- 1Department of Orthopedics, The First Hospital of Jilin University, Changchun, China
- 2Department of Orthopaedic Surgery, The First Affiliated Hospital of Shandong First Medical University & Shandong Provincial Qianfoshan Hospital, Jinan, China
- 3Department of Gynecology and Obstetrics, Jinan Maternity and Child Care Hospital Affiliated to Shandong First Medical University, Jinan, China
Osteosarcoma is the most common primary malignant bone tumor. Although surgery and chemoradiotherapy have made some progress in the treatment of osteosarcoma. However, the high recurrence and metastasis rate of osteosarcoma and bone defects caused by surgery are still the main problems faced by osteosarcoma. Gelatin has excellent biocompatibility and biodegradability, and has made phased progress in tumor treatment. In the treatment of osteosarcoma, gelatin-based biomaterials can be used in delivery strategies to enhance the anti-tumor activity of osteosarcoma and can improve the appropriate compressive strength to improve the bone defects faced after surgery. At present, gelatin-based hydrogels, gelatin scaffolds, and gelatin-based nanoparticles have been reported in preclinical studies. In this article, we introduce the application of gelatin-based biomaterials in the treatment of osteosarcoma, and summarize and look forward to them.
1 Introduction
Osteosarcoma (OS) is the most common primary malignant bone tumor in children and adolescents (Beird et al., 2022). Osteosarcoma is more common in the distal femur, proximal tibia, and humerus, and osteosarcoma cells are derived from osteoblast mesenchymal stem cells (Dong et al., 2022). 4.4 patients with osteosarcoma per million population have been reported (Mirabello et al., 2009). Patients with osteosarcoma often present with excruciating, excruciating pain (Bian et al., 2023). Because osteosarcoma tends to occur in bone, its destruction of bone is usually prone to pathological fractures. OS occurs in osteoid produced by mesenchymal stem cells and osteoblast precursors or osteoblast precursors, so osteosarcoma is extremely malignant, and most of the OS will infiltrate into surrounding tissues and even metastasize at an early stage (Yang et al., 2020). The lung is the most common organ of osteosarcoma metastases (Jiang and He, 2023). Second, osteosarcoma cells have no specific markers, so early osteosarcoma is not easy to detect (Yuan P. et al., 2023). This is another reason for the high mortality rate of osteosarcoma.
Over the past few years, surgical resection and chemotherapy have made significant advances in the treatment of osteosarcoma (Ning et al., 2023). Surgical resection, combination chemotherapy, and targeted therapy for osteosarcoma have a 5 year survival rate of around 60%–70% for patients without metastases (Marina et al., 2004). The scope of surgical removal of osteosarcoma usually exceeds the ability of the bone to repair itself, which not only causes irreparable damage to the bone tissue, but also greatly reduces the patient’s desire to survive (Le Nail et al., 2018). The rationale for OS treatment has shifted from amputation to limb salvage to preserve local functional integrity and improve the patient’s quality of life (Simpson and Brown, 2018). The current standard chemotherapy drugs are mainly methotrexate, doxorubicin, ifosfamide and cisplatin (Zhang et al., 2018). However, as the disease progresses and osteosarcoma becomes resistant to chemotherapy drugs, survival rates for osteosarcoma have remained largely unchanged over the past few decades (Yuan B. et al., 2023). Osteosarcoma often brings great distress to clinicians and patients due to factors such as difficult treatment, recurrence and metastasis, and poor prognosis (Li S. et al., 2023). The toxic effects of chemotherapy drugs and the poor ability to target osteosarcoma cells often make chemotherapy not significantly progress in the treatment of osteosarcoma (Shi et al., 2023). Metastasis and recurrence of OS often result in a poor prognosis, so the 5 year survival rate for patients with OS who have metastases or recurrences is only 10%–20% (Marina et al., 2004). Therefore, the development of new and effective treatment strategies to improve patient survival is a top priority in the treatment of osteosarcoma.
