- 1Student research Committee, Arak University of Medical Sciences, Arak, Iran
- 2Student Research Committee, Khomein University of Medical Sciences, Khomein, Iran
- 3Department of Microbiology, School of Medicine, Hamadan University of Medical Sciences, Hamadan, Iran
- 4Infectious Diseases Research Center (IDRC), Arak University of Medical Sciences, Arak, Iran
Streptococcus mutans is recognized as one of the leading causes of dental caries, and biofilm formation by this bacterium plays a key role in dental plaque development and caries progression. Given the increasing resistance of bacteria to antibiotics and the adverse effects of some synthetic antimicrobials, the search for natural alternatives has received increasing attention. The recently published studies have demonstrated that natural compounds (NCs) such as curcumin, cinnamaldehyde, eugenol, thymol, carvacrol, epigallocatechin gallate, farnesol, catechin, inulin, menthol, apigenin, myricetin, oleanolic acid, and resveratrol, have notable antimicrobial properties and can effectively inhibit the growth of Streptococcus mutans. NCs can disrupt bacterial membrane integrity, leading to cell death, and possess the capability to inhibit acid production, which is a key factor in caries development. NCs can also interfere with bacterial adhesion to surfaces, including teeth. The attachment inhibition is achieved by decreasing the expression of adhesion factors such as gtfs, ftf, fruA, and gbpB. NCs can disrupt bacterial metabolism, inhibit biofilm formation, disperse existing biofilm, and interfere with quorum sensing and two-component signal transduction systems. Moreover, novel drug delivery platforms were used to enhance the bioavailability and stability of NCs. Studies have also indicated that NCs exhibit significant efficacy in combination therapies. Notably, curcumin has shown promising results in photodynamic therapy against S. mutans. The current review article analyzes the mechanisms of action of various NCs against S. mutans and investigates their potential as alternative or complementary therapeutic options for managing this bacterium and dental caries.
Introduction
Dental caries is a prevalent chronic infectious disease affecting the teeth’ hard tissues. Dental caries and its sequelae can aggravate or trigger systemic disorders, significantly diminishing the quality of human life. Dental caries has a complicated etiology; two primary causative variables are the regular intake of free sugars and the metabolic activity of certain commensal, tooth-adherent bacteria. Numerous oral microbes generate organic acids through the metabolism of fermentable carbohydrates. These acids lower salivary pH, leading to the demineralization of tooth tissue. A notable diversity of microbe species linked to dental caries has been identified, including Streptococcus mutans, the most prevalent bacterium observed in individuals with dental caries (Bhaumik et al., 2021).
This bacterium is a Gram-positive, facultative anaerobic, and catalase-negative bacterium that generates lactic acid and can lower the environmental pH from seven to 4.2 within approximately 24 h. Furthermore, it can ferment and create acids from carbohydrates, including glucose, lactose, and raffinose, which is why it is identified as the primary pathogen in the onset of dental caries. This bacterium can degrade carbohydrates and synthesize glucan, which is crucial for interacting with dental structures (Lemos et al., 2019). Carbohydrates like sucrose are metabolized to create intracellular and extracellular polysaccharides. The synthesis of extracellular polysaccharides (EPS) facilitates bacterial adherence and accumulation on the tooth surface, resulting in structural alterations such as enhanced porosity in dental biofilms (Liu et al., 2018; Lemos et al., 2019).
To endure the challenging conditions of the human oral cavity, S. mutans and other oral microorganisms establish a highly structured microbial consortium known as a biofilm (Marsh and Zaura, 2017). Biofilm is a complex structure composed of aggregated microbial cells and microbially produced extracellular polymeric substances (Marsh and Zaura, 2017).
Following initial adhesion, bacteria such as S. mutans begin to grow and synthesize extracellular polymeric substances, forming a stable three-dimensional community that incorporates pathways to distribute nutrients, oxygen, and signalling chemicals efficiently. This bacterium substantially enhances biofilm formation via both sucrose-dependent and sucrose-independent mechanisms. The sucrose-dependent mechanism mostly depends on the extracellular glucose transferase. Streptococcus mutans secretes three extracellular glucose transferases: GtfB, GtfC, and GtfD. GtfB primarily generates viscous, water-insoluble polysaccharides from sucrose, whereas GtfC produces a combination of insoluble and soluble polysaccharides from sucrose. GtfD mainly catalyzes the conversion of sucrose into soluble polysaccharides. The sucrose-independent mechanism involves contact between the sticky particles of S. mutans and the acquired enamel pellicle. Agglutinins present in saliva facilitate the adherence and aggregation of S. mutans by their interaction with the I/II antigen, a multifunctional PI adhesin that is anchored in the bacterial cell wall and encoded by the spaP gene (Bowen and Koo, 2011; Krzyściak et al., 2014).
Numerous techniques exist for addressing oral and dental ailments, including non-surgical and surgical interventions and adjuvant therapies such as antibiotics. Although antibiotics are helpful, excessive dependence on them might result in the emergence of resistant bacterial strains, diminishing their potency over time. Furthermore, broad-spectrum antibiotics can disturb the normal equilibrium of the oral microbiota, potentially resulting in opportunistic infections or other oral health complications (Serino et al., 2001; Ryan, 2005). To this end, certain plants without undesirable side effects have demonstrated more efficacy than manufactured medications in preventing dental caries (Malvania et al., 2019). The natural compounds derived from various plant components, including roots, leaves, and fruits, have distinct medical effects when subjected to alterations (Rangel et al., 2018). Most antibacterial compounds in plants often encompass flavonoids, phenols, alkaloids, and organic acids (Shad et al., 2014). Multiple studies have indicated that managing dental biofilm is essential for preventing tooth decay. Quorum sensing (QS) is a crucial virulence regulator in cariogenic biofilms. Biofilm generation relies on the signal-mediated QS system. Plant extracts can block QS genes, disrupting biofilm formation (Choi et al., 2017; Balhaddad et al., 2019). In addition, these compounds also inhibit glucosyltransferase, which is crucial in creating water-insoluble glucan, preventing the development of cariogenic biofilms (Yabuta et al., 2018; Farkash et al., 2020).
Because of its consistently high frequency and exorbitant treatment costs, dental caries continues to be a significant problem with significant long-term health, economic, and societal effects (Hescot, 2017; Kassebaum et al., 2017). To this end, finding new therapeutic approaches for managing S. mutans-associated dental caries is necessary. In the present review article, we will discuss the interactions of natural compounds with S. mutans to improve the scientists’ knowledge of using these compounds for managing dental caries.
Curcumin
Curcumin is an orange-yellow pigment in the rhizome of Curcuma longa and demonstrates a broad spectrum of medicinal actions, including antibacterial and antiseptic properties (Supplementary Table S1) (Kashi et al., 2024). Studies have investigated and confirmed the antibacterial properties of curcumin against S. mutans (Song et al., 2012; Hu P. et al., 2013). For instance, one study reported that curcumin’s minimum inhibitory concentration (MIC) against S. mutans was 64 μM (Ke et al., 2023). This bacterium metabolizes carbohydrates, resulting in medium acidity. Streptococcus mutans markedly alters the fatty acid composition of its membrane in response to ambient acidity, implicating fatty acid metabolism (Baker et al., 2017). Curcumin can affect fatty acid, carbon and pyruvate metabolism in S. mutans. These metabolic pathways are crucial for the bacteria’s survival as they are its principal energy sources (Guzmán-Flores et al., 2024). Curcumin also influences DNA replication and the metabolism of purines and pyrimidines in S. mutans. Modifying these metabolic pathways will likely lead to bacterial mortality (Guzmán-Flores et al., 2024). Notably, a primary virulence component of S. mutans is the unique lipid composition of its membrane, which varies with pH; thus, curcumin modulates the molecules implicated in the lipid response (Guzmán-Flores et al., 2024).
As mentioned, S. mutans metabolises carbohydrates, generating an acidic microenvironment in tooth plaque. Therefore, this bacterium must have a mechanism for enduring acidic environments. The enzyme F-ATPase regulates cytoplasmic pH by extruding protons from the cytoplasm in acidic environments (Sekiya et al., 2019). A study reported that the expression of the F-ATPase gene in S. mutans was increased in acidic environments (Kuhnert et al., 2004). However, researchers proposed that this enzyme is non-essential for bacterial proliferation under neutral circumstances. Curcumin significantly diminished the ATPase activity of S. mutans F-ATPase and suppressed the proliferation of this bacterium at pH 5.3. The data demonstrated that S. mutans exhibited significant sensitivity to F-ATPase inhibitors in acidic environments, highlighting the critical function of F-ATPase in the acid tolerance of this bacterium (Sekiya et al., 2019). Moreover, a study revealed that the atpH gene, which encodes subunit C of the F-ATPase enzyme, was downregulated in S. mutans following curcumin treatment (Li et al., 2018). Thus, curcumin can impede the acid tolerance capacity of S. mutans and likely diminish its cariogenic characteristics (Galvão et al., 2015). Alongside acid stress, oxidative stress constitutes a primary environmental obstacle encountered by S. mutans in the oral environment (Galvão et al., 2015). This bacterium exhibited susceptibility to hydrogen peroxide (H2O2), which is generated through the metabolic processes of other species in dental plaque (Higuchi et al., 1999). Exposure to elevated concentrations of H2O2 and its deleterious byproducts, hydroxyl and superoxide anions, can induce irreversible cellular damage. Spx, comprising two homologues, SpxA1 and SpxA2, regulates the transcription of nearly all principal activated oxidative stress response genes in S. mutans (Baker et al., 2014; Kajfasz et al., 2017; Ganguly et al., 2020). Ke et al. found that curcumin treatment increased SpxA1 and SpxA2 levels, perhaps leading to increased H2O2 generation through the activation of oxidative stress response genes (Ke et al., 2023). All these effects that occur in the bacteria when exposed to curcumin can lead to growth inhibition and cell death.
In addition, curcumin can impede biofilm formation and markedly affect the biofilm development of S. mutans (Ke et al., 2023). This compound diminished the quantity of viable and total bacteria within the biofilm and decreased the biofilm’s thickness. The diminution in biofilm thickness would result in the alteration of the three-dimensional structures of the biofilm, potentially impacting the cariogenicity of S. mutans (Li B. et al., 2020). Furthermore, curcumin prevented the biofilm development of S. mutans by disrupting EPS (Bottner et al., 2020). This compound can significantly reduce the amounts of EPS in biofilm and destroy the structure of EPS in the short term, decreasing the EPS biomass (Li et al., 2018; Li et al., 2019; Li B. et al., 2020). Furthermore, the expression of gbpB was downregulated after curcumin treatment (Li et al., 2018; Li B. et al., 2020). Bacterial aggregation is a crucial factor in the production of EPS, facilitated by interactions among surface-associated glucan-binding proteins (GbpBs) that stick to glucan, thus enhancing plaque formation (He et al., 2012). The absence or mutation of the gene encoding GbpB leads to alterations in cell morphology and a deceleration of growth. The mentioned change inhibits the proper formation of biofilm, which results from irregular cell clusters encased in a matrix of atypical structure (Duque et al., 2011). The inhibitory effect of curcumin on EPS and GbpB can significantly decrease biofilm formation.
Curcumin can downregulate the expression of rgpG, scrAB, gtfB, gtfC, gtfD, ftf and fruA (Li et al., 2018; Li et al., 2019; Li B. et al., 2020; Ke et al., 2023). As previously mentioned, the Gtfs are essential enzymes for bacteria to utilize sucrose and form glucan. Reduced gtfB, gtfC, and gtfD expression disrupts GbpB-mediated bacterial aggregation (Li B. et al., 2020). RgpG is a protein associated with the cell envelope that is implicated in the biofilm development of S. mutans (De et al., 2017). A loss in the rgpG gene leads to a significant decrease in cell surface antigens and substantial abnormalities in cell shape and division without affecting growth (De et al., 2017; Bischer Andrew et al., 2020). RgpG-deficient mutants showed elongated chains of inflated “Square” developing cells and produced less biofilms irrespective of the carbohydrate source. Curcumin downregulated rgpG, indicating that it influences cell division and diminishes biofilm formation via targeting rgpG (De et al., 2017). Furthermore, both ftf and fruA expressions were dysregulated following short-term exposure to curcumin. Fucosyltransferase (FTF) is an enzyme that catalyzes the conversion of sucrose into extracellular homopolymers of fructose, known as fructans. FTF, derived from the fruA gene, is an exo-β-d-fructosidase that liberates fructose from β(2.6)- and β(2.1)-linked fructans, as well as cleaving fructose from sucrose and raffinose (Li et al., 2018). Consequently, the quantity of fructans synthesized by the bacterium is markedly diminished after treatment with curcumin. This reduction diminished the bacterium’s capacity to adhere, hindering its effective utilization of sucrose as an energy source (Li et al., 2018). There is a decreasing tendency in the VicR expression of S. mutans after curcumin treatment (Li et al., 2018). VicR functions as a response regulator by binding to the promoter regions of the gtfB, gtfC, and ftf genes, thereby activating these genes and promoting biofilm formation (Senadheera et al., 2005). Therefore, the reduction of VicR negatively impacts the biofilm formation process. In the end, the scrA gene in the phosphotransferase system (PTS) of S. mutans encodes a high-affinity permease that facilitates the internalization of sucrose. Subsequently, intracellular sucrose-6-phosphate is initially hydrolyzed by ScrB, a sucrose-6-phosphate hydrolase, yielding fructose and glucose-6-phosphate (Li et al., 2018). The scrAB genes were downregulated in the presence of curcumin, diminishing the availability of sucrose and its derivatives within the cell. This alteration impacts biofilm formation, as sucrose is a primary energy source and nutrient for establishing S. mutans biofilms.
QS systems play a crucial role in regulating biofilm development and activating virulence factors in various bacteria, making them an attractive target for combating biofilm infections. Two extensively researched QS systems exist in S. mutans: the competence-stimulating peptide-QS (CSP-QS) system, which comprises a competence-stimulating peptide (encoded by comC), a histidine kinase sensor protein (encoded by comD), and a cognate response regulator (encoded by comE). This system facilitates intraspecies cell-cell communication and positively regulates the expression of biofilm-related genes such as gtfB, gtfC, and gbpB in S. mutans. The second system is the LuxS system, which catalyzes the synthesis of the signaling peptide Autoinducer 2 (AI-2) to mediate interspecies and intraspecies interactions within the multispecies plaque community (Li et al., 2018; Li et al., 2019). To this end, recently published studies reported that curcumin may reduce the number of viable bacteria in the biofilm and diminish the biomass of EPS in S. mutans biofilm by suppressing the expression of the LuxS system and ComCDE system (Li et al., 2018; Li et al., 2019).
Consequently, as previously stated, curcumin has demonstrated encouraging efficacy against S. mutans. Nonetheless, the low water solubility, inadequate stability, rapid clearance rate, and restricted bioavailability of curcumin have constrained its clinical utilization (Hu et al., 2023). In recent years, nanodrugs delivery technologies have been extensively employed across diverse domains. Nanoparticles can be engineered to induce drug release under various conditions, ensuring that drugs remain unaffected by pH, enzymes, and other variables. Nanoparticles effectively enhance the accessibility of water-insoluble or sparingly soluble bioactive compounds. Hazzah et al. synthesized and analysed curcumin solid lipid nanoparticles (CurSLN) to manage oral mucosal infections. CurSLN had superior antibacterial efficacy to raw curcumin and chemically stabilized curcumin, demonstrating a MIC of 0.09375 mg/mL against S. mutans (Hazzah et al., 2015). In another study, a bio-nanocomposite comprising carboxymethyl starch (CMS), chitosan (CS), and montmorillonite (MMT) was formulated for the delivery of curcumin. The curcumin-loaded bionanocomposite significantly inhibited biofilm growth in dental models (Jahanizadeh et al., 2017). Moreover, researchers assessed the mechanical characteristics and antibacterial efficacy of varying doses of Curcumin-Nisin-poly (L-lactic acid) nanoparticles (CurNisNps) incorporated into orthodontic acrylic resin against S. mutans and Candida albicans. The study results demonstrated significant anti-biofilm efficacy of the synthesized platform against microorganisms throughout a 60-day follow-up period. Furthermore, CurNisNps substantially diminished the expression levels of gtfB (Pourhajibagher et al., 2022). Hence, in recent years, nanotechnology has revolutionized the properties of materials. Researchers have increased its pharmacokinetics properties and antimicrobial activity by incorporating curcumin into nanocarriers, leading to more effective infection treatments.
