- 1College of Chinese Medicine, Hunan University of Chinese Medicine, Changsha, Hunan, China
- 2The Second Hospital of Hunan University of Chinese Medicine, Changsha, Hunan, China
- 3School of Informatics, Hunan University of Chinese Medicine, Changsha, Hunan, China
- 4Guangzhou University of Chinese Medicine, Guangzhou, Guangdong, China
Diabetes mellitus (DM) is a chronic metabolic disorder marked by sustained hyperglycemia. These disturbances contribute to extensive damage across various tissues and organs, giving rise to severe complications such as vision loss, kidney failure, amputations, and higher morbidity and mortality rates. Furthermore, DM imposes a substantial economic and emotional burden on patients, families, and healthcare systems. Mitophagy, a selective process that targets the clearance of damaged or dysfunctional mitochondria, is pivotal for sustaining cellular homeostasis through mitochondrial turnover and recycling. Emerging evidence indicates that dysfunctional mitophagy acts as a key pathogenic driver in the pathogenesis of DM and its associated complications. Natural small molecules are particularly attractive in this regard, offering advantages such as low toxicity, favorable pharmacokinetic profiles, excellent biocompatibility, and a broad range of biochemical activities. This review systematically evaluates the mechanistic roles of natural small molecules—including ginsenosides, resveratrol, and berberine—in enhancing mitophagy and restoring mitochondrial homeostasis via activation of core signaling pathways (e.g., PINK1/Parkin, BNIP3/NIX, and FUNDC1). These pathways collectively ameliorate pathological hallmarks of DM, such as oxidative stress, chronic inflammation, and insulin resistance. Furthermore, the integration of nanotechnology with these compounds optimizes their bioavailability and tissue-specific targeting, thereby establishing a transformative therapeutic platform for DM management. Current evidence demonstrates that mitophagy modulation by natural small molecules not only offers novel therapeutic strategies for DM and its chronic complications but also advances the mechanistic foundation for future drug development targeting metabolic disorders.
1 Introduction
Diabetes mellitus (DM) is a chronic condition featured with persistently elevated blood glucose levels, which disrupt normal metabolic processes and lead to various systemic complications (Yu et al., 2024). In the light of the Global Burden of Disease study published in The Lancet, an estimated 828 million adults (aged 18 years and older) globally were living with DM in 2022, with an age-standardized prevalence rate of 13.9% in women and 14.3% in men (Zhou et al., 2024). Data indicate that the global DM epidemic is worsening, with increasing prevalence in younger populations over time (Stene, 2024). DM and its complications impose significant financial and psychological burdens on individuals, families, and society (The Lancet Diabetes and Endocrinology, 2024). Hyperglycemia, along with long-term metabolic disturbances, exacerbates disease progression, leading to widespread tissue and organ damage and contributing to severe complications. Despite the availability of various preventive and therapeutic interventions, the complex and multifactorial nature of DM makes effective treatment challenging. Thus, a deeper understanding of its pathogenesis is crucial for developing targeted therapeutic strategies to manage DM and its complications. Recent research has underscored the critical involvement of mitophagy in the pathophysiology (Ajoolabady et al., 2022; Ke, 2020; Wang et al., 2023a). Mitophagy, a selective process that targets the clearance of damaged or dysfunctional mitochondria, is pivotal for sustaining cellular homeostasis through mitochondrial turnover and recycling (Picca et al., 2023). Emerging evidence suggests that the proper modulation of mitophagy is critical in ensuring metabolic balance in DM, and its dysfunction may be a contributing factor to the onset and progression of associated complications (Yang et al., 2024a; Zhu et al., 2024; Sriwastawa and Kumar, 2024), positioning it as a promising biomarker and potential therapeutic target. Natural small molecules, a class of bioactive compounds with low molecular weight, are derived from plants, animals, microorganisms, or synthetic methods. These molecules are known for their low toxicity, high druggability, excellent biocompatibility, and diverse biochemical activities (Clardy and Walsh, 2004; Ortholand and Ganesan, 2004). They have demonstrated considerable effectiveness in preventing and mitigating the complications of DM. For example, natural small molecules such as ginsenosides and resveratrol have been shown to ameliorate pathological hallmarks of diabetes (e.g., oxidative stress and insulin resistance) by modulating mitophagy pathways (Li et al., 2023a; Wang et al., 2018a). This review offers a comprehensive analysis of the significance of mitophagy and its related pathways in DM and its complications. It highlights the current advancements in the study of natural small molecules that orchestrate mitophagy to address DM and its associated complications. The findings presented in this review lay a solid groundwork for future investigations and the formulation of innovative therapeutic approaches aimed at combating mitochondrial dysfunction in metabolic disorders.
2 Regulatory pathways of mitophagy
Mitophagy, or mitophagy, was first defined by Lemasters in 2005 (Lemasters, 2005) as a highly regulated process that selectively eliminates damaged or dysfunctional mitochondria to maintain cellular homeostasis. This process involves a series of well-coordinated steps, each crucial for the efficient clearance of defective mitochondria (Palikaras et al., 2018). Mitophagy involves four key steps: first, damaged mitochondria undergo depolarization, leading to a loss of mitochondrial membrane potential (MMP). Second, the damaged mitochondria are then enveloped by autophagosomes, forming mitochondrial autophagosomes. Third, the mitochondrial autophagosomes subsequently fuse with lysosomes, where their contents are degraded. Finally, the lysosome degrades the mitochondrial components, completing the process of mitochondrial quality control (MQC) (Lyamzaev et al., 2018; Samuvel et al., 2022; Miyamoto et al., 2011). Mitophagy serves as a quality control mechanism, ensuring that only healthy, functional mitochondria remain in the cellular network. By selectively eliminating damaged mitochondria, it helps maintain mitochondrial dynamics and cellular function. A summary of these key steps is depicted in Figure 1.
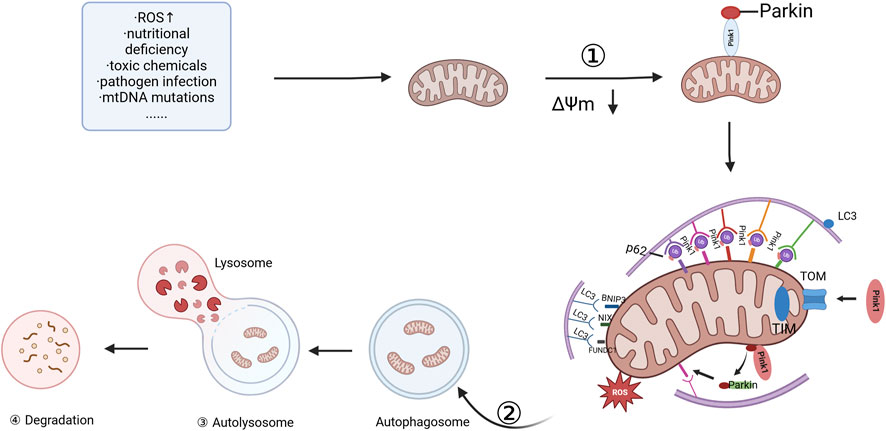
Figure 1. Committed step of mitophagy. ① depolarization of damaged mitochondria; ② wrapping of mitochondria by autophagosomes; ③ fusion of mitochondrial autophagosomes with lysosomes; and ④ mitochondrial contents are degraded by lysosomes, and lysosomal or vesicular acid hydrolases flow into autophagosomes to degrade damaged mitochondria. The figure is drawn with biorender.com.
Mitophagy, a process essential for cellular homeostasis, can be classified into two main pathways: the ubiquitin- and the non-ubiquitin-dependent pathways (Palikaras et al., 2018; Khaminets et al., 2016).
The ubiquitin-dependent pathway involves the tagging of mitochondrial surface proteins with ubiquitin, facilitating their recognition and subsequent degradation (Fritsch et al., 2020). This pathway encompasses both the well-known PINK1-Parkin-mediated mitophagy and alternative, Parkin-independent mechanisms. The PINK1/Parkin axis is the most extensively researched and thoroughly understood mechanism responsible for the targeted removal of dysfunctional mitochondria in mammalian cells (Imberechts et al., 2022; Liu et al., 2023a; Li et al., 2023b). PINK1 is a highly conserved mitochondrial protein that contains a kinase domain and mitochondrial localization sequences, while Parkin is an E3 ubiquitin ligase found in the cytosol. Parkin is responsible for recognizing and ubiquitinating target proteins on damaged mitochondria (Han et al., 2023). The sequence of events in PINK1/Parkin-mediated mitophagy begins when mitochondria become damaged. A loss of MMP prevents PINK1 from entering the inner mitochondrial membrane, causing it to accumulate on the outer membrane. This accumulation acts as a signal, recruiting Parkin from the cytosol to the damaged mitochondria. Upon recruitment, Parkin undergoes a conformational change, activating Its E3 ligase activity. This activation results in the ubiquitination of outer mitochondrial membrane proteins, leading to the formation of protein aggregates that are then recognized by the autophagic receptor protein P62 (Riley et al., 2013). The P62 protein interacts with LC3, facilitating the production of autophagic lysosomes that degrade the damaged mitochondria. This process is highly coordinated, with PINK1 and Parkin working together to orchestrate MQC and ensure mitochondrial homeostasis (Whitworth and Pallanck, 2009). In addition to the canonical PINK1/Parkin axis, there is increasing evidence of a Parkin-independent, ubiquitin-dependent pathway. This pathway implicates other E3 ligases such as MUL1, Gp78, SMURF1, ARIH1, SIAH1, and RNF185 (Igarashi et al., 2020; Liu et al., 2012; Zhang et al., 2022a; Mukherjee and Chakrabarti, 2016). These autophagy receptors bind to the ubiquitinated proteins and initiate mitophagy in a Parkin-independent fashion (Lu et al., 2023).
The non-ubiquitin-dependent pathway involves several receptor proteins that mediate mitophagy and are associated with mitochondrial dynamics. These receptors are classified into two groups: one group contains ubiquitin-binding domains, such as p62, OPTN, and NDP52, while the other group lacks these domains, including C-cbl, NCOA4, BNIP3L/NIX, FUNDC1, STBD1, PHB2, etc (Liu et al., 2012; Xie et al., 2020; Bellot et al., 2009). These receptors facilitate mitophagy by directly binding to LC3, without the need for ubiquitination. Mitochondrial dynamics is critical in ensuring mitochondrial mass through fission and fusion processes. When this balance is disrupted, damaged mitochondria are selectively removed via mitophagy, while healthy mitochondria are reintegrated into the network through fusion. This process, referred to as mitochondrial dynamics-mediated mitophagy, is critical for ensuring cellular energy supply and function (Shirihai et al., 2015; Lacombe and Scorrano, 2024; Xiao et al., 2022). Several pathways are involved in regulating mitophagy. For example, the FOXO1/PINK1 pathway enhances the expression of mitophagy-related proteins such as LC3II, PINK1, and Parkin, while reducing the expression of senescence-related genes (e.g., Rb, p16, p21). This pathway improves insulin sensitivity, fasting insulin levels, and fasting glucose levels in DM mice. As a mitochondrial outer membrane receptor, FUNDC1 orchestrates hypoxia-induced mitophagy through LC3 interaction via its LIR (LC3-interacting region) domain, serving as a critical regulatory node in stress-responsive mitochondrial quality control (Liu et al., 2012). The FUNDC1 pathway is activated under hypoxic conditions, where FUNDC1 binds to LC3, promoting the association of mitochondria with autophagosomes and accelerating mitophagy (Dong et al., 2022). Additionally, the serine/threonine kinase ULK1 phosphorylates FUNDC1, which is critical for the targeting of FUNDC1 to damaged mitochondria during mitophagy (Zhu et al., 2022). FUNDC1, a hypoxia-inducible mitochondrial outer membrane receptor, mediates stress-responsive mitophagy by recruiting ULK1 to phosphorylate Ser17, thereby initiating autophagosome formation under conditions of oxygen deprivation or mitochondrial membrane depolarization (Wu et al., 2014). Concurrently, the AMPK-mTORC1 axis serves as a central energy-sensing regulator of this process: under nutrient-replete conditions, mTORC1 phosphorylates ULK1 at Ser757 to suppress mitophagy by disrupting its interaction with AMPK (Saxton and Sabatini, 2017); conversely, energy depletion (e.g., hypoxia-induced ATP decline) activates AMPK through elevated AMP/ATP ratios, which concurrently phosphorylates ULK1 at Ser317/Ser777 to trigger autophagosome assembly and inactivates mTORC1 via TSC2-Rheb signaling, thereby relieving ULK1 inhibition (Dengler, 2020; Kim et al., 2013; Inoki et al., 2003). Complementing these pathways, the NAD ± dependent deacetylase SIRT1 enhances mitophagy through dual mechanisms—direct deacetylation of LC3 to facilitate autophagosomal membrane expansion (Huang et al., 2015) and activation of PGC1α, a transcriptional coactivator that upregulates mitochondrial biogenesis genes (e.g., TFAM, NRF1) while synergistically promoting mitochondrial turnover (Hwang and Song, 2017; Cantó et al., 2010; Jäger et al., 2007). Nicotinamide (NAM) amplifies this cascade by stabilizing cellular NAD + pools, thereby potentiating SIRT1 activity and linking metabolic sensing to mitochondrial quality control (Hwang and Song, 2017). The BNIP3 and BNIP3L/NIX pathways are also critical in mitochondrial clearance. These proteins interact with LC3 to promote the removal of dysfunctional mitochondria and maintain mitochondrial homeostasis. Their function is modulated by the formation of homo- and heterodimers, which are necessary for ensuring mitochondrial integrity (Hendgen-Cotta et al., 2017). BNIP3, a pro-apoptotic Bcl-2 family member sharing BH3 domain homology, functions as a hypoxia-inducible mitophagy receptor. Under hypoxic stress, BNIP3 undergoes transcriptional upregulation and translocates to the mitochondrial outer membrane via its C-terminal transmembrane domain. Structural stabilization occurs through homodimerization, while its N-terminal LC3-interacting region (LIR) motifs engage LC3 through phosphorylation-dependent mechanisms at Ser17 and Ser24 residues to drive mitophagosome formation (Hanna et al., 2012; Zhu et al., 2013). NIX (BNIP3L), a mitochondrial outer membrane protein exhibiting 56% sequence homology with BNIP3, orchestrates selective mitochondrial degradation during reticulocyte maturation. This process requires phosphorylation at Ser34/Ser35 to enhance LIR-mediated binding to LC3A/B isoforms, coupled with redox-sensitive ROS accumulation and Rheb GTPase signaling (Rogov et al., 2017; Marinković et al., 2021). Notably, BNIP3 cross-talks with mTORC1 by sequestering Rheb, thereby alleviating mTORC1-mediated suppression of ULK1 kinase activity to potentiate mitophagy (Li et al., 2007; Melser et al., 2013). Both receptors interface with the PINK1/Parkin pathway: NIX undergoes Parkin-dependent ubiquitination to amplify damaged mitochondrial recognition, while BNIP3 facilitates PINK1 stabilization, promoting Parkin recruitment to depolarized mitochondria for proteasomal targeting (Gao et al., 2015; Zhang et al., 2016). Furthermore, BNIP3 and BNIP3L/NIX interact with mitochondrial phagocytosis protein Mieap and CDH6, facilitating the elimination of reactive oxygen species (ROS) and modulating Drp1-mediated mitochondrial fission (Nakamura et al., 2012). Recent studies have also identified new therapeutic targets, such as hexokinase-2, small ribosomal subunit protein US3, and L-lactate dehydrogenase A, which have demonstrated crucial roles in mitophagy in diabetic ulcers (Guo et al., 2024).
In summary, the regulation of mitophagy is a complex process governed by a network of physiological mechanisms, encompassing both ubiquitin- and non-ubiquitin-dependent pathways. These intricate pathways are vital in preserving MQC and ensuring optimal cellular functioning.
3 The role of mitophagy in DM and its chronic complications
Mitophagy dysfunction has been linked to the pathogenesis of numerous clinical conditions, with both excessive and insufficient autophagy identified as key factors in the onset and progression of various diseases. In the context of DM, recent research has highlighted the significance of mitophagy, suggesting that its regulation could offer potential therapeutic benefits. Specifically, moderate mitophagy is capable of alleviating symptoms of DM and its complications, while either underactive or overly active autophagy aggravates disease. Hyperglycemia-driven mitophagy impairment in diabetic pathogenesis and complications: Synergistic roles of oxidative and endoplasmic reticulum stress are illustrated in Figure 2.
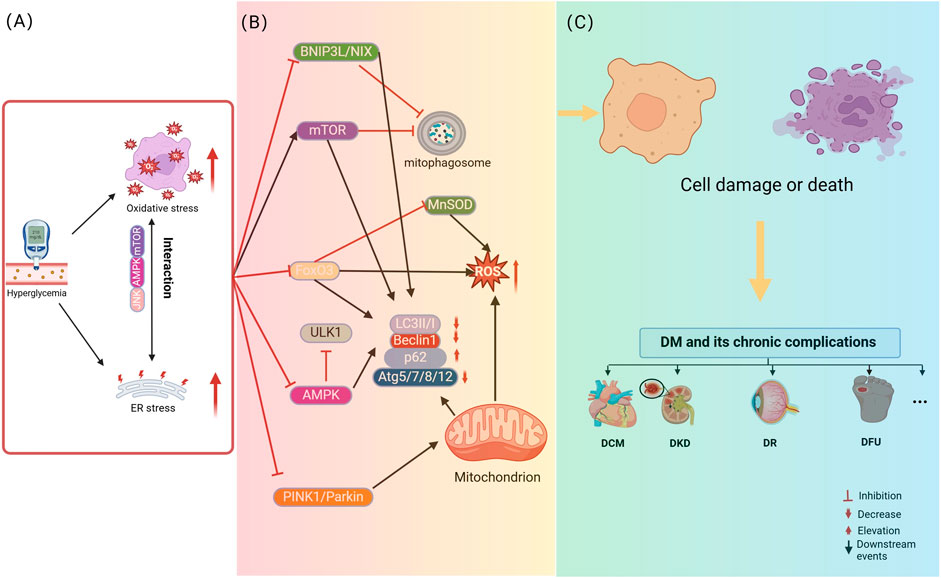
Figure 2. Hyperglycemia-driven mitophagy impairment in diabetic pathogenesis and complications: Synergistic roles of oxidative and endoplasmic reticulum stress. (A) describes that hyperglycemia initiates the process of cellular damage by inducing oxidative stress and ER stress, where oxidative stress and ER stress interact with each other through signaling pathways, such as JNK, to form a vicious cycle. (B) Revealed that hyperglycemia, oxidative stress and ER stress further lead to the disruption of mitochondria-related pathways, including mitophagy dysfunction. (C) Elucidated the consequences of mitophagy dysfunction, i.e., damaged mitochondria cannot be effectively removed, leading to cell damage or death, which ultimately contributes to the development of diabetes and its chronic complications. The figure is drawn with biorender.com.
Type 1 diabetes mellitus (T1DM) is a T cell-mediated autoimmune disease, and the pathogenesis is closely linked to mitophagy and mitochondrial function (Blagov et al., 2023). During the onset of T1DM, the immune system attacks pancreatic β-cells, and a variety of immune cells infiltrate, releasing pro-inflammatory cytokines, which triggers pancreatic islet inflammation, and promotes apoptosis of β-cells (Christianson et al., 1993). Abnormal mitochondrial function is also critical. Damaged mitochondria release mtROS and mtDNA. mtROS cause macrophages to activate and secrete pro-inflammatory factors, and mtDNA activates NLRP3 inflammasome, which drives inflammation and damage β cells (Carlos et al., 2017). Mitophagy is significant in T1DM. It maintains mitochondrial homeostasis in normal conditions, but its function is impaired in T1DM, resulting in the accumulation of damaged mitochondria, which activate inflammatory signaling pathways and trigger chronic inflammation and autoimmune responses (Mills et al., 2017). Compounds such as PMI and Urolithins can induce mitophagy, bringing new possibilities for the treatment of T1DM (East et al., 2014).
Gestational diabetes mellitus (GDM)is a diabetes mellitus that occurs during pregnancy and is closely related to insulin resistance and β-cell dysfunction. It has been shown that GDM leads to mitochondrial dysfunction in placental endothelial and trophoblast cells, especially reduced mitochondrial F0F1-ATP synthase (complex V) activity, which reduces placental oxidative phosphorylation capacity. This phenomenon may be related to the overproduction of superoxide anion and nitric oxide. In addition, the synergistic action of placental mitochondrial biosynthesis and mitophagy is essential for the maintenance of cellular homeostasis, especially during pregnancy, to ensure the efficient production of ATP required for fetal growth and development (Sobrevia et al., 2020).
In DM, defects in pancreatic β-cells or the development of insulin resistance (IR) impair insulin secretion, which ultimately results in persistent hyperglycemia (Jia et al., 2021). Previous studies have documented (Park et al., 2023; Čater and Križančić Bombek, 2022) a close link between mitochondrial dysfunction and IR. Damaged mitochondria accumulate within cells, and increased mitochondrial ROS production further exacerbates oxidative damage and glucose intolerance in β-cells. This oxidative stress (OS) contributes to β-cell apoptosis, worsening IR and creating a cycle that accelerates disease progression. Mitochondria also modulate insulin secretion via the modulation of ATP synthase activity and ATP production, both of which are crucial for normal β-cell function. Growing evidence points to mitophagy as a key regulator of mitochondrial health under hyperglycemic conditions, potentially playing a critical role in mitigating oxidative damage by removing dysfunctional mitochondria and excess ROS (Shan et al., 2022; Shim et al., 2023). For example, Kaniuka et al. (2025) demonstrated that glucotoxicity-induced elevation of GRP78 led to depletion of antioxidant reserves and impaired antioxidant defense mechanisms, which in turn promoted mitophagy in pancreatic cells, helping to mitigate the effects of DM in DM rats. Similarly, Oh et al. (2023) showed that mice with β-cell-specific deletion of Tfeb (TfebΔ β-cell) exhibited β-cell dysfunction and glucose intolerance following exposure to a calmodulin phosphatase inhibitor, which hindered TFEB activation and mitophagy. These findings further pinpoint the critical role of mitophagy in β-cell function. Overall, it is evident that mitochondrial dynamics and autophagy are impaired in DM, leading to dysregulated mitochondrial function and increased IR. Restoring proper mitophagy and dynamics could, therefore, offer a promising therapeutic avenue for addressing DM and its associated complications.
3.1 Microangiopathy
3.1.1 Diabetic kidney disease
DKD is a significant complication of DM and a primary contributor to end-stage renal disease (Ahmad et al., 2021). Hyperglycemia-induced mitochondrial dysfunction has been documented as a key factor driving renal tubular epithelial cell (EC) damage in DKD. Liu et al. (2024) demonstrated that renal tubulointerstitial inflammation serves as a critical predictor for the progression of DKD. Their study revealed a marked increase in TRPC6 levels in DKD, which is pivotal in inhibiting mitophagy through the activation of calcineurin-1. Silencing Trpc6 helped partially restore mitophagy, thereby reducing both renal tubular injury (RTI) and tubulointerstitial inflammation in experimental models. In a separate study, Ji et al. (2024) showed that overexpression of UHRF1 promoted PINK1-mediated mitophagy by diminishing TXNIP expression, which in turn suppressed ferroptosis. These findings uncover that elevated UHRF1 can positively influence mitophagy and help alleviate DKD. Xiao et al. (2017) reported that Nrf2-mediated transcriptional regulation of PINK1 restored mitophagy in renal tubular cells, reducing mitochondrial OS and restoring mitochondrial dynamics. This ultimately alleviated RTI. Furthermore, Yang et al. (2024) reported that WJ-39 treatment in rats with DKD preserved mitochondrial integrity in renal tubules by inhibiting aldose reductase, activating the PINK1/Parkin axis, and promoting mitophagy. This intervention effectively alleviated mitochondrial apoptosis. These findings unveil the significance of mitophagy in DKD. Mitigating RTI by reducing mitochondrial OS in hyperglycemic conditions presents a promising strategy for the therapeutic intervention in DKD.
3.1.2 Diabetic retinopathy
DR is a severe complication of DM and remains the leading cause of blindness on a global scale (Sun et al., 2022). Numerous studies have suggested (Liu et al., 2023b; Wang et al., 2023b) that mitochondrial dysfunction is critical in OS, inflammation, and apoptosis observed in the retina of DR patients. Mitophagy has emerged as a key area of study, as it appears to play a dual role in DR, with both beneficial and deleterious effects. Zhang et al. (2022b) found that TGR5 ameliorated vascular endothelial cell (VEC) dysfunction in DR by inhibiting mitochondrial fission through the regulation of the PKCδ/Drp1-HK2 axis, while also enhancing mitophagy in RMECs. Similarly, Yang et al. (2024c) reported that overexpression of Sirt3 elevated p-AMPK/AMPK and p-ULK1/ULK1, while decreasing p-mTOR/mTOR expression. This regulatory effect inhibited apoptosis and promoted mitophagy, which protected RPE cells from high-glucose (HG)-induced injury. Furthermore, Serikbaeva et al. (2022) found that HG levels promoted mitophagy, which in turn helped attenuate HG-induced injury in DR. Additionally, Zhang et al. (2024) observed that knockdown of TIN2 distinctly declined cellular senescence and mitochondrial OS in ARPE-19 cells under HG conditions. This intervention restored retinal thickness and RPE cell tight junctions in DR mice. The increased mitochondrial localization of TIN2 induced cellular senescence in RPE cells by impairing mitophagy and activating mTOR signaling. The harmful effects of mitophagy in DR were further explored by Cai et al. (2017), who investigated OS-induced apoptosis and autophagy in the retinas of T2DM rats induced by a HG, high-fat diet (HFD) and a single injection of streptozotocin (STZ). Their findings showed increased levels of LC3-II/I, along with enhanced OS enzyme activities such as reductive nicotinamide adenine dinucleotide phosphoryl oxidase 3 and superoxide dismutase 2. The increased autophagy activity inhibited apoptosis and OS, providing evidence that modulating autophagy levels could mitigate the progression of DR. Additional studies support the complex role of mitophagy in DR pathogenesis. For instance, Wu et al. (2022a) demonstrated that knockdown of Drp1 inhibited retinal endothelial cell apoptosis in rats by suppressing mitophagy. Li et al. (2019a) showed that inducing mitophagy promoted tube formation and migration of choroid-retinal endothelial cells in monkeys, suggesting a role for mitophagy in enhancing angiogenesis. In summary, mitophagy plays a complex, dual role in DR.
