H., Zhang, Y., Zhang, Y., Yu, C., Xia, K., Cheng, F., et al. (2024). A novel rat model of annulus fibrosus injury for intervertebral disc degeneration. Spine J. Off. J. North Am. Spine Soc. 24, 373–386. doi:10.1016/j.spinee.2023.09.012
Xu, W.-N., Yang, R.-Z., Zheng, H.-L., Yu, W., Zheng, X.-F., Li, B., et al. (2019a). PGC-1α acts as an mediator of Sirtuin2 to protect annulus fibrosus from apoptosis induced by oxidative stress through restraining mitophagy. Int. J. Biol. Macromol. 136, 1007–1017. doi:10.1016/j.ijbiomac.2019.06.163
Xu, X., Wang, D., Zheng, C., Gao, B., Fan, J., Cheng, P., et al. (2019b). Progerin accumulation in nucleus pulposus cells impairs mitochondrial function and induces intervertebral disc degeneration and therapeutic effects of sulforaphane. Theranostics 9, 2252–2267. doi:10.7150/thno.30658
Xu, Y., Shen, J., and Ran, Z. (2020). Emerging views of mitophagy in immunity and autoimmune diseases. Autophagy 16, 3–17. doi:10.1080/15548627.2019.1603547
Yamano, K., and Youle, R. J. (2013). PINK1 is degraded through the N-end rule pathway. Autophagy 9, 1758–1769. doi:10.4161/auto.24633
Yang, F., Liu, W., Huang, Y., Yang, S., Shao, Z., Cai, X., et al. (2022). Regulated cell death: implications for intervertebral disc degeneration and therapy. J. Orthop. Transl. 37, 163–172. doi:10.1016/j.jot.2022.10.009
Yang, M., Feng, C., Zhang, Y., Liu, C., Li, B., Zhu, Q., et al. (2019). Autophagy protects nucleus pulposus cells from cyclic mechanical tension-induced apoptosis. Int. J. Mol. Med. 44, 750–758. doi:10.3892/ijmm.2019.4212
Yang, W., Jia, C., Liu, L., Fu, Y., Wu, Y., Liu, Z., et al. (2023). Hypoxia-inducible factor-1α protects against intervertebral disc degeneration through antagonizing mitochondrial oxidative stress. Inflammation 46, 270–284. doi:10.1007/s10753-022-01732-y
Yao, X., Zhang, J., Jing, X., Ye, Y., Guo, J., Sun, K., et al. (2019). Fibroblast growth factor 18 exerts anti-osteoarthritic effects through PI3K-AKT signaling and mitochondrial fusion and fission. Pharmacol. Res. 139, 314–324. doi:10.1016/j.phrs.2018.09.026
Youle, R. J., and van der Bliek, A. M. (2012). Mitochondrial fission, fusion, and stress. Science 337, 1062–1065. doi:10.1126/science.1219855
Yu, H., Hou, G., Cao, J., Yin, Y., Zhao, Y., and Cheng, L. (2021). Mangiferin alleviates mitochondrial ROS in nucleus pulposus cells and protects against intervertebral disc degeneration via suppression of NF-κB signaling pathway. Oxid. Med. Cell Longev. 2021, 6632786. doi:10.1155/2021/6632786
Y, W., J, S., Y, C., H, L., H, Z., Z, B., et al. (2018). PINK1 protects against oxidative stress induced senescence of human nucleus pulposus cells via regulating mitophagy. Biochem. Biophys. Res. Commun., 504. doi:10.1016/j.bbrc.2018.06.031
Zhang, G.-Z., Liu, M.-Q., Chen, H.-W., Wu, Z.-L., Gao, Y.-C., Ma, Z.-J., et al. (2021). NF-κB signalling pathways in nucleus pulposus cell function and intervertebral disc degeneration. Cell Prolif. 54, e13057. doi:10.1111/cpr.13057
Zhang, Y., Yao, Y., Qiu, X., Wang, G., Hu, Z., Chen, S., et al. (2019). Listeria hijacks host mitophagy through a novel mitophagy receptor to evade killing. Nat. Immunol. 20, 433–446. doi:10.1038/s41590-019-0324-2