In recent years, biomaterials have been widely used in various fields of nanomedicine and regenerative medicine due to their biological histocompatibility, targeting, and degradability (Xia et al., 2023; Xia et al., 2022a). In oncology treatment, biomaterials have shown strong potential trends in recent years (Xia et al., 2022b). As a type of biomaterial, gelatin is an attractive material for drug delivery and tissue engineering due to its excellent biocompatibility and biodegradability (Zhai et al., 2023). Gelatin-based biomaterials have been reported in the treatment of tumors (Danışman-Kalındemirtaş et al., 2022). However, gelatin-based hydrogels have weaker mechanical properties and are less immunogenic compared to collagen and its precursors (Maji et al., 2018). In physical and chemical modification, gelatin has adjustable mechanical strength and biological activity (Tan et al., 2023). Improved gelatin materials have been widely used in the treatment of osteosarcoma. Gelatin-based biomaterials can be used as a delivery strategy to enhance the anti-tumor activity of osteosarcoma (Scheme 1). In this article, we introduce the application of gelatin-based biomaterials in the treatment of osteosarcoma and summarize and look forward to them.
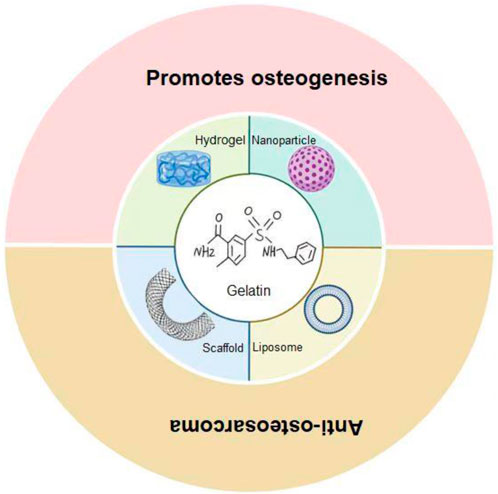
Scheme 1. Gelatin-based biomaterials as a delivery strategy for osteosarcoma treatment. Gelatin-based hydrogels, scaffolds, and nanoparticles/liposomes have demonstrated diverse functions in the treatment of osteosarcoma. Hydrogels release active substances, deliver drugs, antimicrobial and promote bone regeneration; The stent can provide physical support, promote bone growth, and load drugs to inhibit the tumor; Nanoparticles/liposomes are unique in drug delivery and enhanced therapeutic efficacy.
2 Gelatin properties
Gelatin is a denatured derivative of collagen containing a variety of functional amino acids, which has been widely used in tissue engineering due to its bioaffinity, excellent cell attachment, and low antigenicity (Su and Wang, 2015). Because it is derived from animal protein, it is non-toxic and degradable (Ahmed et al., 2020). Studies have shown that gelatin begins to denature above 40°C and loses its structural stability and hydrolyzes into different collagen fragments (Andreazza et al., 2023). What distinguishes gelatin from other natural peptides is the order in which amino acids are arranged in its branched chains, i.e., from the 17th amino acid residue, glycine (GLY) always occupies the third place (Hulmes et al., 1973). Therefore, after gelatin is degraded by proteases in vivo, it will increase cell adhesion and facilitate tissue regeneration (Echave et al., 2017). In addition, the porosity of gelatin ensures the diffusion of nutrients and oxygen, which promotes the normal growth of cells (Zhang et al., 2021). However, porosity is not directly proportional to cell survival. Studies have shown that irregular pores are shown to have lower cell viability (Seliktar, 2012). However, gelatin has poor mechanical stability, which limits its clinical application. Therefore, the combination of gelatin with other biomaterials to improve its stability is a commonly used improvement strategy (Lukin et al., 2022).
