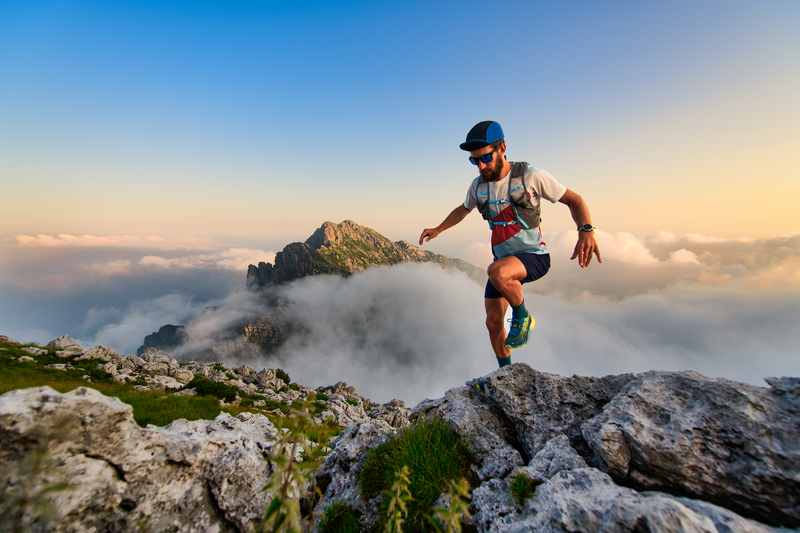
95% of researchers rate our articles as excellent or good
Learn more about the work of our research integrity team to safeguard the quality of each article we publish.
Find out more
REVIEW article
Front. Pharmacol. , 21 March 2025
Sec. Gastrointestinal and Hepatic Pharmacology
Volume 16 - 2025 | https://doi.org/10.3389/fphar.2025.1549526
This article is part of the Research Topic Reviews in Gastrointestinal and Hepatic Pharmacology: 2024 View all 8 articles
Drug-induced liver injury (DILI) results from the liver toxicity caused by drugs or their metabolites. Gallic acid (GA) is a naturally occurring secondary metabolite found in many fruits, plants, and nuts. Recently, GA has drawn increasing attention due to its potent pharmacological properties, particularly its anti-inflammatory and antioxidant capabilities. To the best of our knowledge, this is the first review to focus on the pharmacological properties of GA and related molecular activation mechanisms regarding protection against hepatotoxicity. We also provide a thorough explanation of the physicochemical properties, fruit sources, toxicity, and pharmacokinetics of GA after reviewing a substantial number of studies. Pharmacokinetic studies have shown that GA is quickly absorbed and eliminated when taken orally, which restricts its use in development. However, the bioavailability of GA can be increased by optimizing its structure or changing its form of administration. Notably, according to toxicology studies conducted on a range of animals and clinical trials, GA rarely exhibits toxicity or side effects. The antioxidation mechanisms mainly involved Nrf2, while anti-inflammatory mechanisms involved MAPKs and NF-κB signaling pathways. Owing to its marked pharmacological properties, GA is a prospective candidate for the management of diverse xenobiotic-induced hepatotoxicity. We also discuss the applications of cutting-edge technologies (nano-delivery systems, network pharmacology, and liver organoids) in DILI. In addition to guiding future research and development of GA as a medicine, this study offers a theoretical foundation for its clinical application.
The liver accounts for approximately 2% and 3% of the body weight in adults and adolescents, respectively, and is the largest internal parenchymatous organ of the body (Juza and Pauli, 2014). This organ performs physiological functions by regulating glycolipid and protein metabolism, stimulating the secretion of bile, and detoxifying products, such as drugs, plasma ammonia, and ethanol (Reinke and Asher, 2016; Driskill and Pan, 2021; Almazroo et al., 2017). Thus, the liver is highly vulnerable to toxins. Drug-induced liver injury (DILI) refers to the liver injury caused by adverse drug reaction, including chemicals, biological products, traditional Chinese patent medicines, health products, dietary supplements, as well as their metabolites, excipients, or impurities, which can deteriorate from asymptomatic liver dysfunction to liver failure and even death (Andrade et al., 2019; Li X. et al., 2022). DILI is typically divided into intrinsic and idiosyncratic categories based on the potential mechanism of drug action (Hoofnagle and Bjornsson, 2019). Intrinsic DILI is dose-dependent and predictable, with a relationship between the cytotoxic properties of the causative drug, whereas idiosyncratic DILI is primarily host-dependent and unpredictable because of its interactions with environmental and host elements (Andrade et al., 2019; Chen et al., 2015). The annual incidence of DILI in China is 23.80 per 100,000, and the two leading causes of DILI were traditional Chinese medicines and anti- -tuberculosis (TB) drugs (Shen et al., 2019).
Oxidative stress (OS) refers to a state of imbalance between oxidant agents and antioxidant effects in the body, with a tendency towards oxidation, which is recognized as one of the most central mechanisms participating in the progression of liver diseases, especially DILI (Cichoz-Lach and Michalak, 2014; Villanueva-Paz et al., 2021). In addition to OS, a number of other processes are involved in DILI, including activation of the immune response (Liu et al., 2004; Andrade et al., 2023), bile salt export pump inhibition (Morgan et al., 2010) and direct damage to toxicological drug properties (Price et al., 2009). Here, we discuss the role of OS in DILI. OS leads to generation of reactive radical species (ROS), included by superoxide radical (O2·-), nitric oxide (NO·), hydroxyl radical (HO·), peroxynitrite (ONOO·), and hydrogen peroxide (H2O2), which exert an influence on mitochondrial dysfunction and endoplasmic reticulum stress (Zorov et al., 2014; Uzi et al., 2013). Therefore, an acceptable therapeutic strategy using natural antioxidants instead of conventional treatments may ameliorate liver injury. Simultaneously, many plants and their extracts have been added to liver injury therapy owing to their antioxidant properties.
Gallic acid (GA) is a natural bioactive phenolic compound with antioxidant, anti-inflammatory, antimutagenic, anticarcinogenic, antiviral, antiultraviolet, and antimicrobial properties, contributing to the protection of organs against toxic compound-induced injury (Fanaei et al., 2021; Shruthi and Shenoy, 2021; Wang et al., 2023a; Govea-Salas et al., 2016). Despite the numerous pharmacological actions of GA, its function in DILI has not been elucidated. Thus, this paper aimed to provide a more conceptual and novel insight by reviewing the scientific evidence related to the hepatoprotective effects of GA, indicating that GA has the potential to be extensively used in medical treatments to attenuate liver injury caused by toxicity.
GA monohydrate appears as white or yellowish needle-shaped crystals or powder and was discovered in 1786 b y Scheele. The molecular weight of GA is 170.12 g/mol (Akbari et al., 2019), with a relative density of 1.694, and it is also a relatively thermostable compound (melting point of 252°C and boiling point of 501.1°C). It is soluble in ethanol and ether; hardly soluble in cold water and methanol; and insoluble in benzene and chloroform. Heating to 100°C–120°C will result in the loss of crystal water from GA. Further heating above 200°C will lead to the loss of carbon dioxide and formation of pyrogallic acid. The molecular formula of GA is C7H6O5; structurally, it contains one carboxyl group and three hydroxyl groups attached at positions 3, 4, and 5 on a benzene ring.
GA is a dietary polyphenol found in various fruits, vegetables, plants, and nuts, such as pomegranates and grapes, Acacia confuse Merr. and Graptopetalum paraguayense E. Walther (Tung et al., 2009; Duh et al., 2011; Zhou et al., 2019). Here, we discuss the sources of GA in various fruits (Figure 1). According to the Duke’s Phytochemical and Ethnobotanical Databases, mangoes have the highest average GA concentration, reaching up to 9,000 mg per 100 g of fresh fruit. Up to 95% of all polyphenols have been found in mango pulp from several commercial types and these polyphenols are primarily GA and galloyl-derived polyphenols, such as mono-galloyl glucose and gallotannins (Kim et al., 2021). Furthermore, pomegranates are thought to contain the second highest concentration of GA in any fruit, ranging from 0.45523 to 2045.00000 mg/100 g (Rothwell et al., 2013). Some fruits with lower GA content (such as strawberries, bananas, lemons, and apples) promote gastric acid secretion and encourage gastrointestinal peristalsis due to their natural phenolic acid content, which is beneficial for the digestion and absorption of food in the body. In addition, GA is present in a wide range of plants and vegetables, including Mentha spicata L., Camellia japonica L., Guazuma ulmifolia Lam., and Momordica charantia L. As anticipated, it has been determined that the majority of fruits and plants have the ability to eliminate heat, detoxify, and induce diuresis, all of which help to partially explain the biological actions of GA.
GA combines critical proteins or minerals, such as zinc, calcium, and iron to form an insoluble complex that disturbs other bioactive substances. In vivo studies have been performed with oral administration of GA to animals, and the edible LD50 value for GA is 5,000 mg/kg in rabbits and >2000 mg/kg in mice (Variya et al., 2019; Niho et al., 2001). However, limited information is available regarding the long-term toxicity of GA. Hematological studies showed no discrepancies in serum biomarkers (ALT, AST, GGT, and ACP) (Abarikwu et al., 2016). Histopathological findings demonstrated that only a small number of adipocytes were inhibited without bone marrow suppression. In addition, Variya et al. verified that a high dose of 900 mg/kg GA administered orally to Swiss albino mice daily for 28 days showed no significant morphological and behavioral alterations, and the histopathological findings proved the safety of GA (Variya et al., 2019).
Fischer rats were orally administered 0%–5% GA for 13 weeks. The toxic effects following 5% and 0.6% in females and males, respectively, included anemia (reduction of red blood cell counts, hematocrit, and hemoglobin concentration, and an increase in reticulocytes) and liver cell hypertrophy (Niho et al., 2001). Based on the present toxicology data in vivo models, 0.2% GA is regarded as a no-observed-adverse-effect level, which translates to 119 and 128 mg/kg/day for male and female rats, respectively (Niho et al., 2001). GA exhibits anti-tumor properties by inducing apoptosis in cancerous cell lines; however, it can also be harmful to normal cells by causing chemical changes in the GA molecule (Park et al., 2007; You and Park, 2011; Park et al., 2008). The major difference in cytotoxic potential between molecules is the length of the carbon chain, which influences the physical and chemical properties of GA (including its solubility and dispersion coefficient). Furthermore, changes in the propagation potential through the lipid membrane can affect interactions between molecules and their intracellular targets. The lipophilicity of GA determines its pharmacological activity and drug reaction, which leads to its binding to other targets that induce DNA damage (Locatelli et al., 2008).