Photodynamic therapy (PDT) is a therapeutic approach that utilizes visible light with photosensitizers (PS) or dyes. The PS attaches to the target cell membrane and, when exposed to visible light at a specific wavelength (unique to each PS), induces the generation of various reactive oxygen species (ROS), including singlet oxygen, which triggers a cascade of biological events resulting in apoptosis of cells or the death of microorganisms (Paulino et al., 2005; Paschoal et al., 2015). Curcumin predominantly absorbs light in the wavelength range of 400–500 nm; therefore, a suitable light source must be utilized when employing curcumin as a PS (Figure 1) (Table 1) (Paschoal et al., 2013). The application of curcumin in an in vivo study revealed no burning sensation, mouth discomfort, or ulcers (Araújo et al., 2012b). In this regard, a study examined the differential impacts of nanomicelle curcumin-based PDT (NMCur-aPDT) on the microbial population and pathogenicity of S. mutans. The results indicated that the antibacterial and anti-virulence efficacy of NMCur-aPDT against S. mutans surpassed that of the other treatment groups, and the gene expression level of gtfB diminished (Hosseinpour-Nader et al., 2022). Moreover, Hu et al. engineered a liposome with adhesive characteristics to transport curcumin into the biofilm. This research demonstrated that curcumin can be released from the liposome near the biofilm, exerting an antibacterial impact by dispersing the biofilm under blue light irradiation (Hu et al., 2023). Therefore, PDT, curcumin, and novel drug delivery systems provide an innovative approach to treating bacterial infections, especially S. mutans.
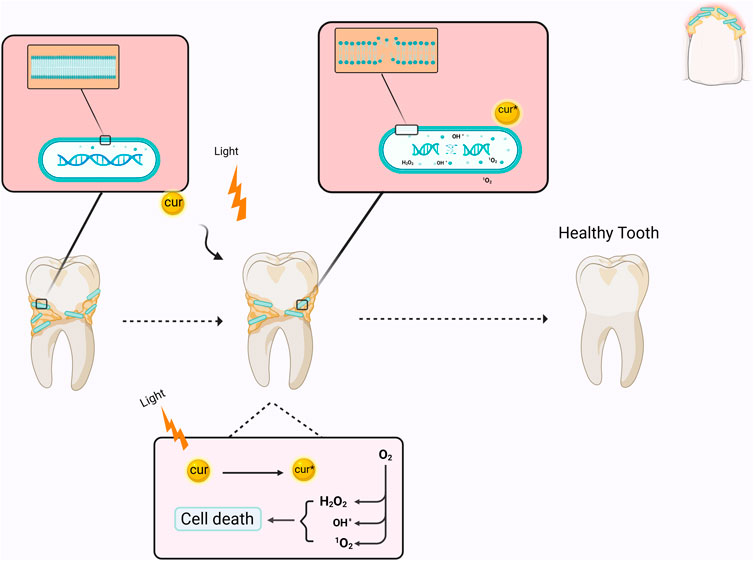
Figure 1. Schematic illustration of the mechanism of curcumin as a photosensitizer in PDT. When curcumin is excited by light irradiation, it converts O2 into reactive oxygen species, which causes bacterial death by affecting the membrane and DNA. Cur: curcumin.
Curcumin has shown significant antibacterial activity against S. mutans, influencing bacterial metabolic pathways and reducing its acid tolerance capacity. This compound also possesses anti-biofilm properties that can affect all stages of biofilm formation. As a photosensitizer, curcumin exerts its antibacterial effects by generating ROS. However, considering its limited pharmacokinetic properties, innovative drug delivery platforms can be employed to enhance its effectiveness.
Cinnamaldehyde
Cinnamaldehyde is a bioactive chemical extracted from cinnamon bark, recognized for its varied effects, including antifungal and antibacterial characteristics (Kashi et al., 2024). Researchers have lately shown interest in utilizing the antibacterial properties of cinnamaldehyde to tackle S. mutans (Sharma et al., 2016; de Almeida et al., 2020; Ngokwe et al., 2024). In one study, the minimum bactericidal concentration (MBC) values of trans-cinnamaldehyde against planktonic S. mutans were reported to be 1728 μg/mL. At this concentration, trans-cinnamaldehyde also caused a 50% reduction in biofilm metabolic activity (Ribeiro et al., 2018). The hydrophobic properties of cinnamaldehyde facilitate its contact with the cell membrane of S. mutans (Ribeiro et al., 2018). Furthermore, the topological polar surface area (TPSA) < 40 Å2 reported for cinnamaldehyde suggests it may possess a favourable capacity for permeating cell membranes, as only compounds with TPSA >140 Å2 often exhibit poor permeability (Veber et al., 2002). Highly hydrophobic chemicals are typically associated with increased toxicity, and the cytoplasmic membrane frequently serves as the principal target for antimicrobial activity. Lipophilic substances exhibit a strong affinity for cell membranes via altering the physicochemical properties of the membrane (Figure 2) (Ribeiro et al., 2018).
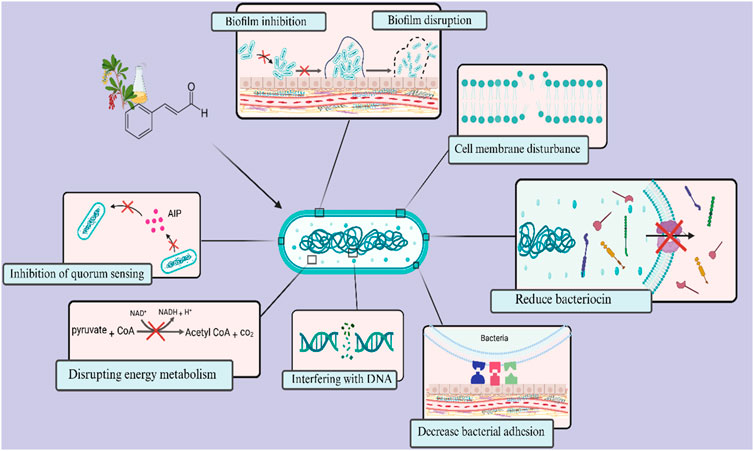
Figure 2. Overview of antimicrobial targets of cinnamaldehyde against Streptococcus mutans. Cinnamaldehyde primarily possesses the ability to penetrate cellular membranes and alter their physicochemical properties. Additionally, it can disrupt the energy metabolism and ATP production in bacteria. Furthermore, it diminishes bacterial survival by influencing the expression of genes associated with bacteriocin production and DNA replication and maintenance. This compound also impacts the formation and maturation of biofilms, affecting critical stages such as surface adhesion and intercellular communication through the quorum sensing system.
Cinnamaldehyde can interfere with carbohydrate metabolism, glycolysis, pyruvate metabolism, and the tricarboxylic acid (TCA) cycle, as well as arginine, tryptophan, and proline metabolism of S. mutans. Researchers speculate that the primary mechanism of action of cinnamaldehyde against S. mutans is targeting pyruvate dehydrogenase (PDH), which affects its downstream TCA cycle pathway, thereby inhibiting energy production. This energy level reduction impacts the bacteria’s upstream carbohydrate metabolism and the glycolytic pathway. Additionally, amino acid metabolism is affected (Zhang et al., 2024). Noteworthy, the TCA cycle is critical in energy metabolism, and PDH is one of the key enzymes entering the TCA cycle. In S. mutans, the TCA cycle has been proven incomplete, and its principal significance is in producing intermediates for other metabolisms (Zhang et al., 2024). By acting on PDH, cinnamaldehyde interferes with the downstream TCA cycle, which could not further generate other metabolic intermediates. The pyruvate-related genes (pdhA, pdhB, pdhC, and pdhD), which encode different subunits of the PDH enzyme complex, were downregulated after the treatment of cinnamaldehyde (Zhang et al., 2024). Additionally, expression of the atpD gene was downregulated in the presence of cinnamaldehyde (Balasubramanian et al., 2021). The atpD gene in S. mutans encodes the β-subunit of the ATP synthase enzyme, which is essential for cellular energy production (Gabe et al., 2019). Therefore, cinnamaldehyde can disturb cell membranes and inhibit energy metabolism and ATP production of S. mutans (Zhang et al., 2024).
Streptococcus mutans has a strong ability to acid production (acidogenicity) and acid resistance (acidurance), and it can convert glucose into lactic acid and other acidic substances by glycolysis (He et al., 2019; Zhang et al., 2024). The findings of recently published studies showed that cinnamaldehyde suppressed glucose and sucrose consumption in S. mutans and downregulated the expression levels of glycolysis-related genes, such as eno, ldh, and pykf (He et al., 2019; Zhang et al., 2024). Under excess carbohydrate and oxygen deficiency conditions, S. mutans tend to convert pyruvate to lactic acid by lactate dehydrogenase (LDH) and convert NADH to NAD again. The accumulation of lactic acid decreases the pH (Abranches et al., 2018). With the cinnamaldehyde interfering with the glycolysis pathway and reducing the LDH activities, the acid production is inhibited, and then the terminal pH increases (He et al., 2019; Zhang et al., 2024). DNA repair processes are enhanced in response to acidogenicity to sustain homeostasis (Senadheera et al., 2009). The genes atpD, dnaK (associated with stress tolerance), and the DNA repair mechanisms idh and recA were consistently downregulated, inhibiting stress tolerance in the presence of cinnamaldehyde (Balasubramanian et al., 2021). In addition, relA gene expressions were downregulated by cinnamaldehyde (He et al., 2019). Guanosine tetra (penta)-phosphate synthetize/hydrolase is encoded by the relA gene, and it is implicated in various processes, including acid and oxidative stress tolerance mechanisms, as well as biofilm production (Lemos José et al., 2004). Furthermore, cinnamaldehyde can decrease the expression of the brpA gene. It has a role in cell wall integrity, which is important to deal with physical and chemical stresses in the environment. The brpA also is known to control recA, dnaK, and atpD (Balasubramanian et al., 2021). As a result, the survival rate of bacteria in an acidic environment and acid resistance of S. mutans gradually decreased after treatment with cinnamaldehyde (He et al., 2019; Zhang et al., 2024).
Bacteriocin immunity proteins are crucial for conferring immunity to bacterial cells. Bacteriocin immunity proteins-related genes, such as immA and immB, nisin-like mutacin C (nlmC), and bsmI, were downregulated by cinnamaldehyde (Balasubramanian et al., 2021). The atlA gene also was downregulated by cinnamaldehyde (Balasubramanian et al., 2021). This gene encodes an autolysin enzyme essential for cell wall biogenesis, facilitating cell growth and division in S. mutans (Ahn and Burne, 2006). In the end, a significant downregulation of ftsZ and gyrA was detected after cinnamaldehyde treatment, suggesting that this compound affects the development and metabolism of S. mutans. The gyrA gene plays a critical role in DNA replication and maintenance of genomic stability, and ftsZ is essential in cell division (Marchese and Debbia, 2016; Barrows and Goley, 2021). Thus, cinnamaldehyde can compromise the viability of S. mutans.
The interactions between the cinnamaldehyde and S. mutans biofilm community are also interesting. The results of the studies showed that S. mutans biofilms were highly dispersed and visibly loose, and there was an irregular distribution of biofilm with reduced biomass following cinnamaldehyde treatment, which can be readily eradicated by host clearance mechanisms (He et al., 2019; Balasubramanian et al., 2021). He et al. reported that cinnamaldehyde might attenuate biofilm development during the initial adhesion and maturation stages. Moreover, this compound enhanced the hydrophobicity and reduced the aggregation of S. mutans (He et al., 2019). A decrease in the attachment and aggregation of bacteria can be related to the downregulation of gtfB, gtfC, gtfD, gbpB, spaP and ftf genes (He et al., 2019; Balasubramanian et al., 2021; Zhang et al., 2024). Also, the ciaH, covR and vicR genes were downregulated by cinnamaldehyde (He et al., 2019). CiaRH is a significant two-component signal transduction systems (TCSTS) associated with biofilm development, acid tolerance, and genetic competence. The removal of the ciaH gene impacts bacterial adhesion, decreases sucrose-independent biofilm formation, eliminates mutacin synthesis, and reduces competence development (Wu et al., 2010). Moreover, the VicRK system and the orphan regulator CovR of S. mutans collaboratively regulate virulence genes. VicR and CovR directly regulate a set of genes, including gtfB/C/D and gbpC, which are involved in the synthesis and interaction with extracellular polysaccharides; alterations in their expression can affect attachment and biofilm formation (Stipp et al., 2013). Finally, cinnamaldehyde also affects the QS system. It can downregulate the expression level of QS-related genes, including comA, comE, comS, comR, comDE, comB, comEC, and luxS (Balasubramanian et al., 2021; Zhang et al., 2024). The downregulation of luxS may lead to the downregulation of brpA, comDE, vicR, recA, and spaP (Wen et al., 2011). Therefore, cinnamaldehyde can interfere with biofilm formation by S. mutans in various ways.
Researchers have devised many techniques to administer cinnamaldehyde to enhance its bioavailability in vivo (Zaltsman et al., 2017; Jailani et al., 2022; Mu et al., 2023). A study investigated the efficacy of trans-cinnamaldehyde (TC) encapsulated in porous silicon (pSi) particles in inhibiting biofilm formation. The results indicated that pSi-TC at concentrations of 0.5 mg/mL and 1.15 mg/mL reduced the development of S. mutans biofilm by 78% and 85%, respectively. The synthesized particles decreased lactic acid production by 25.11% in biofilms and significantly downregulated the S. mutans genes responsible for inter-species communication and biofilm development (luxS), genes that regulate heat and acid-induced stress (dnaK and atpD), and oxidative stress tolerance (nox1). Moreover, pSi-TC markedly downregulated the genes encoding glucosyl transferases (gtfB and gtfC) (Jailani et al., 2022). In another study, a nanosystem designed to combat caries was developed by encapsulating cinnamaldehyde within chitosan-based nanocapsules (CA@CS NC). The authors proposed that CA@CS NC can adsorb the bacterial membrane through electronic interactions and release cinnamaldehyde over an extended period. Simultaneously, the nanoparticles demonstrated consistent antibacterial efficacy against S. mutans and reduced the expression levels of QS, virulence, biofilm, and adhesion genes, including gtfB, gtfC, and gtfD (Mu et al., 2023). Consequently, using nanoparticles to transport cinnamaldehyde presents novel opportunities to enhance its application in creating antibacterial and antibiofilm agents.
Eugenol
Eugenol, or 4-allyl-2-methoxyphenol, is a fragrant oily liquid derived from certain essential oils, notably clove and cinnamon. It has been a flavoring ingredient in culinary and cosmetic preparations (Didehdar et al., 2022). Eugenol has shown good antibacterial activity against S. mutans. In one study, its MBC value against this bacterium was 1,642 μg/mL (Ribeiro et al., 2018). This compound, like cinnamaldehyde, has a hydrophobic state that allows it to interact with the cell membrane of S. mutans (Ribeiro et al., 2018). In addition, a TPSA of <40 Å2 has been reported for this compound, suggesting it can penetrate the cell membrane (Veber et al., 2002). Eugenol impeded the decrease in pH caused by S. mutans. The findings indicated that eugenol can diminish acid generation by S. mutans (Xu et al., 2013). As previously mentioned, acidogenicity is one of the important factors associated with dental caries, and its disruption by eugenol reduces its activity for demineralization.
The antibiofilm efficacy of eugenol is evidenced by the decrease in the proportion of biofilm formation in its presence. Biofilms treated with eugenol exhibited deformed cellular structures, indicating the significant effect of eugenol on cell architecture and cellular damage, perhaps leading to the release of intracellular material. Significantly, biofilm developed in the presence of eugenol exhibited reduced cell aggregation and biofilm disorganization, with a notable reduction in colony-forming units (CFU) and matrix production (Jafri et al., 2019; Jafri et al., 2020). A further consideration is the synthesis of water-insoluble glucans by S. mutans, which was inhibited in the presence of eugenol (Xu et al., 2013). Water-insoluble glucans promote the adhesive interactions of bacteria with the tooth surface and contribute to the formation of dental biofilms (Xu et al., 2013). Additionally, the genes that affect bacterial adhesion and biofilm formation include gtfB, gtfC, gbpB, ftf, vicR, brpA, smu630, relA, comDE, and spaP were downregulated by eugenol (Adil et al., 2014). With all these interpretations, it can be concluded that eugenol can affect the S. mutans biofilm, making it a promising natural agent for combating dental biofilms.
Eugenol can be utilized alongside antibacterial agents to achieve synergistic or additive effects. The synergistic effects of eugenol and carnosic acid on microbial biofilms, specifically S. mutans, were noted. The interactions of eugenol and carnosic acid with the cytoplasmic membrane may elucidate this phenomenon. The combination of eugenol and macrocarpals exhibited synergistic benefits against bacterial biofilms. The rupture of the cytoplasmic membrane by eugenol may elucidate the translocation of macrocarpals into the cell, where they impede enzymatic activities and elevate ROS and intracellular DNA fragmentation (Tsukatani et al., 2020). Furthermore, eugenol showed a synergism effect with antimicrobial agents (fluconazole and azithromycin) against C. albicans and S. mutans. Eugenol can disrupt cell membrane integrity, facilitating the ingress of drugs into the microbial cell. This phenomenon facilitates the accessibility of the antimicrobial agents to the target site, enhancing their efficacy (Jafri et al., 2020). Therefore, the combined use of antimicrobial agents and eugenol may provide numerous benefits, such as increased efficacy, diminished medication dosage, and lowered toxicity, hence helping in the inhibition or eradication of biofilms and the reduction of antimicrobial resistance (Chouhan et al., 2017).