3.1.3 Diabetic cardiomyopathy
DCM is a severe complication of DM, characterized by systolic dysfunction and left ventricular hypertrophy, leading to significant impairment in cardiac performance (Lu et al., 2021). Mitophagy is recognized as a protective mechanism in DCM, as it helps to eliminate damaged mitochondria, alleviates OS, and reduces cardiomyocyte apoptosis (Bai et al., 2020). A study by Mu et al. (2020) explored the role of BRD4 in DCM, showing that elevation of BRD4 in the hearts of DM mice repressed PINK1/Parkin-mediated mitophagy. This inhibition resulted in the generation of damaged mitochondria, which, in turn, led to structural and functional deterioration of the heart. In contrast, the use of JQ1, a BRD4 inhibitor, was found to enhance mitochondrial function and restore the structural and functional integrity of the diabetic heart. Notably, Pink1 deficiency was found to exacerbate DCM by inhibiting mitophagy, highlighting the importance of mitophagy in mitigating myocardial damage. These findings suggest that reduced mitophagy in the diabetic myocardium contributes to myocardial injury, and restoring mitophagy could help alleviate cardiomyocyte damage in DM-related heart disease.
3.2 Atherosclerotic cardiovascular disease
Atherosclerosis is notably prevalent among individuals with DM and tends to progress at an accelerated rate, significantly heightening the risk of cardiovascular and cerebrovascular diseases, which remain leading causes of mortality in DM patients. Atherosclerosis primarily impacts major arteries such as the aortic, coronary, cerebral, renal, and limb arteries, contributing to conditions such as coronary artery disease, ischemic or hemorrhagic stroke, renal arteriosclerosis, and peripheral artery disease. A key mechanism driving the progression of ASCVD is endothelial cell injury, with hyperglycemia playing a critical role in inducing endothelial dysfunction. Mitophagy has emerged as a protective mechanism against hyperglycemia-induced damage in endothelial cells. A previous study (Zhu et al., 2018) demonstrated that PINK1/Parkin-mediated mitophagy alleviated mitochondrial dysfunction in DM rats, thus protecting endothelial cells from hyperglycemia-induced damage. Similarly, Liu et al. (2017a) found that exogenous hydrogen sulfide (H2S) protected rat aortic endothelial cells (RAECs) from HG and palmitate-induced injury by repressing OS, reducing mitochondrial fragmentation, and promoting mitophagy. This intervention helped prevent RAEC apoptosis under HG and palmitate conditions. These results suggest that impaired mitophagy in diabetic endothelial cells exacerbates cellular damage. Therefore, restoring mitophagy in these cells may provide a novel therapeutic approach to mitigate endothelial cell injury in DM and reduce the risk of ASCVDs.
3.3 Neurological complications
3.3.1 Central nervous system complications
CNS complications in DM include ➀ altered mental status, typically associated with severe diabetic ketoacidosis (DKA), hyperosmolar hyperglycemic syndrome, or hypoglycemia; ➁ stroke; and ➂ accelerated brain aging, including Alzheimer’s disease (AD). Mitophagy is crucial in mitigating CNS complications associated with AD and T2DM (Paul et al., 2021). Mitophagy is considered one of the primary mechanisms that alleviates CNS dysfunction in these conditions. For instance, in DM-related stroke, neurodegeneration may occur due to severe ischemia and hypoxia in the ischemic core of the brain (Szydlowska and Tymianski, 2010). Reestablishment of cerebral blood flow after acute stroke treatment, although essential, can lead to ischemia/reperfusion (I/R) injury, which exacerbates neuronal damage (Leech et al., 2019). Excessive mitochondrial dysfunction due to I/R injury is a major contributor to neuronal apoptosis, which is a key cause of cell death in stroke (Wang et al., 2022a; Nhu et al., 2021). As documented by previous studies, mitophagy is activated following brain I/R injury, helping to mitigate this damage (Lan et al., 2018; Cai et al., 2021). For example, Yaqi Guo et al. demonstrated that metformin alleviated hyperglycemia-exacerbated brain I/R injury by activating the AMPK/ULK1/PINK1/Parkin mitophagy axis (Guo et al., 2023).
3.3.2 Diabetic peripheral neuropathy
DPN is a prevalent complication of chronic DM, resulting from impaired blood flow to peripheral nerves, which leads to neuronal dysfunction. This dysfunction manifests through deficits in nerve conduction and other neuropathic symptoms (Khan et al., 2023). Mitophagy plays a significant role in ameliorating DPN. Zheng et al. (2024) found that hyperbaric lidocaine (HL) induced PINK1-mediated mitophagy via the activation of the p38 MAPK/ERK axis, which exacerbated spinal cord tissue injury in DNP rats. Yang et al. (2024d) demonstrated that overexpression of SIRT3 induced mitophagy via activation of the FoxO3a-PINK1-Parkin axis, thereby improving DPN. Furthermore, Yuan et al. (2022a) identified that defects in mitophagy, induced by PARP1 in dorsal root ganglion neurons, were a critical mechanism underlying DM-induced peripheral nerve damage. In a related study, Khan et al. (2024) validated that silibinin elevated the expression of PARL, PINK1, PGAM5, and LC3, ensuring mitophagy through various mechanisms. Additionally, silibinin promoted antioxidant defense by upregulating Nrf2, and enhanced the expression of SIRT1, PGC-1α, and TFAM in sciatic nerves and HG-injured N2A cells. Collectively, these findings pinpoint the significance of mitophagy in the pathogenesis of DPN, suggesting that targeted modulation of mitochondrial autophagic processes could offer a promising therapeutic modality for treating this condition.
3.4 Diabetic foot ulcer
DFU is a severe complication in DM, with many patients at risk of amputation (Jeffcoate et al., 2018). DFUs primarily result from the interplay of multiple factors, including neuropathy, impaired vascularization, and biomechanical abnormalities. The incidence of DFU has been steadily increasing over the years (Armstrong et al., 2023). Clinically, DFU repair progresses through four distinct phases: coagulation, inflammation, proliferation, and remodeling (Liang et al., 2021; Ramachandran et al., 2023). In advanced DM, the generation of advanced glycation end-products and mitophagy dysfunction contribute to pathological changes, such as an imbalanced inflammatory response, elevated OS, impaired VEC regeneration, and compromised fibroblast repair (Magalhaes-Novais et al., 2022; Chen et al., 2022a). During the inflammatory phase, it is beneficial to reduce inflammatory factors by upregulating basal mitophagy, which can mitigate inflammation. Onishi et al. (2021) demonstrated that dysfunction in mitophagy leads to the generation of damaged mitochondria, resulting in the release of mitochondrial ROS and DNA. This triggers excessive activation of the NLRP3 inflammasome, thereby increasing the creation of pro-inflammatory cytokines (PICs) like IL-1β/18. Additionally, mitophagy orchestrates mitochondrial metabolism, influencing the differentiation of immune cells. M2 macrophages, which rely on oxidative phosphorylation for their biosynthetic needs, are inhibited in their transformation under dysfunctional mitophagy, leading to a predominance of M1 macrophages. This shift enhances the inflammatory response (Xu et al., 2020; Peng et al., 2021). In the proliferative phase, moderately regulating mitophagy, often in response to local hypoxia at the wound site, promotes the repair process by alleviating apoptosis, facilitating collagen synthesis, and encouraging neovascularization. Xiang et al. (2022) reported that HG intervention brought about a notable reduction in the expression of mitophagy proteins (Beclin1, PINK1/Parkin, and LC3-II/I) in VECs. This resulted in mitochondrial dysfunction, increased endothelial cell apoptosis, and decreased cell migration and activity. During the remodeling phase, excessive autophagy can lead to mitochondrial loss and increased cellular energy consumption, which may exacerbate fibroblast apoptosis and hinder scar formation in diabetic skin ulcers (Liu et al., 2019a). Yinji Luo et al. found that (Luo et al., 2024) HG exposure reduced cell viability in DFU rats, with elevated ROS, SA-β-gal, p21, p62 proteins, but decreased LC3II/I levels. HG inhibited mitophagy by accelerating dermal fibroblast senescence via the inhibition of the PINK1/Parkin axis. However, the administration of ASC-EVs facilitated mitophagy via activating the PINK1/Parkin axis in vivo, thereby improving DFU pathology. Conclusively, mitophagy is intricately involved in various stages of DFU development. Therefore, regulating mitophagy could be a promising strategy for improving DFU outcomes.
3.5 Other categories
DM can lead to various ocular complications, including cataracts, glaucoma, and iridocyclitis. Mitophagy is critical in the degeneration of ocular tissues (Brooks et al., 2023). It has been demonstrated that a hyperglycemic environment can reduce ATP synthesis, decrease MMP, and alter mitochondrial autophagic flux in lens ECs (LECs), ultimately resulting in LEC apoptosis (Xu et al., 2018; Liu et al., 2020). Aldrich et al. (2017) observed that mitophagy was impaired in human corneal endothelial cells from DM patients. The mitochondria in these cells appeared swollen and contained dark inclusions, suggesting a disruption of mitophagy. Furthermore, Hu et al. (2019) reported that HG diminished the expression of Sirt3 in TKE2 mouse corneal ECs, which in turn reduced Parkin/PINK1-mediated mitochondrial autophagic flux. However, overexpression of Sirt3 activated the Parkin/PINK1 axis, enhancing mitophagy and promoting corneal epithelial wound healing. These findings highlight that modulation of mitophagy could serve as an effective therapeutic strategy to mitigate ocular complications in DM patients.
4 Natural small molecules intervene in chronic complications of DM by modulating autophagy
A growing body of research has demonstrated that natural small molecules can orchestrate mitophagy to effectively treat DM and its chronic complications. Below is an overview of how these natural compounds impact mitophagy in DM and its associated complications. Natural small molecules regulate diabetes and chronic complications by activating mitophagy are illustrated in Figure 3.
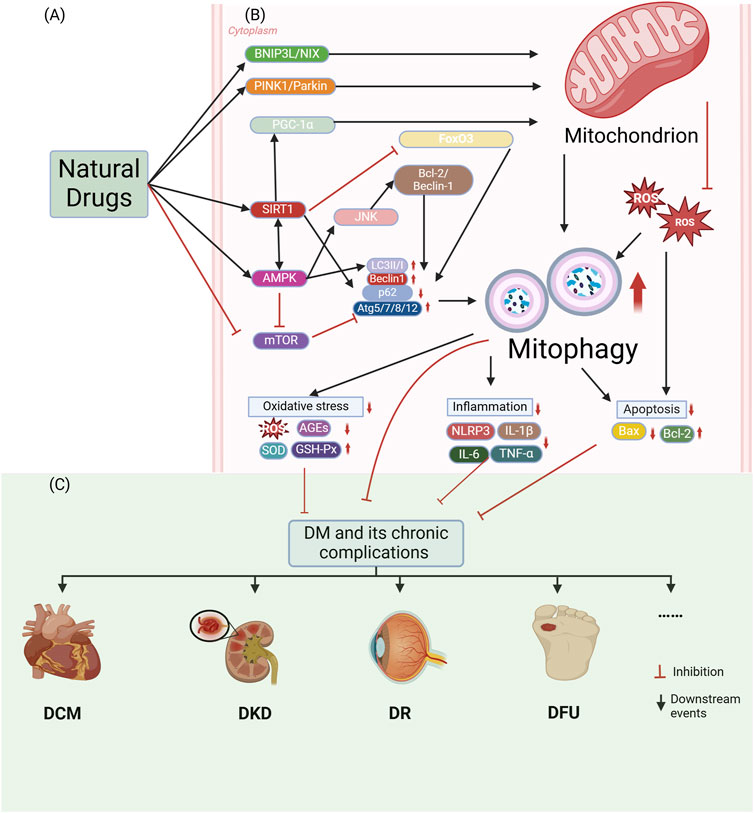
Figure 3. Natural small molecules modulate diabetes and chronic complications through activation of mitophagy. (A) Natural small molecules function as therapeutic interventions targeting mitochondrial homeostasis. (B) Molecular mechanisms of mitophagy involve: Core regulators: PINK1/Parkin signaling, BNIP3L/NIX-mediated mitochondrial recognition, LC3II/I lipidation, Beclin1-dependent autophagy initiation, and Atg5/7/8/12-p62 complexes; Pathogenic drivers: Oxidative stress (ROS/AGEs accumulation, SOD/GSH-Px depletion) and inflammatory activation (NLRP3 inflammasome, IL-1β/IL-6/TNF-α overproduction). (C) Pharmacological activation of mitophagy attenuates diabetic pathogenesis and chronic complications through mitochondrial quality restoration. The figure is drawn with biorender.com.
4.1 Metabolic damage in DM
Ginsenosides (Table 1), the primary bioactive constituents of Panax ginseng (Fan et al., 2023), are renowned for their antioxidant, anti-inflammatory, and anticancer attributes (Sarhene et al., 2021; Oh and Chun, 2022). These compounds have been shown to exert diverse beneficial effects, including the enhancement of insulin sensitivity, promotion of glucose uptake, and mitigation of OS through the reduction of ROS (Paik et al., 2023; Liu et al., 2019b; Zhou et al., 2019a). Additionally, ginsenosides have been reported to support lipid metabolism by lowering triglyceride (TG) and cholesterol levels, bolster mitochondrial function, and safeguard cardiovascular wellbeing by enhancing endothelial function and diminishing blood pressure (Sarhene et al., 2021; Xue et al., 2021; Hou et al., 2020). In a particular study, DM mice were administered ginsenosides (10 mg/kg) daily for 4 weeks. The findings revealed that ginsenosides facilitated the translocation of glucose transporter 4 to the plasma membrane in fatty acid-treated C2C12 cells, thereby enhancing glucose uptake and glycogen synthesis, in addition to improving mitochondrial quality. Notably, the DRP1/PINK1 axis was identified as a critical mediator in the ginsenoside-induced mitophagy process (Li et al., 2023a).
Silymarin (Table 1), a flavonol glycoside derived from Silybum marianum (Bijak, 2017), possesses hepatoprotective, neuroprotective, anti-inflammatory, anticancer, and islet-protective properties. In a study using the rat pancreatic β-cell line INS-1, cells were exposed to 0.4 mM palmitic acid (PA) and 25 mM HG for 24 h, with silymarin administered 4 h prior to PA and HG treatment. The results showed increased levels of total iron, lipid ROS, MDA, and COX-2, alongside decreased levels of iron death inhibitory molecules such as GSH, GPX4, and FSP1. Moreover, PINK1/parkin-mediated mitophagy was repressed. The protective effect of silymarin against PA and HG-induced ferroptosis was shown to be dependent on mitophagy, as evidenced by silencing PINK1 expression using mitophagy agonists and inhibitors like urolithin A (UA) and cyclosporine A (CsA), as well as siRNA transfection (Du et al., 2023).
Punicalagin (PU) (Table 1), a hydrolyzable tannin derived from pomegranate rind polyphenols, is metabolized in vivo to ellagic acid and urolithin, and exerts antioxidant, anti-inflammatory, and lipid metabolism-regulating properties (Alami et al., 2024; Cerdá et al., 2003; Johanningsmeier and Harris, 2011). In animal studies, DM mice were treated with PU (20 mg/kg) via gavage once a day for 8 weeks. Cellular experiments with HepG2 cells exposed to HG for 48 h and treated with PU revealed significant reductions in FBG, fasting serum insulin, and the homeostasis model assessment of IR in DM mice. Additionally, serum and liver levels of markers such as ALT, AST, TC, TG, LDL-C, FFA, MDA, and total superoxide dismutase (T-SOD) were notably reduced. Liver steatosis and inflammation were also mitigated. Importantly, MMP was distinctly increased, and levels of PINK1, Parkin, BNIP3, LC3B, P62, MnSOD, and CAT were evidently elevated in liver tissue. The activities of MnSOD and CAT were also notably elevated in both serum and liver, correlating with the in vitro findings. These results suggest that mitophagy and antioxidant enzyme activities were elevated, offering protection against diabetic liver injury (Zhang et al., 2022c).
Mangiferin (MF) (Table 1), a flavonoid derived from mango, exhibits diverse biological activities, including anticancer, antidiabetic, and anti-obesity effects (Sim et al., 2019; Ramírez et al., 2017; Sekar et al., 2019; Du et al., 2018). In a study involving mouse C3H10T1/2 mesenchymal stem cells (MSCs), MF was incorporated into a lipid-inducing medium (MDI) for 2 to 4 consecutive days, followed by maturation culture. The results identified that MF treatment induced the expression of brown adipose markers such as UCP1, TG, PGC1α, PRDM16, and PPARγ, in addition to upregulating beige adipose markers (CD137, HSPB7, TBX1, and COX2) in both C3H10T1/2 MSCs and human adipose-derived MSCs (hADMSCs). MF modulated mitochondrial fusion dynamics by repressing PINK1-Parkin-mediated mitophagy, thus increasing mitochondrial DNA levels and improving mitochondrial homeostasis. Furthermore, there was an enhancement in mitochondrial respiration, demonstrated by elevated mitochondrial oxygen consumption and an elevation of oxidative phosphorylation (OXPHOS)-related proteins. Through chemical inhibition and knockdown assays, it was revealed that the β3-AR-dependent PKA-p38 MAPK-CREB signaling, mediated by mitophagy, is pivotal in MF-mediated brown fat formation (Rahman and Kim, 2020).
Berberine (BBR) (Table 1), an isoquinoline alkaloid primarily extracted from Berberis vulgaris (Zhu et al., 2019), is well recognized for its broad pharmacological effects, which include anti-inflammatory, antioxidant (Tian et al., 2019), and modulatory actions on glucose and lipid metabolism (Wu et al., 2016). In one experiment using the rodent pancreatic β-cell line INS-1, cells were pretreated with 5 µM of BBR for 1 h, followed by exposure to 0.5 mM PA for 24 h. The results indicated that BBR significantly improved cell viability under PA-induced stress, suppressed apoptosis, and enhanced insulin secretion. Additionally, BBR alleviated oxidative damage by reducing ROS production and boosting the activities of antioxidant enzymes. It was also observed that BBR restored ATP production and MMP, both of which were diminished upon PA treatment. Further analysis revealed that BBR induced mitophagy in PA-treated INS-1 cells, as evidenced by alterations in the expression levels of mitophagy-related proteins. Moreover, BBR promoted the nuclear translocation and DNA-binding activity of Nrf2, a key antioxidant transcription factor involved in mitophagy regulation. Interestingly, the protective effects of BBR on cell viability, apoptosis, and mitochondrial function were abolished by silencing PINK1 expression, underscoring the critical role of mitophagy in mediating its beneficial actions (Li et al., 2022).
Melatonin (Mel) (Table 1), a hormone synthesized by the pineal gland and secreted during the night (Reiter et al., 2009), plays essential roles in regulating the sleep-wake cycle, as well as possessing antioxidant and immunomodulatory properties. In a study containing T2DM rats, Mel (20 mg/kg) was administered daily for 1 week. Results showed that SIRT3 signaling and mitophagy were suppressed after diabetic lung I/R injury (LIRI). Mel treatment distinctly induced mitophagy and restored SIRT3 expression. This intervention improved lung function recovery, suppressed inflammation, reduced oxidative damage, decreased apoptosis, and preserved mitochondrial function, thus attenuating the progression of diabetic LIRI (Song et al., 2023).
Nobiletin (Table 1), a polymethoxyflavonoid derived from the peels of oranges and lemons, is known for its antihypertensive (Potue et al., 2019), anti-cancer (Shi et al., 2013), anti-inflammatory (Nohara et al., 2019; Namkoong et al., 2017), anti-obesity, and anti-aging properties. In animal studies, DM mice were orally administered nobiletin (100 or 150 mg/kg) once daily for 3 weeks. In cellular experiments, NIT-1 cells were pretreated with 10 or 12 µM nobiletin for 3 days. The results indicated that nobiletin evidently alleviated hyperglycemia in DM mice and effectively activated mitophagy in NIT-1 cells. It also suppressed inflammatory pathways and restored MMP that had been disrupted by glucotoxicity in the cells. The hypoglycemic potentials of nobiletin seemed to be mediated by the modulation of intestinal microbiota dysbiosis, activation of mitochondrial autophagic processes, repression of inflammatory vesicle expression, and restoration of pancreatic islet morphology in DM mice (Yuan et al., 2022b).
Puerarin (Table 1), an isoflavonoid predominantly found in the roots of Pueraria lobata (Willd.), has long been used both as a dietary supplement and a traditional remedy for managing diabetic conditions (Zhou et al., 2014; Zhang et al., 2013; Wong et al., 2011). Studies have demonstrated that P. lobata improves insulin signaling dysfunction in skeletal muscle, particularly in HFD/STZ-induced DM rats and palmitate-treated insulin-resistant myotubes (Chen et al., 2018a). In a controlled study, rat L6 skeletal muscle cells were pre-exposed to 0.3 mM puerarin for 24 h, followed by treatment with 0.75 mM palmitate for an additional 24 h. The results demonstrated that puerarin pretreatment significantly improved insulin sensitivity and alleviated palmitate-induced mitochondrial dysfunction in muscle cells. This was evidenced by enhanced complex I activity, elevated MMP, increased ATP production, and a reduction in ROS. Furthermore, puerarin upregulated the expression of mitochondrial biogenesis-related key genes, oxidative phosphorylation, and ROS detoxification. The compound also modulated mitochondrial dynamics, regulating both fusion and fission processes, and reversed palmitate-induced impairments in mitophagy via the PINK1/Parkin axis. Additionally, puerarin mitigated palmitate-induced inflammation by suppressing the TLR4/NF-κB axis (Chen et al., 2018b).
Resveratrol (RES) (Table 1), a naturally occurring polyphenolic antioxidant found in peanuts, pine, and grape skins (Wang et al., 2014; Alamdari et al., 2012; Russell et al., 2006; Wyke and Tisdale, 2006), has garnered significant interest due to its antioxidant properties and its ability to repress protein degradation, thereby mitigating muscle fiber atrophy in various in vitro systems. In recent years, RES has been recognized for its potential to counteract muscle wasting in conditions such as DM, cancer, and cachexia (Momken et al., 2011; Shadfar et al., 2011; Chen et al., 2011; Bennett et al., 2013; Joseph et al., 2013). In one experiment, diabetic mice were fed a diet containing 0.04% RES for a period of 8 weeks. The findings indicated that RES addition evidently reduced muscle atrophy and improved overall skeletal muscle function. This was achieved through the reduction of key markers such as ubiquitin and muscle ring finger protein-1 (MuRF-1), along with a decline in LC3-II and cleaved caspase-3 levels. Additionally, RES treatment promoted mitochondrial biogenesis and mitigated excessive mitophagy activation in the skeletal muscle. Notably, RES also protected muscle tissue from abnormal mitochondrial dynamics, including excessive fusion and fission in DM (Wang et al., 2018a).
Cyanidin 3-glucoside chloride (C3G) (Table 1), a typical anthocyanin, is considered one of the most significant flavonoids with potential health benefits across a range of diseases (Guo and Ling, 2015). Accumulating evidence has demonstrated that C3G exhibits antioxidant, anti-inflammatory, hepatoprotective, and anticancer properties, partly attributed to its free radical scavenging ability (Wang et al., 2019; Li et al., 2020a). In one study, DM mice were given an aqueous solution of C3G (150 mg/kg) once daily for 6 weeks. C3G was capable of activating mitophagy by elevating the expression of PINK1 and PARKIN, as evidenced by the accumulation of LC3 and a reduction in mitochondrial number. The autophagy inhibitor chloroquine (CQ) blocked C3G-induced mitophagy and inhibited the ability of C3G to reduce ROS production, suggesting that mitophagy is crucial in the alleviation of OS in islet cells by C3G (Ye et al., 2021).