In most cases, liver damage involves oxidative stress, which gradually evolves into hepatitis, cirrhosis, and hepatocellular carcinoma (HCC) without proper therapy. GA contains three adjacent reductive phenolic hydroxyl groups in its structural formula, which can bind to surrounding free radicals. Therefore, the antioxidant properties of GA play a considerable role in alleviating the accumulation of free radicals and delay the progression of liver damage (Duh et al., 2011; Gholamine et al., 2021; Wu et al., 2023). A 25-μM dose of GA exerts antioxidative effects by decreasing the inflammatory mediators (such as TNF-α, IL-1β, MCP-1, and iNOS) and promoting the expression of antioxidant enzymes in a co-culture of lipid-laden HEPA one to six hepatocytes and RAW 264 macrophages (Tanaka et al., 2020). GA and its derivatives can neutralize hypochlorous acid (HOCl) to protect α-1-protease against the inactivation, and reduce the peroxidation of the phospholipids in brain when dissolved in ethanol (Aruoma, 1993). In animal models of DILI, the activity of serum antioxidant biomarkers (including glutathione [GSH], superoxide dismutase [SOD], catalase [CAT], and GPx) significantly increased and lipid peroxidation products decreased in the GA-treated group compared with those in the model group (Go et al., 2016; Omobowale et al., 2018; Esmaeilzadeh et al., 2020). Altogether, GA exhibits antioxidative effects on liver damage as well as on other diseases associated with oxidative stress, such as cancer, cardiovascular disease, degenerative disease, and metabolic disease (Bashar et al., 2021; Heidarian et al., 2016; Yan et al., 2019; Mori et al., 2020). Therefore, GA is a potential dietary supplement owing to its ability to scavenge ROS and improve antioxidant capacity.
Liver diseases, such as HCC, are linked to underlying chronic inflammation and fibrosis caused by drug/environment toxicity, alcoholic and non-alcoholic fatty liver diseases, and hepatitis virus infections (Marengo et al., 2016; Yang et al., 2019). The anti-inflammatory properties of GA have been extensively investigated in recent in vivo and in vitro studies. In an in vitro experiment, GA inhibited secretion of pro-inflammatory cytokines (TNF-α, IL-1β, and IL-6), thereby preventing the damage response of lipopolysaccharide (LPS)-stimulated murine BV-2 cells (Lin et al., 2015). GA can impede intracellular receptor-interacting protein (RIP)1 and RIP3 increases and extracellular high mobility group box 1 protein (HMGB1) elevation induced by ethanol in an in vitro study of LO2 cells, resulting in inhibition of hepatocyte necroptosis (Zhou et al., 2019). Consistently, GA significantly protected against alcohol-induced stomach ulcers by lowering submucosal edema and cell infiltration in a dose-dependent manner by blocking the production of TNF-α and IL-1β/6 (Zhou et al., 2020). The downregulation of these pro-inflammatory factors by GA may be due to action of some transcriptional factors and kinases, such as c-JUN N-terminal kinases (JNK), NF-κB, and mitogen-activated protein kinase (MAPK) (Fanaei et al., 2021; Lin et al., 2015; Cai et al., 2024). Taken together, these results demonstrate that GA has anti-inflammatory properties and can serve as a powerful anti-inflammatory agent.
Hepatotoxicity is defined as liver dysfunction or damage caused by exposure to drugs or xenobiotics during liver metabolism (Anita and Om, 2014). Jaundice, edema, and discomfort are the first signs of liver disease, and these symptoms are accompanied by biochemical alterations, including elevated blood levels of hepatic enzymes and reduced hepatic enzymatic activity (Anita and Om, 2014). The examination of histology often reveals the common findings of destruction of intracellular organelles, fatty degeneration and necrosis of central hepatocytes, along with fibrosis and cirrhosis (Omobowale et al., 2018; Chen et al., 2018; Safaei et al., 2018). Hepatotoxicity could be caused by the combined action of the primary substance and reactive metabolites, as well as by immunologically mediated reactions that affect hepatocytes, biliary epithelial cells, the liver vasculature system, and other organs or tissues (Deng et al., 2009). Reactive metabolites refer to the metabolic pathways activated by drugs (including free radicals, quinones, unstable conjugates, and epoxides) that can combine with macromolecular substances (such as proteins and nucleic acids) intracellularly and interfere with the normal metabolism or structure of cells (Attia, 2010). Collectively, GA showed hepatoprotective properties against various liver-damaging agents in rodent models (Table 1).
Table 1. The hepatoprotective effects of gallic acid under various conditions of liver damage models in vivo.
CCl4 is frequently employed as a model chemical for liver injury to evaluate the effects of hepatoprotective treatments and illustrate the mechanisms underlying hepatotoxic reactions. The unstable free radicals trichloromethyl (CCl3·) and trichloromethyl peroxyl (CCl3O2·), which are produced when cytochrome P450 (CYP450) enzymes metabolize CCl4 in the endoplasmic reticulum of hepatocytes (Andritoiu et al., 2014), stimulate Kupffer cells to produce ROS, which results in lipid peroxidation, causing centrilobular hepatic necrosis, inflammation, and fibrosis (Weber et al., 2003). GA ameliorates CCl4-induced chronic liver injury by inhibiting lipid peroxidation and suppressing the activity of CYP2E1, a core component of the CYP450 enzyme superfamily and one of the key enzymes in the human body that metabolizes drugs (Tung et al., 2009). According to Perazzoli et al., GA elevates p53 gene expression in correlation with hepatic GSH concentration, which appears to be related to hepatocyte regeneration and antioxidative responses (Perazzoli et al., 2017). Additionally, GA directly produced antioxidant effects by stabilizing cell membranes and scavenging free radicals (Duh et al., 2011; Kanai and Okano, 1998). Natural extracts containing GA have antifibrotic characteristics, as evidenced by the high expression of metalloproteinase-1 (MMP-1) and the downregulation of profibrotic factors (such as transforming growth factor-β1 [TGF-β1], Smad2/3, matrix metalloproteinase-2 [MMP-2], and tissue inhibitor of matrix metalloproteinase [TIMP-1]) (Go et al., 2016; Wang et al., 2014). -
Currently, more than 80 commonly used antineoplastic drugs are roughly divided into six categories (cytotoxic drugs, hormone drugs, biological response modifiers, monoclonal antibody drugs, adjuvant drugs, and other types of drugs) in clinical practice, which are specifically used for cancer therapy. Nevertheless, DILI, a common organ ailment among the adverse responses to antineoplastic medication, limits the therapeutic efficacy of these treatments to some extent. Doxorubicin, an anthracycline class of compounds, has been widely used over the past several decades as a chemotherapeutic drug to treat various types of cancers (including lymphomas, leukemias, breast carcinoma, ovarian carcinoma, and thyroid carcinomas) by altering DNA and producing free radicals, which induce diverse cardiac, hepatic, hematological, and testicular toxicities (Omobowale et al., 2018; Prasanna et al., 2020). In doxorubicin-induced hepatotoxicity, GA enhanced hepatocyte survival by scavenging ROS and bolstering the antioxidant defense system (Omobowale et al., 2018). Cisplatin, cisplatinum, or cis-diamminedichloroplatinum (II) is commonly employed as an anti-tumor agent for treating solid tumors and hematological malignancies, and simultaneously leads to hepatotoxicity, nephrotoxicity, and ototoxicity (Volarevic et al., 2019; Wang et al., 2023b; Dogan et al., 2022). The protective effects of GA were linked to a decrease in oxidative stress and increase in antioxidant enzymes, both of which successfully reduced cisplatin-induced hepatotoxicity and nephrotoxicity caused by cisplatin (Dogan et al., 2022). Cyclophosphamide (CP), one of the most successful antineoplastic agents, shows acute or long-term toxic consequences, including hematological, cardiac, gonadal, hepatotoxic, and other toxic effects (Emadi et al., 2009). GA has anticlastogenic and antigenotoxic effects against CP-induced chromosomal damage in mouse bone marrow cells and reduces the pathological changes in hepatocytes caused by CP, including lobular necrosis, congestion with sinusoid dilatation, irregular arrangement, and rupture of hepatocytes (Shruthi and Shenoy, 2021). In addition, GA can mitigate biochemical and oxidative stress parameters (the reduction of ALT, ALP, malondialdehyde [MDA], and H2O2; and elevation of GSH, SOD, and CAT) in the liver of Wistar rats exposed to methotrexate, which inhibits dihydrofolate reductase from converting dihydrofolate to tetrahydrofolate to prevent DNA synthesis and cell proliferation (Safaei et al., 2018).
Owing to their widespread use and efficacy in reducing pain and swelling, NSAIDs are listed on the World Health Organization Model List of Essential Medicines. However, several placebo-controlled trials and meta-analyses have documented the negative effects of NSAIDs on hepatic, renal, gastrointestinal, cardiovascular, cerebral, and pulmonary problems (Bindu et al., 2020). Acetaminophen (paracetamol), originally known as 4-hydroxyacetanilide, is frequently used because of its analgesic and antipyretic qualities. Acetaminophen is the most common cause of drug-induced acute liver failure (ALF) in the United States, with an incidence of 0.59 per 1,000,000 person-years of ALF arising from idiosyncratic DILI (Andrade et al., 2019). Acetaminophen overdose causes a multitude of interrelated metabolic events in hepatocytes, including lipid peroxidation, protein oxidation, covalent modification, oxidative stress, mitochondrial dysfunction, and centrilobular necrosis (Jaeschke et al., 2012). Apart from guarding against oxidative stress and minimizing the damage caused by acetaminophen to the liver, GA also exhibits promise in reducing inflammation by dramatically downregulating pro-inflammatory factors (TNF-α, IL-1, p65, and p52) and upregulating IκB expression in liver tissue, which functions as a blocker for the NF-κB pathway (Rasool et al., 2010; Ezhilarasan et al., 2024). Among NSAIDs with anti-inflammatory, antipyretic, and antinociceptive properties, diclofenac (DIC) has severe adverse effects, including gastrointestinal injury and damage to hepatic, renal, lung, and cardiac tissues (Tomic et al., 2008). GA mitigates DIC-induced liver damage by reducing cellular ROS production, suppressing IL-1β gene expression, replenishing enzymatic and non-enzymatic antioxidants, and improving liver function enzymes (Esmaeilzadeh et al., 2020).