Nonetheless, light sensitivity and inadequate water solubility are significant drawbacks of eugenol, as they restrict its practical applications. Sajomsang et al., by incorporating eugenol into the β-cyclodextrin-grafted chitosan derivatives (QCD-g-CS) complex, developed a novel mucoadhesive drug carrier that could improve eugenol’s solubility, stability, and delivery properties while maintaining or enhancing its beneficial biological activities. The antibacterial efficacy of QCD-g-CS-eugenol against Streptococcus oralis, C. albicans, and S. mutans was assessed. Combining eugenol and QCD-g-CSs as an inclusion complex has a synergistic effect on antibacterial activity. The enhanced antibacterial efficacy of the synthesized drug carrier is ascribed to the augmented solubility of eugenol in the aqueous phase, facilitated by the presence of QCD-g-CS, thereby fostering improved interactions between eugenol and microorganisms (Sajomsang et al., 2012).
Finally, it is worth mentioning that studies showed the antibacterial effect of eugenol against S. mutans as a restorative material (Boeckh et al., 2002; Monajemzadeh et al., 2017; Makarla et al., 2023). Coronal leakage following root canal therapy is regarded as a significant cause of endodontic treatment failure due to contamination of the root canal system. Oral bacteria can infiltrate the root canal system during endodontic treatment without a coronal seal above the root filling. A base coronal to the root filling has been demonstrated to diminish microleakage and enhance the long-term prognosis of teeth treated with root canal therapy (Dragland et al., 2019). Nonetheless, microbial leakage has been demonstrated to occur even after installing temporary filling materials above the root filling (Balto, 2002). This issue indicates that the materials employed for a coronal seal must effectively occlude the opening of the root filling and exhibit antimicrobial characteristics. In this regard, a zinc oxide-eugenol (ZOE)-based restorative substance is among dentistry’s most frequently utilized temporary restorative materials. In endodontics, a ZOE restorative material serves as a base beneath the permanent restoration to inhibit bacterial ingress into the root canal between appointments and after the permanent restoration placement (Gimbel et al., 2002). The leaching of eugenol somewhat facilitates the antibacterial and bacteriostatic properties of ZOE-based materials (Dragland et al., 2019).
Collectively, eugenol, as a natural compound, exhibited good antibacterial and anti-biofilm activity against S. mutans, making it a suitable candidate for use in dentistry. Further research should also be considered to enhance its pharmacodynamic properties using novel methods, including nanoparticles and other drug delivery platforms.
Farnesol
Farnesol, a sesquiterpene alcohol (3,7,11-trimethyl-2,6,10-dodecatrien-1-ol), is frequently present in certain essential oils, propolis, and citrus fruits and serves as a unique naturally occurring anticaries agent (Jeon et al., 2011). Koo et al. reported the MIC and MBC of this compound against S. mutans as 125 µM and 500 μM, respectively (Koo et al., 2002). A study’s results indicated that farnesol enhanced the initial rate of proton influx in S. mutans cells (Jeon et al., 2011). Protons from the external environment penetrate inward via the cell membrane following the acidification of the suspension but can subsequently be ejected by the membrane-associated F-ATPase enzyme (Bender et al., 1986). The proton-translocating F-ATPase safeguards S. mutans from ambient acid stress by maintaining pH homeostasis, which is essential for the optimal functioning of glycolysis in S. mutans (Sturr and Marquis, 1992). Consequently, the enhancement of proton permeability induced by farnesol is probably attributable to direct impairment of the membrane barrier function. The alteration of proton permeability in the S. mutans cell membrane caused by farnesol would impact the pH gradient (ΔpH) across the membrane, suppressing total intracellular metabolism, including acidogenesis (Jeon et al., 2011). Such effects may also induce energy deprivation in S. mutans, inhibiting intracellular polysaccharide (IPS) synthesis and accumulation (Jeon et al., 2009). It can be concluded that farnesol can influence the acidogenicity and aciduricity of S. mutans in both the planktonic phase and biofilm by enhancing the proton permeability of the membrane, possibly due to loss of the cell membrane’s functional integrity (Jeon et al., 2011).
Significant antibiofilm activity against S. mutans was shown by farnesol (Koo et al., 2002; Lobo et al., 2021). As previously noted, farnesol can disrupt membrane function, which reduces cell viability and could impact biofilm formation. A significant reduction in total biomass and the number of viable cells was observed in single and mixed species biofilms of S. mutans and C. albicans when exposed to farnesol (Fernandes et al., 2016). De Melo et al. reported the most significant reduction of S. mutans cells after 8 h of exposure to farnesol (de Melo et al., 2015). Notably, biofilms treated with farnesol exhibited a reduced quantity of viable cells and less dense structures. Moreover, the matrix composition study indicated a decrease in protein concentration following exposure to farnesol. These findings underscore the influence of farnesol on the diminution of biofilms, particularly its impact on the disintegration of the extracellular matrix. The extracellular matrix is significant as it constitutes a barrier to medication permeation (Fernandes et al., 2018).
Prior research has indicated that the reduction of long-chained cells constitutes the initial morphological alteration in the process of cell apoptosis, and this modification is also a mechanism of acid tolerance in S. mutans (Fozo and Quivey, 2004). To this end, the microscopic analysis revealed a reduced presence of long-chained cells in the farnesol-treated biofilms compared to the untreated control biofilms. The findings indicated that decreasing long-chained cells in the farnesol-treated biofilms may constitute a damaging mechanism of farnesol against S. mutans biofilm (Cao et al., 2017). Additionally, the transcription levels of brpA, luxS, recA, ffh, smx, and nth were decreased in the farnesol-treated biofilms (Cao et al., 2017). Streptococcus mutans employs a low-pH survival strategy by activating a DNA repair mechanism to safeguard or rectify DNA damage resulting from the detrimental effects of intracellular acidification. Certain proteins, including RecA, Nth, Smx, and Ffh, participate in this process (Cao et al., 2017). Notably, RecA is implicated in stress tolerance and DNA repair. The nth gene encodes a putative EndoIII-related endonuclease implicated in DNA replication, recombination, and repair (Wen and Burne, 2004). A class II-like AP endonuclease that controls an organism’s exonucleolytic activity and acid-adaptive response is encoded by the smx gene (Faustoferri et al., 2005). The ffh gene encodes a homolog of the 54 kDa subunit of a signal recognition particle that contributes to acid stress tolerance (Gutierrez et al., 1999). Finally, the demineralization process induced by S. mutans biofilms diminishes enamel surface micro-hardness, resulting in caries lesions (Jabbarifar et al., 2011). The results indicated that farnesol therapy diminished the reduction of surface enamel micro-hardness caused by S. mutans, implying that farnesol may inhibit the development of caries associated with S. mutans and could serve as a possible agent against this bacterium (Cao et al., 2017). Therefore, farnesol can exert its anti-biofilm effect by disrupting stress and acid tolerance mechanisms, changing the expression of genes related to biofilm formation, and reducing the viable cells.
Farnesol has also demonstrated synergistic effects when combined with other anticaries agents (Koo et al., 2003; Chen et al., 2006; Lobo et al., 2022; Haj-Yahya et al., 2024). For example, Rocha et al. assessed the impact of farnesol, myricetin, and fluoride on dual-species biofilm, including S. mutans and C. albicans. The findings indicated a reduction in water-soluble EPS in the extracellular matrix when combination therapy was employed. Consequently, combination therapy adversely impacted biofilm growth, rendering biofilm potentially less hazardous. The reduction of EPS is crucial, as the capacity of bacteria to manufacture glucan may be more critical for virulence than their population size, given that a compromised extracellular matrix may fail to offer sufficient three-dimensional structure and stability for microorganisms within the biofilm (Rocha et al., 2018). Another study also investigated the effects of farnesol and apigenin in conjunction with fluoride on S. mutans biofilms. The biological effects of each drug were significantly amplified when combined with fluoride. Biofilms treated with farnesol and/or apigenin in conjunction with fluoride exhibited reduced biomass and diminished levels of iodophilic polysaccharides and insoluble glucans compared to those treated with the test agents individually. Findings from this research indicated that apigenin and farnesol may augment the cariostatic efficacy of fluoride (Koo et al., 2005).
In line with these results, farnesol, myricetin and fluoride combinations were examined against S. mutans biofilm (Falsetta et al., 2012). The mentioned combination therapy effectively diminished the formation of cariogenic biofilms via multiple potentially complementary and/or overlapping mechanisms that primarily focus on inhibiting S. mutans EPS-rich matrix production while undermining the organism’s overall fitness by suppressing stress defence and/or modifying bacterial membrane physiology. The mentioned combination therapy interfered with particular genes typically linked to EPS synthesis and/or stress resilience (e.g., gtfB, sloA, sodA, and copY). Notably, GtfB is essential for the formation and preservation of plaque biofilms. Superoxide dismutase (SodA) is a recognized virulence factor that diminishes superoxide and is crucial in vivo (Falsetta et al., 2012). SloA is a manganese/iron transport system component, whereas CopY is also anticipated to participate in copper transport (Mitrakul et al., 2004). The diminished expression of sloA and copY may further inhibit the transcription of the gtf genes, as copper and manganese function as effector molecules that regulate their expression (Chen et al., 2006; Arirachakaran et al., 2007). collectively, the mentioned combination therapies could successfully connect the existing chemical modalities (e.g., fluoride and chlorhexidine) employed to prevent or treat dental caries disease (Falsetta et al., 2012).
However, farnesol cannot attain optimal performance for biofilm therapy owing to its hydrophobic nature and inadequate biofilm retention unless utilized in conjunction with other methods (Rocha et al., 2018). Consequently, to attain optimal efficacy within the intricate biofilm community, drug delivery systems, particularly nanoparticle carriers, have garnered heightened interest in the treatment of oral biofilms in recent years (Horev et al., 2015; Mogen et al., 2015; Supuran, 2015; Sims et al., 2018; Barot et al., 2020; Roncari Rocha et al., 2022). A recently published study evaluated the anti-biofilm effectiveness of farnesol using drug delivery through polymeric nanoparticle carriers (NPCs) against cross-kingdom biofilms. The farnesol-encapsulated nanoparticles (NPC + Far) achieved a 2-log CFU/mL decrease of S. mutans and C. albicans. High-resolution confocal photos indicated a substantial reduction in EPS, smaller microcolonies of S. mutans, and the absence of hyphal forms of C. albicans following treatment with NPC + Far on human tooth enamel (HT) slabs, thereby modifying the biofilm’s three-dimensional structure (Ito et al., 2022). In another study, an NPC capable of co-loading farnesol in the hydrophobic core and myricetin inside the cationic corona was evaluated in vitro, employing both established and developing S. mutans biofilms. Co-loaded NPC treatments significantly diminished biofilm biomass and survival compared to single-drug controls in growing biofilms, indicating that dual-drug delivery demonstrates synergistic anti-biofilm properties. Co-loaded NPCs synergistically suppressed planktonic bacterial proliferation relative to controls and diminished S. mutans acidogenicity by lowering atpD expression (Sims et al., 2020). In another study, researchers developed a micellar drug delivery system capable of successfully adhering to dental surfaces. To attain tooth-binding capability, the terminal ends of biocompatible Pluronic copolymers were altered with a biomineral-binding group (i.e., alendronate). The micelles created with this polymer demonstrated the ability to rapidly (<1 min) adhere to hydroxyapatite (HA; a model tooth surface) and progressively release the encapsulated farnesol. In vitro biofilm inhibition investigations revealed that the synthesized micelles significantly inhibited S. mutans biofilm formation (Chen et al., 2009). Hence, drug delivery systems can be designed to enhance farnesol by prolonging the contact time with S. mutans to improve antibacterial effects. Moreover, these systems can enhance farnesol penetration into biofilms and increase access to bacteria embedded within the EPS matrix.
Epigallocatechin-3-gallate
Epigallocatechin gallate (EGCG) is the predominant catechin in green tea, constituting about 50% of its overall composition. EGCG is recognized for possessing the most potent antibacterial properties among all catechins, according to the galloyl groups within its structure (Sasaki et al., 2004; Wang and Ho, 2009). Recently published studies reported that EGCG has an inhibitory effect against S. mutans (Bai et al., 2016; Hattarki et al., 2021; Garcia-Contreras et al., 2023; Higuchi et al., 2024). EGCG infiltrating lipid bilayers induces lateral membrane expansion, leading to membrane breakdown. Catechins can interact with dissolved oxygen to generate hydrogen peroxide and hydroxyl radicals, thereby causing intracellular lipid oxidation and damage to DNA and proteins (Wu and Brown, 2021). Higuchi et al. indicated that EGCG can suppress the metabolic activity of S. mutans. The authors hypothesized that the metabolic inhibition of this bacteria by EGCG is attributed to the binding of EGCG to the sugar uptake enzyme system, namely, the phosphoenolpyruvate phosphotransferase system (PEP-PTS), which leads to the inhibition of sugar absorption (Higuchi et al., 2024). In line with these findings, Han et al. also reported that EGCG can inhibit the growth and acid generation of S. mutans by suppressing the activity of the PEP-PTS (Han et al., 2021). Notably, the PEP-PTS is a cluster of enzymes responsible for translocating sugars into bacteria, comprising enzymes located on the cell membrane and within the cytoplasm. The results indicated that PEP-PTS activity was inhibited by EGCG, implying that EGCG obstructs glucose absorption in bacterial cells, hence diminishing bacterial metabolism and acidogenesis (Han et al., 2021). In a separate in silico molecular docking investigation, these authors indicated that catechins exert their effects through contact with the cell membrane-bound glucose transporter EIIC, a component of the PEP-PTS. In contrast to nongalloylated catechins, the galloyl structures of EGCG enabled strong binding to the functional region of the EIIC (Han et al., 2023). Furthermore, another research indicated that the inhibition of acid generation by EGCG is due to its suppressive effects on lactate dehydrogenase at both the transcriptional and enzymatic levels (Hirasawa et al., 2006; Xu et al., 2011).
In addition to the mentioned inhibitory mechanisms of EGCG against S. mutans, the results of another investigation indicated that EGCG impeded the adhesion of oral bacteria by diminishing their surface hydrophobicity; however, this effect was not observed on hydroxyapatite surfaces (Wang and Lam, 2020). Besides, Cui et al. demonstrated that EGCG caused aggregation in S. mutans (Cui et al., 2012). Therefore, EGCG can eliminate S. mutans by direct interaction with cellular components, and its inhibition of bacterial metabolism diminishes energy generation, possibly suppressing bacterial growth. Besides impeding metabolism and growth, EGCG obstructs bacterial adhesion and facilitates bacterial aggregation. Consequently, EGCG appears to be able to induce aggregation of salivary bacteria, facilitate their evacuation, and impede the formation of oral biofilm. Ultimately, EGCG can suppress the acid production of S. mutans, a main bacterial component in caries formation.
Previous studies also reported the inhibitory effects of EGCG against the S. mutans biofilm community (Liao et al., 2021; Taylor et al., 2021). To this end, a study indicated that EGCG markedly decreased the buildup of soluble and insoluble polysaccharides, leading to a biofilm characterized by irregularly distributed exopolysaccharide-microcolony complexes on enamel. Consequently, the authors suggested that EGCG diminished the pathogenicity of S. mutans matrix-rich biofilm by inhibiting the production of biofilm matrix constituents and modifying the structure, organization, and dispersion of the biofilm matrix (Aragão et al., 2024a). Rayman et al. also indicated that EGCG diminished biofilm thickness, lowered the viable bacterial count, augmented the number of deceased bacteria, and blocked EPS formation. EGCG markedly suppressed the expression of gtfC, gtfB, and ftf by 77%–90% relative to the control (Schneider-Rayman et al., 2021). The findings of other studies also showed that EGCG decreased levels of extracellular polysaccharides in S. mutans biofilms and reduced the expression of gtf genes (Xu et al., 2012; Wu et al., 2018).
EGCG appears to inhibit the biofilm formation of S. mutans through a sucrose-dependent anti-adhesion mechanism, as it has been demonstrated to obstruct S. mutans glucosyltransferases, which are critical enzymes for bacterial attachment, biofilm development, and pathogenicity in the presence of sucrose. In this context, Islam et al. indicated that EGCG interacted with the amino acids GLU 515 and TRP 517, binding to glucansucrase and inhibiting its enzymatic activity. Enzymatic suppression of glucansucrase reduced the biofilm-forming capability of S. mutans on tooth surfaces (Hairul Islam et al., 2020). Collectively, S. mutans produces extracellular adherent glucans from dietary sucrose through GTFs, hence facilitating the aggregation of oral bacteria on dental surfaces. The earliest phase of S. mutans biofilm, marked by sucrose-dependent bacterial adhesion to dental surfaces, constitutes a crucial preliminary step in the eventual development of the mature biofilm (Burne et al., 2009; Xu et al., 2011). To this end, EGCG has a good potential for inhibiting S. mutans biofilm because it inhibits initial attachment and the specific genes associated with bacterial biofilm formation.