4.2 Microvascular lesions
4.2.1 DKD
Sulforaphane (SFN) (Table 2), an isothiocyanate derived from cruciferous vegetables, is a potent antioxidant with potential protective effects against DM-induced cellular damage (Gu et al., 2017). Previous studies have documented the nephroprotective properties of SFN in DKD (Li et al., 2020b). In one study, DM mice were treated with different doses of SFN (6.25 or 12.5 mg/kg) three times a week for 12 weeks. The results revealed that overexpression of PINK1 in Nrf2 conditional knockout (cKO) mice resulted in a reduction of the urinary albumin/creatinine ratio, blood urea nitrogen, and serum creatinine levels. Furthermore, PINK1 overexpression diminished p62 protein levels and attenuated mitochondrial damage, increasing the number of membrane-intact mitochondria and mitochondrial autophagic vesicles. SFN was shown to activate mitophagy in podocytes, restore urinary albumin levels, and prevent glomerular hypertrophy and excessive pedunculated fusion in DM mice. The nephroprotective effect of SFN was abolished in podocyte-specific Nrf2 cKO mice, suggesting that SFN mitigates DM-induced podocyte damage through the Nrf2/PINK1 axis. In vitro, SFN treatment elevated PINK1 expression and activated mitophagy in HG-treated podocytes. Additionally, SFN translocated Nrf2 to the nucleus, where it bound to the PINK1 promoter, enhancing PINK1 transcription. Conclusively, SFN attenuates podocyte damage in DKD by regulating the Nrf2/PINK1 axis and balancing mitophagy, thereby preserving mitochondrial homeostasis (Wang et al., 2023c).
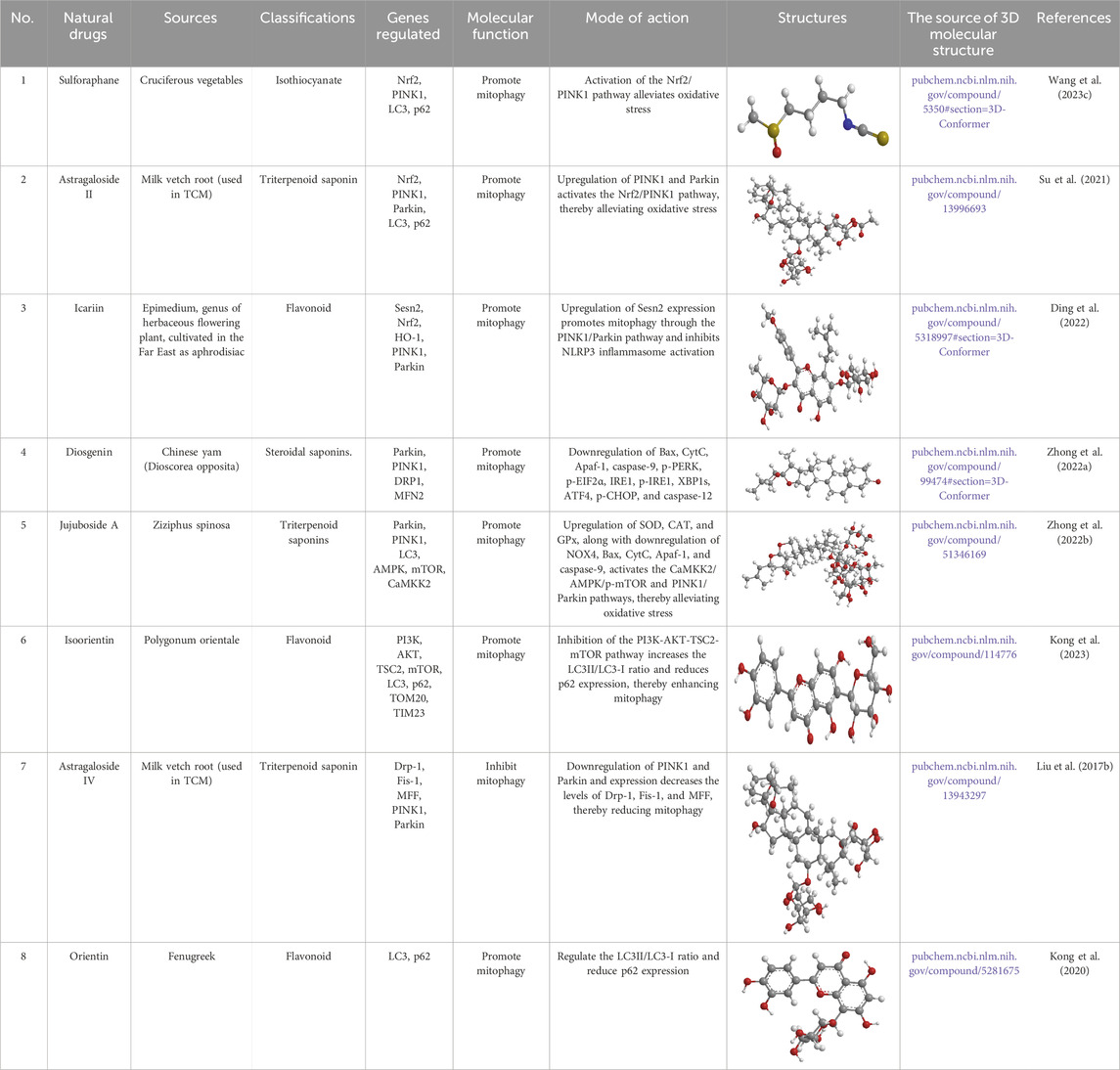
Table 2. Source, classification and structure of compounds for the treatment of diabetic nephropathy.
Astragaloside II (AS II) (Table 2), a bioactive compound derived from Astragalus, is well known for its anti-inflammatory and wound-healing capabilities, particularly in the context of inflammatory bowel disease (Qiao et al., 2019; Lee et al., 2017). In a study involving DM rats, AS II was administered daily at doses of 3.2 or 6.4 mg/kg over a period of 9 weeks. The results revealed that AS II treatment partially restored the expression of critical mitochondrial proteins involved in mitochondrial dynamics, such as Mfn2, Fis1, P62, and LC3. Furthermore, AS II brought about elevations in the expression of PINK1 and Parkin, which are vital for the modulation of mitophagy in these rats. The treatment also elevated Nrf2 expression and suppressed Keap1, thereby promoting resistance to OS. These findings highlight that AS II may help mitigate podocyte damage and mitochondrial dysfunction in DM rats, likely through the modulation of the Nrf2 and PINK1/Parkin signaling (Su et al., 2021).
Mel (Table 1), a hormone produced by the pineal gland, is central to regulating circadian rhythms and has critical roles in mitochondrial function (Reiter et al., 2018), energy metabolism (Cipolla-Neto et al., 2014), lipid regulation (Jin et al., 2017), and reproduction (Olcese, 2020). Mitochondrial dysfunction (Zhou et al., 2019b), abnormal energy homeostasis (Bhargava and Schnellmann, 2017), and lipid metabolism (Herman-Edelstein et al., 2014) are intimately linked to DKD progression. Mel is capable of ameliorating hyperglycemia-induced steroidogenesis impairment in Leydig cells by activating the SIRT1 axis (Wang et al., 2022b). In a study on DM mice with kidney injury, Mel (0.2 mg/kg) was administered daily for 12 weeks. The results demonstrated that Mel treatment stimulated AMPK phosphorylation, which facilitated the translocation of PINK1 and Parkin to mitochondria, thus activating mitophagy. This process alleviated OS and reduced inflammatory responses. Notably, the nephroprotective effects of Mel were partially diminished when PINK1 was diminished or AMPK was inhibited, indicating that Mel’s protective action in DKD is, at least in part, mediated through the AMPK/PINK1/mitophagy axis (Tang et al., 2022).
Icariin (ICA) (Table 2), a flavonoid extracted from the traditional Chinese medicine (TCM) plant Epimedium (Qi et al., 2021a), has been shown to improve lipid metabolism disorders, reduce inflammation, enhance insulin sensitivity, and alleviate mitochondrial dysfunction (Wang et al., 2020; Qiao et al., 2018; Han et al., 2015). ICA also acts on glomerular plasma membrane cells via the TGF-β1/Smad2 axis, increasing gelatinase activity and promoting the degradation of excess extracellular matrix (ECM) (Li et al., 2013). In a study involving DM rats treated with different doses of ICA (20, 40, 80 mg/kg) once daily for 8 weeks, ICA evidently elevated the expression of LC3II, Sesn2, PINK1, and Parkin, while downregulating the inflammatory markers NLRP3, caspase-1 (both pro-caspase-1 and its active form, caspase-1 p10), and IL-1β in a dose-responsive fashion. Conclusively, ICA may attenuate the inflammatory response by inducing autophagy. ICA also activates Nrf2, suppresses NLRP3, and promotes Keap1 degradation through Sesn2-dependent mitophagy. When mitophagy signaling was blocked by Sesn2 siRNA, the results demonstrated that ICA-induced repression of NLRP3 and mitophagy was diminished, confirming the involvement of Sesn2 in this process (Ding et al., 2022).
Diosgenin (Table 2), a steroidal saponin (Mahmoudi et al., 2021), is recognized for its anticancer properties (Zheng et al., 2023a). Diosgenin exerts its therapeutic benefits in type II diabetic nephropathy by targeting CaMKK2 and enhancing autophagy, mitochondrial dynamics, and mitophagy (Zhong et al., 2023). In a study focusing on DM rats, dioscin (20 mg/kg) was administered daily for 8 weeks. The results demonstrated that dioscin mitigated mitochondrial and endoplasmic reticulum stress (ERS)-induced apoptosis by diminishing the expression of pro-apoptotic proteins, including Bax, CytC, Apaf-1, caspase-9/12, pPERK, p-EIF2α, IRE1, p-IRE1, XBP1s, ATF4, and p-CHOP. Additionally, dioscin improved mitochondrial quality and quantity by modulating key mitochondrial proteins like Parkin, PINK1, DRP1, p-DRP1, and MFN2 (Zhong et al., 2022a).
Jujuboside A (JuA) (Table 2), a triterpenoid saponin isolated from Jujube seeds, is renowned for its antioxidant, anti-inflammatory, and anti-apoptotic attributes (Zhong et al., 2022b). JuA has shown potential in mitigating HFD/STZ-induced diabetic nephropathy, by inhibiting OS, reducing apoptosis, and promoting autophagy. In a study with diabetic Sprague-Dawley rats, JuA (20 mg/kg) was administered once daily for 8 weeks. The findings revealed that JuA enhanced mitochondrial respiratory chain function by modulating the expression of respiratory chain complexes, reducing levels of superoxide anion and H2O2, and inducing the activities of antioxidant enzymes like SOD, CAT, and GPx, while decreasing NOX4 expression. Furthermore, JuA diminished mitochondrial apoptotic proteins, including Bax, CytC, Apaf-1, and caspase-9. Additionally, JuA promoted mitophagy through the CaMKK2/AMPK/p-mTOR and PINK1/Parkin pathways (Zhong et al., 2022b).
Isoorientin (ISO) (Table2),a flavonoid also known as 3′,4′,5,7-tetrahydroxyflavone-6-D-glucopyranoside or lignan 6-C-glucoside, is a constituent of fenugreek (Ziqubu et al., 2020). ISO has been found to help prevent metabolic complications such as hyperglycemia, hyperlipidemia, and IR ((Malik et al., 2019; Yuan et al., 2016). Its therapeutic effects are attributed to its antioxidant and anti-inflammatory properties (Alonso-Castro et al., 2012). In a study involving DM mice treated with varying doses of ISO (10, 20, or 40 mg/kg) once daily for 2 months, ISO was shown to promote autophagy in podocytes and protect them from HG-induced injury. Under HG conditions, ISO improved the autophagic clearance of damaged mitochondria. ISO also reversed hyperphosphorylation of TSC2 S939 and stimulated autophagy by inhibiting the PI3K-AKT-TSC2-mTOR axis. Additionally, ISO is validated to bind to the SH2 structural domain of PI3Kp85β, which is critical for its recruitment (Kong et al., 2023).
BBR (Table 1), an alkaloid extracted from the stem bark, roots, and rhizomes of plants in the Berberidaceae family (Neag et al., 2018), exhibits diverse pharmacological effects, including antidiabetic (Kapoor et al., 2014), antimicrobial (Xia et al., 2022), antihyperlipidemic (Wu et al., 2022b), anticancer (Saxena et al., 2018), antihypertensive (Fatehi-Hassanabad et al., 2005), antidepressant (Sun et al., 2014), and neuroprotective effects. In an experimental study, NRK-52E cells were exposed to varying concentrations of D-glucose (5.5, 30, 40 mM) for 24 h, followed by a co-treatment with 20 µM BBR for an additional 24 h. The results indicated that BBR provided protective effects in hyperglycemic cells by ensuring mitochondrial structure and function. Co-treatment with SRT-1720, a SIRT1 activator, enhanced autophagy, decreased apoptosis, elevated the expression of downstream proteins such as FoxO3a and Bnip3, and alleviated mitochondrial dysfunction. Conversely, the use of nicotinamide, a SIRT1 inhibitor, reversed these beneficial effects. Additionally, a GFP reporter gene assay showed that BBR intervention increased the transcriptional activity of FoxO, which was linked to elevated Bnip3 expression. Knockdown of FoxO3a resulted in impaired autophagy and increased apoptosis. Pre-treatment with N-acetyl-L-cysteine demonstrated that ROS were involved in the HG-induced cellular toxicity in NRK-52E cells. Moreover, co-treatment with BBR led to changes in the expression of key proteins related to autophagy and mitophagy, including LC3B, ATGs, Beclin1, Sirt1, Bnip3, FoxO3a, and Parkin. Transmission electron microscopy confirmed the enhancement of mitophagy following BBR treatment (Saxena et al., 2024).
Astragaloside IV (AS-IV) (Table 2), a lanolinoloidal tetracyclic triterpenoid saponin derived from Astragalus membranaceus, has diverse pharmacological activities, including anti-inflammatory, antioxidative, anti-apoptotic, and anti-fibrotic properties (Li et al., 2017a). AS-IV is capable of improving DKD in animal models through its anti-inflammatory mechanisms (Gui et al., 2013), inhibition of ERS (Wang et al., 2015), and protection of podocytes (Chen et al., 2014; Gui et al., 2012). In a study, DM mice were administered AS-IV (1 g/kg) for 12 weeks. The results showed that AS-IV distinctly limited urinary albumin excretion and urinary N-acetyl-β-D-glucosaminidase, while ameliorating renal pathology. Moreover, AS-IV administration was linked reduced expression of mitochondrial fission regulators, including Drp-1, Fis-1, and MFF in DM mice. PINK1/Parkin-mediated mitophagy was found to be abnormally activated in the diabetic group. AS-IV administration notably decreased the expression of Drp-1, Fis-1, and MFF and diminished PINK1/Parkin-mediated mitophagy. This suggests that AS-IV may delay the progression of DKD by modulating mitochondrial dynamics and autophagy in DM mice (Liu et al., 2017b).
Trigonella foenum-graecum (fenugreek), an important treatment for DKD (Jiang et al., 2018), contains Orientin (Table 2), a C-glycosylated flavonoid, which is the primary bioactive component. Orientin has demonstrated antidiabetic, antioxidant, and autophagy-inducing effects (Zhong et al., 2019; Li et al., 2019b; Lawal et al., 2019; Kasangana et al., 2019). In one study, MPC-5 cells were incubated with 30 mM glucose and 120 µM orientin. It was found that Orientin restored cell proliferation in HG-induced conditions by reducing apoptosis. Orientin also protected mitochondrial membrane integrity in HG-treated cells, likely through its role in autophagy. Furthermore, Orientin distinctly enhanced cellular resistance to apoptosis and promoted autophagy by regulating mitochondria in podocytes. The protective effects of Orientin were reversed by 3-MA, an autophagy inhibitor, confirming the involvement of autophagy in its protective action (Kong et al., 2020).
4.2.2 DR
Allicin (Alc) (Table 3), a natural compound found in garlic, is recognized for its antioxidant and anti-inflammatory properties, making it a promising therapeutic agent for DR. In one study, DM rats were treated with Alc (16 mg/kg) for 28 days. Alc treatment effectively ameliorated histopathological changes and metabolic abnormalities associated with T2DM. It diminished focal death-associated proteins, elevated mitophagy-related proteins, reduced PIC levels, and attenuated OS. The antioxidant and anti-inflammatory effects of Alc were, in part, mediated by the activation of the mitophagy axis through the PINK1/Parkin signaling cascade. In conclusion, Alc mitigates DR in rats by activating PINK1/Parkin-mediated mitophagy and inhibiting OS-induced inflammation, specifically through the NOD-like receptor family pyrin structural domains (Xu and Yu, 2024).
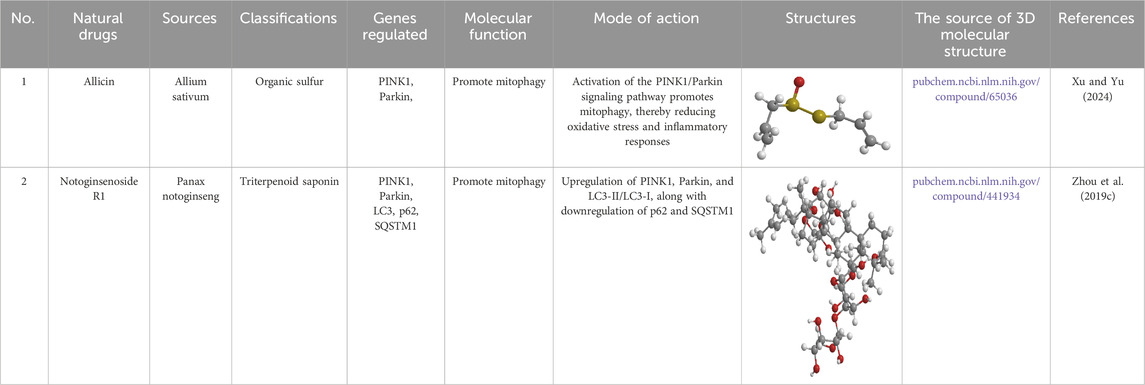
Table 3. Source, classification and structure of compounds for the treatment of diabetic retinopathy.
Notoginsenoside R1 (NGR1) (Table 3), a novel saponin extracted from P. ginseng, has demonstrated therapeutic effects, including the treatment of diabetic encephalopathy and the improvement of microcirculatory disorders. In one study, DM mice were treated with NGR1 (30 mg/kg) daily for 12 weeks. The findings revealed that NGR1 effectively reduced apoptosis, suppressed VEGF expression, and elevated the levels of pigment epithelium-derived factor. Additionally, NGR1 treatment alleviated OS and inflammation in rat retinal Müller cells (rMC-1) exposed to HG and in the retinas of DM mice. Moreover, NGR1 administration increased the expression of PINK1 and Parkin in both HG-treated rMC-1 cells and DM mouse retinas. This was accompanied by a higher LC3-II/I ratio and reduced levels of p62/SQSTM1. Furthermore, NGR1 promoted the co-localization of GFP-LC3 and MitoTracker in rMC-1 cells. Notably, silencing PINK1 abolished the protective potentials of NGR1, suggesting that NGR1 mitigates DR by enhancing mitophagy via a PINK1-dependent mechanism (Zhou et al., 2019c).
Mel (Table 1) has been demonstrated to be effective in the management of DR. Mel helps preserve the integrity of the blood-retinal barrier via diminishing hypoxia-inducible factors (HIF-1α, HIF-1β), VEGF, and its receptor genes. Additionally, Mel downregulates genes associated with mitochondrial fission (e.g., DRP1, hFis1, MIEF2, MFF) and mitophagy (such as PINK1, BNip3, and NIX), while promoting the expression of genes associated with mitochondrial biogenesis, including PGC-1α, NRF2, and PPAR-γ, thus supporting mitochondrial homeostasis. In an in vitro model of diabetic macular edema induced by hyperglycemia and hypoxia, Mel also mitigated blood-retinal barrier dysfunction and mitochondrial damage, demonstrating its protective effects (Doğanlar et al., 2021).
Several natural small molecules also show promise in treating DR. Cannabis derivatives play significant neuroprotective and neuroregenerative roles by reducing neurotoxicity, inflammation, and blood-retinal barrier disruption in diabetic animals, likely through inhibition of MAPK signaling. Cannabidiol may alleviate vascular leakage in DR, with its effects potentially linked to antioxidant, anti-inflammatory properties, and modulation of mitophagy (Kokona et al., 2016; Ramirez et al., 2022). Finally, RSV has been shown to promote mitophagy via SIRT1 activation, increase AMPK activity, inhibit NF-κB to control inflammation, and reduce ROS production, thus exerting protective effects on retinal ganglion cells (Guo et al., 2022).
4.2.3 DCM
RES (Table 1) is a biologically active natural polyphenolic compound known for its antioxidant properties, which can attenuate OS in DCM (Su et al., 2022). In one study, DM mice were injected with RES (50 mg/kg) once daily for 7 days. The results validated that RES elevated mitochondrial Parkin and diminished p62 in myocardial tissues of DM mice, suggesting that RES promotes mitophagy in diabetic myocardium. Additionally, myocardial p53 expression was elevated in DM mice, but RES diminished p53 expression and its binding to Parkin. This reduction in p53 binding to Parkin was inferred to enhance mitophagy, thereby alleviating diabetic myocardial injury (Wu et al., 2020).
Mel is a potent antioxidant with established benefits across various diseases (Fu et al., 2020). In one study, diabetic myocardial infarction mice were treated with Mel (50 mg/kg) once daily for 4 weeks after myocardial infarction surgery. The results revealed that Mel diminished intracellular levels of Bax, caspase-3, and p62, while upregulating Bcl-2, LC3-II/I, and Parkin. Furthermore, Mel distinctly increased intracellular ATP content (Jiao et al., 2022). These findings suggest that Mel can effectively reduce mitochondrial dysfunction and inhibit cardiomyocyte damage after myocardial infarction by enhancing mitophagy.
Fucoxanthin (FX) (Table 4), a carotenoid derived from marine sources, is known for its potent antioxidant properties and has been studied for its therapeutic significance in DCM. In an experimental study, DM rats were administered varying doses of FX (200 mg/kg) daily for 12 weeks. The results demonstrated that FX activated Nrf2 signaling, leading to a reduction in ROS levels. Additionally, FX enhanced Bnip3/Nix pathways, which contributed to improved mitochondrial function and a decrease in both mitochondrial and intracellular ROS, effectively reversing HG-induced hypertrophy in H9c2 cells. However, the application of CQ, an autophagy inhibitor, negated the anti-hypertrophic effects of FX, resulting in compromised mitochondrial function and elevated ROS levels. Furthermore, FX treatment reduced the accumulation of key fibrosis markers, including TGF-β1, fibronectin (FN), and α-smooth muscle actin (α-SMA), thus attenuating myocardial fibrosis in STZ-induced DM rats. FX also promoted mitophagy through the elevation of Bnip3/Nix and enhanced Nrf2-mediated signaling to mitigate OS, which contributed to the inhibition of HG-induced hypertrophy in H9c2 cells (Zheng et al., 2022).
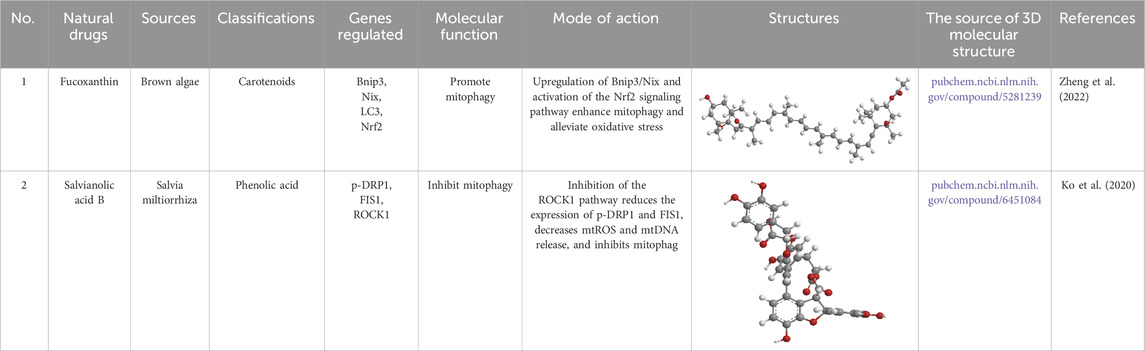
Table 4. Source, classification and structure of compounds for the treatment of atherosclerotic cardiovascular diseases.
4.3 ASCVDs
Scutellarin, a natural compound, exhibits excellent antioxidant (Wang et al., 2016; Mo et al., 2018), anti-inflammatory (Zhang et al., 2017), vasodilator (Koon et al., 2014), antidiabetic activity (Yang et al., 2017), and vasoprotective (Du et al., 2015) properties. In one study, human umbilical vein endothelial cells (HUVECs) were subjected to HG treatment to induce VEC injury in vitro, followed by Scutellarin treatment (30 μM) for 48 h. The results demonstrated that Scutellarin notably improved cell viability in HG-exposed HUVECs. Additionally, Scutellarin diminished apoptosis-related proteins like Bcl-2, Bax, and cytochrome C (Cyt.c), thereby inhibiting cell death through a mitochondrial-dependent mechanism. Furthermore, Scutellarin alleviated OS by enhancing SOD activity, upregulating SOD2, and reversing the loss of MMP. Scutellarin also promoted autophagic flux, as evidenced by elevations in the levels of LC3-II, Beclin 1, and autophagy-related gene 5 (Atg5), while decreasing the levels of Sequestosome1/P62 in HG-treated HUVECs. Moreover, Scutellarin elevated key proteins involved in mitophagy, including PINK1, Parkin, and Mitofusin 2, indicating an enhancement of MQC. Silencing PINK1 diminished the beneficial effects of Scutellarin, specifically attenuating the HG-induced Parkin under-expression, ROS overproduction, and the elevated levels of p62, Cyt. c, and cleaved caspase-3. Molecular docking studies further revealed a strong binding interaction between baicalin and PINK1 proteins, suggesting a direct involvement in the modulation of mitochondrial function (Xi et al., 2021).