Excessive alcohol consumption poses a substantial public health challenge worldwide. Alcohol can affect all the organ systems in the body, leading to various disorders, most prominently in the liver, where it can cause steatosis, steatohepatitis, cirrhosis, and HCC (Mackowiak et al., 2024). GA stimulated the expression levels of peroxisome proliferator-activated receptor γ (PPAR γ), which is crucial for lipogenesis and lipid production and highly expressed in white adipose tissue (Christofides et al., 2021; Kartkaya et al., 2013). Reportedly, GA reversed the ethanol-induced decrease in paraoxonase (PON) activity by promoting PON1 expression and release from hepatocytes via the PPAR γ-PKA-cAMP intracellular signaling cascade (Kartkaya et al., 2013; Khateeb et al., 2010). In a mouse model of alcoholic liver disease (ALD) with iron overload, Wu et al. found that GA not only boosted antioxidant enzyme activity but also cured ALD-associated iron overload by regulating hepcidin downregulation and decreasing iron absorption in the gut (Wu et al., 2019).
Biotoxins, commonly known as natural toxins, are chemical substances produced by animals, plants, and microorganisms that exert toxic effects on other biological species. LPS, composed of lipids and polysaccharides, and the primary element of the outer membrane of gram-negative bacteria, results in a significant increase in the release of pro-inflammatory cytokines and ROS, which leads to liver damage (Lai et al., 2023). LPS-induced injury is characterized by the increased expression of nitric oxide synthase (iNOS), IL-1, IL-6, TLR4, cyclooxygenase-2 (COX-2), and prostaglandin E2 (PGE2) (Guo et al., 2021). GA treatment demonstrated antioxidant and anti-inflammatory effects in Sprague-Dawley rats with LPS-induced liver injury by lowering COX-2 expression and JNK2/1 MAPK phosphorylation (Lin et al., 2015). Aflatoxins, as the most studied largely among the mycotoxins produced by fungi, have multiple derivatives, such as aflatoxin B1 (AFB1), aflatoxin B2 (AFB2), aflatoxin G1 (AFG1), and aflatoxin G2 (AFG2). and AFB1 is the most toxic and can cause a variety of health problems, including cancers of the liver, lung, and gastrointestinal tract, delayed development, immunosuppression, and genotoxic effects (Deng et al., 2018; Marchese et al., 2018). Additionally, GA reduces AFB1-induced hepatorenal impairment by reversing the increase in caspase-3 levels, inhibiting AFB1 activation, and scavenging AFB1-O (exo-8,9-epoxide) (Owumi S. et al., 2020).
Polypharmacy in patients with depression has increased the incidence of antidepressant-induced DILI over the past two decades. Therefore, the hepatotoxicity of various antidepressants has gained interest. Fluoxetine is an antidepressant belonging to the serotonin reuptake inhibitor class and is used as a first-line treatment for depression. It has been proven to cause oxidative damage, which is associated with changes in the liver tissue and blood markers (Inkielewicz-Stępniak, 2011). In addition to reducing oxidative stress and mitigating fluoxetine-induced liver damage, GA modifies the activity of hepatic enzymes that metabolize drugs, such as the inhibition of monooxygenases involved in CYP450 (Karimi-Khouzani et al., 2017). In contrast, GA reduces hyperlipidemia caused by the effects of fluoxetine on the expression of genes linked to fatty acid synthesis and acetyl-CoA carboxylase 1, a lipogenic isoform found in the liver and adipose tissue (Karimi-Khouzani et al., 2017; Cheng et al., 2007). Ketamine (KET) is an anesthetic commonly used in both humans and animals. Recently, it has been shown to effectively and quickly reduce depressive symptoms in individuals with treatment-resistant depression as an N-methyl-D-aspartate receptor (NMDAR) antagonist (Smith-Apeldoorn et al., 2022). Even with acute administration and sub-anesthetic dosage, KET has possible pro-oxidant activity and causes oxidative stress by raising lipoperoxidation while lowering GSH levels, as was previously observed in the prefrontal cortex (De Oliveira et al., 2009). GA administration can prevent and reverse the oxidative damage caused by acute KET administration in brain regions (such as the cortex and hippocampus) and liver, minimizing its noxious effects (Schimites et al., 2020).
Isoniazid (INH) and rifampicin (RFP) are the first-line drugs used to treat tuberculosis. DILI caused by anti-tuberculosis drugs is the most common in India, accounting for 58% of all cases of DILI and 5%–22% of cases of acute liver failure caused by drugs (Devarbhavi et al., 2010; Kumar et al., 2010). By blocking the main bile salt exporter pump, the basolateral Na+/taurocholate cotransporting polypeptide (NTCP), rifampicin causes conjugated hyperbilirubinemia (Mita et al., 2006; Saukkonen et al., 2006). In addition, isoniazid undergoes either direct or indirect metabolism to acetyl hydrazine and hydrazine via N-acetyltransferase (NAT) and amidohydrolase. CYP450 monooxygenases in the liver oxidize these metabolites, producing electrophilic intermediates and free radicals that have been identified as hepatotoxins responsible for liver injury (Tasduq et al., 2005). GA can prevent liver toxicity caused by INH and RFP by maintaining the plasma membrane integrity of hepatocytes, as evidenced by biochemical and histological markers (Sanjay et al., 2021). Moreover, GA has a preventive effect on INH- and RFP-induced liver damage by inhibiting pro-inflammatory signals mediated by NF-κB and upregulating gene production of endogenous antioxidants through the nuclear factor erythroid 2-related factor 2 (Nrf2) pathway (Sanjay et al., 2021). The Nrf2 transcription factor is a major regulator of the antioxidant defense system, which modulates the expression of cytoprotective genes in their regulatory regions and encodes detoxifying enzymes for drug metabolism and redox balance (Kumar et al., 2014). Previous research investigated the inhibitory effects of GA on NF-κB mediated inflammatory response; Kim et al. found that GA reduced NF-κB activation and downregulated the expression of pro-inflammatory cytokines, such as TNF-α and IL-6 (Kim et al., 2006).
Paraquat is an abrasive chemical compound that is widely used to kill plants, particularly for controlling weeds and grasses in developing countries. A blood content of 8.5 μg/mL of paraquat causes harmful effects on organs including the heart, liver, kidneys, and lungs (Amin et al., 2021). The findings of a previous study provided further insight into the free radical scavenging properties of GA by showing that GA (100 mg/kg, po) reduced the levels of plasma protein C, thereby inhibiting the oxidative effects of paraquat on the hepatocytes of Wistar rats (Nouri et al., 2021). Ma et al. demonstrated that GA was able to reduce dimethylnitrosamine (DMN; a potent hepatotoxin, carcinogen, and mutagen)-induced acute liver damage in mice, suggesting the potential mechanism is that detoxifying capacity of liver tissue can be improved by upregulating the expression levels of hemeoxygenase-1 (HO-1) and glutathione-s-transferase-α3 (GST-α3) (Ma, 2014). Simultaneously, another study offered the first evidence that GA, through its enhanced antioxidant capacity and involvement in the regulation of cytokine expression, can reduce DMN-induced liver fibrosis in rats (Chen et al., 2018). Due to its antioxidant qualities, which raise intracellular antioxidant capacity, GA prevented sodium arsenite-induced renal and kidney damage, which was demonstrated by ameliorating tissue histological alterations and serum levels (ALT, AST, ALP, Cr, BUN, MDA, IL-1β, SOD, and CAT) (Gholamine et al., 2021). Additionally, combining polyphenols, such as GA with stable ω-3 fatty acids (ω-3FA) may provide therapeutic drug candidates for treating injuries to target organs caused by oxidative and inflammatory reactions in individuals who have been exposed to manganese or related toxic chemicals at work or in the environment (Owumi SE. et al., 2020).
Altogether, through a number of regulatory mechanisms, the most significant of which are its anti-inflammatory and antioxidant properties, GA ameliorates liver damage caused by hepatotoxicity (Figure 2). Without appropriate treatment, liver injury typically progresses to hepatitis, cirrhosis, and even the most serious form, HCC. HCC arising from the hepatocytes, as a late consequence of chronic progressive liver disease, is a malignant tumor that has grown to be a major global public health concern (Severi et al., 2010; Maida et al., 2014). Notably, previous research demonstrated that the Fuzheng Jiedu Xiaoji formulation of traditional Chinese medicine, which contains GA and chlorogenic acid, considerably increased overall and progression-free survival while lowering the death rate of patients with HCC (Yang et al., 2021). Jagan et al. demonstrated that GA is a strong anti-proliferative agent against diethylnitrosamine-induced HCC by lowering the levels of proliferation markers (Jagan et al., 2008). Consequently, GA plays a critical role in the prevention of HCC by ameliorating liver injury and exerting its anti-tumor property.
The significant biological and pharmacological activities of GA have led to its widespread use in pharmacokinetic testing to study its absorption, distribution, metabolism, and excretion. Evaluation of the optimal dose of GA for disease prevention and treatment is highly beneficial because of these findings.
The release of GA from unprocessed dietary ingredients is its primary source of bioactivity. The gastrointestinal tract quickly absorbs dietary GA when administered orally. The absorption of GA in various intestinal segments revealed that the upper end of the gut was superior to the lower end and the absorption process was passive diffusion, based on a rat intestinal unidirectional perfusion model. Furthermore, intestinal efflux transporters, such as P-glycoprotein (P-gp) and multidrug resistance protein 2 (MRP2) may bind to GA (Cheng et al., 2021). Yu et al. conducted comprehensive analyses that identified the following pharmacokinetic properties of GA in SD rats: the maximum plasma concentration (Cmax), terminal elimination half-life (T1/2), mean time to peak concentration (Tmax), and area under the curve (AUC) of plasma-concentration time at 0.83 μg/mL, 1.5 h, 2.56 h, and 0.137 mg⋅min/mL, respectively (Yu et al., 2018). Similarly, when rats were orally administered pomegranate flower extract (23.94 mg/kg of GA), Tmax of 0.5 h, Cmax of 707.58 ng/mL, and AUC of 5,711.06 (ng⋅h/mL) of GA were noted (Yisimayili et al., 2022). GA and its derivatives are widely found in plants and have a basic chemical structure, therefore, the ability of the gastrointestinal tract to absorb GA following oral administration is dependent on several variables, including sex, age, health state, drug form, and dosage. Therefore, to establish a solid scientific foundation for the dietary supplementation of GA, particular emphasis should be placed on the bioavailability and gastrointestinal absorption mechanism of GA in plant extracts.