Despite their advantages, catechins encounter significant obstacles in their development as medicinal agents, such as inadequate absorption, low bioavailability, and quick destruction. The advent of nanobiotechnology facilitates targeted and steady distribution, hence improving EGCG bioavailability and optimizing therapeutic efficacy. Recently published studies used different EGCG-based drug platforms, such as chitosan nanoparticles loaded with EGCG, mesoporous silica-based EGCG/nanohydroxyapatite delivery, developing silver nanoparticles (AgNPs) using EGCG, and EGCG containing glass ionomer cements (Hu J. et al., 2013; Yu et al., 2017; Yu et al., 2021; Aragão et al., 2024b). Noteworthy, using EGCG can enhance the inhibitory effect of different platforms against S. mutans and the biofilm community of this bacterium. The drug platform can also deliver EGCG over an extended period, ensuring prolonged stability to safeguard the underlying dentin from harmful conditions in oral settings. Consequently, applying EGCG-based pharmacological platforms indicates a feasible approach for prolonging the longevity of restorations and advancing dental material science.
Thymol
Thymus vulgaris (thyme) has been utilized in traditional medicine to treat diverse diseases due to its extensive pharmacological qualities. The primary component of thyme essential oil is thymol, a phenolic monoterpene molecule (Priya et al., 2021). Thymol is a naturally occurring phenolic monoterpenoid compound that is utilized extensively in food and pharmaceutical preservative applications due to its beneficial antifungal and antibacterial qualities (Ferreira et al., 2018; Karimi et al., 2020; Priya et al., 2021). Recently published studies reported that thymol has an inhibitory effect against S. mutans (Botelho et al., 2007; Nunes et al., 2020; Park et al., 2023). For example, in one study, the MIC and MBC values of the phytochemical thymol against S. mutans were 312.5 μg/mL (Ferreira et al., 2018). In another investigation, thymol demonstrated bactericidal and bacteriostatic effects against the planktonic cells of S. mutans, with a MIC and MBC of 100 mg/mL and 400 mg/mL, respectively (Nunes et al., 2020). A recent study demonstrated that thymol at a 300 μg/mL dosage inhibited the growth of dual species of S. mutans and C. albicans (Priya et al., 2021). Cell membrane breakdown, intracellular substance leaking, and ensuing modifications in transmembrane potential are the foundations of the thymol mode of action. Additionally, this compound can enter cells and interact with intracellular locations, which is crucial to its antibacterial action (Vlachojannis et al., 2015). Furthermore, thymol can be incorporated into bacterial cell membranes, readily traverse lipid barriers, and compromise membrane integrity, thereby hindering cell proliferation and inducing cell death. Due to its lipophilic nature and affinity for bacterial cell membranes, thymol can inhibit bacterial activity (Yazdanian et al., 2022; Park et al., 2023). The genes of S. mutans associated with biofilm formation, competence, and glucan synthesis were downregulated by thymol (Priya et al., 2021). In line with these results, Thymus essential oil showed a notable inhibitory effect on the expression of the S. mutans virulence genes, including brpA, vicR, gbpB, gtfB, gtfC, gtfD, relA, and spaP (Table 2). Molecular docking between thymol and virulence proteins revealed that thymol demonstrated a significant binding affinity for the functional domains of virulence genes (Park et al., 2023).
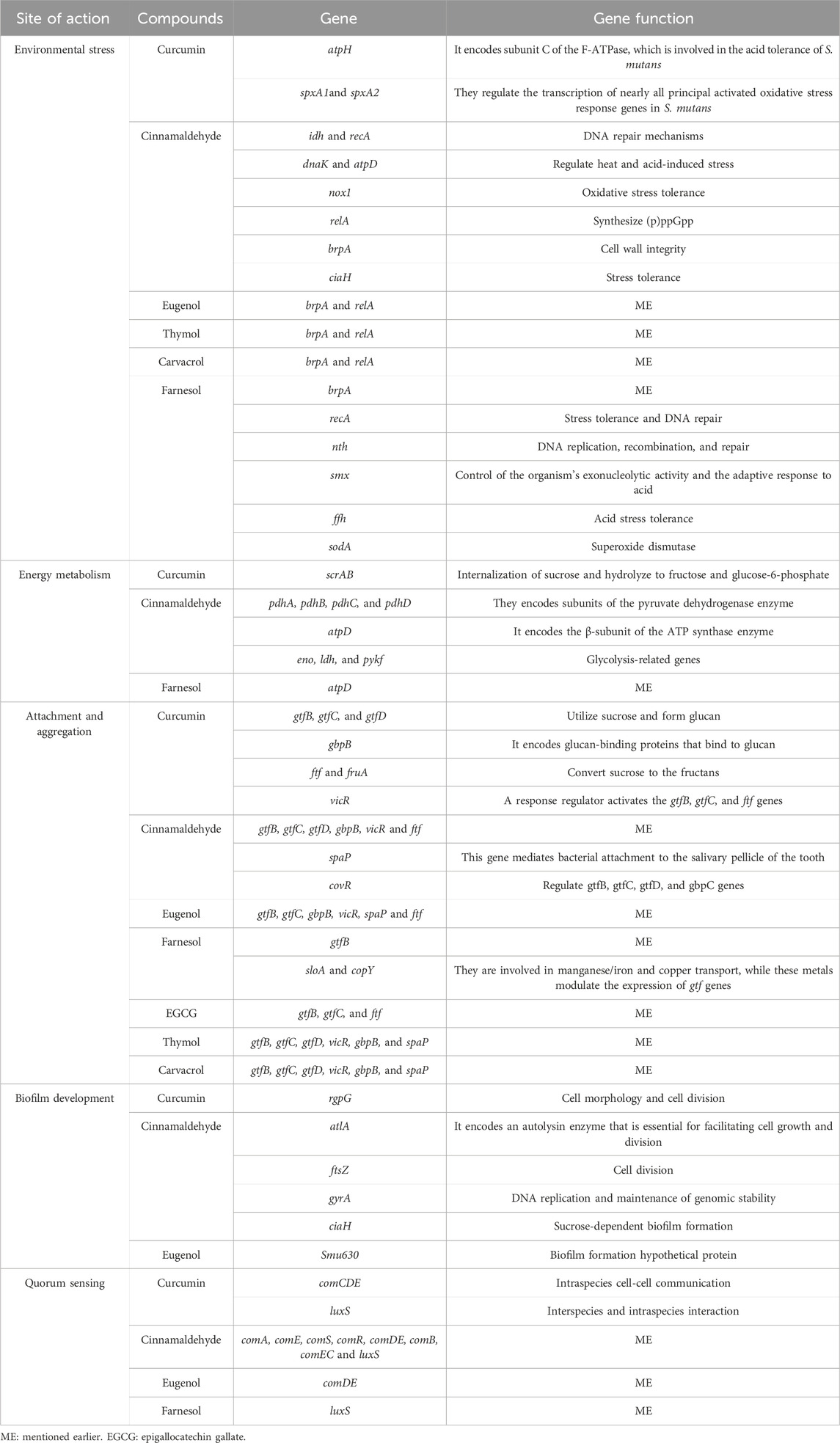
Table 2. The molecular interactions of natural compounds with different pathogenic mechanisms of Streptococcus mutans.
Scientists also considered thymol-based drug delivery platforms for improving thymol activity against S. mutans and dental caries. To this end, thymol-chitosan hydrogels demonstrated biocompatibility with [3T3] fibroblasts, exhibited antibacterial efficacy against S. mutans for 72 h, and showed antioxidant activity for 24 h. These are advantageous characteristics for a mucosal delivery method for an antimicrobial-antioxidant dual treatment targeting periodontal disease. Consequently, the antioxidant qualities of thymol may reduce periodontal inflammation; conversely, thymol could serve as an adjunct to mechanical plaque management due to its antibacterial efficacy. It should be noted that no adhesion or aggregation of S. mutans was detected in thymol-loaded chitosan hydrogels throughout a 24-h (Alvarez Echazú et al., 2017). Another study also nanoencapsulated the combination of clove oil and thymol (CLTY) utilizing chitosan and poly-γ-glutamic acid. Free CLTY demonstrated both additive and synergistic antibacterial effects against S. mutans and Streptococcus sobrinus, respectively. In contrast, in a time-kill kinetic experiment, CLTY nanoparticles (NPs) displayed synergistic action against both strains. CLTY NPs reduced the proliferation of salivary S. mutans during the evaluation, in contrast to free CLTY in the mouth rinse assay. The results demonstrated that nanoencapsulation can enhance the synergistic antibacterial efficacy of CLTY and prolong its antimicrobial activity in oral cavities (Lee et al., 2020). In the end, in another study, chitosan-grafted thymol (CST) coated on gold nanoparticles (AuNPs) was effectively employed to regulate cariogenic bacteria in the oral cavity. The incorporation of AuNPs with CST increased bactericidal efficacy against S. mutans. This CST coating on the AuNPs surface may represent a significant new tool in combating cariogenic bacterial infections (Chittratan et al., 2022). Collectively, the use of such compounds leads to the enhancement of thymols’ pharmacological activity and the expansion of its applications in medicine. Additionally, as mentioned, thymol demonstrated considerable inhibition of bacterial proliferation, acidogenesis, adhesion, and biofilm development of S. mutans. Therefore, because thymol is a natural anti-cariogenic agent with strong antibacterial effects against S. mutans, it can be used in oral and dental hygiene products and edible products such as chewing gum and candies.
Carvacrol
Carvacrol, a phenolic monoterpenoid, is recognized as a principal component of the essential oils derived from several fragrant plants, including thyme (Thymus vulgaris), pepperwort (Lepidium flavum), and oregano (Origanum vulgare). This natural compound has been utilized as a food preservative, additive, flavouring, and scent in cosmetic items (Shariati et al., 2022). Carvacrol possesses multiple biological properties, such as antioxidant and antimicrobial activity. Due to its diverse qualities, including a free hydroxyl group, phenolic moiety, and hydrophobic characteristics, carvacrol demonstrated superior antibacterial efficacy compared to other volatile compounds. Several studies evaluated the antibacterial and antibiofilm effect of carvacrol, the results of which all confirm the antibacterial ability of this substance against S. mutans (Botelho et al., 2007; Ciandrini et al., 2014; Baygar et al., 2018; Pinna et al., 2019; Yazdanian et al., 2022). Babiano et al. assessed the antibacterial efficacy of carvacrol against pathogenic microorganisms responsible for oral infections, including S. mutans and Streptococcus sanguinis. The findings of their research indicated that sub-inhibitory concentration of carvacrol impeded bacterial growth. Furthermore, the death kinetics demonstrated a rapid and effective microbicide activity at doses that impact planktonic bacteria similarly to those shielded within their polymeric matrix. According to the results of morphological changes obtained from microscopic evaluation, the authors of this study explained the anti-biofilm effect of carvacrol as follows: the mechanism of action of carvacrol appears to be multifaceted, mostly contingent upon the molecular structural characteristics, which include a free hydroxyl group, a delocalized electron system, and hydrophobic properties. Carvacrol may target the cytoplasmic membrane, so affecting its development and functionality. Carvacrol’s hydrophobic characteristics enable it to interact with the lipid bilayer of the cytoplasmic membrane, positioning itself among the fatty acid chains. This interaction destabilizes the membrane structure, enhances fluidity, and increases permeability to potassium ions and protons, ultimately leading to cell death (Fernández-Babiano et al., 2022).
In line with this study, another experiment indicated that carvacrol showed significant bactericidal and antibiofilm properties against S. mutans and can serve as an eco-friendly alternative for managing dental caries. Anti-biofilm findings indicated that carvacrol diminished biofilm formation capability. The authors proposed that this compound contributes to the permeabilization and depolarization of the cytoplasmic membrane, reducing the pH gradient across the membrane. The reduction of the pH gradient disrupts the proton motive force, resulting in decreased intracellular ATP levels and, ultimately, cell death (Khan et al., 2017). In another study, thymus essential oils downregulated several genes encoding virulence factors associated with biofilm formation and maintenance, including brpA, gbpB, gtfB, gtfC, gtfD, vicR, spaP, and relA (Park et al., 2023). Collectively, results indicate that carvacrol exhibits antibacterial action against S. mutans and may be beneficial for preserving oral hygiene by inhibiting bacterial proliferation. Further investigations are recommended to enhance the understanding of this compound’s interaction with oral bacterial biofilms and its efficacy.
Finally, it is noteworthy that the interactions of other natural compounds with S. mutans are presented in Table 3.
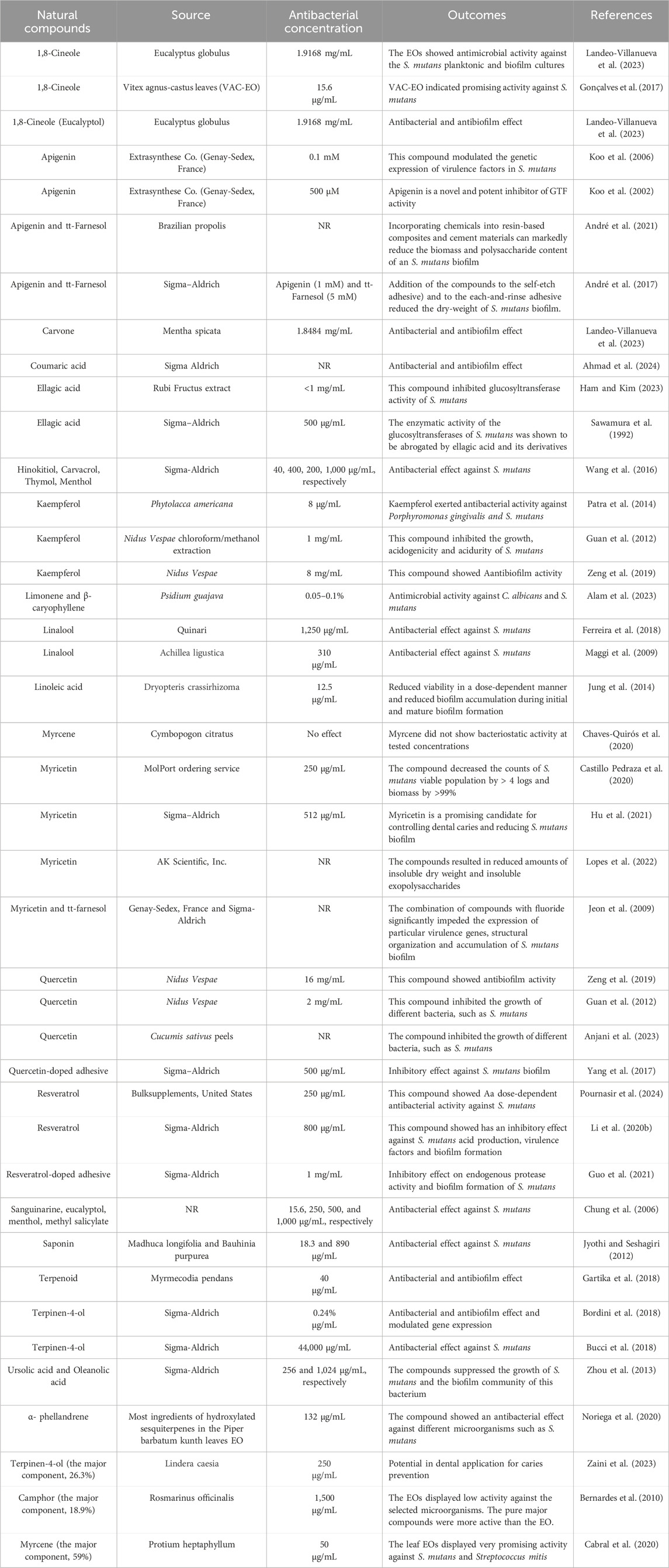
Table 3. The inhibitory effect of natural compounds against Streptococcus mutans and the biofilm community of this bacterium.