Mel (Table 1) has also been shown to ameliorate cardiomyocyte damage. In one study, DM mice were administered Mel (20 mg/kg) once daily for 4 weeks. In cellular experiments, primary cardiomyocytes were treated with Mel (100 μmol/L) for 4 h, followed by HG incubation for 48 h. The results revealed that Mel increased the clearance of dysfunctional mitochondria, promoted LC3-II expression, and enhanced the co-localization of mitochondria and lysosomes in HG-challenged cardiomyocytes. Mel also increased the number of typical autophagosomes phagocytosing mitochondria in the hearts of DM mice. These findings pinpoint that Mel promotes mitophagy. Knockdown of Parkin abolished the beneficial potentials of Mel in cardiac mitochondrial morphology and bioenergetic disturbances, eliminating the significance of Mel on remodeling in DCM hearts. Moreover, Mel inhibited the phosphorylation of mammalian sterile 20-like kinase 1 (Mst1), thereby facilitating Parkin-mediated mitophagy, which contributes to MQC (Wang et al., 2018b).
BBR (Table 1) has also demonstrated beneficial effects on cardiomyocytes. In one study, HG-induced injury was modeled in the H9C2 cardiomyocyte cell line. BBR was pretreated at 100 nM for 30 min, followed by the addition of 50 mM D-glucose for 24 h. The results demonstrated that BBR corrected the imbalance between mitochondrial fusion and fission, significantly alleviating hypertrophy in the H9C2 cells and improving mitochondrial function. BBR further promoted mitochondrial biogenesis and cleared damaged mitochondria through mitophagy. This effect was mediated via the activation of the AMPK axis, which restored autophagic flux in HG-induced cardiomyocyte injury (Hang et al., 2018).
Salvianolic acid B (Sal B) (Table 4), from the roots and rhizomes of Salvia divinorum (family Labiatae), possesses antioxidant, anti-inflammatory, and antithrombotic properties. In one study, DM mice were administered Sal B (50 mg/kg) once daily for 14 days. It was found that Sal B reduced inflammatory cell infiltration in tissues and facilitated angiogenesis. In both diabetic tissues and HG-induced human microvascular endothelial cells, Sal B improved apoptosis and enhanced mitophagy. Inhibition of Parkin impaired cell migration, promoted apoptosis, and inhibited mitophagy in human microvascular endothelial cells (Zhang et al., 2025). Moreover, Sal B prevented oxLDL-induced endothelial dysfunction under HG conditions by diminishing ROCK1-mediated mitochondrial dynamics and apoptosis (Ko et al., 2020).
4.4 Neurological complications
Baicalin, a naturally occurring flavonoid from Scutellaria baicalensis Georgi, is recognized for its neuroprotective properties. In an experimental study, DM rats were administered baicalin (100 mg/kg) daily for 1 week, while PC12 cells were cultured under HG conditions and treated with baicalin for 24 h. The results demonstrated that baicalin administration effectively reduced blood glucose levels, mitigated neurological impairments, and decreased infarct size. In vitro, under OGD/R conditions, ROS production and mitochondrial dysfunction were notably elevated in HG-treated PC12 cells. However, baicalin treatment suppressed the expression of dynamin-related protein 1 (Drp-1), which is involved in mitochondrial fission, while enhancing mitochondrial fusion by promoting the production of MFN2. Additionally, baicalin increased Drp-1 Ser637 phosphorylation, contributing to improved MMP by reducing ROS production (Li et al., 2017b).
Piceatannol (PCN) (Table 5), found in foods such as peanuts, mountain grapes, and lingonberries, is a natural analog of RES. It exhibits antioxidant, anticancer, anti-inflammatory, and neuroprotective properties (Jia et al., 2020; Setoguchi et al., 2014; Suh et al., 2018; Uchida-Maruki et al., 2015). In one study, DM rats were treated with various doses of PCN (10 or 20 mg/kg) once daily for 2 weeks. The results showed that PCN exposure restored mitochondrial function in HG-induced N2A cells by reducing ROS production, restoring mitochondrial superoxide levels, and improving MMP. Additionally, PCN exposure promoted neurite growth and induced mitochondrial biogenesis through PGC-1α activation, which was mediated by enhanced SIRT1 activation. SIRT1 activation also enhanced Nrf2-mediated antioxidant signaling, thereby counteracting mitochondrial dysfunction and reduced antioxidant activity in DM rats and HG-treated N2A cells (Khan et al., 2023).
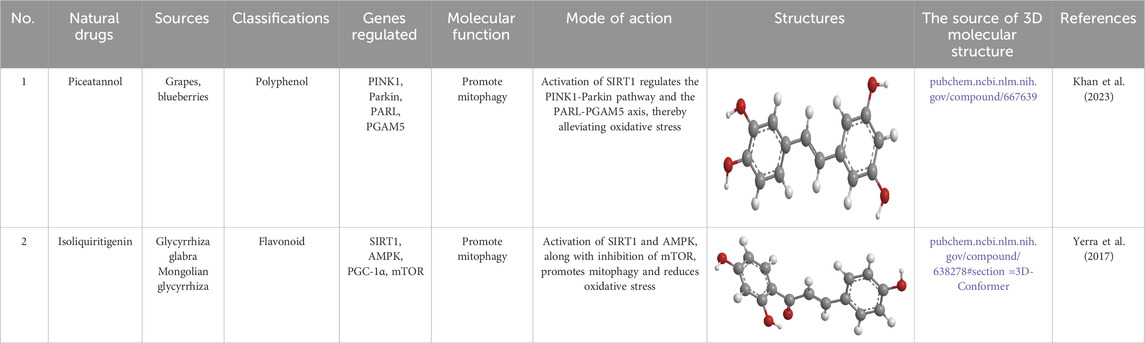
Table 5. Source, classification and structure of compounds for the treatment of diabetic neuropathy.
AS-IV (Table 2) also plays a significant role in diabetic neuropathy (DN). In a cellular experiment, Xuewang cells were treated with 50 μmol/L AS-IV for 72 h. The results showed that MMP was elevated in both the AS-IV and N-acetylcysteine (NAC) treatment groups, with MtMP evidently higher in the AS-IV group relative to the HG group (p < 0.01). AS-IV exhibited a protective effect on mitochondrial function, significantly increasing MtMP in Schwann cells (SCs) under HG conditions. Furthermore, the expression of autophagy-related proteins such as LC3, PINK1, and Parkin was diminished in the AS-IV group, suggesting that AS-IV inhibits the over-activation of autophagy in SCs. This reduction in autophagy-related proteins was linked to a notable decrease in ROS levels and improvements in mitochondrial morphology and membrane potential. These findings unfold that AS-IV protects mitochondrial integrity in SCs by reducing OS and autophagy activation in a HG environment (Wei et al., 2022).
Isoliquiritigenin (ILQ) (Table 5), a licorice-derived compound, is recognized for its potent antioxidant, anti-inflammatory, and anticancer capabilities (Kwon et al., 2009; Watanabe et al., 2016). It has also been investigated for its potential antidiabetic effects and other therapeutic benefits (Gaur et al., 2014). In a recent study, DM rats were treated daily with different doses of ILQ (10 or 20 mg/kg) for 2 weeks. The findings revealed that ILQ treatment notably activated SIRT1, which in turn enhanced mitophagy. Additionally, ILQ administration increased the NAD+/NADH ratio in the peripheral sciatic nerve. Functional and behavioral analyses showed that ILQ improved nerve conduction, restored blood flow to the nerves, and alleviated nociceptive hypersensitivity and abnormalities in the DM rats. In cultured N2A cells, ILQ was found to mitigate HG-induced ROS generation and mitochondrial membrane depolarization. Overall, ILQ’s activation of SIRT1 mimicked the effects of caloric restriction, promoting PGC-1α-mediated mitochondrial biogenesis, FOXO3a-mediated stress resilience, and AMPK-mediated autophagy, which collectively counteracted several pathophysiological features of experimental DN (Yerra et al., 2017).
Mel (Table 1) has also been implicated in DN. In an experiment using DMEM incubated for 24 h, HG intervention notably elevated PINK1 and LC3B expression. In addition, HG exposure reduced the levels of cytochrome c oxidase subunit 4 and decreased Mitotracker™ fluorescence intensity. Silencing PINK1 expression resulted in a marked accumulation of mitochondrial ROS, disruption of MMP, and an increase in the expression of cleaved caspase-3/9. Furthermore, the number of cells positive for the membrane-bound protein V was elevated. Notably, silencing PINK1 abolished the regulatory effects of Mel on mitochondrial ROS production, as well as its ability to activate cleaved caspase-3/9, and to increase the number of V-positive cells (Onphachanh et al., 2017).
4.5 DFUs and other categories
Cinnamaldehyde (Table 6), the principal bioactive compound in cinnamon, has long been recognized for its anti-inflammatory and wound-healing properties in TCM. Cinnamaldehyde exerts its beneficial effects by activating the PINK1/Parkin axis, which enhances mitophagy. This pathway activation leads to a decrease in PICs, such as IL-6 and TNF-α, while simultaneously increasing the expression of VEGF and collagen. These changes not only help in mitigating inflammation but also support angiogenesis and collagen production, ultimately accelerating wound healing in DM rats (Hong et al., 2023). RES (Table 1), a well-known natural antioxidant, has also demonstrated notable potential in treating diabetic ocular complications. Studies indicate that RES is effective in reducing ROS production in LECs exposed to HG conditions (Chen et al., 2022b). Moreover, RES improves mitophagy and decreases apoptosis in these cells, highlighting its therapeutic potential in managing diabetic eye diseases.

Table 6. Source, classification and structure of compounds for the treatment of diabetic foot ulcers.
In summary, Natural small molecules have shown potential in treating DM and its complications by regulating mitophagy. Current mechanistic studies have predominantly focused on the Pink1/Parkin-dependent pathway and the mitophagy receptor-dependent pathways (e.g., FUNDC1, BNIP3, NIX) to modulate mitochondrial dynamics in mammalian cells. The primary mechanism of action appears to involve alleviating OS, which in turn orchestrates mitophagy.
5 Clinical trials and patents
Natural small molecules demonstrate significant therapeutic potential for DM and its complications through modulation of mitophagy. However, clinical translation of these discoveries remains challenging. This Section summarizes the natural small molecules discussed in this review that have progressed to human clinical trials and analyzes key barriers to clinical validation, and examines the current status of related patents.
5.1 Natural small molecule clinical trials and challenges
Several natural small molecules have advanced to human clinical trials, demonstrating therapeutic potential for the management of DM and its associated complications. Clinical Research Summary of Mitophagy - Modulated Natural Small Molecules in Diabetes Mellitus are illustrated in Table 7.
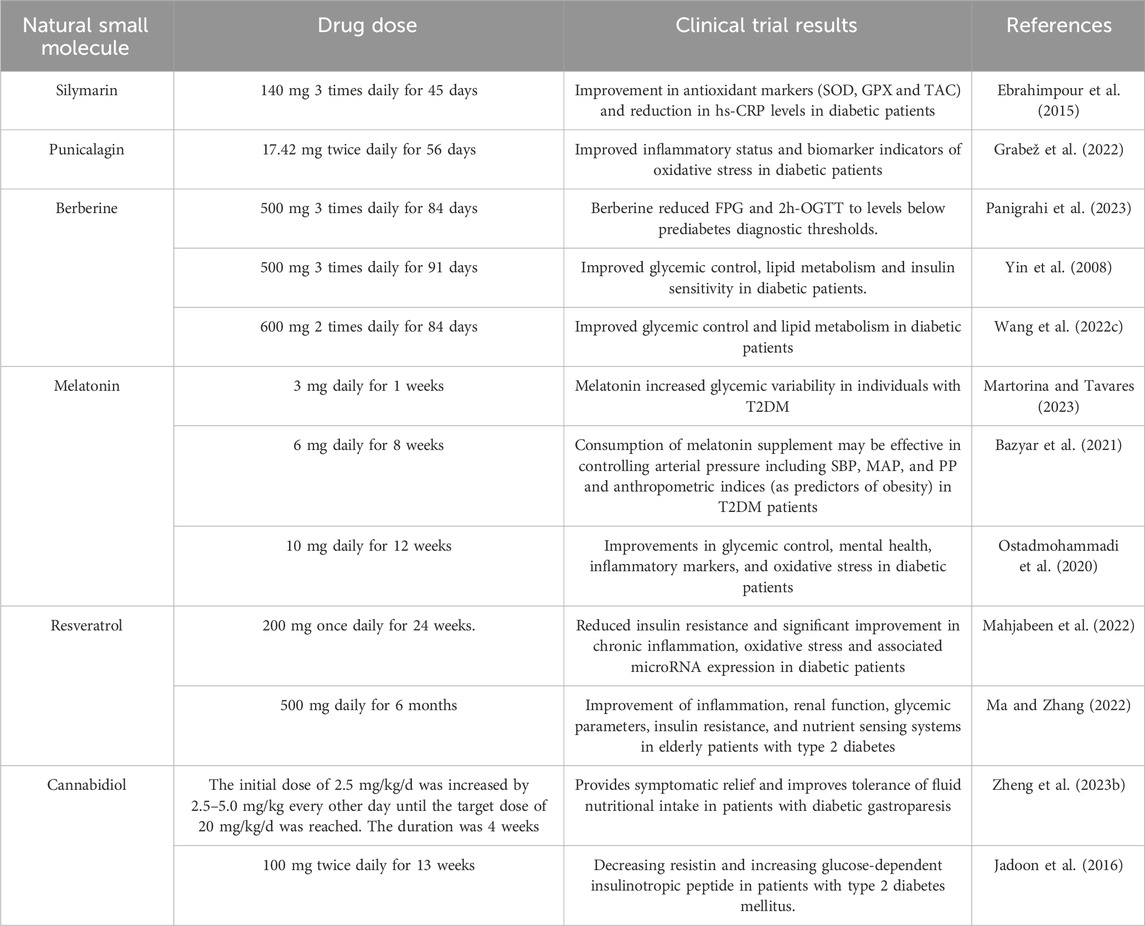
Table 7. Mitophagy-modulated natural small molecules in diabetes mellitus: Clinical research summary.
Although natural small molecules exhibit considerable therapeutic promise in preclinical research, their clinical translation faces five principal challenges: (1) Limited bioavailability due to inadequate absorption, non-targeted delivery mechanisms, off-target effects, and inherent toxicity (2) Significant pharmacokinetic variability affecting metabolic stability and therapeutic consistency (3) Insufficiently powered clinical trials with restricted longitudinal evaluation of safety profiles (4) Non-standardized extraction methodologies and formulation protocols leading to batch-to-batch variability (5) Absence of unified quality control standards compromising dosage reproducibility.
5.2 Patent status
The patent research, from the year 2023–2025, in the discipline of targeting the modulation of the mitophagy pathway using natural small molecules for the treatment of diabetes and its chronic complications, with the primary objective of either treating the condition or diminishing the disease progression, was conducted using the World Intellectual Property Organization’s official website. Patent Landscape Analysis of Mitophagy - Modulating natural small molecules for DM and Chronic Comorbidities is illustrated in Table 8.
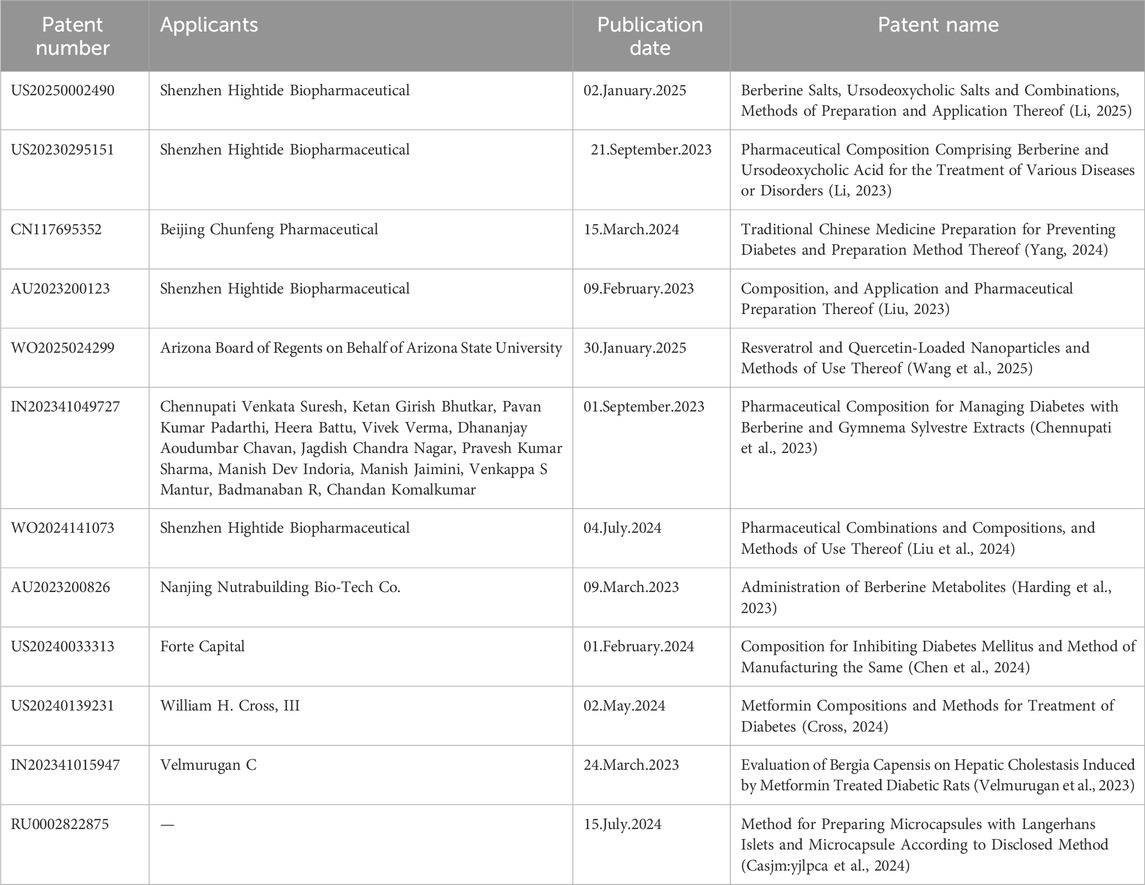
Table 8. Patent Landscape Analysis of Mitophagy-Modulating natural small molecules for DM and Chronic Comorbidities.
The therapeutic potential of natural small molecule in DM and its complications has garnered significant scientific interest, evidenced by exponentially increasing patent filings. Current intellectual property developments focus on three strategic domains: 1) Advanced drug delivery systems including nanoformulations and controlled-release matrices to enhance bioavailability (Uppal et al., 2018); 2) Synergistic combination therapies with conventional pharmacotherapeutics or nutraceutical adjuvants; 3) Novel therapeutic applications targeting diabetic complications such as cardiovascular pathologies, nephropathies, and neuropathies. Despite this surge in patent activity, translational implementation remains suboptimal, constrained by formulation challenges, market viability barriers, and intellectual property protection complexities.
In summary, mitophagy-modulating natural small molecules represent emerging therapeutic candidates for diabetes pathophysiology and its progressive sequelae. While select molecules have progressed to early-phase clinical evaluation, their therapeutic validation necessitates overcoming pharmacological barriers including suboptimal pharmacokinetic profiles, interindividual metabolic variability, and inadequately powered phase III trials.
6 Nanotechnology and natural small molecules
6.1 Nanotechnology, new opportunities for natural small molecules
Natural small molecules exhibit unique therapeutic advantages, yet face pharmacological challenges including low bioavailability and poor targeting specificity. The integration of nanotechnology has revolutionized their delivery, particularly for mitophagy-inducing agents such as resveratrol, silymarin, and berberine (Habtemariam, 2020; Kumar et al., 2015; Tillhon et al., 2012; Patel et al., 2011; Ochi et al., 2016; Elgizawy et al., 2021). Despite their mitophagy modulation potential, clinical translation of these molecules remains constrained by inherent stability limitations and nonspecific biodistribution. Nanocarrier systems including liposomes, polymeric micelles, and mesoporous silica nanoparticles have emerged as viable solutions: MSN-based formulations enhance resveratrol solubility and controlled release (Juère et al., 2017), while liposomal encapsulation improves biocompatibility and enables pharmacokinetic optimization through compositional modifications (Lian and Ho, 2001; Handa et al., 2021; Cui et al., 2022). The mechanistic basis of nanoparticle - mediated mitophagy regulation is illustrated in Figure 4. Natural small molecules regulating mitophagy by nanotechnology is illustrated in Table 9.
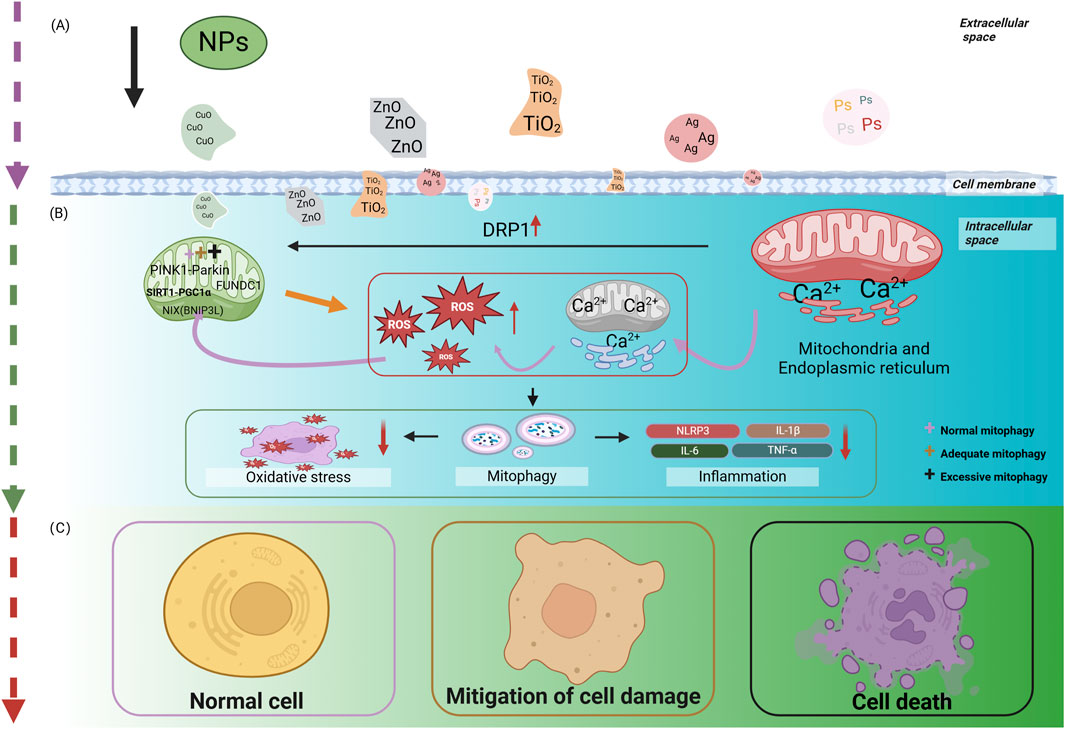
Figure 4. Illustrates the mechanistic basis of nanoparticle-mediated mitophagy regulation. (A) NPs penetrate the cell membrane. (B) NPs modulate the outcomes of mitophagy. (C) NPs regulate the outcomes of cells through mitophagy. PS-NPs, polystyrene nanoparticles; AgNPs, silver nanoparticles; TiO2NPs, titanium dioxide nanoparticles; CuONPs, copper oxide nanoparticles; DRP1, dynamin - related protein 1; NPs, nanoparticles; ROS, reactive oxygen species; ZnONPs, zinc oxide nanoparticles. The figure is drawn with biorender.com.
Diabetic heart disease exemplifies the critical role of mitochondrial dysfunction in disease pathogenesis, where impaired mitophagy exacerbates cardiac deterioration (Zorov et al., 2014; Mu et al., 2020) Natural small molecules demonstrate therapeutic potential by restoring mitophagy in cardiomyocytes. Specifically, resveratrol attenuates myocardial injury in diabetic murine models through p53/Parkin axis modulation, enhancing mitophagic flux (Wu et al., 2020). Nevertheless, the clinical utility of these molecules remains constrained by pharmacokinetic limitations. Nanocarrier-mediated delivery systems overcome these barriers by enabling cardiac-targeted RES, transport while stimuli-responsive nanoplatforms (e.g., pH- or ROS-activated systems) achieve spatiotemporal drug release, optimizing therapeutic outcomes (Uppal et al., 2018; Wu et al., 2022c; Qiu et al., 2023; Nag et al., 2024).
6.2 Challenges and limitations
Mitophagy research confronts three principal methodological and translational challenges. First, current detection modalities—including LC3-II/p62 immunoblotting and transmission electron microscopy—lack real-time monitoring capacity for autophagic flux dynamics and cannot distinguish mitophagy from general macroautophagy. Second, clinical translation barriers persist due to suboptimal bioavailability of natural small molecules, absence of validated biomarkers, and interpatient heterogeneity. Third, mechanistic knowledge gaps hinder targeted therapy development, particularly regarding crosstalk between mitophagy and parallel cellular processes (e.g., apoptosis-inflammation networks).