GA is rapidly absorbed into the circulatory system and distributed to various organs in a wide range of areas. Healthy Sprague-Dawley rats were orally administered Polygonum chinense Linn extract equivalent to 2.68 mg/kg of GA. The tissue-to-plasma concentration ratios of GA decreased in the following order: kidneys (6.50), heart (0.99), liver (0.93), lungs (0.473), and spleen (0.155) (Chen et al., 2020). Furthermore, the accumulation of GA in the brain within the first 15 s of the experiment was almost 11 times higher than the basal concentration in the control group, indicating that GA may have brain-targeting characteristics (Gasperotti et al., 2015). As previously indicated, the brain distribution propensity of GA correlates with its protective benefits against neurological disorders caused by medicines, diseases, and environmental toxins; hence, its significance in brain disorders warrants further investigation. The findings of the aforementioned studies indicate that following oral administration, GA is preferentially distributed to the kidney, heart, liver, and brain, and associated with the metabolic processes of the kidney and liver, as well as the pumping function of the heart. Nevertheless, current research has ignored the distribution patterns of GA metabolites in organs, focusing on the detection of these metabolites (such as 3-dihydroshikimic acid, syringic acid, ethyl gallate, and propyl gallate) in plasma, urine, and feces instead (Choubey et al., 2015; Ow and Stupans, 2003). Hence, designing and conducting additional correlation studies between the distribution of GA and its metabolites in tissues, and their biological activities are crucial.
There is a growing consensus that two major phases are involved in the metabolism of GA, and more than 30 metabolites (mostly pyrogallol, protocatechuic acid, methylates, sulfates, and glucuronides associated with these two compounds) have been found in the plasma, urine, and feces (Liang et al., 2013; Zhang et al., 2017). In the phase I metabolism of GA, the principal phase biotransformation pathways are decarboxylation and dehydroxylation processes according to the structure of GA, which produce pyrogallol and protocatechuic acid, respectively, the two main GA-metabolite structures in investigations involving humans (Liang et al., 2013; Zhong et al., 2016). The metabolites of GA are created by sulfation (pyrogallol-2-O-sulfate, protocatechuic acid-3-O-sulfate, and GA sulfate), glucuronidation (GA glucuronide, pyrogallol-O-glucuronidation, and protocatechuic acid glucoside), and methylation (4-O-methylgallic acid) following the creation of phase I metabolites, which are excreted through urine and feces (Wang et al., 2019). The liver is responsible for phase II metabolism of GA, which involves the action of sulfotransferases (SULTs), UDP-glucosyltransferases (UGTs), and catechol-O-methyltransferases (COMTs) (Isvoran et al., 2022; Jarrar and Lee, 2021; Bastos et al., 2017). Similarly, the intestinal microbiota was capable of regulating GA metabolism involving five metabolic reactions (including methylation, dimethylation, trimethylation, decarboxylation, and dehydroxylation) in a cultivation system in which GA and protocatechuic acid were incubated with the intestinal flora fluid of SD rats (Luo et al., 2017).
Bioavailability is the relative quantity and rate at which drugs are absorbed into the circulation by the body and is influenced by formulation factors (including the size and crystal shape of the drug particles, molecular structure, excipients, tightness of fillers, and manufacturing procedures) and physiological factors (including digestibility, intestinal flora, transporter proteins, and metabolizing enzyme availability) (Kreider et al., 2022). Although GA has been shown to be effective in treating a range of diseases, one of its disadvantages is that it has a low bioavailability due to rapid elimination and poor absorption, which restricts its clinical application and promotion (Shahrzad et al., 2001).
The bioactivity of GA depends largely on its bioavailability, therefore, numerous attempts have been made to increase its bioavailability to enhance its nutritional benefits. In a study using a rat model of hepatotoxicity generated by CCl4, a phospholipid complex of GA was designed to improve lipophilicity and prevent poor absorption, boosting antioxidant capacity with enhanced bioavailability (Bhattacharyya et al., 2013). Further investigations found that GA was more stable and sustained when conjugated with phosphatidylcholine or polyamidoamine dendrimers, which enhanced its bioavailability and increased its hepatoprotective effects (Abdou and Masoud, 2018). Furthermore, compared with the polyherbal extracts of amla and pomegranate fruit peels, Patil et al. demonstrated a significant improvement in the oral bioavailability and anti-colon cancer activity of polyherbal nanoparticles (GA isolated from amla and quercetin separated from pomegranate fruit peel extract) (Patil and Killedar, 2021). The incorporation of GA into nanocarriers has the potential to improve oral bioavailability by enhancing its stability in the gastrointestinal tract, increasing its solubility, promoting organ targeting, facilitating barrier penetration, and extending circulation time (Sahyon et al., 2023).
Due to the low bioavailability of GA, its therapeutic applicability in hepatoprotection is currently limited. Therefore, it is critical to advance our understanding of drug delivery technologies and conduct further preclinical research using in vivo and in vitro models. The physicochemical properties of small molecules play a major role in determining how well drugs are absorbed into the body, therefore, efforts to improve the solubility, release control, activity range, and pharmacokinetics of drugs should come first in the delivery process. In the relatively new but rapidly expanding fields of nanomedicine and nano-delivery systems, nanoscale materials (liposomes, nanoparticles, polymeric micelles, polymer-drug conjugates, nanosuspensions, and nano-emulsions) are used as diagnostic tools or to carefully distribute therapeutic medicines to precise target areas (Li B. et al., 2022). Simultaneously, it is challenging to attain effective therapeutic results focused exclusively on a single target for a variety of chronic disorders (such as chronic liver damage) caused by multiple factors related to clinical practice. Consequently, network pharmacology has been widely used to discover drugs and active compounds in traditional Chinese medicine. This clarifies the overall mechanism of action, analyzes drug combinations, and determines the formula compatibility to design multi-target molecular drugs, and provide new ideas for the study of complex systems in traditional Chinese medicine and new technological support for rational clinical drug use (Jiashuo et al., 2022; Li and Zhang, 2013; Nogales et al., 2022). Molecular docking, as a chemical calculation method, can theoretically simulate interactions between small molecule medicines and their targets (such as protein receptors), predicting the manner and affinity of binding (Paggi et al., 2024). Combining network pharmacology and molecular docking can significantly increase drug discovery efficiency and contribute to comprehensive evaluation of multi-target mechanisms of action (Zhou et al., 2024; Tan et al., 2023). Moreover, organoid models are more similar to physiological cells in composition and activity, have a more stable genome, and require fewer operations during model development than typical 2D cell lines and animal models. They offer several considerable benefits in cost, clinical relevance, and high-throughput screening (Han et al., 2024; Heydari et al., 2021). Liver organoids can also be used in toxicological research, medication screening, and regenerative medicine, particularly for irreversible liver diseases (Prior et al., 2019; Messina et al., 2020). These new techniques will undoubtedly reveal hitherto unidentified molecular pathways and initiate an important new avenue of research on liver damage caused by hepatic toxicity (Figure 3).
Figure 3. New technologies are applied in liver injury caused by toxins. Nano-delivery systems, network pharmacology, and liver organoid can all be used to reveal more molecular processes in diseases and develop novel treatment strategies.
Recently, the scientific community has gradually become interested in GA, a naturally occurring polyphenolic molecule, owing to its antioxidant, anti-inflammatory, and hepatotoxic properties. This paper reviewed recent developments in GA research on its physicochemical properties, fruit origin, toxicity, prevention of hepatotoxicity, molecular mechanisms, pharmacokinetics, and bioavailability. In vivo models have provided convincing evidence that GA ameliorated liver toxicity induced by CCl4, antineoplastic drugs, NSAIDs, alcohols, biotoxins, antidepressants, anti-tuberculosis drugs, and other environmental toxins. GA is anticipated to be a successful medication for the long-term prevention of DILI because of its weak toxicity in animal and clinical trials. The main mechanisms by which GA reduces DILI include modulation of CYP450 enzyme activity, regulation of the MAPKs signaling pathway, free radical scavenging, upregulation of Nrf2, and downregulation of NF-κB and pro-inflammatory cytokines. Notably, further research is needed on the interactions between GA and other medicines to determine whether GA intervenes in the efficacy of other medicines. Although there is still much to learn about the hepatoprotective properties of GA, preclinical research and clinical trials are required to assess the pharmacological potential of GA metabolites, either in conjunction with medical interventions or as a dietary supplement to prevent toxicity-related liver damage.
Low bioavailability is a characteristic of GA that is influenced by several factors, including absorption, metabolism, distribution, excretion, and disease condition. Consequently, although GA improves DILI, its therapeutic application may be limited due to these disadvantages. Additionally, it is now an accepted practice to enhance the bioavailability of GA and adjust its physicochemical properties using nanotechnology to improve common dosage forms. Common types of nanocarriers include nanoliposomes, nanoparticles, nanosuspensions, nano-emulsions, polymeric micelles, polymer-drug conjugates, and nanosheets. However, more thorough investigations are needed to determine the biocompatibility and stability of the antioxidant activity of these nano-combinations at the animal level. Finally, the quick advancement of cutting-edge methods, such as the use of network pharmacology and emergence of organoids present previously unknown opportunities to research molecular pathways and subsequent pharmacological development in DILI therapy. These groundbreaking investigations could represent a substantial advancement in the search for naturally occurring substances with positive effects on humans and provide a foundation for future research aimed at preventing, mitigating, or curing liver damage caused by xenobiotic exposure or illness. In conclusion, several disorders linked to DILI can be treated with the robust anti-hepatotoxic properties of GA.
PC: Conceptualization, Data curation, Formal Analysis, Funding acquisition, Investigation, Methodology, Project administration, Resources, Software, Supervision, Validation, Visualization, Writing–original draft, Writing–review and editing. FZ: Conceptualization, Investigation, Software, Writing–review and editing. WL: Formal Analysis, Project administration, Supervision, Validation, Visualization, Writing–review and editing.
The author(s) declare that financial support was received for the research, authorship, and/or publication of this article. This work was supported by The Open Project of Hubei Key Laboratory (2023KFZZ001) and Key Laboratory of Neuropsychiatric Drug Research of Zhejiang Province (2019E10021).
The authors declare that the research was conducted in the absence of any commercial or financial relationships that could be construed as a potential conflict of interest.
The author(s) declare that no Generative AI was used in the creation of this manuscript.
All claims expressed in this article are solely those of the authors and do not necessarily represent those of their affiliated organizations, or those of the publisher, the editors and the reviewers. Any product that may be evaluated in this article, or claim that may be made by its manufacturer, is not guaranteed or endorsed by the publisher.