Conclusion
Recently published studies have focused on the role of microorganisms in the pathogenesis of caries, but oral bacterial resistance to newly developed antibacterial drugs is a newer concern. Hence, it is urgent to develop new drugs that inhibit bacterial QS and biofilm formation for antibacterial to prevent dental caries. Due to the increasing microbial resistance to existing antibiotics and the decline in the development of new drugs, global interest in natural antiseptic products derived from medicinal plants has grown. Previous studies have shown that natural products are promising for developing new anti-caries materials. In this review, natural compounds showed inhibitory effects on S. mutans, one of the primary causative agents of dental caries. These compounds reduced the planktonic population of this bacterium and had a remarkable effect on its biofilm population. However, these compounds have poor pharmacodynamic properties. Hence, novel drug delivery platforms can enhance their efficacy and productivity. In addition, since S. mutans alone does not cause caries and it develops caries with the help of other microorganisms, future studies should be more focused on investigating multi-species biofilms and optimizing the delivery methodologies of these compounds. Given the promising effects of these compounds, they could be used as a supplement alongside conventional anti-caries drugs such as fluoride or chlorhexidine. Combining these compounds with other antimicrobial agents may lead to synergistic effects and, in addition to enhancing overall efficacy, could reduce the required dosage of conventional drugs and possible side effects. Finally, although laboratory studies are valuable, their results must be validated in vivo and clinical settings to ensure their practical application in prevention and treatment.
Author contributions
MK: Investigation, Software, Writing – review and editing. MV: Writing – review and editing. YH: Writing – original draft. ZC: Investigation, Writing – original draft. AS: Conceptualization, Investigation, Software, Supervision, Validation, Visualization, Writing – original draft, Writing – review and editing.
Funding
The author(s) declare that no financial support was received for the research and/or publication of this article.
Acknowledgments
We greatly appreciate the input from the BioRender team (BioRender.com) for their collaboration with us in figure design.
Conflict of interest
The authors declare that the research was conducted without any commercial or financial relationships that could be construed as a potential conflict of interest.
The author(s) declared that they were an editorial board member of Frontiers, at the time of submission. This had no impact on the peer review process and the final decision.
Generative AI statement
The author(s) declare that no Generative AI was used in the creation of this manuscript.
Publisher’s note
All claims expressed in this article are solely those of the authors and do not necessarily represent those of their affiliated organizations, or those of the publisher, the editors and the reviewers. Any product that may be evaluated in this article, or claim that may be made by its manufacturer, is not guaranteed or endorsed by the publisher.
Supplementary material
The Supplementary Material for this article can be found online at: https://www.frontiersin.org/articles/10.3389/fphar.2025.1548117/full#supplementary-material
References
Abranches, J., Zeng, L., Kajfasz, J. K., Palmer, S. R., Chakraborty, B., Wen, Z. T., et al. (2018). Biology of oral streptococci. Microbiol. Spectr. 6 (5). doi:10.1128/microbiolspec.GPP3-0042-2018
Adil, M., Singh, K., Verma, P. K., and Khan, A. U. (2014). Eugenol-induced suppression of biofilm-forming genes in Streptococcus mutans: an approach to inhibit biofilms. J. Glob. Antimicrob. Resist 2 (4), 286–292. doi:10.1016/j.jgar.2014.05.006
Ahmad, S. S., Siddiqui, M. F., Maqbool, F., Ullah, I., Adnan, F., Albutti, A., et al. (2024). Combating cariogenic Streptococcus mutans biofilm formation and disruption with coumaric acid on dentin surface. Molecules 29 (2), 397. doi:10.3390/molecules29020397
Ahn, S. J., and Burne, R. A. (2006). The atlA operon of Streptococcus mutans: role in autolysin maturation and cell surface biogenesis. J. Bacteriol. 188 (19), 6877–6888. doi:10.1128/jb.00536-06
Ahrari, F., Nazifi, M., Mazhari, F., Ghazvini, K., Menbari, S., Fekrazad, R., et al. (2024). Photoinactivation effects of curcumin, nano-curcumin, and erythrosine on planktonic and biofilm cultures of Streptococcus mutans. J. Lasers Med. Sci. 15, e7. doi:10.34172/jlms.2024.07
Alam, A., Jawaid, T., Alsanad, S. M., Kamal, M., and Balaha, M. F. (2023). Composition, antibacterial efficacy, and anticancer activity of essential oil extracted from psidium guajava (L.) leaves. Plants (Basel) 12 (2), 246. doi:10.3390/plants12020246
Alvarez Echazú, M. I., Olivetti, C. E., Anesini, C., Perez, C. J., Alvarez, G. S., and Desimone, M. F. (2017). Development and evaluation of thymol-chitosan hydrogels with antimicrobial-antioxidant activity for oral local delivery. Mater Sci. Eng. C Mater Biol. Appl. 81, 588–596. doi:10.1016/j.msec.2017.08.059
André, C. B., Rosalen, P. L., Galvão, L. C. C., Fronza, B. M., Ambrosano, G. M. B., Ferracane, J. L., et al. (2017). Modulation of Streptococcus mutans virulence by dental adhesives containing anti-caries agents. Dent. Mater 33 (10), 1084–1092. doi:10.1016/j.dental.2017.07.006
André, C. B., Rosalen, P. L., Giannini, M., Bueno-Silva, B., Pfeifer, C. S., and Ferracane, J. L. (2021). Incorporation of Apigenin and tt-Farnesol into dental composites to modulate the Streptococcus mutans virulence. Dent. Mater 37 (4), e201–e212. doi:10.1016/j.dental.2020.12.005
Anjani, , Srivastava, N., and Mathur, J. (2023). Isolation, purification and characterization of quercetin from Cucumis sativus peels; its antimicrobial, antioxidant and cytotoxicity evaluations. 3 Biotech. 13 (2), 46. doi:10.1007/s13205-023-03464-8
Aragão, M. G. B., He, X., Aires, C. P., and Corona, S. a.M. (2024a). Epigallocatechin gallate reduces the virulence of cariogenic Streptococcus mutans biofilm by affecting the synthesis of biofilm matrix components. Arch. Oral Biol. 164, 105990. doi:10.1016/j.archoralbio.2024.105990
Aragão, M. G. B., Tedesco, A. C., Borges, H. S., Aires, C. P., and Corona, S. a.M. (2024b). Chitosan nanoparticles loaded with epigallocatechin-3-gallate: synthesis, characterisation, and effects against Streptococcus mutans biofilmEpigallocatechin-loaded chitosan nanoparticles: effects against Streptococcus mutans biofilm. Nat. Prod. Res., 1–8. doi:10.1080/14786419.2024.2302321
Araújo, N. C., Fontana, C. R., Bagnato, V. S., and Gerbi, M. E. (2012a). Photodynamic effects of curcumin against cariogenic pathogens. Photomed. Laser Surg. 30 (7), 393–399. doi:10.1089/pho.2011.3195
Araújo, N. C., Fontana, C. R., Bagnato, V. S., and Gerbi, M. E. (2014). Photodynamic antimicrobial therapy of curcumin in biofilms and carious dentine. Lasers Med. Sci. 29 (2), 629–635. doi:10.1007/s10103-013-1369-3
Araújo, N. C., Fontana, C. R., Gerbi, M. E., and Bagnato, V. S. (2012b). Overall-mouth disinfection by photodynamic therapy using curcumin. Photomed. Laser Surg. 30 (2), 96–101. doi:10.1089/pho.2011.3053
Arirachakaran, P., Benjavongkulchai, E., Luengpailin, S., Ajdić, D., and Banas, J. A. (2007). Manganese affects Streptococcus mutans virulence gene expression. Caries Res. 41 (6), 503–511. doi:10.1159/000110883
Azizi, A., Shohrati, P., Goudarzi, M., Lawaf, S., and Rahimi, A. (2019). Comparison of the effect of photodynamic therapy with curcumin and methylene Blue on streptococcus mutans bacterial colonies. Photodiagnosis Photodyn. Ther. 27, 203–209. doi:10.1016/j.pdpdt.2019.06.002
Bai, L., Takagi, S., Ando, T., Yoneyama, H., Ito, K., Mizugai, H., et al. (2016). Antimicrobial activity of tea catechin against canine oral bacteria and the functional mechanisms. J. Vet. Med. Sci. 78 (9), 1439–1445. doi:10.1292/jvms.16-0198
Baker, J. L., Derr, A. M., Karuppaiah, K., Macgilvray, M. E., Kajfasz, J. K., Faustoferri, R. C., et al. (2014). Streptococcus mutans NADH oxidase lies at the intersection of overlapping regulons controlled by oxygen and NAD+ levels. J. Bacteriol. 196 (12), 2166–2177. doi:10.1128/jb.01542-14
Baker, J. L., Faustoferri, R. C., and Quivey, R. G. (2017). Acid-adaptive mechanisms of Streptococcus mutans-the more we know, the more we don't. Mol. Oral Microbiol. 32 (2), 107–117. doi:10.1111/omi.12162
Balasubramanian, A. R., Vasudevan, S., Shanmugam, K., Lévesque, C. M., Solomon, A. P., and Neelakantan, P. (2021). Combinatorial effects of trans-cinnamaldehyde with fluoride and chlorhexidine on Streptococcus mutans. J. Appl. Microbiol. 130 (2), 382–393. doi:10.1111/jam.14794
Balto, H. (2002). An assessment of microbial coronal leakage of temporary filling materials in endodontically treated teeth. J. Endod. 28 (11), 762–764. doi:10.1097/00004770-200211000-00004
Barot, T., Rawtani, D., Kulkarni, P., Hussain, C. M., and Akkireddy, S. (2020). Physicochemical and biological assessment of flowable resin composites incorporated with farnesol loaded halloysite nanotubes for dental applications. J. Mech. Behav. Biomed. Mater 104, 103675. doi:10.1016/j.jmbbm.2020.103675
Barrows, J. M., and Goley, E. D. (2021). FtsZ dynamics in bacterial division: what, how, and why? Curr. Opin. Cell Biol. 68, 163–172. doi:10.1016/j.ceb.2020.10.013
Baygar, T., Ugur, A., Sarac, N., Balci, U., and Ergun, G. (2018). Functional denture soft liner with antimicrobial and antibiofilm properties. J. Dent. Sci. 13 (3), 213–219. doi:10.1016/j.jds.2017.10.002
Bender, G. R., Sutton, S. V., and Marquis, R. E. (1986). Acid tolerance, proton permeabilities, and membrane ATPases of oral streptococci. Infect. Immun. 53 (2), 331–338. doi:10.1128/iai.53.2.331-338.1986
Bernardes, W. A., Lucarini, R., Tozatti, M. G., Flauzino, L. G., Souza, M. G., Turatti, I. C., et al. (2010). Antibacterial activity of the essential oil from Rosmarinus officinalis and its major components against oral pathogens. Z Naturforsch C J. Biosci. 65 (9-10), 588–593. doi:10.1515/znc-2010-9-1009
Bhaumik, D., Manikandan, D., and Foxman, B. (2021). Cariogenic and oral health taxa in the oral cavity among children and adults: a scoping review. Arch. Oral Biol. 129, 105204. doi:10.1016/j.archoralbio.2021.105204
Bischer, A. P., Kovacs, C. J., Faustoferri, R. C., and Quivey, R. G. (2020). Disruption of l-rhamnose biosynthesis results in severe growth defects in Streptococcus mutans. J. Bacteriol. 202 (6), e00728-19. doi:10.1128/JB.00728-19
Boeckh, C., Schumacher, E., Podbielski, A., and Haller, B. (2002). Antibacterial activity of restorative dental biomaterials in vitro. Caries Res. 36 (2), 101–107. doi:10.1159/000057867
Bordini, E. a.F., Tonon, C. C., Francisconi, R. S., Magalhães, F. a.C., Huacho, P. M. M., Bedran, T. L., et al. (2018). Antimicrobial effects of terpinen-4-ol against oral pathogens and its capacity for the modulation of gene expression. Biofouling 34 (7), 815–825. doi:10.1080/08927014.2018.1504926
Botelho, M. A., Nogueira, N. A., Bastos, G. M., Fonseca, S. G., Lemos, T. L., Matos, F. J., et al. (2007). Antimicrobial activity of the essential oil from Lippia sidoides, carvacrol and thymol against oral pathogens. Braz J. Med. Biol. Res. 40 (3), 349–356. doi:10.1590/s0100-879x2007000300010
Bottner, A., He, R. Y., Sarbu, A., Nainar, S. M. H., Dufour, D., Gong, S. G., et al. (2020). Streptococcus mutans isolated from children with severe-early childhood caries form higher levels of persisters. Arch. Oral Biol. 110, 104601. doi:10.1016/j.archoralbio.2019.104601
Bowen, W. H., and Koo, H. (2011). Biology of Streptococcus mutans-derived glucosyltransferases: role in extracellular matrix formation of cariogenic biofilms. Caries Res. 45 (1), 69–86. doi:10.1159/000324598
Bucci, A. R., Marcelino, L., Mendes, R. K., and Etchegaray, A. (2018). The antimicrobial and antiadhesion activities of micellar solutions of surfactin, CTAB and CPCl with terpinen-4-ol: applications to control oral pathogens. World J. Microbiol. Biotechnol. 34 (6), 86. doi:10.1007/s11274-018-2472-1
Burne, R. A., Ahn, S. J., Wen, Z. T., Zeng, L., Lemos, J. A., Abranches, J., et al. (2009). Opportunities for disrupting cariogenic biofilms. Adv. Dent. Res. 21 (1), 17–20. doi:10.1177/0895937409335593
Cabral, R. S. C., Alves, C. C. F., Batista, H. R. F., Sousa, W. C., Abrahão, I. S., Crotti, A. E. M., et al. (2020). Chemical composition of essential oils from different parts of Protium heptaphyllum (Aubl.) Marchand and their in vitro antibacterial activity. Nat. Prod. Res. 34 (16), 2378–2383. doi:10.1080/14786419.2018.1536659
Cao, L., Zhang, Z. Z., Xu, S. B., Ma, M., and Wei, X. (2017). Farnesol inhibits development of caries by augmenting oxygen sensitivity and suppressing virulence-associated gene expression inStreptococcus mutans. J. Biomed. Res. 31 (4), 333–343. doi:10.7555/jbr.31.20150151
Castillo Pedraza, M. C., De Oliveira Fratucelli, E. D., Ribeiro, S. M., Florez Salamanca, E. J., Da Silva Colin, J., and Klein, M. I. (2020). Modulation of lipoteichoic acids and exopolysaccharides prevents Streptococcus mutans biofilm accumulation. Molecules 25 (9), 2232. doi:10.3390/molecules25092232
Chaves-Quirós, C., Usuga-Usuga, J. S., Morales-Uchima, S. M., Tofiño-Rivera, A. P., Tobón-Arroyave, S. I., and Martínez-Pabón, M. C. (2020). Assessment of cytotoxic and antimicrobial activities of two components of Cymbopogon citratus essential oil. J. Clin. Exp. Dent. 12 (8), e749–e754. doi:10.4317/jced.56863
Chen, F., Liu, X. M., Rice, K. C., Li, X., Yu, F., Reinhardt, R. A., et al. (2009). Tooth-binding micelles for dental caries prevention. Antimicrob. Agents Chemother. 53 (11), 4898–4902. doi:10.1128/aac.00387-09
Chen, P. M., Chen, J. Y., and Chia, J. S. (2006). Differential regulation of Streptococcus mutans gtfBCD genes in response to copper ions. Arch. Microbiol. 185 (2), 127–135. doi:10.1007/s00203-005-0076-2
Chittratan, P., Chalitangkoon, J., Wongsariya, K., Mathaweesansurn, A., Detsri, E., and Monvisade, P. (2022). New chitosan-grafted thymol coated on gold nanoparticles for control of cariogenic bacteria in the oral cavity. ACS Omega 7 (30), 26582–26590. doi:10.1021/acsomega.2c02776
Chouhan, S., Sharma, K., and Guleria, S. (2017). Antimicrobial activity of some essential oils-present status and future perspectives. Med. (Basel) 4 (3), 58. doi:10.3390/medicines4030058
Chung, J. Y., Choo, J. H., Lee, M. H., and Hwang, J. K. (2006). Anticariogenic activity of macelignan isolated from Myristica fragrans (nutmeg) against Streptococcus mutans. Phytomedicine 13 (4), 261–266. doi:10.1016/j.phymed.2004.04.007
Ciandrini, E., Campana, R., Federici, S., Manti, A., Battistelli, M., Falcieri, E., et al. (2014). In vitro activity of Carvacrol against titanium-adherent oral biofilms and planktonic cultures. Clin. Oral Investig. 18 (8), 2001–2013. doi:10.1007/s00784-013-1179-9