Emerging strategies address these limitations through (1) Nanodelivery platforms (liposomes, polymeric nanoparticles) enhancing molecular stability and tissue specificity (2) Prodrug engineering via strategic chemical modifications to optimize pharmacokinetics (3) Rational combination regimens integrating mitophagy modulators with adjuvants like antioxidants to potentiate therapeutic synergism. Complementary approaches employing CRISPR/Cas9-based gene editing and multiomics profiling are elucidating fundamental mitophagy mechanisms to inform clinical development.
7 Conclusion and outlook
In recent years, mitophagy has gained significant attention as a critical process in the development of various diseases. Much of the focus has been on understanding the Pink1/Parkin- and receptor-dependent pathways, such as those involving BNIP3, NIX, and FUNDC1, which play essential roles in modulating mitophagy. Growing evidence supports the idea that modulating mitophagy through natural small molecules could offer promising therapeutic modalities for addressing DM and its associated complications. Nevertheless, establishing the precise modulation thresholds of mitophagy for therapeutic intervention in diabetes mellitus and its chronic complications persists as a critical unresolved challenge in translational endocrinology. Despite these advancements, several critical questions remain unresolved regarding the mechanisms of mitophagy and the modulation of mitochondrial homeostasis (1) While current methodologies for assessing mitochondrial autophagic flux—including TOM20 degradation assays, mito-QC fluorescent probes, and mt-Keima imaging—provide discrete measurements, the field critically lacks a unified platform capable of real-time dynamic monitoring with integrated quantification. Current protocols necessitate multimodal verification through complementary techniques (e.g., immunoblotting, flow cytometry, electron microscopy), yet exhibit model-dependent variability in sensitivity and specificity across experimental systems, significantly complicating mechanistic investigations and pharmacological development (2) Most studies on the regulation of mitophagy by natural small molecules have been conducted in animal and cellular models, with limited multicenter clinical research. Future studies should involve larger sample sizes and multicenter trials to validate these findings in humans (3) The specific mechanisms linking mitophagy to DM remain unclear. The signaling pathways and molecular targets involved in autophagy are complex, and there is a need for targeted, systematic investigation into the autophagy-related pathways in DM. (4) Poor bioavailability and stability of natural compounds. Lack of targeted delivery mechanisms, Potential off-target effects, and toxicity concerns. Moving forward, expanding the research scope to explore the molecular mechanisms of mitophagy in greater depth is critical. The development of small molecule drugs targeting mitophagy regulation remains a key challenge. Future investigations should prioritize four strategic directions: First, leveraging artificial intelligence and machine learning to accelerate nanocarrier optimization and bioactive compound discovery. AI-powered molecular docking platforms enable high-precision prediction of natural small molecule interactions with mitophagy-related proteins (e.g., PINK1/Parkin), streamlining lead compound identification. Second, integrative approaches combining CRISPR-Cas9 screening, multi-omics profiling, and high-content histological analysis will elucidate mitophagy regulatory networks, facilitating novel target discovery. Third, advanced pharmaceutical engineering strategies—including prodrug design and stimuli-responsive nanodelivery systems—require development to overcome pharmacokinetic limitations of natural compounds. Fourth, synergistic therapeutic platforms merging nanotechnology-enhanced natural molecules with conventional agents hold promise for amplified clinical efficacy through multimodal mechanisms. Addressing these questions is essential for advancing our understanding of mitophagy-related diseases, identifying new therapeutic targets, and facilitating the clinical translation of novel drugs. These endeavors will not only improve the management of DM and its associated complications but will also offer valuable perspectives for future fundamental research in this domain.
Author contributions
DY: Formal Analysis, Methodology, Software, Validation, Visualization, Writing–original draft, Writing–review and editing. JZ: Formal Analysis, Methodology, Software, Validation, Writing–original draft, Writing–review and editing. SS: Data curation, Investigation, Writing–original draft. YnY: Writing–original draft. JZ: Writing–original draft. YmY: Writing–original draft. CL: Writing–review and editing. XX: Writing–review and editing. QX: Funding acquisition, Project administration, Writing–original draft. RY: Conceptualization, Funding acquisition, Investigation, Project administration, Supervision, Writing–review and editing.
Funding
The author(s) declare that financial support was received for the research and/or publication of this article. This study was supported by The Key Support Project National Natural Science Foundation of China projects: The Key Support Project of the National Natural Science Foundation of China (U21A20411, T2341009), Hunan University of Chinese Medicine Disciplinary Construction’ Revealing the List and Appointing Leaders’ Project (22JBZ002), Hunan Provincial Natural Science Foundation Innovation Research Group Project (2024JJ1007), and the Key Project of Hunan Provincial Department of Education (24A0263).
Acknowledgments
We sincerely thank BioRender for the platform and tools used to create the figures in this work. Also, gratitude goes to PubChem for providing 3D molecular formula images that are crucial for our research.
Conflict of interest
The authors declare that the research was conducted in the absence of any commercial or financial relationships that could be construed as a potential conflict of interest.
Generative AI statement
The author(s) declare that no Generative AI was used in the creation of this manuscript.
Publisher’s note
All claims expressed in this article are solely those of the authors and do not necessarily represent those of their affiliated organizations, or those of the publisher, the editors and the reviewers. Any product that may be evaluated in this article, or claim that may be made by its manufacturer, is not guaranteed or endorsed by the publisher.
References
Ahmad, A. A., Draves, S. O., and Rosca, M. (2021). Mitochondria in diabetic kidney disease. Cells 10 (11), 2945. doi:10.3390/cells10112945
Ajoolabady, A., Chiong, M., Lavandero, S., Klionsky, D. J., and Ren, J. (2022). Mitophagy in cardiovascular diseases: molecular mechanisms, pathogenesis, and treatment. Trends Mol. Med. 28 (10), 836–849. doi:10.1016/j.molmed.2022.06.007
Alamdari, N., Aversa, Z., Castillero, E., Gurav, A., Petkova, V., Tizio, S., et al. (2012). Resveratrol prevents dexamethasone-induced expression of the muscle atrophy-related ubiquitin ligases atrogin-1 and MuRF1 in cultured myotubes through a SIRT1-dependent mechanism. Biochem. Biophysical Res. Commun. 417 (1), 528–533. doi:10.1016/j.bbrc.2011.11.154
Alami, M., Boumezough, K., Zerif, E., Zoubdane, N., Khalil, A., Bunt, T., et al. (2024). In vitro assessment of the neuroprotective effects of pomegranate (punica granatum L.) polyphenols against tau phosphorylation, neuroinflammation, and oxidative stress. Nutrients 16 (21), 3667. doi:10.3390/nu16213667
Aldrich, B. T., Schlötzer-Schrehardt, U., Skeie, J. M., Burckart, K. A., Schmidt, G. A., Reed, C. R., et al. (2017). Mitochondrial and morphologic alterations in native human corneal endothelial cells associated with diabetes mellitus. Invest Ophthalmol. Vis. Sci. 58 (4), 2130–2138. doi:10.1167/iovs.16-21094
Alonso-Castro, A. J., Zapata-Bustos, R., Gómez-Espinoza, G., and Salazar-Olivo, L. A. (2012). Isoorientin reverts TNF-α-induced insulin resistance in adipocytes activating the insulin signaling pathway. Endocrinology 153 (11), 5222–5230. doi:10.1210/en.2012-1290
Ao, H., Li, H., Zhao, X., Liu, B., and Lu, L. (2021). TXNIP positively regulates the autophagy and apoptosis in the rat müller cell of diabetic retinopathy. Life Sci. 267, 118988. doi:10.1016/j.lfs.2020.118988
Armstrong, D. G., Tan, T. W., Boulton, A. J. M., and Bus, S. A. (2023). Diabetic foot ulcers: a review. JAMA 330 (1), 62–75. doi:10.1001/jama.2023.10578
Bai, J., Liu, C., Zhu, P., and Li, Y. (2020). Novel insights into molecular mechanism of mitochondria in diabetic cardiomyopathy. Front. Physiol. 11, 609157. doi:10.3389/fphys.2020.609157
Bazyar, H., Zare Javid, A., Bavi Behbahani, H., Moradi, F., Moradi Poode, B., and Amiri, P. (2021). Consumption of melatonin supplement improves cardiovascular disease risk factors and anthropometric indices in type 2 diabetes mellitus patients: a double-blind, randomized, placebo-controlled trial. Trials 22 (1), 231. doi:10.1186/s13063-021-05174-z
Bellot, G., Garcia-Medina, R., Gounon, P., Chiche, J., Roux, D., Pouysségur, J., et al. (2009). Hypoxia-induced autophagy is mediated through hypoxia-inducible factor induction of BNIP3 and BNIP3L via their BH3 domains. Mol. Cell. Biol. 29 (10), 2570–2581. doi:10.1128/MCB.00166-09
Bennett, B. T., Mohamed, J. S., and Alway, S. E. (2013). Effects of resveratrol on the recovery of muscle mass following disuse in the plantaris muscle of aged rats. PLoS ONE 8 (12), e83518. doi:10.1371/journal.pone.0083518
Bhargava, P., and Schnellmann, R. G. (2017). Mitochondrial energetics in the kidney. Nat. Rev. Nephrol. 13 (10), 629–646. doi:10.1038/nrneph.2017.107
Bijak, M. (2017). Silybin, a major bioactive component of milk thistle (silybum marianum L. Gaernt.)-chemistry, bioavailability, and metabolism. Molecules 22 (11), 1942. doi:10.3390/molecules22111942
Blagov, A. V., Summerhill, V. I., Sukhorukov, V. N., Popov, M. A., Grechko, A. V., and Orekhov, A. N. (2023). Type 1 diabetes mellitus: inflammation, mitophagy, and mitochondrial function. Mitochondrion 72, 11–21. doi:10.1016/j.mito.2023.07.002
Brooks, C. D., Kodati, B., Stankowska, D. L., and Krishnamoorthy, R. R. (2023). Role of mitophagy in ocular neurodegeneration. Front. Neurosci. 17, 1299552. doi:10.3389/fnins.2023.1299552
Cai, X., Li, J., Wang, M., She, M., Tang, Y., Li, J., et al. (2017). GLP-1 treatment improves diabetic retinopathy by alleviating autophagy through GLP-1R-ERK1/2-HDAC6 signaling pathway. Int. J. Med. Sci. 14 (12), 1203–1212. doi:10.7150/ijms.20962
Cai, Y., Yang, E., Yao, X., Zhang, X., Wang, Q., Wang, Y., et al. (2021). FUNDC1-dependent mitophagy induced by tPA protects neurons against cerebral ischemia-reperfusion injury. Redox Biol. 38, 101792. doi:10.1016/j.redox.2020.101792
Cantó, C., Jiang, L. Q., Deshmukh, A. S., Mataki, C., Coste, A., Lagouge, M., et al. (2010). Interdependence of AMPK and SIRT1 for metabolic adaptation to fasting and exercise in skeletal muscle. Cell Metab. 11 (3), 213–219. doi:10.1016/j.cmet.2010.02.006
Carlos, D., Costa, F. R. C., Pereira, C. A., Rocha, F. A., Yaochite, J. N. U., Oliveira, G. G., et al. (2017). Mitochondrial DNA activates the NLRP3 inflammasome and predisposes to type 1 diabetes in murine model. Front. Immunol. 8, 164. doi:10.3389/fimmu.2017.00164
Čater, M., and Križančić Bombek, L. K. (2022). Protective role of mitochondrial uncoupling proteins against age-related oxidative stress in type 2 diabetes mellitus. Antioxidants 11 (8), 1473. doi:10.3390/antiox11081473
Cerdá, B., Cerón, J. J., Tomás-Barberán, F. A., and Espín, J. C. (2003). Repeated oral administration of high doses of the pomegranate ellagitannin punicalagin to rats for 37 Days is not toxic. J. Agric. Food Chem. 51 (11), 3493–3501. doi:10.1021/jf020842c
Casjm:yjlpca, F. A., Frnalpca, U. S., Gfsoplpc, S. A., Batfo:ljo, N. A., Lpofc, A. O., Aojsjnpca, O. E., et al. (2024). Method for preparing microcapsules with langerhans islets and microcapsule according to disclosed method. RU Patent RU0002822875
Chen, B. H., Huang, Y. C., Chang, Y. S., and He, T. Y. (2024). Composition for inhibiting diabetes mellitus and method of manufacturing the same. US Patent. US20240033313.
Chen, J., Chen, Y., Luo, Y., Gui, D., Huang, J., and He, D. (2014). Astragaloside IV ameliorates diabetic nephropathy involving protection of podocytes in streptozotocin induced diabetic rats. Eur. J. Pharmacol. 736, 86–94. doi:10.1016/j.ejphar.2014.04.037
Chen, K. H., Cheng, M. L., Jing, Y. H., Chiu, D. T. Y., Shiao, M. S., and Chen, J. K. (2011). Resveratrol ameliorates metabolic disorders and muscle wasting in streptozotocin-induced diabetic rats. Am. J. Physiology-Endocrinology Metabolism 301 (5), E853–E863. doi:10.1152/ajpendo.00048.2011
Chen, M., Chen, Z., Wang, Y., Tan, Z., Zhu, C., Li, Y., et al. (2016). Mitophagy receptor FUNDC1 regulates mitochondrial dynamics and mitophagy. Autophagy 12 (4), 689–702. doi:10.1080/15548627.2016.1151580
Chen, S., Zhu, Y., Xu, Q., Jiang, Q., Chen, D., Chen, T., et al. (2022a). Photocatalytic glucose depletion and hydrogen generation for diabetic wound healing. Nat. Commun. 13 (1), 5684. doi:10.1038/s41467-022-33475-7
Chen, X., Wang, L., Fan, S., Song, S., Min, H., Wu, Y., et al. (2018a). Puerarin acts on the skeletal muscle to improve insulin sensitivity in diabetic rats involving μ-opioid receptor. Eur. J. Pharmacol. 818, 115–123. doi:10.1016/j.ejphar.2017.10.033
Chen, X., Yi, L., Song, S., Wang, L., Liang, Q., Wang, Y., et al. (2018b). Puerarin attenuates palmitate-induced mitochondrial dysfunction, impaired mitophagy and inflammation in L6 myotubes. Life Sci. 206, 84–92. doi:10.1016/j.lfs.2018.05.041
Chen, Y. Y., Wu, T. T., Ho, C. Y., Yeh, T. C., Sun, G. C., Tseng, C. J., et al. (2022b). Blocking of SGLT2 to eliminate NADPH-induced oxidative stress in lenses of animals with fructose-induced diabetes mellitus. Int. J. Mol. Sci. 23 (13), 7142. doi:10.3390/ijms23137142
Chennupati, V. S., Ketan, G. B., Kumar Padarthi, P., Battu, H., Verma, V., Chavan, D. A., et al. (2023). Pharmaceutical composition for managing diabetes with berberine and gymnema sylvestre extracts. IN Patent. IN202311052461.
Christianson, S. W., Shultz, L. D., and Leiter, E. H. (1993). Adoptive transfer of diabetes into immunodeficient NOD-scid/scid mice. Relative contributions of CD4+ and CD8+ T-cells from diabetic versus prediabetic NOD.NON-thy-1a donors. Diabetes 42 (1), 44–55. doi:10.2337/diab.42.1.44
Cipolla-Neto, J., Amaral, F. G., Afeche, S. C., Tan, D. X., and Reiter, R. J. (2014). Melatonin, energy metabolism, and obesity: a review. J. Pineal Res. 56 (4), 371–381. doi:10.1111/jpi.12137
Clardy, J., and Walsh, C. (2004). Lessons from natural molecules. Nature 432 (7019), 829–837. doi:10.1038/nature03194
Cross, W. H. (2024). Metformin compositions and methods for treatment of diabetes. US patent US20240139231.
Cui, J., Wen, Z., Zhang, W., and Wu, W. (2022). Recent advances in oral peptide or protein-based drug liposomes. Pharm. (Basel) 15 (9), 1072. doi:10.3390/ph15091072
Dengler, F. (2020). Activation of AMPK under hypoxia: many roads leading to rome. Int. J. Mol. Sci. 21 (7), 2428. doi:10.3390/ijms21072428
Ding, X., Zhao, H., and Qiao, C. (2022). Icariin protects podocytes from NLRP3 activation by Sesn2-induced mitophagy through the Keap1-Nrf2/HO-1 axis in diabetic nephropathy. Phytomedicine 99, 154005. doi:10.1016/j.phymed.2022.154005
Dong, D, Wu, J, Sheng, L, Gong, X, Zhang, Z, and Yu, C. FUNDC1 Induces Apoptosis and Autophagy Under Oxidative Stress via PI3K/Akt/mTOR Pathway in Cataract Lens Cells. Curr Eye Res. 2022 Apr; 47 (4) 547–554. doi:10.1080/02713683.2021.2021586
Doğanlar, Z. B., Doğanlar, O., Kurtdere, K., Güçlü, H., Chasan, T., and Turgut, E. (2021). Melatonin prevents blood-retinal barrier breakdown and mitochondrial dysfunction in high glucose and hypoxia-induced in vitro diabetic macular edema model. Toxicol. Vitro 75, 105191. doi:10.1016/j.tiv.2021.105191
Du, M., Wen, G., Jin, J., Chen, Y., Cao, J., and Xu, A. (2018). Mangiferin prevents the growth of gastric carcinoma by blocking the PI3K-Akt signalling pathway. Anticancer Drugs 29 (2), 167–175. doi:10.1097/CAD.0000000000000583
Du, Q., Wu, X., Ma, K., Liu, W., Liu, P., Hayashi, T., et al. (2023). Silibinin alleviates ferroptosis of rat islet β cell INS-1 induced by the treatment with palmitic acid and high glucose through enhancing PINK1/parkin-mediated mitophagy. Archives Biochem. Biophysics 743, 109644. doi:10.1016/j.abb.2023.109644
Du, X., Chen, C., Zhang, M., Cai, D., Sun, J., Yang, J., et al. (2015). Scutellarin reduces endothelium dysfunction through the PKG-I pathway. Evidence-Based Complementary Altern. Med. 2015, 430271–430312. doi:10.1155/2015/430271
East, D. A., Fagiani, F., Crosby, J., Georgakopoulos, N. D., Bertrand, H., Schaap, M., et al. (2014). PMI: a ΔΨm independent pharmacological regulator of mitophagy. Chem. Biol. 21 (11), 1585–1596. doi:10.1016/j.chembiol.2014.09.019
Ebrahimpour, K. S., Gargari, B. P., Mobasseri, M., Valizadeh, H., and Asghari-Jafarabadi, M. (2015). Effects of silybum marianum (L.) gaertn. (silymarin) extract supplementation on antioxidant status and hs-CRP in patients with type 2 diabetes mellitus: a randomized, triple-blind, placebo-controlled clinical trial. Phytomedicine 22 (2), 290–296. doi:10.1016/j.phymed.2014.12.010
Elgizawy, H. A., Ali, A. A., and Hussein, M. A. (2021). Resveratrol: isolation, and its nanostructured lipid carriers, inhibits cell proliferation, induces cell apoptosis in certain human cell lines carcinoma and exerts protective effect against paraquat-induced hepatotoxicity. J. Med. Food 24 (1), 89–100. doi:10.1089/jmf.2019.0286
Fan, M., Lan, X., Wang, Q., Shan, M., Fang, X., Zhang, Y., et al. (2023). Renal function protection and the mechanism of ginsenosides: current progress and future perspectives. Front. Pharmacol. 14, 1070738. doi:10.3389/fphar.2023.1070738
Fatehi-Hassanabad, Z., Jafarzadeh, M., Tarhini, A., and Fatehi, M. (2005). The antihypertensive and vasodilator effects of aqueous extract fromBerberis vulgaris fruit on hypertensive rats. Phytother. Res. 19 (3), 222–225. doi:10.1002/ptr.1661
Feng, Y., Li, N. X., Yin, H. L., Chen, T. Y., Yang, Q., and Wu, M. (2019). Thermo- and pH-responsive, lipid-coated, mesoporous silica nanoparticle-based dual drug delivery system to improve the antitumor effect of hydrophobic drugs. Mol. Pharm. 16 (1), 422–436. doi:10.1021/acs.molpharmaceut.8b01073
Feng, Z., Xu, J., and Ni, C. (2020). Preparation of redox responsive modified xanthan gum nanoparticles and the drug controlled release. Int. J. Polym. Mater. Polym. Biomaterials 70 (14), 994–1001. doi:10.1080/00914037.2020.1767618
Fritsch, L. E., Moore, M. E., Sarraf, S. A., and Pickrell, A. M. (2020). Ubiquitin and receptor-dependent mitophagy pathways and their implication in neurodegeneration. J. Mol. Biol. 432 (8), 2510–2524. doi:10.1016/j.jmb.2019.10.015
Fu, Z., Jiao, Y., Wang, J., Zhang, Y., Shen, M., Reiter, R. J., et al. (2020). Cardioprotective role of melatonin in acute myocardial infarction. Front. Physiol. 11, 366. doi:10.3389/fphys.2020.00366
Gao, F., Chen, D., Si, J., Hu, Q., Qin, Z., Fang, M., et al. (2015). The mitochondrial protein BNIP3L is the substrate of PARK2 and mediates mitophagy in PINK1/PARK2 pathway. Hum. Mol. Genet. 24 (9), 2528–2538. doi:10.1093/hmg/ddv017
Gaur, R., Yadav, K. S., Verma, R. K., Yadav, N. P., and Bhakuni, R. S. (2014). In vivo anti-diabetic activity of derivatives of isoliquiritigenin and liquiritigenin. Phytomedicine 21 (4), 415–422. doi:10.1016/j.phymed.2013.10.015
Grabež, M., Škrbić, R., Stojiljković, M. P., Vučić, V., Rudić Grujić, V., Jakovljević, V., et al. (2022). A prospective, randomized, double-blind, placebo-controlled trial of polyphenols on the outcomes of inflammatory factors and oxidative stress in patients with type 2 diabetes mellitus. Rev. Cardiovasc Med. 23 (2), 57. doi:10.31083/j.rcm2302057
Gu, J., Cheng, Y., Wu, H., Kong, L., Wang, S., Xu, Z., et al. (2017). Metallothionein is downstream of Nrf2 and partially mediates sulforaphane prevention of diabetic cardiomyopathy. Diabetes 66 (2), 529–542. doi:10.2337/db15-1274
Gui, D., Guo, Y., Wang, F., Liu, W., Chen, J., Chen, Y., et al. (2012). Astragaloside IV, a novel antioxidant, prevents glucose-induced podocyte apoptosis in vitro and in vivo. PLoS One 7(6), e39824. doi:10.1371/journal.pone.0039824
Gui, D., Huang, J., Guo, Y., Chen, J., Chen, Y., Xiao, W., et al. (2013). Astragaloside IV ameliorates renal injury in streptozotocin-induced diabetic rats through inhibiting NF-κB-mediated inflammatory genes expression. Cytokine 61 (3), 970–977. doi:10.1016/j.cyto.2013.01.008
Guo, C., Xu, Y., Ma, Y., Xu, X., Peng, F., Li, H. H., et al. (2022). Individual and joint effects of trehalose and glutamate on diabetic retinopathy: a propensity score-matched case–control study. Endocr. Connect. 11 (2), e210474. doi:10.1530/EC-21-0474
Guo, H., and Ling, W. (2015). The update of anthocyanins on obesity and type 2 diabetes: experimental evidence and clinical perspectives. Rev. Endocr. Metab. Disord. 16 (1), 1–13. doi:10.1007/s11154-014-9302-z
Guo, H., Xiao, K., Zheng, Y., and Zong, J. (2024). Integrating bioinformatics and multiple machine learning to identify mitophagy-related targets for the diagnosis and treatment of diabetic foot ulcers: evidence from transcriptome analysis and drug docking. Front. Mol. Biosci. 11, 1420136. doi:10.3389/fmolb.2024.1420136
Guo, Y., Jiang, H., Wang, M., Ma, Y., Zhang, J., and Jing, L. (2023). Metformin alleviates cerebral ischemia/reperfusion injury aggravated by hyperglycemia via regulating AMPK/ULK1/PINK1/Parkin pathway-mediated mitophagy and apoptosis. Chemico-Biological Interact. 384, 110723. doi:10.1016/j.cbi.2023.110723
Habtemariam, S. (2020). Berberine pharmacology and the gut microbiota: a hidden therapeutic link. Pharmacol. Res. 155, 104722. doi:10.1016/j.phrs.2020.104722
Han, H., Hu, S., Hu, Y., Liu, D., Zhou, J., Liu, X., et al. (2023). Mitophagy in ototoxicity. Front. Cell Neurosci. 17, 1140916. doi:10.3389/fncel.2023.1140916
Han, Y., Jung, H. W., and Park, Y. K. (2015). Effects of Icariin on insulin resistance via the activation of AMPK pathway in C2C12 mouse muscle cells. Eur. J. Pharmacol. 758, 60–63. doi:10.1016/j.ejphar.2015.03.059
Handa, M., Beg, S., Shukla, R., Barkat, M. A., Choudhry, H., and Singh, K. K. (2021). Recent advances in lipid-engineered multifunctional nanophytomedicines for cancer targeting. J. Control Release 340, 48–59. doi:10.1016/j.jconrel.2021.10.025
Hang, W., He, B., Chen, J., Xia, L., Wen, B., Liang, T., et al. (2018). Berberine ameliorates high glucose-induced cardiomyocyte injury via AMPK signaling activation to stimulate mitochondrial biogenesis and restore autophagic flux. Front. Pharmacol. 9, 1121. doi:10.3389/fphar.2018.01121
Hanna, R. A., Quinsay, M. N., Orogo, A. M., Giang, K., Rikka, S., and Gustafsson, Å. B. (2012). Microtubule-associated protein 1 light chain 3 (LC3) interacts with Bnip3 protein to selectively remove endoplasmic reticulum and mitochondria via autophagy. J. Biol. Chem. 287 (23), 19094–19104. doi:10.1074/jbc.M111.322933
Harding, C. N., Lacore, T., Lowery, R., Underwood, B., Wells, S., and Wilson, J. (2023). Administration of berberine metabolites. AU Patent AU2023200826.