Abarikwu, S. O., Durojaiye, M., Alabi, A., Asonye, B., and Akiri, O. (2016). Curcumin protects against gallic acid-induced oxidative stress, suppression of glutathione antioxidant defenses, hepatic and renal damage in rats. Ren. Fail 38 (2), 321–329. doi:10.3109/0886022X.2015.1127743
Abdou, E. M., and Masoud, M. M. (2018). Gallic acid-PAMAM and gallic acid-phospholipid conjugates, physicochemical characterization and in vivo evaluation. Pharm. Dev. Technol. 23 (1), 55–66. doi:10.1080/10837450.2017.1344994
Akbari, G., Savari, F., Mard, S. A., Rezaie, A., and Moradi, M. (2019). Gallic acid protects the liver in rats against injuries induced by transient ischemia-reperfusion through regulating microRNAs expressions. Iran. J. Basic Med. Sci. 22 (4), 439–444. doi:10.22038/ijbms.2018.31589.7605
Almazroo, O. A., Miah, M. K., and Venkataramanan, R. (2017). Drug metabolism in the liver. Clin. Liver Dis. 21 (1), 1–20. doi:10.1016/j.cld.2016.08.001
Amin, F., Roohbakhsh, A., Memarzia, A., Kazerani, H. R., and Boskabady, M. H. (2021). Immediate and late systemic and lung effects of inhaled paraquat in rats. J. Hazard Mater 415, 125633. doi:10.1016/j.jhazmat.2021.125633
Andrade, R. J., Aithal, G. P., De Boer, Y. S., Liberal, R., Gerbes, A., Regev, A., et al. (2023). Nomenclature, diagnosis and management of drug-induced autoimmune-like hepatitis (DI-ALH): an expert opinion meeting report. J. Hepatol. 79 (3), 853–866. doi:10.1016/j.jhep.2023.04.033
Andrade, R. J., Chalasani, N., Bjornsson, E. S., Suzuki, A., Kullak-Ublick, G. A., Watkins, P. B., et al. (2019). Drug-induced liver injury. Nat. Rev. Dis. Prim. 5 (1), 58. doi:10.1038/s41572-019-0105-0
Andritoiu, C. V., Ochiuz, L., Andritoiu, V., and Popa, M. (2014). Effect of apitherapy formulations against carbon tetrachloride-induced toxicity in Wistar rats after three weeks of treatment. Molecules 19 (9), 13374–13391. doi:10.3390/molecules190913374
Anita, S., and Om, S. (2014). Clinical biochemistry of hepatotoxicity. J. Clin. Toxicol. 04 (01). doi:10.4172/2161-0495.s4-001
Aruoma, O. I. (1993). Evaluation of the antioxidant and prooxidant actions of gallic acid and its derivatives.
Attia, S. M. (2010). Deleterious effects of reactive metabolites. Oxid. Med. Cell Longev. 3 (4), 238–253. doi:10.4161/oxim.3.4.13246
Bashar, S. M., Elhadidy, M. G., Mostafa, A. F., Hamed, B., Helmy, S., and Abd-Elmoniem, H. A. (2021). Hepatoprotective effect of gallic acid against type 2-induced diabetic liver injury in male rats through modulation of fetuin-A and GLP-1 with involvement of ERK1/2/NF-κB and Wnt1/β-catenin signaling pathways. Gen. Physiol. Biophys. 40 (3), 221–234. doi:10.4149/gpb_2021005
Bastos, P., Gomes, T., and Ribeiro, L. (2017). Catechol-O-Methyltransferase (COMT): an update on its role in cancer, neurological and cardiovascular diseases. Rev. Physiol. Biochem. Pharmacol. 173, 1–39. doi:10.1007/112_2017_2
Bhattacharyya, S., Ahammed, S. M., Saha, B. P., and Mukherjee, P. K. (2013). The gallic acid-phospholipid complex improved the antioxidant potential of gallic acid by enhancing its bioavailability. AAPS PharmSciTech 14 (3), 1025–1033. doi:10.1208/s12249-013-9991-8
Bindu, S., Mazumder, S., and Bandyopadhyay, U. (2020). Non-steroidal anti-inflammatory drugs (NSAIDs) and organ damage: a current perspective. Biochem. Pharmacol. 180, 114147. doi:10.1016/j.bcp.2020.114147
Cai, Y., Zhao, D., Pan, Y., Chen, B., Cao, Y., Han, S., et al. (2024). Gallic acid attenuates sepsis-induced liver injury through C/EBPβ-Dependent MAPK signaling pathway. Mol. Nutr. Food Res. 68, e2400123. doi:10.1002/mnfr.202400123
Cheng, Z. Z., Zhou, B. H., Jiang, S., Li, K. Y., and Jin, D. (2021). Study on rat small intestinal absorption characteristics of gallic acid based on single-pass perfusion model. Chinese Pharmacological Bulletin, 37 (05), 669–673.
Cheng, D., Chu, C. H., Chen, L., Feder, J. N., Mintier, G. A., Wu, Y., et al. (2007). Expression, purification, and characterization of human and rat acetyl coenzyme A carboxylase (ACC) isozymes. Protein Expr. Purif. 51 (1), 11–21. doi:10.1016/j.pep.2006.06.005
Chen, M., Suzuki, A., Borlak, J., Andrade, R. J., and Lucena, M. I. (2015). Drug-induced liver injury: interactions between drug properties and host factors. J. Hepatol. 63 (2), 503–514. doi:10.1016/j.jhep.2015.04.016
Chen, X., Wei, L., Pu, X., Wang, Y., and Xu, Y. (2020). Pharmacokinetics and tissue distribution study of 15 ingredients of Polygonum chinense Linn extract in rats by UHPLC–MS/MS. Biomed. Chromatogr. 35 (2), e4975. doi:10.1002/bmc.4975
Chen, Y., Zhou, Z., Mo, Q., Zhou, G., and Wang, Y. (2018). Gallic acid attenuates dimethylnitrosamine-induced liver fibrosis by alteration of Smad phosphoisoform signaling in rats. Biomed. Res. Int. 2018, 1682743. doi:10.1155/2018/1682743
Choubey, S., Varughese, L. R., Kumar, V., and Beniwal, V. (2015). Medicinal importance of gallic acid and its ester derivatives: a patent review. Pharm. Pat. Anal. 4 (4), 305–315. doi:10.4155/ppa.15.14
Christofides, A., Konstantinidou, E., Jani, C., and Boussiotis, V. A. (2021). The role of peroxisome proliferator-activated receptors (PPAR) in immune responses. Metabolism 114, 154338. doi:10.1016/j.metabol.2020.154338
Cichoz-Lach, H., and Michalak, A. (2014). Oxidative stress as a crucial factor in liver diseases. World J. Gastroenterol. 20 (25), 8082–8091. doi:10.3748/wjg.v20.i25.8082
Deng, J., Zhao, L., Zhang, N. Y., Karrow, N. A., Krumm, C. S., Qi, D. S., et al. (2018). Aflatoxin B(1) metabolism: regulation by phase I and II metabolizing enzymes and chemoprotective agents. Mutat. Res. Rev. Mutat. Res. 778, 79–89. doi:10.1016/j.mrrev.2018.10.002
Deng, X., Luyendyk, J. P., Ganey, P. E., and Roth, R. A. (2009). Inflammatory stress and idiosyncratic hepatotoxicity: hints from animal models. Pharmacol. Rev. 61 (3), 262–282. doi:10.1124/pr.109.001727
De Oliveira, L., Spiazzi, C. M., Bortolin, T., Canever, L., Petronilho, F., Mina, F. G., et al. (2009). Different sub-anesthetic doses of ketamine increase oxidative stress in the brain of rats. Prog. Neuropsychopharmacol. Biol. Psychiatry 33 (6), 1003–1008. doi:10.1016/j.pnpbp.2009.05.010
Devarbhavi, H., Dierkhising, R., Kremers, W. K., Sandeep, M. S., Karanth, D., and Adarsh, C. K. (2010). Single-center experience with drug-induced liver injury from India: causes, outcome, prognosis, and predictors of mortality. Am. J. Gastroenterol. 105 (11), 2396–2404. doi:10.1038/ajg.2010.287
Dogan, D., Meydan, I., and Komuroglu, A. U. (2022). Protective effect of silymarin and gallic acid against cisplatin-induced nephrotoxicity and hepatotoxicity. Int. J. Clin. Pract. 2022, 6541026. doi:10.1155/2022/6541026
Driskill, J. H., and Pan, D. (2021). The hippo pathway in liver homeostasis and pathophysiology. Annu. Rev. Pathol. 16, 299–322. doi:10.1146/annurev-pathol-030420-105050
Duh, P. D., Lin, S. L., and Wu, S. C. (2011). Hepatoprotection of Graptopetalum paraguayense E. Walther on CCl₄-induced liver damage and inflammation. J. Ethnopharmacol. 134 (2), 379–385. doi:10.1016/j.jep.2010.12.029
Emadi, A., Jones, R. J., and Brodsky, R. A. (2009). Cyclophosphamide and cancer: golden anniversary. Nat. Rev. Clin. Oncol. 6 (11), 638–647. doi:10.1038/nrclinonc.2009.146
Esmaeilzadeh, M., Heidarian, E., Shaghaghi, M., Roshanmehr, H., Najafi, M., Moradi, A., et al. (2020). Gallic acid mitigates diclofenac-induced liver toxicity by modulating oxidative stress and suppressing IL-1β gene expression in male rats. Pharm. Biol. 58 (1), 590–596. doi:10.1080/13880209.2020.1777169
Ezhilarasan, D., Shree Harini, K., Karthick, M., and Selvaraj, C. (2024). Ethyl gallate concurrent administration protects against acetaminophen-induced acute liver injury in mice: an in vivo and in silico approach. Chem. Biol. Drug Des. 103 (1), e14369. doi:10.1111/cbdd.14369
Fanaei, H., Mard, S. A., Sarkaki, A., Goudarzi, G., and Khorsandi, L. (2021). Gallic acid treats dust-induced NAFLD in rats by improving the liver's anti-oxidant capacity and inhibiting ROS/NFκβ/TNFα inflammatory pathway. Iran. J. Basic Med. Sci. 24 (2), 240–247. doi:10.22038/IJBMS.2021.51036.11603
Gasperotti, M., Passamonti, S., Tramer, F., Masuero, D., Guella, G., Mattivi, F., et al. (2015). Fate of microbial metabolites of dietary polyphenols in rats: is the brain their target destination? ACS Chem. Neurosci. 6 (8), 1341–1352. doi:10.1021/acschemneuro.5b00051