Comeau, P., Panariello, B., Duarte, S., and Manso, A. (2022). Impact of curcumin loading on the physicochemical, mechanical and antimicrobial properties of a methacrylate-based experimental dental resin. Sci. Rep. 12 (1), 18691. doi:10.1038/s41598-022-21363-5
Cui, Y., Oh, Y. J., Lim, J., Youn, M., Lee, I., Pak, H. K., et al. (2012). AFM study of the differential inhibitory effects of the green tea polyphenol (-)-epigallocatechin-3-gallate (EGCG) against Gram-positive and Gram-negative bacteria. Food Microbiol. 29 (1), 80–87. doi:10.1016/j.fm.2011.08.019
De, A., Liao, S., Bitoun Jacob, P., Roth, R., Beatty Wandy, L., Wu, H., et al. (2017). Deficiency of RgpG causes major defects in cell division and biofilm formation, and deficiency of LytR-CpsA-psr family proteins leads to accumulation of cell wall antigens in culture medium by Streptococcus mutans. Appl. Environ. Microbiol. 83 (17), e00928-17–e00917. doi:10.1128/AEM.00928-17
De Almeida, M. a.L., Batista, A. U. D., De Araújo, M. R. C., De Almeida, V., Bonan, P. R. F., Nóbrega Alves, D., et al. (2020). Cinnamaldehyde is a biologically active compound for the disinfection of removable denture: blinded randomized crossover clinical study. BMC Oral Health 20 (1), 223. doi:10.1186/s12903-020-01212-5
De Melo, N. I., De Carvalho, C. E., Fracarolli, L., Cunha, W. R., Veneziani, R. C., Martins, C. H., et al. (2015). Antimicrobial activity of the essential oil of Tetradenia riparia (Hochst.) Codd. (Lamiaceae) against cariogenic bacteria. Braz J. Microbiol. 46 (2), 519–525. doi:10.1590/s1517-838246246220140649
Didehdar, M., Chegini, Z., and Shariati, A. (2022). Eugenol: a novel therapeutic agent for the inhibition of Candida species infection. Front. Pharmacol. 13, 872127. doi:10.3389/fphar.2022.872127
Dragland, I. S., Wellendorf, H., Kopperud, H., Stenhagen, I., and Valen, H. (2019). Investigation on the antimicrobial activity of chitosan-modified zinc oxide-eugenol cement. Biomater. Investig. Dent. 6 (1), 99–106. doi:10.1080/26415275.2019.1697621
Duque, C., Stipp, R. N., Wang, B., Smith, D. J., Höfling, J. F., Kuramitsu, H. K., et al. (2011). Downregulation of GbpB, a component of the VicRK regulon, affects biofilm formation and cell surface characteristics of Streptococcus mutans. Infect. Immun. 79 (2), 786–796. doi:10.1128/iai.00725-10
Falsetta, M. L., Klein, M. I., Lemos, J. A., Silva, B. B., Agidi, S., Scott-Anne, K. K., et al. (2012). Novel antibiofilm chemotherapy targets exopolysaccharide synthesis and stress tolerance in Streptococcus mutans to modulate virulence expression in vivo. Antimicrob. Agents Chemother. 56 (12), 6201–6211. doi:10.1128/aac.01381-12
Farkash, Y., Feldman, M., Ginsburg, I., Shalish, M., and Steinberg, D. (2020). The effect of Padma-hepaten herbal combination on the caries-inducing properties of Streptococcus mutans on orthodontic surfaces. J. Herb. Med. 20, 100321. doi:10.1016/j.hermed.2019.100321
Faustoferri, R. C., Hahn, K., Weiss, K., and Quivey, R. G. (2005). Smx nuclease is the major, low-pH-inducible apurinic/apyrimidinic endonuclease in Streptococcus mutans. J. Bacteriol. 187 (8), 2705–2714. doi:10.1128/jb.187.8.2705-2714.2005
Fernandes, R. A., Monteiro, D. R., Arias, L. S., Fernandes, G. L., Delbem, A. C., and Barbosa, D. B. (2016). Biofilm formation by Candida albicans and Streptococcus mutans in the presence of farnesol: a quantitative evaluation. Biofouling 32 (3), 329–338. doi:10.1080/08927014.2016.1144053
Fernandes, R. A., Monteiro, D. R., Arias, L. S., Fernandes, G. L., Delbem, A. C. B., and Barbosa, D. B. (2018). Virulence factors in Candida albicans and Streptococcus mutans biofilms mediated by farnesol. Indian J. Microbiol. 58 (2), 138–145. doi:10.1007/s12088-018-0714-4
Fernández-Babiano, I., Navarro-Pérez, M. L., Pérez-Giraldo, C., and Fernández-Calderón, M. C. (2022). Antibacterial and antibiofilm activity of carvacrol against oral pathogenic bacteria. Metabolites 12 (12), 1255. doi:10.3390/metabo12121255
Ferreira, G. L. S., Bezerra, L. M. D., Ribeiro, I. L. A., Morais Júnior, R. C. D., and Castro, R. D. (2018). Susceptibility of cariogenic microorganisms to phytoconstituents. Braz J. Biol. 78 (4), 691–696. doi:10.1590/1519-6984.174147
Fozo, E. M., and Quivey, R. G. (2004). Shifts in the membrane fatty acid profile of Streptococcus mutans enhance survival in acidic environments. Appl. Environ. Microbiol. 70 (2), 929–936. doi:10.1128/aem.70.2.929-936.2004
Gabe, V., Kacergius, T., Abu-Lafi, S., Kalesinskas, P., Masalha, M., Falah, M., et al. (2019). Inhibitory effects of ethyl gallate on Streptococcus mutans biofilm formation by optical profilometry and gene expression analysis. Molecules 24 (3), 529. doi:10.3390/molecules24030529
Galvão, L. C., Miller, J. H., Kajfasz, J. K., Scott-Anne, K., Freires, I. A., Franco, G. C., et al. (2015). Transcriptional and phenotypic characterization of novel spx-regulated genes in Streptococcus mutans. PLoS One 10 (4), e0124969. doi:10.1371/journal.pone.0124969
Ganguly, T., Kajfasz, J. K., Abranches, J., and Lemos, J. A. (2020). Regulatory circuits controlling Spx levels in Streptococcus mutans. Mol. Microbiol. 114 (1), 109–126. doi:10.1111/mmi.14499
Garcia-Contreras, R., Chavez-Granados, P. A., Jurado, C. A., Aranda-Herrera, B., Afrashtehfar, K. I., and Nurrohman, H. (2023). Natural bioactive epigallocatechin-gallate promote bond strength and differentiation of odontoblast-like cells. Biomimetics (Basel) 8 (1), 75. doi:10.3390/biomimetics8010075
Gartika, M., Pramesti, H. T., Kurnia, D., and Satari, M. H. (2018). A terpenoid isolated from sarang semut (Myrmecodia pendans) bulb and its potential for the inhibition and eradication of Streptococcus mutans biofilm. BMC Complement. Altern. Med. 18 (1), 151. doi:10.1186/s12906-018-2213-x
GBD 2017 Disease and Injury Incidence and Prevalence Collaborators (2018). Global, regional, and national incidence, prevalence, and years lived with disability for 354 diseases and injuries for 195 countries and territories, 1990-2017: a systematic analysis for the Global Burden of Disease Study 2017. Lancet 392 (10159), 1789–1858. doi:10.1016/s0140-6736(18)32279-7
Gimbel, M., Correa, A., and Lin, L. M. (2002). Calcium hydroxide as a temporary filling of the post space in root-filled teeth. Oral Surg. Oral Med. Oral Pathol. Oral Radiol. Endod. 94 (1), 98–102. doi:10.1067/moe.2002.123865
Gonçalves, R., Ayres, V. F. S., Carvalho, C. E., Souza, M. G. M., Guimarães, A. C., Corrêa, G. M., et al. (2017). Chemical composition and antibacterial activity of the essential oil of vitex agnus-castus L. (Lamiaceae). Acad Bras Cienc 89 (4), 2825–2832. doi:10.1590/0001-3765201720170428
Guan, X., Zhou, Y., Liang, X., Xiao, J., He, L., and Li, J. (2012). Effects of compounds found in Nidus Vespae on the growth and cariogenic virulence factors of Streptococcus mutans. Microbiol. Res. 167 (2), 61–68. doi:10.1016/j.micres.2011.03.002
Guo, R., Peng, W., Yang, H., Yao, C., Yu, J., and Huang, C. (2021). Evaluation of resveratrol-doped adhesive with advanced dentin bond durability. J. Dent. 114, 103817. doi:10.1016/j.jdent.2021.103817
Gutierrez, J. A., Crowley, P. J., Cvitkovitch, D. G., Brady, L. J., Hamilton, I. R., Hillman, J. D., et al. (1999). Streptococcus mutans ffh, a gene encoding a homologue of the 54 kDa subunit of the signal recognition particle, is involved in resistance to acid stress. Microbiol. Read. 145 (Pt 2), 357–366. doi:10.1099/13500872-145-2-357
Guzmán-Flores, J. M., Pérez-Reyes, Á., Vázquez-Jiménez, S. I., Isiordia-Espinoza, M. A., and Martínez-Esquivias, F. (2024). A docking and network pharmacology study on the molecular mechanisms of curcumin in dental caries and Streptococcus mutans. Dent. J. (Basel) 12 (6), 153. doi:10.3390/dj12060153
Hairul Islam, M. I., Arokiyaraj, S., Kuralarasan, M., Senthil Kumar, V., Harikrishnan, P., Saravanan, S., et al. (2020). Inhibitory potential of EGCG on Streptococcus mutans biofilm: a new approach to prevent Cariogenesis. Microb. Pathog. 143, 104129. doi:10.1016/j.micpath.2020.104129
Haj-Yahya, F., Steinberg, D., and Sionov, R. V. (2024). Trans, trans-farnesol enhances the anti-bacterial and anti-biofilm effect of arachidonic acid on the cariogenic bacteria Streptococcus mutans and Streptococcus sobrinus. Int. J. Mol. Sci. 25 (21), 11770. doi:10.3390/ijms252111770
Ham, Y., and Kim, T. J. (2023). Synergistic inhibitory activity of Glycyrrhizae Radix and Rubi Fructus extracts on biofilm formation of Streptococcus mutans. BMC Complement. Med. Ther. 23 (1), 22. doi:10.1186/s12906-023-03861-9
Han, S., Abiko, Y., Washio, J., Luo, Y., Zhang, L., and Takahashi, N. (2021). Green tea-derived epigallocatechin gallate inhibits acid production and promotes the aggregation of Streptococcus mutans and non-mutans streptococci. Caries Res. 55 (3), 205–214. doi:10.1159/000515814
Han, S., Washio, J., Abiko, Y., Zhang, L., and Takahashi, N. (2023). Green tea-derived catechins suppress the acid productions of Streptococcus mutans and enhance the efficiency of fluoride. Caries Res. 57 (3), 255–264. doi:10.1159/000534055
Hattarki, S. A., Bogar, C., and Bhat, K. G. (2021). Green tea catechins showed antibacterial activity on streptococcus mutans -An in vitro study. Indian J. Dent. Res. 32 (2), 226–229. doi:10.4103/ijdr.ijdr_512_21
Hazzah, H. A., Farid, R. M., Nasra, M. M. A., Hazzah, W. A., El-Massik, M. A., and Abdallah, O. Y. (2015). Gelucire-based nanoparticles for curcumin targeting to oral mucosa: preparation, characterization, and antimicrobial activity assessment. J. Pharm. Sci. 104 (11), 3913–3924. doi:10.1002/jps.24590
He, Z., Huang, Z., Jiang, W., and Zhou, W. (2019). Antimicrobial activity of cinnamaldehyde on Streptococcus mutans biofilms. Front. Microbiol. 10, 2241. doi:10.3389/fmicb.2019.02241
He, Z., Wang, Q., Hu, Y., Liang, J., Jiang, Y., Ma, R., et al. (2012). Use of the quorum sensing inhibitor furanone C-30 to interfere with biofilm formation by Streptococcus mutans and its luxS mutant strain. Int. J. Antimicrob. Agents 40 (1), 30–35. doi:10.1016/j.ijantimicag.2012.03.016
Hescot, P. (2017). The new definition of oral health and relationship between oral health and quality of life. Chin. J. Dent. Res. 20 (4), 189–192. doi:10.3290/j.cjdr.a39217
Higuchi, M., Abiko, Y., Washio, J., and Takahashi, N. (2024). Antimicrobial effects of epigallocatechin-3-gallate, a catechin abundant in green tea, on periodontal disease-associated bacteria. Arch. Oral Biol. 167, 106063. doi:10.1016/j.archoralbio.2024.106063
Higuchi, M., Yamamoto, Y., Poole Leslie, B., Shimada, M., Sato, Y., Takahashi, N., et al. (1999). Functions of two types of NADH oxidases in energy metabolism and oxidative stress of Streptococcus mutans. J. Bacteriol. 181 (19), 5940–5947. doi:10.1128/jb.181.19.5940-5947.1999
Hirasawa, M., Takada, K., and Otake, S. (2006). Inhibition of acid production in dental plaque bacteria by green tea catechins. Caries Res. 40 (3), 265–270. doi:10.1159/000092236
Horev, B., Klein, M. I., Hwang, G., Li, Y., Kim, D., Koo, H., et al. (2015). pH-activated nanoparticles for controlled topical delivery of farnesol to disrupt oral biofilm virulence. ACS Nano 9 (3), 2390–2404. doi:10.1021/nn507170s
Hosseinpour-Nader, A., Karimi, N., Ghafari, H. A., and Ghorbanzadeh, R. (2022). Effect of nanomicelle curcumin-based photodynamic therapy on the dynamics of white spot lesions and virulence of Streptococcus mutans in patients undergoing fixed orthodontic treatment: a randomized double-blind clinical trial. Photodiagnosis Photodyn. Ther. 40, 103183. doi:10.1016/j.pdpdt.2022.103183
Hu, J., Du, X., Huang, C., Fu, D., Ouyang, X., and Wang, Y. (2013a). Antibacterial and physical properties of EGCG-containing glass ionomer cements. J. Dent. 41 (10), 927–934. doi:10.1016/j.jdent.2013.07.014
Hu, P., Huang, P., and Chen, W. M. (2013b). Curcumin inhibits the Sortase A activity of the Streptococcus mutans UA159. Appl. Biochem. Biotechnol. 171 (2), 396–402. doi:10.1007/s12010-013-0378-9
Hu, P., Lv, B., Yang, K., Lu, Z., and Ma, J. (2021). Discovery of myricetin as an inhibitor against Streptococcus mutans and an anti-adhesion approach to biofilm formation. Int. J. Med. Microbiol. 311 (4), 151512. doi:10.1016/j.ijmm.2021.151512
Hu, Z., Tang, Y., Jiang, B., Xu, Y., Liu, S., and Huang, C. (2023). Functional liposome loaded curcumin for the treatment of Streptococcus mutans biofilm. Front. Chem. 11, 1160521. doi:10.3389/fchem.2023.1160521
Ito, T., Sims, K. R., Liu, Y., Xiang, Z., Arthur, R. A., Hara, A. T., et al. (2022). Farnesol delivery via polymeric nanoparticle carriers inhibits cariogenic cross-kingdom biofilms and prevents enamel demineralization. Mol. Oral Microbiol. 37 (5), 218–228. doi:10.1111/omi.12379
Jabbarifar, S. E., Salavati, S., Akhavan, A., Khosravi, K., Tavakoli, N., and Nilchian, F. (2011). Effect of fluoridated dentifrices on surface microhardness of the enamel of deciduous teeth. Dent. Res. J. (Isfahan) 8 (3), 113–117.
Jafri, H., Banerjee, G., Khan, M. S. A., Ahmad, I., Abulreesh, H. H., and Althubiani, A. S. (2020). Synergistic interaction of eugenol and antimicrobial drugs in eradication of single and mixed biofilms of Candida albicans and Streptococcus mutans. Amb. Express 10 (1), 185. doi:10.1186/s13568-020-01123-2
Jafri, H., Khan, M. S. A., and Ahmad, I. (2019). In vitro efficacy of eugenol in inhibiting single and mixed-biofilms of drug-resistant strains of Candida albicans and Streptococcus mutans. Phytomedicine 54, 206–213. doi:10.1016/j.phymed.2018.10.005
Jahanizadeh, S., Yazdian, F., Marjani, A., Omidi, M., and Rashedi, H. (2017). Curcumin-loaded chitosan/carboxymethyl starch/montmorillonite bio-nanocomposite for reduction of dental bacterial biofilm formation. Int. J. Biol. Macromol. 105 (Pt 1), 757–763. doi:10.1016/j.ijbiomac.2017.07.101
Jailani, A., Kalimuthu, S., Rajasekar, V., Ghosh, S., Collart-Dutilleul, P. Y., Fatima, N., et al. (2022). Trans-cinnamaldehyde eluting porous silicon microparticles mitigate cariogenic biofilms. Pharmaceutics 14 (7), 1428. doi:10.3390/pharmaceutics14071428
Jeon, J. G., Klein, M. I., Xiao, J., Gregoire, S., Rosalen, P. L., and Koo, H. (2009). Influences of naturally occurring agents in combination with fluoride on gene expression and structural organization of Streptococcus mutans in biofilms. BMC Microbiol. 9, 228. doi:10.1186/1471-2180-9-228
Jeon, J. G., Pandit, S., Xiao, J., Gregoire, S., Falsetta, M. L., Klein, M. I., et al. (2011). Influences of trans-trans farnesol, a membrane-targeting sesquiterpenoid, on Streptococcus mutans physiology and survival within mixed-species oral biofilms. Int. J. Oral Sci. 3 (2), 98–106. doi:10.4248/ijos11038
Jung, J. E., Pandit, S., and Jeon, J. G. (2014). Identification of linoleic acid, a main component of the n-hexane fraction from Dryopteris crassirhizoma, as an anti-Streptococcus mutans biofilm agent. Biofouling 30 (7), 789–798. doi:10.1080/08927014.2014.930446
Jyothi, K. S., and Seshagiri, M. (2012). In-vitro activity of saponins of bauhinia purpurea, madhuca longifolia, celastrus paniculatus and semecarpus anacardium on selected oral pathogens. J. Dent. (Tehran) 9(4), 216–223.