Hendgen-Cotta, U., Esfeld, S., Rudi, K., Miinalainen, I., Klare, J., and Rassaf, T. (2017). Cytosolic BNIP3 dimer interacts with mitochondrial BAX forming heterodimers in the mitochondrial outer membrane under basal conditions. IJMS 18 (4), 687. doi:10.3390/ijms18040687
Herman-Edelstein, M., Scherzer, P., Tobar, A., Levi, M., and Gafter, U. (2014). Altered renal lipid metabolism and renal lipid accumulation in human diabetic nephropathy. J. Lipid Res. 55 (3), 561–572. doi:10.1194/jlr.P040501
Hong, K. Q., Chen, L., Zhu, Z. H., Wang, Y. M., Yuan, Z. H., Wang, W., et al. (2023). Mechanism of cinnamaldehyde in promoting wound healing in diabetes rats via PINK1/Parkin-mediated mitochondrial autophagy. Chin. J. Exp. Traditional Med. Formulae (16), 134–143. doi:10.13422/j.cnki.syfjx.20230539
Hou, Y., Gu, D., Peng, J., Jiang, K., Li, Z., Shi, J., et al. (2020). Ginsenoside Rg1 regulates liver lipid factor metabolism in NAFLD model rats. ACS Omega 5 (19), 10878–10890. doi:10.1021/acsomega.0c00529
Hu, J., Kan, T., and Hu, X. (2019). Sirt3 regulates mitophagy level to promote diabetic corneal epithelial wound healing. Exp. Eye Res. 181, 223–231. doi:10.1016/j.exer.2019.02.011
Huang, R., Xu, Y., Wan, W., Shou, X., Qian, J., You, Z., et al. (2015). Deacetylation of nuclear LC3 drives autophagy initiation under starvation. Mol. Cell 57 (3), 456–466. doi:10.1016/j.molcel.2014.12.013
Huang, X., Wang, P., Li, T., Tian, X., Guo, W., Xu, B., et al. (2020). Self-assemblies based on traditional medicine berberine and cinnamic acid for adhesion-induced inhibition multidrug-resistant staphylococcus aureus. ACS Appl. Mater Interfaces 12 (1), 227–237. doi:10.1021/acsami.9b17722
Hwang, E. S., and Song, S. B. (2017). Nicotinamide is an inhibitor of SIRT1 in vitro, but can be a stimulator in cells. Cell Mol. Life Sci. 74 (18), 3347–3362. doi:10.1007/s00018-017-2527-8
Igarashi, R., Yamashita, S., Yamashita, T., Inoue, K., Fukuda, T., Fukuchi, T., et al. (2020). Gemcitabine induces Parkin-independent mitophagy through mitochondrial-resident E3 ligase MUL1-mediated stabilization of PINK1. Sci. Rep. 10 (1), 1465. doi:10.1038/s41598-020-58315-w
Imberechts, D., Kinnart, I., Wauters, F., Terbeek, J., Manders, L., Wierda, K., et al. (2022). DJ-1 is an essential downstream mediator in PINK1/parkin-dependent mitophagy. Brain 145 (12), 4368–4384. doi:10.1093/brain/awac313
Inoki, K., Li, Y., Xu, T., and Guan, K. L. (2003). Rheb GTPase is a direct target of TSC2 GAP activity and regulates mTOR signaling. Genes Dev. 17 (15), 1829–1834. doi:10.1101/gad.1110003
Jadoon, K. A., Ratcliffe, S. H., Barrett, D. A., Thomas, E. L., Stott, C., Bell, J. D., et al. (2016). Efficacy and safety of cannabidiol and tetrahydrocannabivarin on glycemic and lipid parameters in patients with type 2 diabetes: a randomized, double-blind, placebo-controlled, parallel group pilot study. Diabetes Care 39 (10), 1777–1786. doi:10.2337/dc16-0650
Jäger, S., Handschin, C., St-Pierre, J., and Spiegelman, B. M. (2007). AMP-activated protein kinase (AMPK) action in skeletal muscle via direct phosphorylation of PGC-1alpha. Proc. Natl. Acad. Sci. U. S. A. 104 (29), 12017–12022. doi:10.1073/pnas.0705070104
Jeffcoate, W. J., Vileikyte, L., Boyko, E. J., Armstrong, D. G., and Boulton, A. J. M. (2018). Current challenges and opportunities in the prevention and management of diabetic foot ulcers. Diabetes Care 41 (4), 645–652. doi:10.2337/dc17-1836
Ji, H., Zhao, Y., Ma, X., Wu, L., Guo, F., Huang, F., et al. (2024). Upregulation of UHRF1 promotes PINK1-mediated mitophagy to alleviates ferroptosis in diabetic nephropathy. Inflammation 47 (2), 718–732. doi:10.1007/s10753-023-01940-0
Jia, J. D., Jiang, W. G., Luo, X., Li, R. R., Zhao, Y. C., Tian, G., et al. (2021). Vascular endothelial growth factor B inhibits insulin secretion in MIN6 cells and reduces Ca2+ and cyclic adenosine monophosphate levels through PI3K/AKT pathway. WJD 12 (4), 480–498. doi:10.4239/wjd.v12.i4.480
Jia, P., Ji, S., Zhang, H., Chen, Y., and Wang, T. (2020). Piceatannol ameliorates hepatic oxidative damage and mitochondrial dysfunction of weaned piglets challenged with diquat. Animals 10 (7), 1239. doi:10.3390/ani10071239
Jiang, W., Si, L., Li, P., Bai, B., Qu, J., Hou, B., et al. (2018). Serum metabonomics study on antidiabetic effects of fenugreek flavonoids in streptozotocin-induced rats. J. Chromatogr. B 1092, 466–472. doi:10.1016/j.jchromb.2018.06.041
Jiao, Y., Wang, J. H., Li, Y., Shen, J., Hou, X. L., Shen, M. Z., et al. (2022). Melatonin ameliorates myocardial injury after myocardial infarction with diabetes mellitus by mediating mitophagy. Chin. J. Mult. Organ Dis. Elder. (3), 208–215. doi:10.11915/jissn.1671-5403.2022.03.045
Jin, J., Lee, S., Taweechaipaisankul, A., Kim, G. A., and Lee, B. C. (2017). Melatonin regulates lipid metabolism in porcine oocytes. J. Pineal Res. 62 (2), e12388. doi:10.1111/jpi.12388
Johanningsmeier, S. D., and Harris, G. K. (2011). Pomegranate as a functional food and nutraceutical source. Annu. Rev. Food Sci. Technol. 2 (1), 181–201. doi:10.1146/annurev-food-030810-153709
Joseph, A. M., Malamo, A. G., Silvestre, J., Wawrzyniak, N., Carey-Love, S., Nguyen, L. M. D., et al. (2013). Short-term caloric restriction, resveratrol, or combined treatment regimens initiated in late-life alter mitochondrial protein expression profiles in a fiber-type specific manner in aged animals. Exp. Gerontol. 48 (9), 858–868. doi:10.1016/j.exger.2013.05.061
Juère, E., Florek, J., Bouchoucha, M., Jambhrunkar, S., Wong, K. Y., Popat, A., et al. (2017). In vitro dissolution, cellular membrane permeability, and anti-inflammatory response of resveratrol-encapsulated mesoporous silica nanoparticles. Mol. Pharm. 14 (12), 4431–4441. doi:10.1021/acs.molpharmaceut.7b00529
Kaniuka, O., Deregowska, A., Bandura, Y., Sabadashka, M., Chala, D., Kulachkovskyi, O., et al. (2025). Upregulation of GRP78 is accompanied by decreased antioxidant response and mitophagy promotion in streptozotocin-induced type 1 diabetes in rats. Biochimica Biophysica Acta (BBA) - Mol. Basis Dis. 1871 (1), 167531. doi:10.1016/j.bbadis.2024.167531
Kapoor, R., Singh, S., Tripathi, M., Bhatnagar, P., Kakkar, P., and Gupta, K. C. (2014). O-Hexadecyl-Dextran entrapped berberine nanoparticles abrogate high glucose stress induced apoptosis in primary rat hepatocytes. PLoS One 9 (2), e89124. doi:10.1371/journal.pone.0089124
Kasangana, P. B., Eid, H. M., Nachar, A., Stevanovic, T., and Haddad, P. S. (2019). Further isolation and identification of anti-diabetic principles from root bark of Myrianthus arboreus P. Beauv.: the ethyl acetate fraction contains bioactive phenolic compounds that improve liver cell glucose homeostasis. J. Ethnopharmacol. 245, 112167. doi:10.1016/j.jep.2019.112167
Ke, P. Y. (2020). Mitophagy in the pathogenesis of liver diseases. Cells 9 (4), 831. doi:10.3390/cells9040831
Khaminets, A., Behl, C., and Dikic, I. (2016). Ubiquitin-dependent and independent signals in selective autophagy. Trends Cell Biol. 26 (1), 6–16. doi:10.1016/j.tcb.2015.08.010
Khan, I., Preeti, K., Kumar, R., Khatri, D. K., and Singh, S. B. (2024). Activation of SIRT1 by silibinin improved mitochondrial health and alleviated the oxidative damage in experimental diabetic neuropathy and high glucose-mediated neurotoxicity. Archives Physiology Biochem. 130 (4), 420–436. doi:10.1080/13813455.2022.2108454
Khan, I., Preeti, K., Kumar, R., Kumar Khatri, D., and Bala Singh, S. (2023). Piceatannol promotes neuroprotection by inducing mitophagy and mitobiogenesis in the experimental diabetic peripheral neuropathy and hyperglycemia-induced neurotoxicity. Int. Immunopharmacol. 116, 109793. doi:10.1016/j.intimp.2023.109793
Kim, J., Kim, Y. C., Fang, C., Russell, R. C., Kim, J. H., Fan, W., et al. (2013). Differential regulation of distinct Vps34 complexes by AMPK in nutrient stress and autophagy. Cell 152 (1–2), 290–303. doi:10.1016/j.cell.2012.12.016
Ko, Y. S., Jin, H., Park, S. W., and Kim, H. J. (2020). Salvianolic acid B protects against oxLDL-induced endothelial dysfunction under high-glucose conditions by downregulating ROCK1-mediated mitophagy and apoptosis. Biochem. Pharmacol. 174, 113815. doi:10.1016/j.bcp.2020.113815
Kokona, D., Georgiou, P. C., Kounenidakis, M., Kiagiadaki, F., and Thermos, K. (2016). Endogenous and synthetic cannabinoids as therapeutics in retinal disease. Neural Plast. 2016, 8373020–8373112. doi:10.1155/2016/8373020
Kong, Z., Che, K., Hu, J., Chen, Y., Wang, Y., Wang, X., et al. (2020). Orientin protects podocytes from high glucose induced apoptosis through mitophagy. Chem. and Biodivers. 17 (3), e1900647. doi:10.1002/cbdv.201900647
Kong, Z., Xiao, M., Wang, B., Zhang, W., Che, K., Lv, W., et al. (2023). Renoprotective effect of isoorientin in diabetic nephropathy via activating autophagy and inhibiting the PI3K-AKT-TSC2-mTOR pathway. Am. J. Chin. Med. 51 (05), 1269–1291. doi:10.1142/S0192415X23500581
Koon, C. M., Fong, S., Wat, E., Wang, Y. P., Wing-Shing Cheung, D., Bik-San Lau, C., et al. (2014). Mechanisms of the dilator action of the Erigerontis Herba on rat aorta. J. Ethnopharmacol. 155 (3), 1561–1567. doi:10.1016/j.jep.2014.07.053
Kumar, A., Ekavali, null, Chopra, K., Mukherjee, M., Pottabathini, R., and Dhull, D. K. (2015). Current knowledge and pharmacological profile of berberine: an update. Eur. J. Pharmacol. 761, 288–297. doi:10.1016/j.ejphar.2015.05.068
Kwon, G. T., Cho, H. J., Chung, W. Y., Park, K. K., Moon, A., and Park, J. H. Y. (2009). Isoliquiritigenin inhibits migration and invasion of prostate cancer cells: possible mediation by decreased JNK/AP-1 signaling. J. Nutr. Biochem. 20 (9), 663–676. doi:10.1016/j.jnutbio.2008.06.005
Lacombe, A., and Scorrano, L. (2024). The interplay between mitochondrial dynamics and autophagy: from a key homeostatic mechanism to a driver of pathology. Seminars Cell and Dev. Biol. 161–162, 1–19. doi:10.1016/j.semcdb.2024.02.001
Lan, R., Wu, J. T., Wu, T., Ma, Y. Z., Wang, B. Q., Zheng, H. Z., et al. (2018). Mitophagy is activated in brain damage induced by cerebral ischemia and reperfusion via the PINK1/Parkin/p62 signalling pathway. Brain Res. Bull. 142, 63–77. doi:10.1016/j.brainresbull.2018.06.018
Lawal, A. O., Davids, L. M., and Marnewick, J. L. (2019). Rooibos (Aspalathus linearis) and honeybush (Cyclopia species) modulate the oxidative stress associated injury of diesel exhaust particles in human umbilical vein endothelial cells. Phytomedicine 59, 152898. doi:10.1016/j.phymed.2019.152898
Lee, S. Y., Tsai, W. C., Lin, J. C., Ahmetaj-Shala, B., Huang, S. F., Chang, W. L., et al. (2017). Astragaloside II promotes intestinal epithelial repair by enhancing L-arginine uptake and activating the mTOR pathway. Sci. Rep. 7 (1), 12302. doi:10.1038/s41598-017-12435-y
Leech, T., Chattipakorn, N., and Chattipakorn, S. C. (2019). The beneficial roles of metformin on the brain with cerebral ischaemia/reperfusion injury. Pharmacol. Res. 146, 104261. doi:10.1016/j.phrs.2019.104261
Lemasters, J. J. (2005). Selective mitochondrial autophagy, or mitophagy, as a targeted defense against oxidative stress, mitochondrial dysfunction, and aging. Rejuvenation Res. 8 (1), 3–5. doi:10.1089/rej.2005.8.3
Li, J., Yang, D., Li, Z., Zhao, M., Wang, D., Sun, Z., et al. (2023b). PINK1/Parkin-mediated mitophagy in neurodegenerative diseases. Ageing Res. Rev. 84, 101817. doi:10.1016/j.arr.2022.101817
Li, L. (2025). Berberine salts, ursodeoxycholic salts and combinations. Methods Prep. Appl. Thereof. US20250002490.
Li, L., Hou, X., Xu, R., Liu, C., and Tu, M. (2017a). Research review on the pharmacological effects of astragaloside IV. Fundamemntal Clin. Pharma 31 (1), 17–36. doi:10.1111/fcp.12232
Li, L. (2023). Pharmaceutical composition comprising berberine and ursodeoxycholic acid for the treatment of various diseases or disorders. US Patent US20230295151.
Li, M., She, J., Ma, L., Ma, L., Ma, X., and Zhai, J. (2022). Berberine protects against palmitate induced beta cell injury via promoting mitophagy. Genes Genom 44 (7), 867–878. doi:10.1007/s13258-022-01250-z
Li, X, Li, S, Ma, C, Li, T, and Yang, L (2022). Preparation of baicalin-loaded ligand-modified nanoparticles for nose-to-brain delivery for neuroprotection in cerebral ischemia. Drug Deliv. 29 (1), 1282–1298. doi:10.1080/10717544.2022.2064564
Li, R., Du, J., Yao, Y., Yao, G., and Wang, X. (2019a). Adiponectin inhibits high glucose-induced angiogenesis via inhibiting autophagy in RF/6A cells. J. Cell. Physiology 234 (11), 20566–20576. doi:10.1002/jcp.28659
Li, S., Luo, S., Chen, H., Zheng, Y., Lin, L., Yao, H., et al. (2019b). Protective effects of five compounds from Livistona chinensis R. Brown leaves against hypoxia/reoxygenation, H2O2, or adriamycin-induced injury in H9c2 cells. DDDT 13, 1555–1566. doi:10.2147/DDDT.S201816
Li, S., Sun, X., Xu, L., Sun, R., Ma, Z., Deng, X., et al. (2017b). Baicalin attenuates in vivo and in vitro hyperglycemia-exacerbated ischemia/reperfusion injury by regulating mitochondrial function in a manner dependent on AMPK. Eur. J. Pharmacol. 815, 118–126. doi:10.1016/j.ejphar.2017.07.041
Li, W., Li, H., Zheng, L., Xia, J., Yang, X., Men, S., et al. (2023a). Ginsenoside CK improves skeletal muscle insulin resistance by activating DRP1/PINK1-mediated mitophagy. Food Funct. 14 (2), 1024–1036. doi:10.1039/d2fo02026b
Li, X., Sun, M., and Long, Y. (2020a). Cyanidin-3-O-Glucoside attenuates lipopolysaccharide-induced inflammation in human corneal epithelial cells by inducing let-7b-5p-mediated HMGA2/PI3K/akt pathway. Inflammation 43 (3), 1088–1096. doi:10.1007/s10753-020-01194-0
Li, Y., Ding, X., Li, H., and Zhang, C. (2013). Icariin attenuates high glucose-induced type IV collagen and fibronectin accumulation in glomerular mesangial cells by inhibiting transforming growth factor-β production and signalling through G protein-coupled oestrogen receptor 1. Clin. Exp. Pharma Physio 40 (9), 635–643. doi:10.1111/1440-1681.12143
Li, Y., Wang, Y., Kim, E., Beemiller, P., Wang, C. Y., Swanson, J., et al. (2007). Bnip3 mediates the hypoxia-induced inhibition on mammalian target of rapamycin by interacting with rheb. J. Biol. Chem. 282 (49), 35803–35813. doi:10.1074/jbc.M705231200
Li, Z., Guo, H., Li, J., Ma, T., Zhou, S., Zhang, Z., et al. (2020b). Sulforaphane prevents type 2 diabetes-induced nephropathy via AMPK-mediated activation of lipid metabolic pathways and Nrf2 antioxidative function. Clin. Sci. (Lond). 134 (18), 2469–2487. doi:10.1042/CS20191088
Lian, T., and Ho, R. J. (2001). Trends and developments in liposome drug delivery systems. J. Pharm. Sci. 90 (6), 667–680. doi:10.1002/jps.1023
Liang, Y., He, J., and Guo, B. (2021). Functional hydrogels as wound dressing to enhance wound healing. ACS Nano 15 (8), 12687–12722. doi:10.1021/acsnano.1c04206
Liu, C., Cao, Q., Chen, Y., Chen, X., Zhu, Y., Zhang, Z., et al. (2023b). Rhein protects retinal Müller cells from high glucose-induced injury via activating the AMPK/Sirt1/PGC-1α pathway. J. Recept. Signal Transduct. 43 (2), 62–71. doi:10.1080/10799893.2023.2223319
Liu, C. C., Ji, J. L., Wang, Z., Zhang, X. J., Ding, L., Zhang, Y., et al. (2024). TRPC6-Calpain-1 Axis promotes tubulointerstitial inflammation by inhibiting mitophagy in diabetic kidney disease. Kidney Int. Rep. 9 (11), 3301–3317. doi:10.1016/j.ekir.2024.08.019
Liu, K., Zhang, J. X., and Liu, L. P. (2024). Pharmaceutical combinations and compositions, and methods of use thereof, WO Patent WO2024141073.
Liu, L. (2023). Composition, and application and pharmaceutical preparation thereof. AU Patent AU2023200123.