Gholamine, B., Houshmand, G., Hosseinzadeh, A., Kalantar, M., Mehrzadi, S., and Goudarzi, M. (2021). Gallic acid ameliorates sodium arsenite-induced renal and hepatic toxicity in rats. Drug Chem. Toxicol. 44 (4), 341–352. doi:10.1080/01480545.2019.1591434
Go, J., Kim, J. E., Koh, E. K., Song, S. H., Sung, J. E., Lee, H. A., et al. (2016). Protective effect of gallotannin-enriched extract isolated from galla rhois against CCl₄-Induced hepatotoxicity in ICR mice. Nutrients 8 (3), 107. doi:10.3390/nu8030107
Govea-Salas, M., Rivas-Estilla, A. M., Rodriguez-Herrera, R., Lozano-Sepúlveda, S. A., Aguilar-Gonzalez, C. N., Zugasti-Cruz, A., et al. (2016). Gallic acid decreases hepatitis C virus expression through its antioxidant capacity. Exp. Ther. Med. 11 (2), 619–624. doi:10.3892/etm.2015.2923
Guo, W., Xiang, Q., Mao, B., Tang, X., Cui, S., Li, X., et al. (2021). Protective effects of microbiome-derived inosine on lipopolysaccharide-induced acute liver damage and inflammation in mice via mediating the TLR4/NF-κB pathway. J. Agric. Food Chem. 69 (27), 7619–7628. doi:10.1021/acs.jafc.1c01781
Han, X., Cai, C., Deng, W., Shi, Y., Li, L., Wang, C., et al. (2024). Landscape of human organoids: ideal model in clinics and research. Innov. (Camb) 5 (3), 100620. doi:10.1016/j.xinn.2024.100620
Heidarian, E., Keloushadi, M., Ghatreh-Samani, K., and Valipour, P. (2016). The reduction of IL-6 gene expression, pAKT, pERK1/2, pSTAT3 signaling pathways and invasion activity by gallic acid in prostate cancer PC3 cells. Biomed. Pharmacother. 84, 264–269. doi:10.1016/j.biopha.2016.09.046
Heydari, Z., Moeinvaziri, F., Agarwal, T., Pooyan, P., Shpichka, A., Maiti, T. K., et al. (2021). Organoids: a novel modality in disease modeling. Biodes Manuf. 4 (4), 689–716. doi:10.1007/s42242-021-00150-7
Hoofnagle, J. H., and Bjornsson, E. S. (2019). Drug-induced liver injury - types and phenotypes. N. Engl. J. Med. 381 (3), 264–273. doi:10.1056/NEJMra1816149
Inkielewicz-Stępniak, I. (2011). Impact of fluoxetine on liver damage in rats. Pharmacol. Rep. 63 (2), 441–447. doi:10.1016/s1734-1140(11)70510-2
Isvoran, A., Peng, Y., Ceauranu, S., Schmidt, L., Nicot, A. B., and Miteva, M. A. (2022). Pharmacogenetics of human sulfotransferases and impact of amino acid exchange on Phase II drug metabolism. Drug Discov. Today 27 (11), 103349. doi:10.1016/j.drudis.2022.103349
Jaeschke, H., Williams, C. D., Ramachandran, A., and Bajt, M. L. (2012). Acetaminophen hepatotoxicity and repair: the role of sterile inflammation and innate immunity. Liver Int. 32 (1), 8–20. doi:10.1111/j.1478-3231.2011.02501.x
Jagan, S., Ramakrishnan, G., Anandakumar, P., Kamaraj, S., and Devaki, T. (2008). Antiproliferative potential of gallic acid against diethylnitrosamine-induced rat hepatocellular carcinoma. Mol. Cell Biochem. 319 (1-2), 51–59. doi:10.1007/s11010-008-9876-4
Jarrar, Y., and Lee, S. J. (2021). The functionality of UDP-glucuronosyltransferase genetic variants and their association with drug responses and human diseases. J. Pers. Med. 11 (6), 554. doi:10.3390/jpm11060554
Jiashuo, W. U., Fangqing, Z., Zhuangzhuang, L. I., Weiyi, J., and Yue, S. (2022). Integration strategy of network pharmacology in Traditional Chinese Medicine: a narrative review. J. Tradit. Chin. Med. 42 (3), 479–486. doi:10.19852/j.cnki.jtcm.20220408.003
Juza, R. M., and Pauli, E. M. (2014). Clinical and surgical anatomy of the liver: a review for clinicians. Clin. Anat. 27 (5), 764–769. doi:10.1002/ca.22350
Kanai, S., and Okano, H. (1998). Mechanism of the protective effects of sumac gall extract and gallic acid on the progression of CCl4-induced acute liver injury in rats. Am. J. Chin. Med. 26 (3-4), 333–341. doi:10.1142/S0192415X98000373
Karimi-Khouzani, O., Heidarian, E., and Amini, S. A. (2017). Anti-inflammatory and ameliorative effects of gallic acid on fluoxetine-induced oxidative stress and liver damage in rats. Pharmacol. Rep. 69 (4), 830–835. doi:10.1016/j.pharep.2017.03.011
Kartkaya, K., Oglakci, A., Senturk, H., Bayramoğlu, G., Canbek, M., and Kanbak, G. (2013). Investigation of the possible protective role of gallic acid on paraoxanase and arylesterase activities in livers of rats with acute alcohol intoxication. Cell Biochem. Funct. 31 (3), 208–213. doi:10.1002/cbf.2874
Khateeb, J., Gantman, A., Kreitenberg, A. J., Aviram, M., and Fuhrman, B. (2010). Paraoxonase 1 (PON1) expression in hepatocytes is upregulated by pomegranate polyphenols: a role for PPAR-gamma pathway. Atherosclerosis 208 (1), 119–125. doi:10.1016/j.atherosclerosis.2009.08.051
Kim, S. H., Jun, C. D., Suk, K., Choi, B. J., Lim, H., Park, S., et al. (2006). Gallic acid inhibits histamine release and pro-inflammatory cytokine production in mast cells. Toxicol. Sci. 91 (1), 123–131. doi:10.1093/toxsci/kfj063
Kim, H., Castellon-Chicas, M. J., Arbizu, S., Talcott, S. T., Drury, N. L., Smith, S., et al. (2021). Mango (mangifera indica L.) polyphenols: anti-inflammatory intestinal microbial health benefits, and associated mechanisms of actions. Molecules 26 (9), 2732. doi:10.3390/molecules26092732
Kreider, R. B., JäGER, R., and Purpura, M. (2022). Bioavailability, efficacy, safety, and regulatory status of creatine and related compounds: a critical review. Nutrients 14 (5), 1035. doi:10.3390/nu14051035
Kumar, H., Kim, I. S., More, S. V., Kim, B. W., and Choi, D. K. (2014). Natural product-derived pharmacological modulators of Nrf2/ARE pathway for chronic diseases. Nat. Prod. Rep. 31 (1), 109–139. doi:10.1039/c3np70065h
Kumar, R., Shalimar, , Bhatia, V., Khanal, S., Sreenivas, V., Gupta, S. D., et al. (2010). Antituberculosis therapy-induced acute liver failure: magnitude, profile, prognosis, and predictors of outcome [J]. Hepatology, 51 (5), 1665–1674. doi:10.1002/hep.23534
Lai, X., Wu, A., Bing, Y., Liu, Y., Luo, J., Yan, H., et al. (2023). Retinoic acid protects against lipopolysaccharide-induced ferroptotic liver injury and iron disorders by regulating Nrf2/HO-1 and RARβ signaling. Free Radic. Biol. Med. 205, 202–213. doi:10.1016/j.freeradbiomed.2023.06.003
Liang, J., Xu, F., Zhang, Y. Z., Huang, S., Zang, X. Y., Zhao, X., et al. (2013). The profiling and identification of the absorbed constituents and metabolites of Paeoniae Radix Rubra decoction in rat plasma and urine by the HPLC-DAD-ESI-IT-TOF-MS(n) technique: a novel strategy for the systematic screening and identification of absorbed constituents and metabolites from traditional Chinese medicines. J. Pharm. Biomed. Anal. 83, 108–121. doi:10.1016/j.jpba.2013.04.029
Li, B., Shao, H., Gao, L., Li, H., Sheng, H., and Zhu, L. (2022b). Nano-drug co-delivery system of natural active ingredients and chemotherapy drugs for cancer treatment: a review. Drug Deliv. 29 (1), 2130–2161. doi:10.1080/10717544.2022.2094498
Lin, W.-H., Kuo, H. H., Ho, L. H., Tseng, M. L., Siao, A. C., Hung, C. T., et al. (2015). Gardenia jasminoides extracts and gallic acid inhibit lipopolysaccharide-induced inflammation by suppression of JNK2/1 signaling pathways in BV-2 cells. Iran. J. Basic Med. Sci. 18, 555–562.
Li, S., and Zhang, B. (2013). Traditional Chinese medicine network pharmacology: theory, methodology and application. Chin. J. Nat. Med. 11 (2), 110–120. doi:10.1016/S1875-5364(13)60037-0
Liu, Z. X., Govindarajan, S., and Kaplowitz, N. (2004). Innate immune system plays a critical role in determining the progression and severity of acetaminophen hepatotoxicity. Gastroenterology 127 (6), 1760–1774. doi:10.1053/j.gastro.2004.08.053
Li, X., Tang, J., and Mao, Y. (2022a). Incidence and risk factors of drug-induced liver injury. Liver Int. 42 (9), 1999–2014. doi:10.1111/liv.15262
Locatelli, C., Rosso, R., Santos-Silva, M. C., de Souza, C. A., Licínio, M. A., Leal, P., et al. (2008). Ester derivatives of gallic acid with potential toxicity toward L1210 leukemia cells. Bioorg Med. Chem. 16 (7), 3791–3799. doi:10.1016/j.bmc.2008.01.049
Luo, Y., Yang, W., Sun, H. Y., Sun, J., Dong, L., Chen, S. Y., et al. (2017). Studies on the metabolism of gallic acid and protocatechuic acid in rat intestinal flora [J]. Journal of Shenyang Pharmaceutical University, 34 (01), 43–47.