Kajfasz, J. K., Ganguly, T., Hardin, E. L., Abranches, J., and Lemos, J. A. (2017). Transcriptome responses of Streptococcus mutans to peroxide stress: identification of novel antioxidant pathways regulated by Spx. Sci. Rep. 7(1), 16018. doi:10.1038/s41598-017-16367-5
Karimi, N., Jabbari, V., Nazemi, A., Ganbarov, K., Karimi, N., Tanomand, A., et al. (2020). Thymol, cardamom and Lactobacillus plantarum nanoparticles as a functional candy with high protection against Streptococcus mutans and tooth decay. Microb. Pathog. 148, 104481. doi:10.1016/j.micpath.2020.104481
Kashi, M., Noei, M., Chegini, Z., and Shariati, A. (2024). Natural compounds in the fight against Staphylococcus aureus biofilms: a review of antibiofilm strategies. Front. Pharmacol. 15, 1491363. doi:10.3389/fphar.2024.1491363
Kassebaum, N. J., Smith, A. G. C., Bernabé, E., Fleming, T. D., Reynolds, A. E., Vos, T., et al. (2017). Global, regional, and national prevalence, incidence, and disability-adjusted life years for oral conditions for 195 countries, 1990-2015: a systematic analysis for the global burden of diseases, injuries, and risk factors. J. Dent. Res. 96 (4), 380–387. doi:10.1177/0022034517693566
Ke, L., Wang, J., Liu, Y., Sun, Z., Li, Y., and Xiao, X. (2023). Identification of the antibacterial action mechanism of curcumin on Streptococcus mutans through transcriptome profiling. Arch. Oral Biol. 149, 105655. doi:10.1016/j.archoralbio.2023.105655
Khan, S. T., Khan, M., Ahmad, J., Wahab, R., Abd-Elkader, O. H., Musarrat, J., et al. (2017). Thymol and carvacrol induce autolysis, stress, growth inhibition and reduce the biofilm formation by Streptococcus mutans. Amb. Express 7 (1), 49. doi:10.1186/s13568-017-0344-y
Koo, H., Hayacibara, M. F., Schobel, B. D., Cury, J. A., Rosalen, P. L., Park, Y. K., et al. (2003). Inhibition of Streptococcus mutans biofilm accumulation and polysaccharide production by apigenin and tt-farnesol. J. Antimicrob. Chemother. 52 (5), 782–789. doi:10.1093/jac/dkg449
Koo, H., Rosalen, P. L., Cury, J. A., Park, Y. K., and Bowen, W. H. (2002). Effects of compounds found in propolis on Streptococcus mutans growth and on glucosyltransferase activity. Antimicrob. Agents Chemother. 46 (5), 1302–1309. doi:10.1128/aac.46.5.1302-1309.2002
Koo, H., Schobel, B., Scott-Anne, K., Watson, G., Bowen, W. H., Cury, J. A., et al. (2005). Apigenin and tt-farnesol with fluoride effects on S. mutans biofilms and dental caries. J. Dent. Res. 84 (11), 1016–1020. doi:10.1177/154405910508401109
Koo, H., Seils, J., Abranches, J., Burne, R. A., Bowen, W. H., and Quivey, R. G. (2006). Influence of apigenin on gtf gene expression in Streptococcus mutans UA159. Antimicrob. Agents Chemother. 50 (2), 542–546. doi:10.1128/aac.50.2.542-546.2006
Krzyściak, W., Jurczak, A., Kościelniak, D., Bystrowska, B., and Skalniak, A. (2014). The virulence of Streptococcus mutans and the ability to form biofilms. Eur. J. Clin. Microbiol. Infect. Dis. 33 (4), 499–515. doi:10.1007/s10096-013-1993-7
Kuhnert, W. L., Zheng, G., Faustoferri, R. C., and Quivey, R. G. (2004). The F-ATPase operon promoter of Streptococcus mutans is transcriptionally regulated in response to external pH. J. Bacteriol. 186 (24), 8524–8528. doi:10.1128/jb.186.24.8524-8528.2004
Landeo-Villanueva, G. E., Salazar-Salvatierra, M. E., Ruiz-Quiroz, J. R., Zuta-Arriola, N., Jarama-Soto, B., Herrera-Calderon, O., et al. (2023). Inhibitory activity of essential oils of mentha spicata and Eucalyptus globulus on biofilms of Streptococcus mutans in an in vitro model. Antibiot. (Basel) 12 (2), 369. doi:10.3390/antibiotics12020369
Lee, H. J., Kang, S. M., Jeong, S. H., Chung, K. H., and Kim, B. I. (2017). Antibacterial photodynamic therapy with curcumin and Curcuma xanthorrhiza extract against Streptococcus mutans. Photodiagnosis Photodyn. Ther. 20, 116–119. doi:10.1016/j.pdpdt.2017.09.003
Lee, J. S., Choi, Y. S., and Lee, H. G. (2020). Synergistic antimicrobial properties of nanoencapsulated clove oil and thymol against oral bacteria. Food Sci. Biotechnol. 29 (11), 1597–1604. doi:10.1007/s10068-020-00803-w
Lemos, J. A., Palmer, S. R., Zeng, L., Wen, Z. T., Kajfasz, J. K., Freires, I. A., et al. (2019). The Biology of Streptococcus mutans. Microbiol. Spectr. 7 (1). doi:10.1128/microbiolspec.GPP3-0051-2018
Lemos José, A. C., Brown Thomas, A., and Burne Robert, A. (2004). Effects of RelA on key virulence properties of planktonic and biofilm populations of Streptococcus mutans. Infect. Immun. 72 (3), 1431–1440. doi:10.1128/iai.72.3.1431-1440.2004
Li, B., Li, X., Lin, H., and Zhou, Y. (2018). Curcumin as a promising antibacterial agent: effects on metabolism and biofilm formation in S. Mutans. Biomed. Res. Int. 2018, 4508709. doi:10.1155/2018/4508709
Li, B., Pan, T., Lin, H., and Zhou, Y. (2020a). The enhancing antibiofilm activity of curcumin on Streptococcus mutans strains from severe early childhood caries. BMC Microbiol. 20 (1), 286. doi:10.1186/s12866-020-01975-5
Li, J., Wu, T., Peng, W., and Zhu, Y. (2020b). Effects of resveratrol on cariogenic virulence properties of Streptococcus mutans. BMC Microbiol. 20 (1), 99. doi:10.1186/s12866-020-01761-3
Li, X., Yin, L., Ramage, G., Li, B., Tao, Y., Zhi, Q., et al. (2019). Assessing the impact of curcumin on dual-species biofilms formed by Streptococcus mutans and Candida albicans. Microbiologyopen 8 (12), e937. doi:10.1002/mbo3.937
Liao, M. H., Wang, X. R., Hsu, W. L., and Tzen, J. T. C. (2021). Pu'er tea rich in strictinin and catechins prevents biofilm formation of two cariogenic bacteria, Streptococcus mutans and Streptococcus sobrinus. J. Dent. Sci. 16 (4), 1331–1334. doi:10.1016/j.jds.2021.05.011
Liu, Y., Ren, Z., Hwang, G., and Koo, H. (2018). Therapeutic strategies targeting cariogenic biofilm microenvironment. Adv. Dent. Res. 29 (1), 86–92. doi:10.1177/0022034517736497
Lobo, C. I., Barbugli, P. A., Rocha, G. R., and Klein, M. I. (2022). Topical application of 4'-hydroxychalcone in combination with tt-farnesol is effective against Candida albicans and Streptococcus mutans biofilms. ACS Omega 7 (26), 22773–22786. doi:10.1021/acsomega.2c02318
Lobo, C. I. V., Lopes, A., and Klein, M. I. (2021). Compounds with distinct targets present diverse antimicrobial and antibiofilm efficacy against Candida albicans and Streptococcus mutans, and combinations of compounds potentiate their effect. J. Fungi (Basel) 7 (5), 340. doi:10.3390/jof7050340
Lopes, A., Lobo, C. I. V., Ribeiro, S. M., Colin, J. D. S., Constantino, V. C. N., Canonici, M. M., et al. (2022). Distinct agents induce Streptococcus mutans cells with altered biofilm formation capacity. Microbiol. Spectr. 10 (4), e0065022. doi:10.1128/spectrum.00650-22
Maggi, F., Bramucci, M., Cecchini, C., Coman, M. M., Cresci, A., Cristalli, G., et al. (2009). Composition and biological activity of essential oil of Achillea ligustica All. (Asteraceae) naturalized in central Italy: ideal candidate for anti-cariogenic formulations. Fitoterapia 80 (6), 313–319. doi:10.1016/j.fitote.2009.04.004
Makarla, S., Venugopal, R., Bavle, R. M., Selvan, A. K., Muniswamappa, S. S., and Dinesh, R. (2023). Determining the best anti-microbial properties of dental cements used for pulp capping procedures using deep dentinal carious material. J. Oral Maxillofac. Pathol. 27 (1), 239. doi:10.4103/jomfp.jomfp_109_21
Malvania, E. A., Sharma, A. S., Sheth, S. A., Rathod, S., Chovatia, N. R., and Kachwala, M. S. (2019). In vitro analysis of licorice (Glycyrrhiza glabra) root extract activity on Streptococcus mutans in comparison to chlorhexidine and fluoride mouthwash. J. Contemp. Dent. Pract. 20 (12), 1389–1394. doi:10.5005/jp-journals-10024-2724
Marchese, A., and Debbia, E. A. (2016). The role of gyrA, gyrB, and dnaA functions in bacterial conjugation. Ann. Microbiol. 66(1), 223–228. doi:10.1007/s13213-015-1098-x
Marsh, P. D., and Zaura, E. (2017). Dental biofilm: ecological interactions in health and disease. J. Clin. Periodontol. 44 (Suppl. 18), S12–s22. doi:10.1111/jcpe.12679
Merigo, E., Conti, S., Ciociola, T., Manfredi, M., Vescovi, P., and Fornaini, C. (2019). Antimicrobial photodynamic therapy protocols on Streptococcus mutans with different combinations of wavelengths and photosensitizing dyes. Bioeng. (Basel) 6 (2), 42. doi:10.3390/bioengineering6020042
Mitrakul, K., Loo, C. Y., Hughes, C. V., and Ganeshkumar, N. (2004). Role of a Streptococcus gordonii copper-transport operon, copYAZ, in biofilm detachment. Oral Microbiol. Immunol. 19 (6), 395–402. doi:10.1111/j.1399-302x.2004.00176.x
Mogen, A. B., Chen, F., Ahn, S. J., Burne, R. A., Wang, D., and Rice, K. C. (2015). Pluronics-formulated farnesol promotes efficient killing and demonstrates novel interactions with Streptococcus mutans biofilms. PLoS One 10 (7), e0133886. doi:10.1371/journal.pone.0133886
Monajemzadeh, A., Ahmadi Asoor, S., Aslani, S., and Sadeghi-Nejad, B. (2017). In vitro antimicrobial effect of different root canal sealers against oral pathogens. Curr. Med. Mycol. 3 (2), 7–12. doi:10.18869/acadpub.cmm.3.2.7
Mu, R., Zhang, H., Zhang, Z., Li, X., Ji, J., Wang, X., et al. (2023). Trans-cinnamaldehyde loaded chitosan based nanocapsules display antibacterial and antibiofilm effects against cavity-causing Streptococcus mutans. J. Oral Microbiol. 15 (1), 2243067. doi:10.1080/20002297.2023.2243067
Ngokwe, Z. B., Wolfoviz-Zilberman, A., Sharon, E., Zabrovsky, A., Beyth, N., Houri-Haddad, Y., et al. (2024). Trans-cinnamaldehyde-fighting Streptococcus mutans using nature. Pharmaceutics 16 (1), 113. doi:10.3390/pharmaceutics16010113
Nima, G., Soto-Montero, J., Alves, L. A., Mattos-Graner, R. O., and Giannini, M. (2021). Photodynamic inactivation of Streptococcus mutans by curcumin in combination with EDTA. Dent. Mater 37 (1), e1–e14. doi:10.1016/j.dental.2020.09.015
Noriega, P., Ballesteros, J., De La Cruz, A., and Veloz, T. (2020). Chemical composition and preliminary antimicrobial activity of the hydroxylated sesquiterpenes in the essential oil from piper barbatum kunth leaves. Plants (Basel) 9 (2), 211. doi:10.3390/plants9020211
Nunes, J., Farias, I. a.P., Vieira, C. A., Ribeiro, T. M., Sampaio, F. C., and Menezes, V. A. (2020). Antimicrobial activity and toxicity of glass ionomer cement containing an essential oil. Braz J. Med. Biol. Res. 53 (12), e9468. doi:10.1590/1414-431x20209468
Park, S. Y., Raka, R. N., Hui, X. L., Song, Y., Sun, J. L., Xiang, J., et al. (2023). Six Spain Thymus essential oils composition analysis and their in vitro and in silico study against Streptococcus mutans. BMC Complement. Med. Ther. 23 (1), 106. doi:10.1186/s12906-023-03928-7
Paschoal, M. A., Lin, M., Santos-Pinto, L., and Duarte, S. (2015). Photodynamic antimicrobial chemotherapy on Streptococcus mutans using curcumin and toluidine blue activated by a novel LED device. Lasers Med. Sci. 30 (2), 885–890. doi:10.1007/s10103-013-1492-1
Paschoal, M. A., Santos-Pinto, L., Lin, M., and Duarte, S. (2014). Streptococcus mutans photoinactivation by combination of short exposure of a broad-spectrum visible light and low concentrations of photosensitizers. Photomed. Laser Surg. 32 (3), 175–180. doi:10.1089/pho.2013.3656
Paschoal, M. A., Tonon, C. C., Spolidório, D. M., Bagnato, V. S., Giusti, J. S., and Santos-Pinto, L. (2013). Photodynamic potential of curcumin and blue LED against Streptococcus mutans in a planktonic culture. Photodiagnosis Photodyn. Ther. 10 (3), 313–319. doi:10.1016/j.pdpdt.2013.02.002
Patra, J. K., Kim, E. S., Oh, K., Kim, H. J., Kim, Y., and Baek, K. H. (2014). Antibacterial effect of crude extract and metabolites of Phytolacca americana on pathogens responsible for periodontal inflammatory diseases and dental caries. BMC Complement. Altern. Med. 14, 343. doi:10.1186/1472-6882-14-343
Paulino, T. P., Ribeiro, K. F., Thedei, G., Tedesco, A. C., and Ciancaglini, P. (2005). Use of hand held photopolymerizer to photoinactivate Streptococcus mutans. Arch. Oral Biol. 50 (3), 353–359. doi:10.1016/j.archoralbio.2004.09.002
Pinna, R., Filigheddu, E., Juliano, C., Palmieri, A., Manconi, M., D'hallewin, G., et al. (2019). Antimicrobial effect of thymus capitatus and citrus limon var. pompia as raw extracts and nanovesicles. Pharmaceutics 11 (5), 234. doi:10.3390/pharmaceutics11050234
Pordel, E., Ghasemi, T., Afrasiabi, S., Benedicenti, S., Signore, A., and Chiniforush, N. (2023). The effect of different output powers of blue diode laser along with curcumin and riboflavin against Streptococcus mutans around orthodontic brackets: an in vitro study. Biomedicines 11 (8), 2248. doi:10.3390/biomedicines11082248
Pourhajibagher, M., Noroozian, M., Ahmad Akhoundi, M. S., and Bahador, A. (2022). Antimicrobial effects and mechanical properties of poly(methyl methacrylate) as an orthodontic acrylic resin containing Curcumin-Nisin-poly(L-lactic acid) nanoparticle: an in vitro study. BMC Oral Health 22 (1), 158. doi:10.1186/s12903-022-02197-z
Pournasir, M., Alavi, F. N., Davalloo, R. T., Ebrahim-Saraie, H. S., and Ghaffari, M. E. (2024). Antibacterial effect of resveratrol extract compared to chlorhexidine mouthwash against primary cariogenic pathogen, Streptococcus mutans. J. Clin. Exp. Dent. 16 (7), e802–e807. doi:10.4317/jced.61456
Priya, A., Selvaraj, A., Divya, D., Karthik Raja, R., and Pandian, S. K. (2021). In vitro and in vivo anti-infective potential of thymol against early childhood caries causing dual species Candida albicans and Streptococcus mutans. Front. Pharmacol. 12, 760768. doi:10.3389/fphar.2021.760768