Liu, L., Feng, D., Chen, G., Chen, M., Zheng, Q., Song, P., et al. (2012). Mitochondrial outer-membrane protein FUNDC1 mediates hypoxia-induced mitophagy in mammalian cells. Nat. Cell Biol. 14 (2), 177–185. doi:10.1038/ncb2422
Liu, N., Wu, J., Zhang, L., Gao, Z., Sun, Y., Yu, M., et al. (2017a). Hydrogen Sulphide modulating mitochondrial morphology to promote mitophagy in endothelial cells under high-glucose and high-palmitate. J. Cell. Mol. Medi 21 (12), 3190–3203. doi:10.1111/jcmm.13223
Liu, T., Ma, X., Ouyang, T., Chen, H., Xiao, Y., Huang, Y., et al. (2019a). Efficacy of 5-aminolevulinic acid–based photodynamic therapy against keloid compromised by downregulation of SIRT1-SIRT3-SOD2-mROS dependent autophagy pathway. Redox Biol. 20, 195–203. doi:10.1016/j.redox.2018.10.011
Liu, T., Zhu, H., Ge, M., Pan, Z., Zeng, Y., Leng, Y., et al. (2023a). GPD1L inhibits renal cell carcinoma progression by regulating PINK1/Parkin-mediated mitophagy. J. Cell. Mol. Medi 27 (16), 2328–2339. doi:10.1111/jcmm.17813
Liu, X., Wang, W., Song, G., Wei, X., Zeng, Y., Han, P., et al. (2017b). Astragaloside IV ameliorates diabetic nephropathy by modulating the mitochondrial quality control network. PLoS One 12(8), e0182558. doi:10.1371/journal.pone.0182558
Liu, X., Zhao, X., Cheng, R., and Huang, Y. (2020). Autophagy attenuates high glucose-induced oxidative injury to lens epithelial cells. Biosci. Rep. 40 (4), BSR20193006. doi:10.1042/BSR20193006
Liu, Y., Deng, J., and Fan, D. (2019b). Ginsenoside Rk3 ameliorates high-fat-diet/streptozocin induced type 2 diabetes mellitus in mice via the AMPK/Akt signaling pathway. Food Funct. 10 (5), 2538–2551. doi:10.1039/c9fo00095j
Lu, Y., Li, Z., Zhang, S., Zhang, T., Liu, Y., and Zhang, L. (2023). Cellular mitophagy: mechanism, roles in diseases and small molecule pharmacological regulation. Theranostics 13 (2), 736–766. doi:10.7150/thno.79876
Lu, Y., Lu, Y., Meng, J., and Wang, Z. (2021). Pyroptosis and its regulation in diabetic cardiomyopathy. Front. Physiol. 12, 791848. doi:10.3389/fphys.2021.791848
Luo, Y., Guo, Q., Liu, C., Zheng, Y., Wang, Y., and Wang, B. (2024). Adipose mesenchymal stem cell-derived extracellular vesicles regulate PINK1/parkin-mediated mitophagy to repair high glucose-induced dermal fibroblast senescence and promote wound healing in rats with diabetic foot ulcer. Acta Diabetol. doi:10.1007/s00592-024-02422-x
Lyamzaev, K. G., Tokarchuk, A. V., Panteleeva, A. A., Mulkidjanian, A. Y., Skulachev, V. P., and Chernyak, B. V. (2018). Induction of autophagy by depolarization of mitochondria. Autophagy 14 (5), 921–924. doi:10.1080/15548627.2018.1436937
Ma, N., and Zhang, Y. (2022). Effects of resveratrol therapy on glucose metabolism, insulin resistance, inflammation, and renal function in the elderly patients with type 2 diabetes mellitus: a randomized controlled clinical trial protocol. Med. Baltim. 101 (32), e30049. doi:10.1097/MD.0000000000030049
Magalhaes-Novais, S., Blecha, J., Naraine, R., Mikesova, J., Abaffy, P., Pecinova, A., et al. (2022). Mitochondrial respiration supports autophagy to provide stress resistance during quiescence. Autophagy 18 (10), 2409–2426. doi:10.1080/15548627.2022.2038898
Mahjabeen, W., Khan, D. A., and Mirza, S. A. (2022). Role of resveratrol supplementation in regulation of glucose hemostasis, inflammation and oxidative stress in patients with diabetes mellitus type 2: a randomized, placebo-controlled trial. Complement. Ther. Med. 66, 102819. doi:10.1016/j.ctim.2022.102819
Mahmoudi, N., Kiasalari, Z., Rahmani, T., Sanaierad, A., Afshin-Majd, S., Naderi, G., et al. (2021). Diosgenin attenuates cognitive impairment in streptozotocin-induced diabetic rats: underlying mechanisms. Neuropsychobiology 80 (1), 25–35. doi:10.1159/000507398
Malik, A., Jamil, U., Butt, T. T., Waquar, S., Gan, S. H., Shafique, H., et al. (2019). In silico and in vitro studies of lupeol and iso-orientin as potential antidiabetic agents in a rat model. DDDT 13, 1501–1513. doi:10.2147/DDDT.S176698
Marinković, M., Šprung, M., and Novak, I. (2021). Dimerization of mitophagy receptor BNIP3L/NIX is essential for recruitment of autophagic machinery. Autophagy 17 (5), 1232–1243. doi:10.1080/15548627.2020.1755120
Martorina, W., and Tavares, A. (2023). Glycemic variability in patients with type 2 diabetes mellitus (T2DM): the role of melatonin in a crossover, double-blind, placebo-controlled, randomized study. Nutrients 15 (16), 3523. doi:10.3390/nu15163523
Melser, S., Chatelain, E. H., Lavie, J., Mahfouf, W., Jose, C., Obre, E., et al. (2013). Rheb regulates mitophagy induced by mitochondrial energetic status. Cell Metab. 17 (5), 719–730. doi:10.1016/j.cmet.2013.03.014
Mills, E. L., Kelly, B., and O’Neill, L. A. J. (2017). Mitochondria are the powerhouses of immunity. Nat. Immunol. 18 (5), 488–498. doi:10.1038/ni.3704
Miyamoto, Y., Kitamura, N., Nakamura, Y., Futamura, M., Miyamoto, T., Yoshida, M., et al. (2011). “Possible existence of lysosome-like organella within mitochondria and its role in mitochondrial quality control,”. Editor J. Santos, 6. doi:10.1371/journal.pone.0016054
Mo, J., Yang, R., Li, F., Zhang, X., He, B., Zhang, Y., et al. (2018). Scutellarin protects against vascular endothelial dysfunction and prevents atherosclerosis via antioxidation. Phytomedicine 42, 66–74. doi:10.1016/j.phymed.2018.03.021
Momken, I., Stevens, L., Bergouignan, A., Desplanches, D., Rudwill, F., Chery, I., et al. (2011). Resveratrol prevents the wasting disorders of mechanical unloading by acting as a physical exercise mimetic in the rat. FASEB J. 25 (10), 3646–3660. doi:10.1096/fj.10-177295
Mu, J., Zhang, D., Tian, Y., Xie, Z., and Zou, M. H. (2020). BRD4 inhibition by JQ1 prevents high-fat diet-induced diabetic cardiomyopathy by activating PINK1/parkin-mediated mitophagy in vivo. J. Mol. Cell Cardiol. 149, 1–14. doi:10.1016/j.yjmcc.2020.09.003
Mukherjee, R., and Chakrabarti, O. (2016). Ubiquitin mediated regulation of the E3 ligase GP78 by Mahogunin in trans affects mitochondrial homeostasis. J. Cell Sci. 129 (4), 176537. doi:10.1242/jcs.176537
Nag, S., Mitra, O., Maturi, B., Kaur, S. P., Saini, A., Nama, M., et al. (2024). Autophagy and mitophagy as potential therapeutic targets in diabetic heart condition: harnessing the power of nanotheranostics. Asian J. Pharm. Sci. 19 (3), 100927. doi:10.1016/j.ajps.2024.100927
Nakamura, Y., Kitamura, N., Shinogi, D., Yoshida, M., Goda, O., Murai, R., et al. (2012). BNIP3 and NIX mediate mieap-induced accumulation of lysosomal proteins within mitochondria. PLoS One 7(1), e30767. doi:10.1371/journal.pone.0030767
Namkoong, S., Sung, J., Yang, J., Choi, Y., Jeong, H. S., and Lee, J. (2017). Nobiletin attenuates the inflammatory response through heme oxygenase-1 induction in the crosstalk between adipocytes and macrophages. J. Med. Food 20 (9), 873–881. doi:10.1089/jmf.2017.3921
Neag, M. A., Mocan, A., Echeverría, J., Pop, R. M., Bocsan, C. I., Crişan, G., et al. (2018). Berberine: botanical occurrence, traditional uses, extraction methods, and relevance in cardiovascular, metabolic, hepatic, and renal disorders. Front. Pharmacol. 9, 557. doi:10.3389/fphar.2018.00557
Nhu, N. T., Li, Q., Liu, Y., Xu, J., Xiao, S. Y., and Lee, S. D. (2021). Effects of mdivi-1 on neural mitochondrial dysfunction and mitochondria-mediated apoptosis in ischemia-reperfusion injury after stroke: a systematic review of preclinical studies. Front. Mol. Neurosci. 14, 778569. doi:10.3389/fnmol.2021.778569
Nohara, K., Mallampalli, V., Nemkov, T., Wirianto, M., Yang, J., Ye, Y., et al. (2019). Nobiletin fortifies mitochondrial respiration in skeletal muscle to promote healthy aging against metabolic challenge. Nat. Commun. 10 (1), 3923. doi:10.1038/s41467-019-11926-y
Ochi, M. M., Amoabediny, G., Rezayat, S. M., Akbarzadeh, A., and Ebrahimi, B. (2016). In vitro co-delivery evaluation of novel pegylated nano-liposomal herbal drugs of silibinin and glycyrrhizic acid (nano-phytosome) to hepatocellular carcinoma cells. Cell J. 18 (2), 135–148. doi:10.22074/cellj.2016.4308
Oh, J. M., and Chun, S. (2022). Ginsenoside CK inhibits the early stage of adipogenesis via the AMPK, MAPK, and AKT signaling pathways. Antioxidants 11 (10), 1890. doi:10.3390/antiox11101890
Oh, S. J., Park, K., Sonn, S. K., Oh, G. T., and Lee, M. S. (2023). Pancreatic β-cell mitophagy as an adaptive response to metabolic stress and the underlying mechanism that involves lysosomal Ca2+ release. Exp. Mol. Med. 55 (9), 1922–1932. doi:10.1038/s12276-023-01055-4
Olcese, J. M. (2020). Melatonin and female reproduction: an expanding universe. Front. Endocrinol. 11, 85. doi:10.3389/fendo.2020.00085
Onishi, M., Yamano, K., Sato, M., Matsuda, N., and Okamoto, K. (2021). Molecular mechanisms and physiological functions of mitophagy. EMBO J. 40 (3), e104705. doi:10.15252/embj.2020104705
Onphachanh, X., Lee, H. J., Lim, J. R., Jung, Y. H., Kim, J. S., Chae, C. W., et al. (2017). Enhancement of high glucose-induced PINK1 expression by melatonin stimulates neuronal cell survival: involvement of MT2/Akt/NF-κB pathway. J. Pineal Res. 63 (2), e12427. doi:10.1111/jpi.12427
Ortholand, J. Y., and Ganesan, A. (2004). Natural products and combinatorial chemistry: back to the future. Curr. Opin. Chem. Biol. 8 (3), 271–280. doi:10.1016/j.cbpa.2004.04.011
Ostadmohammadi, V., Soleimani, A., Bahmani, F., Aghadavod, E., Ramezani, R., Reiter, R. J., et al. (2020). The effects of melatonin supplementation on parameters of mental health, glycemic control, markers of cardiometabolic risk, and oxidative stress in diabetic hemodialysis patients: a randomized, double-blind, placebo-controlled trial. J. Ren. Nutr. 30 (3), 242–250. doi:10.1053/j.jrn.2019.08.003
Paik, S., Song, G. Y., and Jo, E. K. (2023). Ginsenosides for therapeutically targeting inflammation through modulation of oxidative stress. Int. Immunopharmacol. 121, 110461. doi:10.1016/j.intimp.2023.110461
Palikaras, K., Lionaki, E., and Tavernarakis, N. (2018). Mechanisms of mitophagy in cellular homeostasis, physiology and pathology. Nat. Cell Biol. 20 (9), 1013–1022. doi:10.1038/s41556-018-0176-2
Pan, T. L., Wang, P. W., Hung, C. F., Aljuffali, I. A., Dai, Y. S., and Fang, J. Y. (2016). The impact of retinol loading and surface charge on the hepatic delivery of lipid nanoparticles. Colloids Surf. B Biointerfaces 141, 584–594. doi:10.1016/j.colsurfb.2016.02.029
Panigrahi, A., and Mohanty, S. (2023). Efficacy and safety of HIMABERB® berberine on glycemic control in patients with prediabetes: double-blind, placebo-controlled, and randomized pilot trial. BMC Endocr. Disord. 23 (1), 190. doi:10.1186/s12902-023-01442-y
Park, K., Sonn, S. K., Seo, S., Kim, J., Hur, K. Y., Oh, G. T., et al. (2023). Impaired TFEB activation and mitophagy as a cause of PPP3/calcineurin inhibitor-induced pancreatic β-cell dysfunction. Autophagy 19 (5), 1444–1458. doi:10.1080/15548627.2022.2132686
Patel, K. R., Scott, E., Brown, V. A., Gescher, A. J., Steward, W. P., and Brown, K. (2011). Clinical trials of resveratrol. Ann. N. Y. Acad. Sci. 1215, 161–169. doi:10.1111/j.1749-6632.2010.05853.x
Paul, S., Saha, D., and Bk, B. (2021). Mitochondrial dysfunction and mitophagy closely cooperate in neurological deficits associated with alzheimer’s disease and type 2 diabetes. Mol. Neurobiol. 58 (8), 3677–3691. doi:10.1007/s12035-021-02365-2
Peng, W., Peng, F., Lou, Y., Li, Y., Zhao, N., Shao, Q., et al. (2021). Autophagy alleviates mitochondrial DAMP-induced acute lung injury by inhibiting NLRP3 inflammasome. Life Sci. 265, 118833. doi:10.1016/j.lfs.2020.118833
Picca, A., Faitg, J., Auwerx, J., Ferrucci, L., and D’Amico, D. (2023). Mitophagy in human health, ageing and disease. Nat. Metab. 5 (12), 2047–2061. doi:10.1038/s42255-023-00930-8
Potue, P., Wunpathe, C., Maneesai, P., Kukongviriyapan, U., Prachaney, P., and Pakdeechote, P. (2019). Nobiletin alleviates vascular alterations through modulation of Nrf-2/HO-1 and MMP pathways in l -NAME induced hypertensive rats. Food Funct. 10 (4), 1880–1892. doi:10.1039/c8fo02408a
Qi, L., Mao, H., Lu, X., Shi, T., and Wang, J. (2021b). Cinnamaldehyde promotes the intestinal barrier functions and reshapes gut microbiome in early weaned rats. Front. Nutr. 8, 748503. doi:10.3389/fnut.2021.748503
Qi, M. Y., He, Y. H., Cheng, Y., Fang, Q., Ma, R. Y., Zhou, S. J., et al. (2021a). Icariin ameliorates streptozocin-induced diabetic nephropathy through suppressing the TLR4/NF-κB signal pathway. Food Funct. 12 (3), 1241–1251. doi:10.1039/d0fo02335c
Qiao, C., Wan, J., Zhang, L., Luo, B., Liu, P., Di, A., et al. (2019). Astragaloside II alleviates the symptoms of experimental ulcerative colitis in vitro and in vivo. Am. J. Transl. Res. 11 (11), 7074–7083.
Qiao, C., Ye, W., Li, S., Wang, H., and Ding, X. (2018). Icariin modulates mitochondrial function and apoptosis in high glucose-induced glomerular podocytes through G protein-coupled estrogen receptors. Mol. Cell. Endocrinol. 473, 146–155. doi:10.1016/j.mce.2018.01.014
Qiu, C., Zhang, J. Z., Wu, B., Xu, C. C., Pang, H. H., Tu, Q. C., et al. (2023). Advanced application of nanotechnology in active constituents of traditional Chinese medicines. J. Nanobiotechnology 21 (1), 456. doi:10.1186/s12951-023-02165-x
Rahman, M. S., and Kim, Y. S. (2020). PINK1–PRKN mitophagy suppression by mangiferin promotes a brown-fat-phenotype via PKA-p38 MAPK signalling in murine C3H10T1/2 mesenchymal stem cells. Metabolism 107, 154228. doi:10.1016/j.metabol.2020.154228
Ramachandran, V., Mohanasundaram, T., Karunakaran, D., Gunasekaran, M., and Tiwari, R. (2023). Physiological and pathophysiological aspects of diabetic foot ulcer andits treatment strategies. CDR 19 (8), e031122210617. doi:10.2174/1573399819666221103141715
Ramírez, N. M., Toledo, R. C. L., Moreira, M. E. C., Martino, H. S. D., Benjamin, L. D. A., De Queiroz, J. H., et al. (2017). Anti-obesity effects of tea from Mangifera indica L. leaves of the Ubá variety in high-fat diet-induced obese rats. Biomed. and Pharmacother. 91, 938–945. doi:10.1016/j.biopha.2017.05.015
Ramirez, A, Old, W, Selwood, DL, and Liu, X. Cannabidiol activates PINK1-Parkin-dependent mitophagy and mitochondrial-derived vesicles. Eur J Cell Biol. 2022 Jan;101(1):151185. doi:10.1016/j.ejcb.2021.151185
Reiter, R., Tan, D., Rosales-Corral, S., Galano, A., Zhou, X., and Xu, B. (2018). Mitochondria: central organelles for Melatonin′s antioxidant and anti-aging actions. Molecules 23 (2), 509. doi:10.3390/molecules23020509
Reiter, R. J., Paredes, S. D., Manchester, L. C., and Tan, D. X. (2009). Reducing oxidative/nitrosative stress: a newly-discovered genre for melatonin. Crit. Rev. Biochem. Mol. Biol. 44 (4), 175–200. doi:10.1080/10409230903044914
Ren, P., Hu, H., and Zhang, R. (2000). [Observation on efficacy of puerarin in treating diabetic retinopathy]bservation on efficacy of puerarin in treating diabetic retinopathy. Zhongguo Zhong Xi Yi Jie He Za Zhi 20 (8), 574–576.
Riley, B. E., Lougheed, J. C., Callaway, K., Velasquez, M., Brecht, E., Nguyen, L., et al. (2013). Structure and function of parkin E3 ubiquitin ligase reveals aspects of RING and HECT ligases. Nat. Commun. 4, 1982. doi:10.1038/ncomms2982
Rogov, V. V., Suzuki, H., Marinković, M., Lang, V., Kato, R., Kawasaki, M., et al. (2017). Phosphorylation of the mitochondrial autophagy receptor nix enhances its interaction with LC3 proteins. Sci. Rep. 7 (1), 1131. doi:10.1038/s41598-017-01258-6
Russell, S. T., Wyke, S. M., and Tisdale, M. J. (2006). Mechanism of induction of muscle protein degradation by angiotensin II. Cell. Signal. 18 (7), 1087–1096. doi:10.1016/j.cellsig.2005.09.009
Samuvel, D. J., Li, L., Krishnasamy, Y., Gooz, M., Takemoto, K., Woster, P. M., et al. (2022). Mitochondrial depolarization after acute ethanol treatment drives mitophagy in living mice. Autophagy 18 (11), 2671–2685. doi:10.1080/15548627.2022.2046457
Sarhene, M., Ni, J. Y., Duncan, E. S., Liu, Z., Li, S., Zhang, J., et al. (2021). Ginsenosides for cardiovascular diseases; update on pre-clinical and clinical evidence, pharmacological effects and the mechanisms of action. Pharmacol. Res. 166, 105481. doi:10.1016/j.phrs.2021.105481
Saxena, S., Anand, S. K., Sharma, A., and Kakkar, P. (2024). Involvement of Sirt1-FoxO3a-Bnip3 axis and autophagy mediated mitochondrial turnover in according protection to hyperglycemic NRK-52E cells by Berberine. Toxicol. Vitro 100, 105916. doi:10.1016/j.tiv.2024.105916
Saxena, S., Shukla, S., and Kakkar, P. (2018). Berberine induced modulation of PHLPP2-Akt-MST1 kinase signaling is coupled with mitochondrial impairment and hepatoma cell death. Toxicol. Appl. Pharmacol. 347, 92–103. doi:10.1016/j.taap.2018.03.033
Saxton, R. A., and Sabatini, D. M. (2017). mTOR signaling in growth, metabolism, and disease. Cell 168 (6), 960–976. doi:10.1016/j.cell.2017.02.004
Sekar, V., Mani, S., Malarvizhi, R., Nithya, P., and Vasanthi, H. R. (2019). Antidiabetic effect of mangiferin in combination with oral hypoglycemic agents metformin and gliclazide. Phytomedicine 59, 152901. doi:10.1016/j.phymed.2019.152901
Serikbaeva, A., Li, Y., Ganesh, B., Zelkha, R., and Kazlauskas, A. (2022). Hyperglycemia promotes mitophagy and thereby mitigates hyperglycemia-induced damage. Am. J. Pathology 192 (12), 1779–1794. doi:10.1016/j.ajpath.2022.08.004
Setoguchi, Y., Oritani, Y., Ito, R., Inagaki, H., Maruki-Uchida, H., Ichiyanagi, T., et al. (2014). Absorption and metabolism of piceatannol in rats. J. Agric. Food Chem. 62 (12), 2541–2548. doi:10.1021/jf404694y
Shadfar, S., Couch, M. E., McKinney, K. A., Weinstein, L. J., Yin, X., Rodríguez, J. E., et al. (2011). Oral resveratrol therapy inhibits cancer-induced skeletal muscle and cardiac atrophy in vivo. Nutr. Cancer 63 (5), 749–762. doi:10.1080/01635581.2011.563032
Shan, Z., Fa, W. H., Tian, C. R., Yuan, C. S., and Jie, N. (2022). Mitophagy and mitochondrial dynamics in type 2 diabetes mellitus treatment. Aging 14 (6), 2902–2919. doi:10.18632/aging.203969
Shi, M. D., Liao, Y. C., Shih, Y. W., and Tsai, L. Y. (2013). Nobiletin attenuates metastasis via both ERK and PI3K/Akt pathways in HGF-treated liver cancer HepG2 cells. Phytomedicine 20 (8–9), 743–752. doi:10.1016/j.phymed.2013.02.004
Shim, J. Y., Chung, J. O., Jung, D., Kang, P. S., Park, S. Y., Kendi, A. T., et al. (2023). Parkin-mediated mitophagy is negatively regulated by FOXO3A, which inhibits Plk3-mediated mitochondrial ROS generation in STZ diabetic stress-treated pancreatic β cells. PLoS ONE 18 (5), e0281496. doi:10.1371/journal.pone.0281496
Shirihai, O. S., Song, M., and Dorn, G. W. (2015). How mitochondrial dynamism orchestrates mitophagy. Circulation Res. 116 (11), 1835–1849. doi:10.1161/CIRCRESAHA.116.306374
Sim, M. O., Lee, H. J., Jeong, D. E., Jang, J. H., Jung, H. K., and Cho, H. W. (2019). 6′-O-acetyl mangiferin from Iris rossii Baker inhibits lipid accumulation partly via AMPK activation in adipogenesis. Chemico-Biological Interact. 311, 108755. doi:10.1016/j.cbi.2019.108755
Sobrevia, L., Valero, P., Grismaldo, A., Villalobos-Labra, R., Pardo, F., Subiabre, M., et al. (2020). Mitochondrial dysfunction in the fetoplacental unit in gestational diabetes mellitus. Biochim. Biophys. Acta Mol. Basis Dis. 1866 (12), 165948. doi:10.1016/j.bbadis.2020.165948
Song, Z., Yan, C., Zhan, Y., Wang, Q., Zhang, Y., and Jiang, T. (2023). Melatonin attenuates lung ischemia-reperfusion injury through SIRT3 signaling-dependent mitophagy in type 2 diabetic rats. Exp. Lung Res. 49 (1), 101–115. doi:10.1080/01902148.2023.2213335
Sriwastawa, K., and Kumar, A. (2024). Mitochondrial dysfunction in diabetic neuropathy: impaired mitophagy triggers NLRP3 inflammasome. Mitochondrion 79, 101972. doi:10.1016/j.mito.2024.101972
Stene, L. C. (2024). Global diabetes incidence trends in young adults. Lancet Diabetes and Endocrinol. 12 (12), 870–872. doi:10.1016/S2213-8587(24)00286-9
Su, J., Gao, C., Xie, L., Fan, Y., Shen, Y., Huang, Q., et al. (2021). Astragaloside II ameliorated podocyte injury and mitochondrial dysfunction in streptozotocin-induced diabetic rats. Front. Pharmacol. 12, 638422. doi:10.3389/fphar.2021.638422
Su, M., Zhao, W., Xu, S., and Weng, J. (2022). Resveratrol in treating diabetes and its cardiovascular complications: a review of its mechanisms of action. Antioxidants (Basel) 11 (6), 1085. doi:10.3390/antiox11061085
Suh, K. S., Chon, S., and Choi, E. M. (2018). Protective effects of piceatannol on methylglyoxal-induced cytotoxicity in MC3T3-E1 osteoblastic cells. Free Radic. Res. 52 (6), 712–723. doi:10.1080/10715762.2018.1467010
Sun, S., Wang, K., Lei, H., Li, L., Tu, M., Zeng, S., et al. (2014). Inhibition of organic cation transporter 2 and 3 may be involved in the mechanism of the antidepressant-like action of berberine. Prog. Neuro-Psychopharmacology Biol. Psychiatry 49, 1–6. doi:10.1016/j.pnpbp.2013.11.005
Sun, Y., Kong, L., Zhang, A. H., Han, Y., Sun, H., Yan, G. L., et al. (2022). A hypothesis from metabolomics analysis of diabetic retinopathy: arginine-creatine metabolic pathway may Be a new treatment strategy for diabetic retinopathy. Front. Endocrinol. 13, 858012. doi:10.3389/fendo.2022.858012
Szydlowska, K., and Tymianski, M. (2010). Calcium, ischemia and excitotoxicity. Cell Calcium 47 (2), 122–129. doi:10.1016/j.ceca.2010.01.003
Tang, H., Yang, M., Liu, Y., Zhu, X., Liu, S., Liu, H., et al. (2022). Melatonin alleviates renal injury by activating mitophagy in diabetic nephropathy. Front. Endocrinol. 13, 889729. doi:10.3389/fendo.2022.889729
The Lancet Diabetes and Endocrinology (2024). Diabetes-related complications: a toll too high. Lancet Diabetes and Endocrinol. 12 (9), 601. doi:10.1016/S2213-8587(24)00246-8
Tian, H., Kang, Y. M., Gao, H. L., Shi, X. L., Fu, L. Y., Li, Y., et al. (2019). Chronic infusion of berberine into the hypothalamic paraventricular nucleus attenuates hypertension and sympathoexcitation via the ROS/Erk1/2/iNOS pathway. Phytomedicine 52, 216–224. doi:10.1016/j.phymed.2018.09.206
Tillhon, M., Guamán Ortiz, L. M., Lombardi, P., and Scovassi, A. I. (2012). Berberine: new perspectives for old remedies. Biochem. Pharmacol. 84 (10), 1260–1267. doi:10.1016/j.bcp.2012.07.018
Uchida-Maruki, H., Inagaki, H., Ito, R., Kurita, I., Sai, M., and Ito, T. (2015). Piceatannol lowers the blood glucose level in diabetic mice. Biol. and Pharm. Bull. 38 (4), 629–633. doi:10.1248/bpb.b15-00009
Uppal, S., Italiya, K. S., Chitkara, D., and Mittal, A. (2018). Nanoparticulate-based drug delivery systems for small molecule anti-diabetic drugs: an emerging paradigm for effective therapy. Acta Biomater. 81, 20–42. doi:10.1016/j.actbio.2018.09.049
Velmurugan, C., Sujith, T., Prasanth Kumar, M., Akmal Ali Baig, M., Ilanthalir, S., and Chandran, G. (2023). Evaluation of bergia capensis on hepatic cholestasis induced by metformin treated diabetic rats. IN Patent IN202341015947.
Wang, D., Sun, H., Song, G., Yang, Y., Zou, X., Han, P., et al. (2018a). Resveratrol improves muscle atrophy by modulating mitochondrial quality control in STZ-induced diabetic mice. Mol. Nutr. Food Res. 62 (9), 1700941. doi:10.1002/mnfr.201700941
Wang, D. T., Yin, Y., Yang, Y. J., Lv, P. J., Shi, Y., Lu, L., et al. (2014). Resveratrol prevents TNF-α-induced muscle atrophy via regulation of Akt/mTOR/FoxO1 signaling in C2C12 myotubes. Int. Immunopharmacol. 19 (2), 206–213. doi:10.1016/j.intimp.2014.02.002
Wang, K., Zheng, X., Pan, Z., Yao, W., Gao, X., Wang, X., et al. (2020). Icariin prevents extracellular matrix accumulation and ameliorates experimental diabetic kidney disease by inhibiting oxidative stress via GPER mediated p62-dependent Keap1 degradation and Nrf2 activation. Front. Cell Dev. Biol. 8, 559. doi:10.3389/fcell.2020.00559
Wang, L., Liu, F., Liu, Y., Gao, H., and Dong, M. (2019). [Cyanidin-3-O-glucoside inhibits proliferation of colorectal cancer cells by targeting TOPK]yanidin-3-O-glucoside inhibits proliferation of colorectal cancer cells by targeting TOPK. Xi Bao Yu Fen Zi Mian Yi Xue Za Zhi 35 (12), 1101–1108.
Wang, P., Cui, Y., Liu, Y., Li, Z., Bai, H., Zhao, Y., et al. (2022a). Mitochondrial ferritin alleviates apoptosis by enhancing mitochondrial bioenergetics and stimulating glucose metabolism in cerebral ischemia reperfusion. Redox Biol. 57, 102475. doi:10.1016/j.redox.2022.102475
Wang, P., Zhang, S., Lin, S., and Lv, Z. (2022b). Melatonin ameliorates diabetic hyperglycaemia-induced impairment of Leydig cell steroidogenic function through activation of SIRT1 pathway. Reprod. Biol. Endocrinol. 20 (1), 117. doi:10.1186/s12958-022-00991-6
Wang, S., Long, H., Hou, L., Feng, B., Ma, Z., Wu, Y., et al. (2023a). The mitophagy pathway and its implications in human diseases. Signal Transduct. Target Ther. 8 (1), 304. doi:10.1038/s41392-023-01503-7
Wang, S., Ren, H., Zhong, H., Zhao, X., Li, C., Ma, J., et al. (2022c). Combined berberine and probiotic treatment as an effective regimen for improving postprandial hyperlipidemia in type 2 diabetes patients: a double blinded placebo controlled randomized study. Gut Microbes 14 (1), 2003176. doi:10.1080/19490976.2021.2003176
Wang, S., Zhao, Z., Feng, X., Cheng, Z., Xiong, Z., Wang, T., et al. (2018b). Melatonin activates Parkin translocation and rescues the impaired mitophagy activity of diabetic cardiomyopathy through Mst1 inhibition. J. Cell. Mol. Medi 22 (10), 5132–5144. doi:10.1111/jcmm.13802
Wang, S., Zhou, F., Whisner, C., and Fan, Z. (2025). Resveratrol and quercetin-loaded nanoparticles and methods of use thereof. WO Patent WO2025024299.