Mackowiak, B., Fu, Y., Maccioni, L., and Gao, B. (2024). Alcohol-associated liver disease. J. Clin. Invest. 134 (3), e176345. doi:10.1172/JCI176345
Maida, M., Orlando, E., Cammà, C., and Cabibbo, G. (2014). Staging systems of hepatocellular carcinoma: a review of literature. World J. Gastroenterol. 20 (15), 4141–4150. doi:10.3748/wjg.v20.i15.4141
Marchese, S., Polo, A., Ariano, A., Velotto, S., Costantini, S., and Severino, L. (2018). Aflatoxin B1 and M1: biological properties and their involvement in cancer development. Toxins (Basel) 10 (6), 214. doi:10.3390/toxins10060214
Marengo, A., Rosso, C., and Bugianesi, E. (2016). Liver cancer: connections with obesity, fatty liver, and cirrhosis. Annu. Rev. Med. 67, 103–117. doi:10.1146/annurev-med-090514-013832
Ma, S. (2014). Gallic acid attenuates dimethylnitrosamine-induced acute liver injury in mice through nrf2-mediated induction of heme oxygenase-1 and glutathione-s-transferase alpha 3. Med. Chem. 4 (9). doi:10.4172/2161-0444.1000208
Messina, A., Luce, E., Hussein, M., and Dubart-Kupperschmitt, A. (2020). Pluripotent-stem-cell-derived hepatic cells: hepatocytes and organoids for liver therapy and regeneration. Cells 9 (2), 420. doi:10.3390/cells9020420
Mita, S., Suzuki, H., Akita, H., Hayashi, H., Onuki, R., Hofmann, A. F., et al. (2006). Inhibition of bile acid transport across Na+/taurocholate cotransporting polypeptide (SLC10A1) and bile salt export pump (ABCB 11)-coexpressing LLC-PK1 cells by cholestasis-inducing drugs. Drug Metab. Dispos. 34 (9), 1575–1581. doi:10.1124/dmd.105.008748
Morgan, R. E., Trauner, M., Van Staden, C. J., Lee, P. H., Ramachandran, B., Eschenberg, M., et al. (2010). Interference with bile salt export pump function is a susceptibility factor for human liver injury in drug development. Toxicol. Sci. 118 (2), 485–500. doi:10.1093/toxsci/kfq269
Mori, T., Koyama, N., Yokoo, T., Segawa, T., Maeda, M., Sawmiller, D., et al. (2020). Gallic acid is a dual α/β-secretase modulator that reverses cognitive impairment and remediates pathology in Alzheimer mice. J. Biol. Chem. 295 (48), 16251–16266. doi:10.1074/jbc.RA119.012330
Niho, N., Shibutani, M., Tamura, T., Toyoda, K., Uneyama, C., Takahashi, N., et al. (2001). Subchronic toxicity study of gallic acid by oral administration in F344 rats. Food Chem. Toxicol. 39 (11), 1063–1070. doi:10.1016/s0278-6915(01)00054-0
Nogales, C., Mamdouh, Z. M., List, M., Kiel, C., Casas, A. I., and Schmidt, H. H. H. W. (2022). Network pharmacology: curing causal mechanisms instead of treating symptoms. Trends Pharmacol. Sci. 43 (2), 136–150. doi:10.1016/j.tips.2021.11.004
Nouri, A., Salehi-Vanani, N., and Heidarian, E. (2021). Antioxidant, anti-inflammatory and protective potential of gallic acid against paraquat-induced liver toxicity in male rats. Avicenna J. Phytomed 11 (6), 633–644. doi:10.22038/AJP.2021.18581
Omobowale, T. O., Oyagbemi, A. A., Ajufo, U. E., Adejumobi, O. A., Ola-Davies, O. E., Adedapo, A. A., et al. (2018). Ameliorative effect of gallic acid in doxorubicin-induced hepatotoxicity in wistar rats through antioxidant defense system. J. Diet. Suppl. 15 (2), 183–196. doi:10.1080/19390211.2017.1335822
Ow, Y. Y., and Stupans, I. (2003). Gallic acid and gallic acid derivatives: effects on drug metabolizing enzymes. Curr. Drug Metab. 4 (3), 241–248. doi:10.2174/1389200033489479
Owumi, S. E., Nwozo, S. O., Effiong, M. E., and Najophe, E. S. (2020b). Gallic acid and omega-3 fatty acids decrease inflammatory and oxidative stress in manganese-treated rats. Exp. Biol. Med. (Maywood) 245 (9), 835–844. doi:10.1177/1535370220917643
Owumi, S., Najophe, E. S., Farombi, E. O., and Oyelere, A. K. (2020a). Gallic acid protects against Aflatoxin B(1) -induced oxidative and inflammatory stress damage in rats kidneys and liver. J. Food Biochem. 44 (8), e13316. doi:10.1111/jfbc.13316
Paggi, J. M., Pandit, A., and Dror, R. O. (2024). The art and science of molecular docking. Annu. Rev. Biochem. 93 (1), 389–410. doi:10.1146/annurev-biochem-030222-120000
Park, W. H., Chang, M. S., Yang, W. M., Bae, H., Kim, N. I., and Park, S. K. (2007). Cytoprotective effect of Panax ginseng on gallic acid-induced toxicity in TM3 mouse Leydig cells. Fitoterapia 78 (7-8), 577–579. doi:10.1016/j.fitote.2007.03.019
Park, W., Chang, M. S., Kim, H., Choi, H. Y., Yang, W. M., Kim, D. R., et al. (2008). Cytotoxic effect of gallic acid on testicular cell lines with increasing H2O2 level in GC-1 spg cells. Toxicol Vitro 22 (1), 159–163. doi:10.1016/j.tiv.2007.08.010
Patil, P., and Killedar, S. (2021). Improving gallic acid and quercetin bioavailability by polymeric nanoparticle formulation. Drug Dev. Ind. Pharm. 47 (10), 1656–1663. doi:10.1080/03639045.2022.2043353
Perazzoli, M. R., Perondi, C. K., Baratto, C. M., Winter, E., Creczynski-Pasa, T. B., and Locatelli, C. (2017). Gallic acid and dodecyl gallate prevents carbon tetrachloride-induced acute and chronic hepatotoxicity by enhancing hepatic antioxidant status and increasing p53 expression. Biol. Pharm. Bull. 40 (4), 425–434. doi:10.1248/bpb.b16-00782
Prasanna, P. L., Renu, K., and Valsala Gopalakrishnan, A. (2020). New molecular and biochemical insights of doxorubicin-induced hepatotoxicity. Life Sci. 250, 117599. doi:10.1016/j.lfs.2020.117599
Price, D. A., Blagg, J., Jones, L., Greene, N., and Wager, T. (2009). Physicochemical drug properties associated with in vivo toxicological outcomes: a review. Expert Opin. Drug Metab. Toxicol. 5 (8), 921–931. doi:10.1517/17425250903042318
Prior, N., Inacio, P., and Huch, M. (2019). Liver organoids: from basic research to therapeutic applications. Gut 68 (12), 2228–2237. doi:10.1136/gutjnl-2019-319256
Rasool, M. K., Sabina, E. P., Ramya, S. R., Preety, P., Patel, S., Mandal, N., et al. (2010). Hepatoprotective and antioxidant effects of gallic acid in paracetamol-induced liver damage in mice. J. Pharm. Pharmacol. 62 (5), 638–643. doi:10.1211/jpp.62.05.0012
Reinke, H., and Asher, G. (2016). Circadian clock control of liver metabolic functions. Gastroenterology 150 (3), 574–580. doi:10.1053/j.gastro.2015.11.043
Rothwell, J. A., Perez-Jimenez, J., Neveu, V., Medinaremon, A., Mhiri, N., Garcialobato, P., et al. (2013). Phenol-Explorer 3.0: a major update of the Phenol-Explorer database to incorporate data on the effects of food processing on polyphenol content [J]. Oxford, bat070.