Zhang, Z., Xu, T., Chen, J., Shao, Z., Wang, K., Yan, Y., et al. (2018). Parkin-mediated mitophagy as a potential therapeutic target for intervertebral disc degeneration. Cell Death Dis. 9, 980. doi:10.1038/s41419-018-1024-9
Zhao, Y., Li, Y., Qu, R., Chen, X., Wang, W., Qiu, C., et al. (2019). Cortistatin binds to TNF-α receptors and protects against osteoarthritis. EBioMedicine 41, 556–570. doi:10.1016/j.ebiom.2019.02.035
Zhao, Y., Qiu, C., Wang, W., Peng, J., Cheng, X., Shangguan, Y., et al. (2020). Cortistatin protects against intervertebral disc degeneration through targeting mitochondrial ROS-dependent NLRP3 inflammasome activation. Theranostics 10, 7015–7033. doi:10.7150/thno.45359
Zhao, Y., Wang, W., Wu, X., Ma, X., Qu, R., Chen, X., et al. (2017). Mangiferin antagonizes TNF-α-mediated inflammatory reaction and protects against dermatitis in a mice model. Int. Immunopharmacol. 45, 174–179. doi:10.1016/j.intimp.2017.02.014
Zhou, B., Kreuzer, J., Kumsta, C., Wu, L., Kamer, K. J., Cedillo, L., et al. (2019). Mitochondrial permeability uncouples elevated autophagy and lifespan extension. Cell 177, 299–314. doi:10.1016/j.cell.2019.02.013
Keywords: mechanisms, therapeutic strategies, mitophagy, intervertebral disc degeneration, nucleus pulposus, annulus fibrosus, cartilage endplate
Citation: Feng C, Hu Z, Zhao M, Leng C, Li G, Yang F and Fan X (2025) Region-specific mitophagy in nucleus pulposus, annulus fibrosus, and cartilage endplate of intervertebral disc degeneration: mechanisms and therapeutic strategies. Front. Pharmacol. 16:1579507. doi: 10.3389/fphar.2025.1579507
Received: 20 February 2025; Accepted: 24 March 2025;
Published: 01 April 2025.
Edited by:
Pier Maria Fornasari, Regenhealthsolutions, ItalyReviewed by:
Yizhong Peng, Huazhong University of Science and Technology, ChinaCopyright © 2025 Feng, Hu, Zhao, Leng, Li, Yang and Fan. This is an open-access article distributed under the terms of the Creative Commons Attribution License (CC BY). The use, distribution or reproduction in other forums is permitted, provided the original author(s) and the copyright owner(s) are credited and that the original publication in this journal is cited, in accordance with accepted academic practice. No use, distribution or reproduction is permitted which does not comply with these terms.
*Correspondence: Xiaohong Fan, ZmFueGlhb2hvbmdAY2R1dGNtLmVkdS5jbg==; Fei Yang, NTU3N0BjZHV0Y20uZWR1LmNu
†ORCID: Chaoqun Feng, orcid.org/0000-0003-3210-9791; Ziang Hu, orcid.org/0009-0006-6777-6761; Min Zhao, orcid.org/0009-0002-7744-151X; Chuan Leng, orcid.org/0009-0003-2095-061X; Guangye Li, orcid.org/0009-0009-2582-611X; Fei Yang, orcid.org/0000-0003-3213-1518; Xiaohong Fan, orcid.org/0000-0002-7313-139X
‡These authors have contributed equally to this work and share first authorship
Disclaimer: All claims expressed in this article are solely those of the authors and do not necessarily represent those of their affiliated organizations, or those of the publisher, the editors and the reviewers. Any product that may be evaluated in this article or claim that may be made by its manufacturer is not guaranteed or endorsed by the publisher.
Research integrity at Frontiers
Learn more about the work of our research integrity team to safeguard the quality of each article we publish.