Wang, Y., Li, H., Cao, J., Liu, A., Kou, Z., An, W., et al. (2023b). BMP4 aggravates mitochondrial dysfunction of HRMECs. Heliyon 9 (3), e13824. doi:10.1016/j.heliyon.2023.e13824
Wang, Y., Xu, Y., Wang, Q., Guo, F., Song, Y., Fan, X., et al. (2023c). Sulforaphane ameliorated podocyte injury according to regulation of the Nrf2/PINK1 pathway for mitophagy in diabetic kidney disease. Eur. J. Pharmacol. 958, 176042. doi:10.1016/j.ejphar.2023.176042
Wang, Y. P., Wat, E., Koon, C. M., Wong, C. W., Cheung, D. W. S., Leung, P. C., et al. (2016). The beneficial potential of polyphenol-enriched fraction from Erigerontis Herba on metabolic syndrome. J. Ethnopharmacol. 187, 94–103. doi:10.1016/j.jep.2016.04.040
Wang, Z. S., Xiong, F., Xie, X. H., Chen, D., Pan, J. H., and Cheng, L. (2015). Astragaloside IV attenuates proteinuria in streptozotocin-induced diabetic nephropathy via the inhibition of endoplasmic reticulum stress. BMC Nephrol. 16 (1), 44. doi:10.1186/s12882-015-0031-7
Watanabe, Y., Nagai, Y., Honda, H., Okamoto, N., Yamamoto, S., Hamashima, T., et al. (2016). Isoliquiritigenin attenuates adipose tissue inflammation in vitro and adipose tissue fibrosis through inhibition of innate immune responses in mice. Sci. Rep. 6 (1), 23097. doi:10.1038/srep23097
Wei, X., Zheng, Y., Ai, Y., and Li, B. (2022). Regulatory effects of astragaloside IV on hyperglycemia-induced mitophagy in Schwann cells. Evidence-Based Complementary Altern. Med. 2022, 1–8. doi:10.1155/2022/7864308
Whitworth, A. J., and Pallanck, L. J. (2009). The PINK1/Parkin pathway: a mitochondrial quality control system? J. Bioenerg. Biomembr. 41 (6), 499–503. doi:10.1007/s10863-009-9253-3
Wong, K. H., Li, G. Q., Li, K. M., Razmovski-Naumovski, V., and Chan, K. (2011). Kudzu root: traditional uses and potential medicinal benefits in diabetes and cardiovascular diseases. J. Ethnopharmacol. 134 (3), 584–607. doi:10.1016/j.jep.2011.02.001
Wu, B., Liu, R., Huang, H., and Chen, Y. Q. (2020). Resveratrol in the regulation of mitophagy of diabetic cardiomyopathy through AMPK-P53 signaling pathway. J. Lanzhou Univ. Sci. 1, 67–71, 76. doi:10.13885/j.issn.1000-2812.2020.01.013
Wu, C., Zhao, Y., Zhang, Y., Yang, Y., Su, W., Yang, Y., et al. (2022b). Gut microbiota specifically mediates the anti-hypercholesterolemic effect of berberine (BBR) and facilitates to predict BBR’s cholesterol-decreasing efficacy in patients. J. Adv. Res. 37, 197–208. doi:10.1016/j.jare.2021.07.011
Wu, H., Li, G., Chen, W., Luo, W., Yang, Z., You, Z., et al. (2022a). Drp1 knockdown represses apoptosis of rat retinal endothelial cells by inhibiting mitophagy. Acta Histochem. 124 (1), 151837. doi:10.1016/j.acthis.2021.151837
Wu, J., Qin, Z., Jiang, X., Fang, D., Lu, Z., Zheng, L., et al. (2022c). ROS-responsive PPGF nanofiber membrane as a drug delivery system for long-term drug release in attenuation of osteoarthritis. NPJ Regen. Med. 7 (1), 66. doi:10.1038/s41536-022-00254-3
Wu, W., Tian, W., Hu, Z., Chen, G., Huang, L., Li, W., et al. (2014). ULK1 translocates to mitochondria and phosphorylates FUNDC1 to regulate mitophagy. EMBO Rep. 15 (5), 566–575. doi:10.1002/embr.201438501
Wu, Y. S., Chen, Y. T., Bao, Y. T., Li, Z. M., Zhou, X. J., He, J. N., et al. (2016). Identification and verification of potential therapeutic target genes in berberine-treated zucker diabetic fatty rats through bioinformatics analysis. PLoS One 11(11), e0166378. doi:10.1371/journal.pone.0166378
Wyke, S. M., and Tisdale, M. J. (2006). Induction of protein degradation in skeletal muscle by a phorbol ester involves upregulation of the ubiquitin–proteasome proteolytic pathway. Life Sci. 78 (25), 2898–2910. doi:10.1016/j.lfs.2005.11.014
Xi, J., Rong, Y., Zhao, Z., Huang, Y., Wang, P., Luan, H., et al. (2021). Scutellarin ameliorates high glucose-induced vascular endothelial cells injury by activating PINK1/Parkin-mediated mitophagy. J. Ethnopharmacol. 271, 113855. doi:10.1016/j.jep.2021.113855
Xia, S., Ma, L., Wang, G., Yang, J., Zhang, M., Wang, X., et al. (2022). In vitro antimicrobial activity and the mechanism of berberine against methicillin-resistant Staphylococcus aureus isolated from bloodstream infection patients. IDR 15, 1933–1944. doi:10.2147/IDR.S357077
Xiang, J., Zhang, C., Di, T., Chen, L., Zhao, W., Wei, L., et al. (2022). Salvianolic acid B alleviates diabetic endothelial and mitochondrial dysfunction by down-regulating apoptosis and mitophagy of endothelial cells. Bioengineered 13 (2), 3486–3502. doi:10.1080/21655979.2022.2026552
Xiao, F., Zhang, R., and Wang, L. (2022). Inhibitors of mitochondrial dynamics mediated by dynamin-related protein 1 in pulmonary arterial hypertension. Front. Cell Dev. Biol. 10, 913904. doi:10.3389/fcell.2022.913904
Xiao, L., Xu, X., Zhang, F., Wang, M., Xu, Y., Tang, D., et al. (2017). The mitochondria-targeted antioxidant MitoQ ameliorated tubular injury mediated by mitophagy in diabetic kidney disease via Nrf2/PINK1. Redox Biol. 11, 297–311. doi:10.1016/j.redox.2016.12.022
Xie, Y., Liu, J., Kang, R., and Tang, D. (2020). Mitophagy receptors in tumor biology. Front. Cell Dev. Biol. 8, 594203. doi:10.3389/fcell.2020.594203
Xu, Y., Li, Y., Ma, L., Xin, G., Wei, Z., Zeng, Z., et al. (2018). d-galactose induces premature senescence of lens epithelial cells by disturbing autophagy flux and mitochondrial functions. Toxicol. Lett. 289, 99–106. doi:10.1016/j.toxlet.2018.02.001
Xu, Y., Shen, J., and Ran, Z. (2020). Emerging views of mitophagy in immunity and autoimmune diseases. Autophagy 16 (1), 3–17. doi:10.1080/15548627.2019.1603547
Xu, Y., and Yu, J. (2024). Allicin mitigates diabetic retinopathy in rats by activating phosphatase and tensin homolog-induced kinase 1/Parkin-mitophagy and inhibiting oxidative stress-mediated NOD-like receptor family pyrin domain containing 3 inflammasome. J. Physiol. Investig. 67 (4), 215–224. doi:10.4103/ejpi.EJPI-D-24-00039
Xue, Q., He, N., Wang, Z., Fu, X., Aung, L. H. H., Liu, Y., et al. (2021). Functional roles and mechanisms of ginsenosides from Panax ginseng in atherosclerosis. J. Ginseng Res. 45 (1), 22–31. doi:10.1016/j.jgr.2020.07.002
Yang, H. (2024). Traditional Chinese medicine preparation for preventing diabetes and preparation method thereof. CN Patent CN117695352.
Yang, J., Yu, Z., Jiang, Y., Zhang, Z., Tian, Y., Cai, J., et al. (2024d). SIRT3 alleviates painful diabetic neuropathy by mediating the FoxO3a-PINK1-Parkin signaling pathway to activate mitophagy. CNS Neurosci. Ther. 30 (4), e14703. doi:10.1111/cns.14703
Yang, L., Xu, L., Hao, X., Song, Z., Zhang, X., Liu, P., et al. (2024b). An aldose reductase inhibitor, WJ-39, ameliorates renal tubular injury in diabetic nephropathy by activating PINK1/Parkin signaling. Eur. J. Pharmacol. 967, 176376. doi:10.1016/j.ejphar.2024.176376
Yang, L. L., Xiao, N., Liu, J., Liu, K., Liu, B., Li, P., et al. (2017). Differential regulation of baicalin and scutellarin on AMPK and Akt in promoting adipose cell glucose disposal. Biochimica Biophysica Acta (BBA) - Mol. Basis Dis. 1863 (2), 598–606. doi:10.1016/j.bbadis.2016.11.024
Yang, N., Zhang, R., Zhang, H., Yu, Y., and Xu, Z. (2024a). ZIP7 contributes to the pathogenesis of diabetic cardiomyopathy by suppressing mitophagy in mouse hearts. Cardiovasc Diabetol. 23 (1), 399. doi:10.1186/s12933-024-02499-2
Yang, W., Qiu, C., Lv, H., Zhang, Z., Yao, T., Huang, L., et al. (2024c). Sirt3 protects retinal pigment epithelial cells from high glucose-induced injury by promoting mitophagy through the AMPK/mTOR/ULK1 pathway. Trans. Vis. Sci. Tech. 13 (3), 19. doi:10.1167/tvst.13.3.19
Ye, X., Chen, W., Tu, P., Jia, R., Liu, Y., Li, Y., et al. (2021). Food-derived cyanidin-3- O -glucoside alleviates oxidative stress: evidence from the islet cell line and diabetic db/db mice. Food Funct. 12 (22), 11599–11610. doi:10.1039/d1fo02385c
Yerra, V. G., Kalvala, A. K., and Kumar, A. (2017). Isoliquiritigenin reduces oxidative damage and alleviates mitochondrial impairment by SIRT1 activation in experimental diabetic neuropathy. J. Nutr. Biochem. 47, 41–52. doi:10.1016/j.jnutbio.2017.05.001
Yin, J., Xing, H., and Ye, J. (2008). Efficacy of berberine in patients with type 2 diabetes mellitus. Metabolism 57 (5), 712–717. doi:10.1016/j.metabol.2008.01.013
Yu, M. G., Gordin, D., Fu, J., Park, K., Li, Q., and King, G. L. (2024). Protective factors and the pathogenesis of complications in diabetes. Endocr. Rev. 45 (2), 227–252. doi:10.1210/endrev/bnad030
Yuan, L., Han, X., Li, W., Ren, D., and Yang, X. (2016). Isoorientin prevents hyperlipidemia and liver injury by regulating lipid metabolism, antioxidant capability, and inflammatory cytokine release in high-fructose-fed mice. J. Agric. Food Chem. 64 (13), 2682–2689. doi:10.1021/acs.jafc.6b00290
Yuan, P., Song, F., Zhu, P., Fan, K., Liao, Q., Huang, L., et al. (2022a). Poly (ADP -ribose) polymerase 1-mediated defective mitophagy contributes to painful diabetic neuropathy in the db/db model. J. Neurochem. 162 (3), 276–289. doi:10.1111/jnc.15606
Yuan, S., Ye, Z., Li, Y., Zou, J., Wu, M., Wang, K., et al. (2022b). Hypoglycemic effect of nobiletin via regulation of islet β-cell mitophagy and gut microbiota homeostasis in streptozocin-challenged mice. J. Agric. Food Chem. 70 (19), 5805–5818. doi:10.1021/acs.jafc.2c00148
Zhang, C., Xiang, J., Wang, G., Di, T., Chen, L., Zhao, W., et al. (2025). Salvianolic acid B improves diabetic skin wound repair through Pink1/Parkin-mediated mitophagy. Archives Physiology Biochem. 131 (1), 40–51. doi:10.1080/13813455.2024.2387693
Zhang, J., Wang, B., Gao, X., Peng, C., Shan, C., Johnson, S. F., et al. (2022a). RNF185 regulates proteostasis in Ebolavirus infection by crosstalk between the calnexin cycle, ERAD, and reticulophagy. Nat. Commun. 13 (1), 6007. doi:10.1038/s41467-022-33805-9
Zhang, L., Sun, S., Li, W., Zhang, W., Wang, X., and Yang, S. Y. (2017). Effect of Scutellarin inhibits collagen-induced arthritis through TLR4/NF-κB-mediated inflammation. Mol. Med. Rep. 16 (4), 5555–5560. doi:10.3892/mmr.2017.7292
Zhang, M. Y., Zhu, L., Zheng, X., Xie, T. H., Wang, W., Zou, J., et al. (2022b). TGR5 activation ameliorates mitochondrial homeostasis via regulating the pkcδ/drp1-HK2 signaling in diabetic retinopathy. Front. Cell Dev. Biol. 9, 759421. doi:10.3389/fcell.2021.759421
Zhang, S., Chen, S., Sun, D., Li, S., Sun, J., Gu, Q., et al. (2024). TIN2-mediated reduction of mitophagy induces RPE senescence under high glucose. Cell. Signal. 119, 111188. doi:10.1016/j.cellsig.2024.111188
Zhang, T., Xue, L., Li, L., Tang, C., Wan, Z., Wang, R., et al. (2016). BNIP3 protein suppresses PINK1 kinase proteolytic cleavage to promote mitophagy. J. Biol. Chem. 291 (41), 21616–21629. doi:10.1074/jbc.M116.733410
Zhang, Y., Tan, X., Cao, Y., An, X., Chen, J., and Yang, L. (2022c). Punicalagin protects against diabetic liver injury by upregulating mitophagy and antioxidant enzyme activities. Nutrients 14 (14), 2782. doi:10.3390/nu14142782
Zhang, Y., Zhang, H., Zhang, K., Li, Z., Guo, T., Wu, T., et al. (2020). Co-hybridized composite nanovesicles for enhanced transdermal eugenol and cinnamaldehyde delivery and their potential efficacy in ulcerative colitis. Nanomedicine 28, 102212. doi:10.1016/j.nano.2020.102212
Zhang, Z., Lam, T. N., and Zuo, Z. (2013). Radix puerariae: an overview of its chemistry, pharmacology, pharmacokinetics, and clinical use: the journal of clinical pharmacology. J. Clin. Pharmacol. 53 (8), 787–811. doi:10.1002/jcph.96
Zheng, D., Chen, L., Li, G., Jin, L., Wei, Q., Liu, Z., et al. (2022). Fucoxanthin ameliorated myocardial fibrosis in STZ-induced diabetic rats and cell hypertrophy in HG-induced H9c2 cells by alleviating oxidative stress and restoring mitophagy. Food Funct. 13 (18), 9559–9575. doi:10.1039/d2fo01761j
Zheng, G., Zhang, Q., Chang, B., Xie, P., Liao, H., Du, S., et al. (2023a). Dioscin induces osteosarcoma cell apoptosis by upregulating ROS-mediated P38 MAPK signaling. Drug Dev. Res. 84 (1), 25–35. doi:10.1002/ddr.22009
Zheng, T., BouSaba, J., Taylor, A., Dilmaghani, S., Busciglio, I., Carlson, P., et al. (2023b). A randomized, controlled trial of efficacy and safety of cannabidiol in idiopathic and diabetic gastroparesis. Clin. Gastroenterol. Hepatol. 21 (13), 3405–3414.e4. doi:10.1016/j.cgh.2023.07.008
Zheng, X. L., Chen, L., Fan, C. L., Xiao, S. J., Chen, S. B., and Yang, F. M. (2024). Hyperbaric lidocaine aggravates diabetic neuropathic pain by targeting p38 MAPK/ERK and PINK1/parkin-mediated mitophagy signaling pathway. Discov. Med. 36 (184), 992–1001. doi:10.24976/Discov.Med.202436184.92
Zhi, K., Wang, J., Zhao, H., and Yang, X. (2020). Self-assembled small molecule natural product gel for drug delivery: a breakthrough in new application of small molecule natural products. Acta Pharm. Sin. B 10 (5), 913–927. doi:10.1016/j.apsb.2019.09.009
Zhong, Y., Jin, R., Luo, R., Liu, J., Ren, L., Zhang, Y., et al. (2023). Diosgenin targets CaMKK2 to alleviate type II diabetic nephropathy through improving autophagy, mitophagy and mitochondrial dynamics. Nutrients 15 (16), 3554. doi:10.3390/nu15163554
Zhong, Y., Liu, J., Sun, D., Guo, T., Yao, Y., Xia, X., et al. (2022a). Dioscin relieves diabetic nephropathy via suppressing oxidative stress and apoptosis, and improving mitochondrial quality and quantity control. Food Funct. 13 (6), 3660–3673. doi:10.1039/d1fo02733f
Zhong, Y., Luo, R., Liu, Q., Zhu, J., Lei, M., Liang, X., et al. (2022b). Jujuboside A ameliorates high fat diet and streptozotocin induced diabetic nephropathy via suppressing oxidative stress, apoptosis, and enhancing autophagy. Food Chem. Toxicol. 159, 112697. doi:10.1016/j.fct.2021.112697
Zhong, Y., Zheng, Q. Y., Sun, C. Y., Zhang, Z., Han, K., and Jia, N. (2019). Orientin improves cognition by enhancing autophagosome clearance in an alzheimer’s mouse model. J. Mol. Neurosci. 69 (2), 246–253. doi:10.1007/s12031-019-01353-5
Zhou, B., Rayner, A. W., Gregg, E. W., Sheffer, K. E., Carrillo-Larco, R. M., Bennett, J. E., et al. (2024). Worldwide trends in diabetes prevalence and treatment from 1990 to 2022: a pooled analysis of 1108 population-representative studies with 141 million participants. Lancet 404 (10467), 2077–2093. doi:10.1016/S0140-6736(24)02317-1
Zhou, D., Zhou, M., Wang, Z., Fu, Y., Jia, M., Wang, X., et al. (2019b). PGRN acts as a novel regulator of mitochondrial homeostasis by facilitating mitophagy and mitochondrial biogenesis to prevent podocyte injury in diabetic nephropathy. Cell Death Dis. 10 (7), 524. doi:10.1038/s41419-019-1754-3
Zhou, P., Xie, W., He, S., Sun, Y., Meng, X., Sun, G., et al. (2019a). Ginsenoside Rb1 as an anti-diabetic agent and its underlying mechanism analysis. Cells 8 (3), 204. doi:10.3390/cells8030204
Zhou, P., Xie, W., Meng, X., Zhai, Y., Dong, X., Zhang, X., et al. (2019c). Notoginsenoside R1 ameliorates diabetic retinopathy through PINK1-dependent activation of mitophagy. Cells 8 (3), 213. doi:10.3390/cells8030213
Zhou, Y., Zhang, H., and Peng, C. (2014). Puerarin: a review of pharmacological effects. Phytotherapy Res. 28 (7), 961–975. doi:10.1002/ptr.5083
Zhu, J. Y., Van De Leemput, J., and Han, Z. (2024). Promoting mitochondrial dynamics by inhibiting the PINK1–PRKN pathway to relieve diabetic nephropathy. Dis. Model Mech. 17 (4), dmm050471. doi:10.1242/dmm.050471
Zhu, W., Yuan, Y., Liao, G., Li, L., Liu, J., Chen, Y., et al. (2018). Mesenchymal stem cells ameliorate hyperglycemia-induced endothelial injury through modulation of mitophagy. Cell Death Dis. 9 (8), 837. doi:10.1038/s41419-018-0861-x
Zhu, X., Bian, H., Wang, L., Sun, X., Xu, X., Yan, H., et al. (2019). Berberine attenuates nonalcoholic hepatic steatosis through the AMPK-SREBP-1c-SCD1 pathway. Free Radic. Biol. Med. 141, 192–204. doi:10.1016/j.freeradbiomed.2019.06.019
Zhu, Y., Gu, Z., Shi, J., Chen, C., Xu, H., and Lu, Q. (2022). “Vaspin attenuates atrial abnormalities by promoting ULK1/FUNDC1-mediated mitophagy,” in Oxidative medicine and cellular longevity. Editor R. Kosuru, 1–21.
Zhu, Y., Massen, S., Terenzio, M., Lang, V., Chen-Lindner, S., Eils, R., et al. (2013). Modulation of serines 17 and 24 in the LC3-interacting region of Bnip3 determines pro-survival mitophagy versus apoptosis. J. Biol. Chem. 288 (2), 1099–1113. doi:10.1074/jbc.M112.399345
Ziqubu, K., Dludla, P. V., Joubert, E., Muller, C. J. F., Louw, J., Tiano, L., et al. (2020). Isoorientin: a dietary flavone with the potential to ameliorate diverse metabolic complications. Pharmacol. Res. 158, 104867. doi:10.1016/j.phrs.2020.104867
Zorov, D. B., Juhaszova, M., and Sollott, S. J. (2014). Mitochondrial reactive oxygen species (ROS) and ROS-induced ROS release. Physiol. Rev. 94 (3), 909–950. doi:10.1152/physrev.00026.2013
Glossary
DM Diabetes mellitus
MMP Mitochondrial membrane potential
MQC Mitochondrial quality control
ROS Reactive oxygen species
T1DM Type 1 diabetes mellitus
GDM Gestational diabetes mellitus
IR Insulin resistance
OS Oxidative stress
DKD Diabetic kidney disease
EC Epithelial cell
RTI Renal tubular injury
DR Diabetic retinopathy
VEC Vascular endothelial cell
HG High-glucose
HFD High-fat diet
STZ Streptozotocin
VEGF Vascular endothelial growth factor
DCM Diabetic cardiomyopathy
ASCVD Atherosclerotic cardiovascular disease
H2S Hydrogen sulfide
RAECs Rat aortic endothelial cells
CNS Central nervous system
DKA Diabetic ketoacidosis
AD Alzheimer’s disease
I/R Ischemia/reperfusion
DPN Diabetic peripheral neuropathy
HL Hyperbaric lidocaine
DFU Diabetic foot ulcer
PICs Pro-inflammatory cytokines
LECs Lens ECs
PA Palmitic acid
UA Urolithin A
CsA Cyclosporine A
T-SOD Total superoxide dismutase
MF Mangiferin
MSCs Mesenchymal stem cells
MDI Medium
hADMSCs Human adipose-derived MSCs
OXPHOS Oxidative phosphorylation
BBR Berberine
Mel Melatonin
LIRI lung I/R injury
RES Resveratrol
MuRF-1 Muscle ring finger protein-1
C3G Cyanidin 3-glucoside chloride
CQ Chloroquine
SFN Sulforaphane
cKO Conditional knockout
AS II Astragaloside II
ICA Icariin
TCM Traditional Chinese medicine
ECM Extracellular matrix
ERS endoplasmic reticulum stress
JuA Jujuboside A
ISO Isoorientin
AS-IV Astragaloside IV
Alc Allicin
NGR1 Notoginsenoside R1
rMC-1 rat retinal Müller cells
FX Fucoxanthin
FN Fibronectin
α-SMA α-smooth muscle actin
HUVECs Human umbilical vein endothelial cells
Cyt.c Cytochrome C
Atg5 Autophagy-related gene 5
Mst1 Mammalian sterile 20-like kinase 1
Sal B Salvia divinorum B
PCN Piceatannol
DN Diabetic neuropathy
NAC N-acetylcysteine
SCs Schwann cells
Keywords: mitophagy, natural small molecules, diabetes mellitus, signaling pathway, review
Citation: Ye D, Zhu J, Su S, Yu Y, Zhang J, Yin Y, Lin C, Xie X, Xiang Q and Yu R (2025) Natural small molecules regulating the mitophagy pathway counteract the pathogenesis of diabetes and chronic complications. Front. Pharmacol. 16:1571767. doi: 10.3389/fphar.2025.1571767
Received: 06 February 2025; Accepted: 03 March 2025;
Published: 16 April 2025.
Edited by:
Jinlong Ma, Shandong Second Medical University, ChinaReviewed by:
Sagnik Nag, Monash University Malaysia, MalaysiaMeiqi Miao, Kunshan Traditional Chinese Medicine Hospital, China
Copyright © 2025 Ye, Zhu, Su, Yu, Zhang, Yin, Lin, Xie, Xiang and Yu. This is an open-access article distributed under the terms of the Creative Commons Attribution License (CC BY). The use, distribution or reproduction in other forums is permitted, provided the original author(s) and the copyright owner(s) are credited and that the original publication in this journal is cited, in accordance with accepted academic practice. No use, distribution or reproduction is permitted which does not comply with these terms.
*Correspondence: Rong Yu, MDAyMTY1QGhudWNtLmVkdS5jbg==
†These authors have contributed equally to this work