Safaei, F., Mehrzadi, S., Khadem Haghighian, H., Hosseinzadeh, A., Nesari, A., Dolatshahi, M., et al. (2018). Protective effects of gallic acid against methotrexate-induced toxicity in rats. Acta Chir. Belg 118 (3), 152–160. doi:10.1080/00015458.2017.1394672
Sahyon, H. A., El-Shafai, N. M., El-Mehasseb, I., Althobaiti, F., Aldhahrani, A., and Elnajjar, N. (2023). The anti-toxic effect of the date palm fruit extract loaded on chitosan nanoparticles against CCl(4)-induced liver fibrosis in a mouse model. Int. J. Biol. Macromol. 235, 123804. doi:10.1016/j.ijbiomac.2023.123804
Sanjay, S., Girish, C., Toi, P. C., and Bobby, Z. (2021). Gallic acid attenuates isoniazid and rifampicin-induced liver injury by improving hepatic redox homeostasis through influence on Nrf2 and NF-κB signalling cascades in Wistar Rats. J. Pharm. Pharmacol. 73 (4), 473–486. doi:10.1093/jpp/rgaa048
Saukkonen, J. J., Cohn, D. L., Jasmer, R. M., Schenker, S., Jereb, J. A., Nolan, C. M., et al. (2006). An official ATS statement: hepatotoxicity of antituberculosis therapy. Am. J. Respir. Crit. Care Med. 174 (8), 935–952. doi:10.1164/rccm.200510-1666ST
Schimites, P. I., Segat, H. J., Teixeira, L. G., Martins, L. R., Mangini, L. T., Baccin, P. S., et al. (2020). Gallic acid prevents ketamine-induced oxidative damages in brain regions and liver of rats. Neurosci. Lett. 714, 134560. doi:10.1016/j.neulet.2019.134560
Severi, T., Van Malenstein, H., Verslype, C., and van Pelt, J. F. (2010). Tumor initiation and progression in hepatocellular carcinoma: risk factors, classification, and therapeutic targets. Acta Pharmacol. Sin. 31 (11), 1409–1420. doi:10.1038/aps.2010.142
Shahrzad, S., Aoyagi, K., Winter, A., Koyama, A., and Bitsch, I. (2001). Pharmacokinetics of gallic acid and its relative bioavailability from tea in healthy humans. J. Nutr. 131 (4), 1207–1210. doi:10.1093/jn/131.4.1207
Shen, T., Liu, Y., Shang, J., Xie, Q., Li, J., Yan, M., et al. (2019). Incidence and etiology of drug-induced liver injury in mainland China. Gastroenterology 156 (8), 2230–2241. doi:10.1053/j.gastro.2019.02.002
Shruthi, S., and Shenoy, K. B. (2021). Gallic acid: a promising genoprotective and hepatoprotective bioactive compound against cyclophosphamide induced toxicity in mice. Environ. Toxicol. 36 (1), 123–131. doi:10.1002/tox.23018
Smith-Apeldoorn, S. Y., Veraart, J. K., Spijker, J., Kamphuis, J., and Schoevers, R. A. (2022). Maintenance ketamine treatment for depression: a systematic review of efficacy, safety, and tolerability. Lancet Psychiatry 9 (11), 907–921. doi:10.1016/S2215-0366(22)00317-0
Tanaka, M., Sato, A., Kishimoto, Y., Mabashi-Asazuma, H., Kondo, K., and Iida, K. (2020). Gallic acid inhibits lipid accumulation via AMPK pathway and suppresses apoptosis and macrophage-mediated inflammation in hepatocytes. Nutrients 12 (5), 1479. doi:10.3390/nu12051479
Tan, Y., Zhang, F., Fan, X., Lu, S., Liu, Y., Wu, Z., et al. (2023). Exploring the effect of Yinzhihuang granules on alcoholic liver disease based on pharmacodynamics, network pharmacology and molecular docking. Chin. Med. 18 (1), 52. doi:10.1186/s13020-023-00759-z
Tasduq, S. A., Peerzada, K., Koul, S., Bhat, R., and Johri, R. K. (2005). Biochemical manifestations of anti-tuberculosis drugs induced hepatotoxicity and the effect of silymarin. Hepatol. Res. 31 (3), 132–135. doi:10.1016/j.hepres.2005.01.005
Tomic, Z., Milijasevic, B., Sabo, A., Dusan, L., Jakovljevic, V., Mikov, M., et al. (2008). Diclofenac and ketoprofen liver toxicity in rat. Eur. J. Drug Metab. Pharmacokinet. 33 (4), 253–260. doi:10.1007/BF03190881
Tung, Y. T., Wu, J. H., Huang, C. C., Peng, H. C., Chen, Y. L., Yang, S. C., et al. (2009). Protective effect of Acacia confusa bark extract and its active compound gallic acid against carbon tetrachloride-induced chronic liver injury in rats. Food Chem. Toxicol. 47 (6), 1385–1392. doi:10.1016/j.fct.2009.03.021
Uzi, D., Barda, L., Scaiewicz, V., Mills, M., Mueller, T., Gonzalez-Rodriguez, A., et al. (2013). CHOP is a critical regulator of acetaminophen-induced hepatotoxicity. J. Hepatol. 59 (3), 495–503. doi:10.1016/j.jhep.2013.04.024
Variya, B. C., Bakrania, A. K., Madan, P., and Patel, S. S. (2019). Acute and 28-days repeated dose sub-acute toxicity study of gallic acid in albino mice. Regul. Toxicol. Pharmacol. 101, 71–78. doi:10.1016/j.yrtph.2018.11.010
Villanueva-Paz, M., Moran, L., Lopez-Alcantara, N., Freixo, C., Andrade, R. J., Lucena, M. I., et al. (2021). Oxidative stress in drug-induced liver injury (DILI): from mechanisms to biomarkers for use in clinical practice. Antioxidants (Basel) 10 (3), 390. doi:10.3390/antiox10030390
Volarevic, V., Djokovic, B., Jankovic, M. G., Harrell, C. R., Fellabaum, C., Djonov, V., et al. (2019). Molecular mechanisms of cisplatin-induced nephrotoxicity: a balance on the knife edge between renoprotection and tumor toxicity. J. Biomed. Sci. 26 (1), 25. doi:10.1186/s12929-019-0518-9
Wang, J., Tang, L., White, J., and Fang, J. (2014). Inhibitory effect of gallic acid on CCl4-mediated liver fibrosis in mice. Cell Biochem. Biophys. 69 (1), 21–26. doi:10.1007/s12013-013-9761-y
Wang, L., Sun, R., Zhang, Q., Luo, Q., Zeng, S., Li, X., et al. (2019). An update on polyphenol disposition via coupled metabolic pathways. Expert Opin. Drug Metab. Toxicol. 15 (2), 151–165. doi:10.1080/17425255.2019.1559815
Wang, X., Cong, J., Zhang, L., Jiang, X., and Yu, L. (2023a). Antiultraviolet, antioxidant, and antimicrobial properties and anticancer potential of novel environmentally friendly amide-modified gallic acid derivatives. J. Agric. Food Chem. 71 (41), 15352–15362. doi:10.1021/acs.jafc.3c04096
Wang, X., Zhou, Y., Wang, D., Wang, Y., Zhou, Z., Ma, X., et al. (2023b). Cisplatin-induced ototoxicity: From signaling network to therapeutic targets [J]. Biomed Pharmacother, 157, 114045. doi:10.1016/j.biopha.2022.114045
Weber, L. W., Boll, M., and Stampfl, A. (2003). Hepatotoxicity and mechanism of action of haloalkanes: carbon tetrachloride as a toxicological model. Crit. Rev. Toxicol. 33 (2), 105–136. doi:10.1080/713611034
Wu, C., Zhang, W., Yan, F., Dai, W., Fang, F., Gao, Y., et al. (2023). Amelioration effects of the soybean lecithin-gallic acid complex on iron-overload-induced oxidative stress and liver damage in C57BL/6J mice. Pharm. Biol. 61 (1), 37–49. doi:10.1080/13880209.2022.2151632
Wu, X., Wang, Y., Jia, R., Fang, F., Liu, Y., and Cui, W. (2019). Computational and biological investigation of the soybean lecithin-gallic acid complex for ameliorating alcoholic liver disease in mice with iron overload. Food Funct. 10 (8), 5203–5214. doi:10.1039/c9fo01022j
Yang, Y. M., Kim, S. Y., and Seki, E. (2019). Inflammation and liver cancer: molecular mechanisms and therapeutic targets. Semin. Liver Dis. 39 (1), 26–42. doi:10.1055/s-0038-1676806
Yang, X., Feng, Y., Liu, Y., Ye, X., Ji, X., Sun, L., et al. (2021). Fuzheng Jiedu Xiaoji formulation inhibits hepatocellular carcinoma progression in patients by targeting the AKT/CyclinD1/p21/p27 pathway. Phytomedicine 87, 153575. doi:10.1016/j.phymed.2021.153575
Yan, X., Zhang, Y. L., Zhang, L., Zou, L. X., Chen, C., Liu, Y., et al. (2019). Gallic acid suppresses cardiac hypertrophic remodeling and heart failure. Mol. Nutr. Food Res. 63 (5), e1800807. doi:10.1002/mnfr.201800807
Yisimayili, Z., Tian, Q., Xie, Y., Hu, P., Abdulla, R., Wu, T., et al. (2022). Simultaneous quantification of four compounds in rat plasma by HPLC-MS/MS and its application to pharmacokinetic study after oral administration of pomegranate flowers. J. Chromatogr. Sci. 60 (4), 348–356. doi:10.1093/chromsci/bmab077
You, B. R., and Park, W. H. (2011). Gallic acid-induced human pulmonary fibroblast cell death is accompanied by increases in ROS level and GSH depletion. Drug Chem. Toxicol. 34 (1), 38–44. doi:10.3109/01480545.2010.494182
Yu, X. A., Teye Azietaku, J., Li, J., Wang, H., Zheng, F., Hao, J., et al. (2018). Simultaneous quantification of gallic acid, bergenin, epicatechin, epicatechin gallate, isoquercitrin, and quercetin-3-rhamnoside in rat plasma by LC-MS/MS method and its application to pharmacokinetics after oral administration of Ardisia japonica extract. Evid. Based Complement. Altern. Med. 2018, 4964291. doi:10.1155/2018/4964291
Zhang, Y., Cheng, Y., Liu, Z., Ding, L., Qiu, T., Chai, L., et al. (2017). Systematic screening and characterization of multiple constituents in Guizhi Fuling capsule and metabolic profiling of bioactive components in rats using ultra-high-performance liquid chromatography/quadrupole-time-of-flight mass spectrometry. J. Chromatogr. B Anal. Technol. Biomed. Life Sci. 1061-1062, 474–486. doi:10.1016/j.jchromb.2017.07.021
Zhong, Y. X., Jin, X. L., Gu, S. Y., Peng, Y., Zhang, K. R., Ou-Yang, B. C., et al. (2016). Integrated identification, qualification and quantification strategy for pharmacokinetic profile study of Guizhi Fuling capsule in healthy volunteers. Sci. Rep. 6, 31364. doi:10.1038/srep31364
Zhou, D., Yang, Q., Tian, T., Chang, Y., Li, Y., Duan, L. R., et al. (2020). Gastroprotective effect of gallic acid against ethanol-induced gastric ulcer in rats: involvement of the Nrf2/HO-1 signaling and anti-apoptosis role. Biomed. Pharmacother. 126, 110075. doi:10.1016/j.biopha.2020.110075
Zhou, S., Zhang, H., Li, J., Li, W., Su, M., Ren, Y., et al. (2024). Potential anti-liver cancer targets and mechanisms of kaempferitrin based on network pharmacology, molecular docking and experimental verification. Comput. Biol. Med. 178, 108693. doi:10.1016/j.compbiomed.2024.108693
Zhou, Y., Jin, H., Wu, Y., Chen, L., Bao, X., and Lu, C. (2019). Gallic acid protects against ethanol-induced hepatocyte necroptosis via an NRF2-dependent mechanism. Toxicol Vitro 57, 226–232. doi:10.1016/j.tiv.2019.03.008
Keywords: hepatotoxicity, DILI, gallic acid, molecular mechanisms, bioavailability
Citation: Chen P, Zou F and Liu W (2025) Recent advancement in prevention against hepatotoxicity, molecular mechanisms, and bioavailability of gallic acid, a natural phenolic compound: challenges and perspectives. Front. Pharmacol. 16:1549526. doi: 10.3389/fphar.2025.1549526
Received: 21 December 2024; Accepted: 19 February 2025;
Published: 21 March 2025.
Edited by:
Ralf Weiskirchen, RWTH Aachen University, GermanyReviewed by:
Alaadin E. El-Haddad, October 6 University, EgyptCopyright © 2025 Chen, Zou and Liu. This is an open-access article distributed under the terms of the Creative Commons Attribution License (CC BY). The use, distribution or reproduction in other forums is permitted, provided the original author(s) and the copyright owner(s) are credited and that the original publication in this journal is cited, in accordance with accepted academic practice. No use, distribution or reproduction is permitted which does not comply with these terms.
*Correspondence: Peng Chen, MjI4Mjk2ODkwOEBxcS5jb20=
†These authors have contributed equally to this work
Disclaimer: All claims expressed in this article are solely those of the authors and do not necessarily represent those of their affiliated organizations, or those of the publisher, the editors and the reviewers. Any product that may be evaluated in this article or claim that may be made by its manufacturer is not guaranteed or endorsed by the publisher.
Research integrity at Frontiers
Learn more about the work of our research integrity team to safeguard the quality of each article we publish.