Quishida, C. C., De Oliveira Mima, E. G., Jorge, J. H., Vergani, C. E., Bagnato, V. S., and Pavarina, A. C. (2016). Photodynamic inactivation of a multispecies biofilm using curcumin and LED light. Lasers Med. Sci. 31 (5), 997–1009. doi:10.1007/s10103-016-1942-7
Rangel, M. L., De Aquino, S. G., De Lima, J. M., Castellano, L. R., and De Castro, R. D. (2018). In vitro effect of cinnamomum zeylanicum blume essential oil on Candida spp. involved in oral infections. Evid. Based Complement. Altern. Med. 2018, 4045013. doi:10.1155/2018/4045013
Ribeiro, M., Malheiro, J., Grenho, L., Fernandes, M. H., and Simões, M. (2018). Cytotoxicity and antimicrobial action of selected phytochemicals against planktonic and sessile Streptococcus mutans. PeerJ 6, e4872. doi:10.7717/peerj.4872
Rocha, G. R., Florez Salamanca, E. J., De Barros, A. L., Lobo, C. I. V., and Klein, M. I. (2018). Effect of tt-farnesol and myricetin on in vitro biofilm formed by Streptococcus mutans and Candida albicans. BMC Complement. Altern. Med. 18 (1), 61. doi:10.1186/s12906-018-2132-x
Roncari Rocha, G., Sims, K. R., Xiao, B., Klein, M. I., and Benoit, D. S. W. (2022). Nanoparticle carrier co-delivery of complementary antibiofilm drugs abrogates dual species cariogenic biofilm formation in vitro. J. Oral Microbiol. 14 (1), 1997230. doi:10.1080/20002297.2021.1997230
Ryan, M. E. (2005). Nonsurgical approaches for the treatment of periodontal diseases. Dent. Clin. North Am. 49(3), 611–636. doi:10.1016/j.cden.2005.03.010
Sajomsang, W., Nuchuchua, O., Gonil, P., Saesoo, S., Sramala, I., Soottitantawat, A., et al. (2012). Water-soluble β-cyclodextrin grafted with chitosan and its inclusion complex as a mucoadhesive eugenol carrier. Carbohydr. Polym. 89 (2), 623–631. doi:10.1016/j.carbpol.2012.03.060
Sanches, C. V. G., Sardi, J. C. O., Terada, R. S. S., Lazarini, J. G., Freires, I. A., Polaquini, C. R., et al. (2019). Diacetylcurcumin: a new photosensitizer for antimicrobial photodynamic therapy in Streptococcus mutans biofilms. Biofouling 35 (3), 340–349. doi:10.1080/08927014.2019.1606907
Sasaki, H., Matsumoto, M., Tanaka, T., Maeda, M., Nakai, M., Hamada, S., et al. (2004). Antibacterial activity of polyphenol components in oolong tea extract against Streptococcus mutans. Caries Res. 38 (1), 2–8. doi:10.1159/000073913
Sawamura, S., Tonosaki, Y., and Hamada, S. (1992). Inhibitory effects of ellagic acid on glucosyltransferases from mutans streptococci. Biosci. Biotechnol. Biochem. 56 (5), 766–768. doi:10.1271/bbb.56.766
Schneider-Rayman, M., Steinberg, D., Sionov, R. V., Friedman, M., and Shalish, M. (2021). Effect of epigallocatechin gallate on dental biofilm of Streptococcus mutans: an in vitro study. BMC Oral Health 21 (1), 447. doi:10.1186/s12903-021-01798-4
Sekiya, M., Izumisawa, S., Iwamoto-Kihara, A., Fan, Y., Shimoyama, Y., Sasaki, M., et al. (2019). Proton-pumping F-ATPase plays an important role in Streptococcus mutans under acidic conditions. Arch. Biochem. Biophys. 666, 46–51. doi:10.1016/j.abb.2019.03.014
Senadheera, D., Krastel, K., Mair, R., Persadmehr, A., Abranches, J., Burne, R. A., et al. (2009). Inactivation of VicK affects acid production and acid survival of Streptococcus mutans. J. Bacteriol. 191 (20), 6415–6424. doi:10.1128/jb.00793-09
Senadheera, M. D., Guggenheim, B., Spatafora, G. A., Huang, Y. C., Choi, J., Hung, D. C., et al. (2005). A VicRK signal transduction system in Streptococcus mutans affects gtfBCD, gbpB, and ftf expression, biofilm formation, and genetic competence development. J. Bacteriol. 187 (12), 4064–4076. doi:10.1128/jb.187.12.4064-4076.2005
Serino, G., Rosling, B., Ramberg, P., Socransky, S. S., and Lindhe, J. (2001). Initial outcome and long-term effect of surgical and non-surgical treatment of advanced periodontal disease. J. Clin. Periodontol. 28 (10), 910–916. doi:10.1034/j.1600-051x.2001.028010910.x
Shad, A. A., Ahmad, S., Ullah, R., Abdel-Salam, N. M., Fouad, H., Ur Rehman, N., et al. (2014). Phytochemical and biological activities of four wild medicinal plants. ScientificWorldJournal 2014, 857363. doi:10.1155/2014/857363
Shariati, A., Didehdar, M., Razavi, S., Heidary, M., Soroush, F., and Chegini, Z. (2022). Natural compounds: a hopeful promise as an antibiofilm agent against Candida species. Front. Pharmacol. 13, 917787. doi:10.3389/fphar.2022.917787
Sharma, U. K., Sharma, A. K., and Pandey, A. K. (2016). Medicinal attributes of major phenylpropanoids present in cinnamon. BMC Complement. Altern. Med. 16, 156. doi:10.1186/s12906-016-1147-4
Sims, K. R., Maceren, J. P., Liu, Y., Rocha, G. R., Koo, H., and Benoit, D. S. W. (2020). Dual antibacterial drug-loaded nanoparticles synergistically improve treatment of Streptococcus mutans biofilms. Acta Biomater. 115, 418–431. doi:10.1016/j.actbio.2020.08.032
Sims, K. R., Liu, Y., Hwang, G., Jung, H.I., Koo, H., and Benoit, D. S. W. (2018). Enhanced design and formulation of nanoparticles for anti-biofilm drug delivery. Nanoscale 11 (1), 219–236. doi:10.1039/c8nr05784b
Song, J., Choi, B., Jin, E. J., Yoon, Y., and Choi, K. H. (2012). Curcumin suppresses Streptococcus mutans adherence to human tooth surfaces and extracellular matrix proteins. Eur. J. Clin. Microbiol. Infect. Dis. 31 (7), 1347–1352. doi:10.1007/s10096-011-1448-y
Soria-Lozano, P., Gilaberte, Y., Paz-Cristobal, M. P., Pérez-Artiaga, L., Lampaya-Pérez, V., Aporta, J., et al. (2015). In vitro effect photodynamic therapy with differents photosensitizers on cariogenic microorganisms. BMC Microbiol. 15, 187. doi:10.1186/s12866-015-0524-3
Stipp, R. N., Boisvert, H., Smith, D. J., Höfling, J. F., Duncan, M. J., and Mattos-Graner, R. O. (2013). CovR and VicRK regulate cell surface biogenesis genes required for biofilm formation in Streptococcus mutans. PLoS One 8 (3), e58271. doi:10.1371/journal.pone.0058271
Sturr, M. G., and Marquis, R. E. (1992). Comparative acid tolerances and inhibitor sensitivities of isolated F-ATPases of oral lactic acid bacteria. Appl. Environ. Microbiol. 58 (7), 2287–2291. doi:10.1128/aem.58.7.2287-2291.1992
Supuran, C. T. (2015). Nanoparticles for controlled release of anti-biofilm agents WO2014130994 (A1): a patent evaluation. Expert Opin. Ther. Pat. 25 (8), 945–948. doi:10.1517/13543776.2015.1053207
Taylor, E. S., Gomez, G. F., Moser, E. a.S., Sanders, B. J., and Gregory, R. L. (2021). Effect of a tea polyphenol on different levels of exposure of nicotine and tobacco extract on Streptococcus mutans biofilm formation. Front. Oral Health 2, 737378. doi:10.3389/froh.2021.737378
Tonon, C. C., Paschoal, M. A., Correia, M., Spolidório, D. M., Bagnato, V. S., Giusti, J. S., et al. (2015). Comparative effects of photodynamic therapy mediated by curcumin on standard and clinical isolate of Streptococcus mutans. J. Contemp. Dent. Pract. 16 (1), 1–6. doi:10.5005/jp-journals-10024-1626
Tsukatani, T., Sakata, F., Kuroda, R., and Akao, T. (2020). Biofilm eradication activity of herb and spice extracts alone and in combination against oral and food-borne pathogenic bacteria. Curr. Microbiol. 77 (9), 2486–2495. doi:10.1007/s00284-020-02017-z
Veber, D. F., Johnson, S. R., Cheng, H.-Y., Smith, B. R., Ward, K. W., and Kopple, K. D. (2002). Molecular properties that influence the oral bioavailability of drug candidates. J. Med. Chem. 45 (12), 2615–2623. doi:10.1021/jm020017n
Vlachojannis, C., Chrubasik-Hausmann, S., Hellwig, E., and Al-Ahmad, A. (2015). A preliminary investigation on the antimicrobial activity of Listerine®, its components, and of mixtures thereof. Phytother. Res. 29 (10), 1590–1594. doi:10.1002/ptr.5399
Wang, T. H., Hsia, S. M., Wu, C. H., Ko, S. Y., Chen, M. Y., Shih, Y. H., et al. (2016). Evaluation of the antibacterial potential of liquid and vapor phase phenolic essential oil compounds against oral microorganisms. PLoS One 11 (9), e0163147. doi:10.1371/journal.pone.0163147
Wang, Y., and Ho, C. T. (2009). Polyphenolic chemistry of tea and coffee: a century of progress. J. Agric. Food Chem. 57 (18), 8109–8114. doi:10.1021/jf804025c
Wang, Y., and Lam, A. T. W. (2020). Epigallocatechin gallate and gallic acid affect colonization of abiotic surfaces by oral bacteria. Arch. Oral Biol. 120, 104922. doi:10.1016/j.archoralbio.2020.104922
Wen, Z. T., and Burne, R. A. (2004). LuxS-mediated signaling in Streptococcus mutans is involved in regulation of acid and oxidative stress tolerance and biofilm formation. J. Bacteriol. 186 (9), 2682–2691. doi:10.1128/jb.186.9.2682-2691.2004
Wen, Z. T., Nguyen, A. H., Bitoun, J. P., Abranches, J., Baker, H. V., and Burne, R. A. (2011). Transcriptome analysis of LuxS-deficient Streptococcus mutans grown in biofilms. Mol. Oral Microbiol. 26 (1), 2–18. doi:10.1111/j.2041-1014.2010.00581.x
Wu, C., Ayala Eduardo, A., Downey Jennifer, S., Merritt, J., Goodman Steven, D., and Qi, F. (2010). Regulation of ciaXRH operon expression and identification of the CiaR regulon in Streptococcus mutans. J. Bacteriol. 192 (18), 4669–4679. doi:10.1128/jb.00556-10
Wu, C. Y., Su, T. Y., Wang, M. Y., Yang, S. F., Mar, K., and Hung, S. L. (2018). Inhibitory effects of tea catechin epigallocatechin-3-gallate against biofilms formed from Streptococcus mutans and a probiotic lactobacillus strain. Arch. Oral Biol. 94, 69–77. doi:10.1016/j.archoralbio.2018.06.019
Wu, M., and Brown, A. C. (2021). Applications of catechins in the treatment of bacterial infections. Pathogens 10 (5), 546. doi:10.3390/pathogens10050546
Xu, J. S., Li, Y., Cao, X., and Cui, Y. (2013). The effect of eugenol on the cariogenic properties of Streptococcus mutans and dental caries development in rats. Exp. Ther. Med. 5 (6), 1667–1670. doi:10.3892/etm.2013.1066
Xu, X., Zhou, X. D., and Wu, C. D. (2011). The tea catechin epigallocatechin gallate suppresses cariogenic virulence factors of Streptococcus mutans. Antimicrob. Agents Chemother. 55 (3), 1229–1236. doi:10.1128/aac.01016-10
Xu, X., Zhou, X. D., and Wu, C. D. (2012). Tea catechin epigallocatechin gallate inhibits Streptococcus mutans biofilm formation by suppressing gtf genes. Arch. Oral Biol. 57 (6), 678–683. doi:10.1016/j.archoralbio.2011.10.021
Yabuta, Y., Mukoyama, H., Kaneda, Y., Kimura, N., Bito, T., Ichiyanagi, T., et al. (2018). A lemon myrtle extract inhibits glucosyltransferases activity of Streptococcus mutans. Biosci. Biotechnol. Biochem. 82 (9), 1584–1590. doi:10.1080/09168451.2018.1478714
Yang, H., Li, K., Yan, H., Liu, S., Wang, Y., and Huang, C. (2017). High-performance therapeutic quercetin-doped adhesive for adhesive-dentin interfaces. Sci. Rep. 7 (1), 8189. doi:10.1038/s41598-017-08633-3
Yazdanian, M., Rostamzadeh, P., Alam, M., Abbasi, K., Tahmasebi, E., Tebyaniyan, H., et al. (2022). Evaluation of antimicrobial and cytotoxic effects of Echinacea and Arctium extracts and Zataria essential oil. Amb. Express 12 (1), 75. doi:10.1186/s13568-022-01417-7
Yu, J., Yang, H., Li, K., Ren, H., Lei, J., and Huang, C. (2017). Development of epigallocatechin-3-gallate-encapsulated nanohydroxyapatite/mesoporous silica for therapeutic management of dentin surface. ACS Appl. Mater Interfaces 9 (31), 25796–25807. doi:10.1021/acsami.7b06597
Yu, J., Yi, L., Guo, R., Guo, J., Yang, H., and Huang, C. (2021). The stability of dentin surface biobarrier consisting of mesoporous delivery system on dentinal tubule occlusion and Streptococcus mutans biofilm inhibition. Int. J. Nanomedicine 16, 3041–3057. doi:10.2147/ijn.s290254
Zaini, N. N. M., Salleh, W., Arzmi, M. H., Salihu, A. S., and Ab Ghani, N. (2023). Chemical composition of essential oil from Lindera caesia Reinw. ex Fern.-Vill. and its antifungal, antibiofilm, and molecular docking studies. Nat. Prod. Res. 39, 1723–1728. doi:10.1080/14786419.2023.2298720
Zaltsman, N., Ionescu, A. C., Weiss, E. I., Brambilla, E., Beyth, S., and Beyth, N. (2017). Surface-modified nanoparticles as anti-biofilm filler for dental polymers. PLoS One 12 (12), e0189397. doi:10.1371/journal.pone.0189397
Zeng, Y., Nikitkova, A., Abdelsalam, H., Li, J., and Xiao, J. (2019). Activity of quercetin and kaemferol against Streptococcus mutans biofilm. Arch. Oral Biol. 98, 9–16. doi:10.1016/j.archoralbio.2018.11.005
Zhang, H., Mu, R., Wang, Z., Peng, S., Yang, X. Y., and Qin, X. (2024). Trans-cinnamaldehyde inhibition of pyruvate dehydrogenase: effects on Streptococcus mutans carbohydrate metabolism. J. Proteome Res. 23 (8), 3682–3695. doi:10.1021/acs.jproteome.4c00382
Keywords: natural compounds, Streptococcus mutans, biofilm, dental caries, new treatment
Citation: Kashi M, Varseh M, Hariri Y, Chegini Z and Shariati A (2025) Natural compounds: new therapeutic approach for inhibition of Streptococcus mutans and dental caries. Front. Pharmacol. 16:1548117. doi: 10.3389/fphar.2025.1548117
Received: 19 December 2024; Accepted: 12 March 2025;
Published: 01 April 2025.
Edited by:
Santosh Pandit, Chalmers University of Technology, SwedenReviewed by:
Arijit Bhattacharya, Adamas University, IndiaLong Chen, Beijing University of Chemical Technology, China
Copyright © 2025 Kashi, Varseh, Hariri, Chegini and Shariati. This is an open-access article distributed under the terms of the Creative Commons Attribution License (CC BY). The use, distribution or reproduction in other forums is permitted, provided the original author(s) and the copyright owner(s) are credited and that the original publication in this journal is cited, in accordance with accepted academic practice. No use, distribution or reproduction is permitted which does not comply with these terms.
*Correspondence: Aref Shariati, YXJlZnNoYXJpYXRpMDExMUBnbWFpbC5jb20=; Zahra Chegini, cGFyaXNhLmNoZWdpbmk3MkBnbWFpbC5jb20=