2.1 Hydrogel
Hydrogel is a hydrophilic macromolecular network, and hydrogel can fill various irregularly shaped defects for the treatment of osteosarcoma due to its softness (Li et al., 2019). The controllable and reversible swelling capacity of microgels with a diameter of 10 nm–100 μm can in turn improve drug delivery capabilities (Zhang et al., 2023). Reactive oxygen and nitrogen species (RONS) has recently been shown to have selective properties that kill cancer cells (Chauvin et al., 2017). Different tumor cells tolerate RONS differently, and studies have shown that injection of RONS around the tumor can promote osteosarcoma cell death (Van Boxem et al., 2017). Gelatin treated with cold atmospheric plasma is able to release RONS. Labay et al. gelatin treated by cold atmosphere plasma was used to deliver RONS to osteosarcoma (Labay et al., 2020). In vitro experiments confirmed that gelatin-released RONS could reduce osteosarcoma cell survival to 12%–23% after 72 h. In addition, gelatin can also inhibit the activity of MMP-2 and MMP-9 around tumors, thereby achieving anti-tumor effects (Hsu et al., 2019). Although gelatin has a certain anti-tumor effect, its effect is weak. Liu et al. prepared methacrylic acid gelatin/oxidized dextran/montmorillonite-strontium/polypyrrole (GOMP) hydrogels for the delivery of doxorubicin (DOX) (Liu et al., 2023b). The dual network structure of GOMP hydrogels formed by photoinitiator-initiated double bond polymerization and Schiff base reaction enables DOX to be payloaded and continuously released around the tumor. The compressive modulus of the GOMP@DOX hydrogel is 224.0 ± 40.2 kPa, which has good biocompatibility and biodegradability. In mice, it was confirmed that the GOMP@DOX hydrogel was fully degraded at 56 days. GOMP@DOX hydrogel can quickly release DOX within 12 h and reduce the toxic side effects of DOX. Compared with DOX, the hepatorenal toxicity of the GOMP@DOX hydrogel was reduced at 7 and 28 days in mice. In tumor-bearing mice, it was confirmed that GOMP@DOX hydrogel could effectively inhibit the proliferation and metastasis of osteosarcoma cells. Not only that, but GOMP@DOX hydrogels also provide mechanical support for bone regeneration. It not only improves the support capacity of the defective bone, but also provides the foundation for bone regeneration.
Giada Loi et al. developed a chitosan/gelatin hydrogel for the treatment of osteosarcoma using methylfuran group functionalization (Loi et al., 2023). Studies have shown that hydrogels can provide suitable compressive strength and crosslinking temperatures. After 14 days in the body, the hydrogel can be degraded into fragments, forming a negative interference with osteosarcoma cells. Not only that, the gelatin-based material will not cause necrosis of the surrounding tissues after degradation in vivo, indicating that its safety in vivo is satisfactory. Mojgan Ghanbari et al. developed a heat-sensitive injectable hydrogel that oxidizes alginate, gelatin, and carbon nitride quantum dots (CNQDs) (Ghanbari et al., 2021). Alginate hydrogels are a widely used biomaterial due to their biodegradability, biocompatibility, and ease of manufacturing (Balakrishnan et al., 2014). However, the poor adhesion of alginate limits its clinical application. Gelatin is combined with alginate to provide adhesion to alginate while increasing the rheological properties of the hydrogel. Not only that, the improved hydrogel enhances its mechanical strength, swelling rate, biodegradability and stability. Chen et al. used ALG/GelAGE to develop a hydrogel for the delivery of DOX and PDA particles (Chen et al., 2022). PDA can enhance the photothermal effect of hydrogels due to its excellent photothermal properties (Cheng et al., 2017). PDA particles have a diameter of about 200 nm and can be easily and uniformly dispersed in hydrogels compared to other photothermal agents. The hydrogel is porosity, and its porosity is 56.75% ± 0.64%. The DOX loading efficiency in PDA@DOX particles can be as high as 95%, which can fully release DOX to achieve anti-tumor effect. PDA@DOX particles can enhance the mechanical properties of hydrogels (compressive strength of 31.51 ± 0.84 KPa) and increase their stability. In vivo experiments confirmed that the modified hydrogel could promote apoptosis of tumor cells and reduce tumor volume in tumor-bearing mice.
After osteosarcoma resection, a large amount of bone is lost and cannot resist the attack of residual tumor cells and bacteria (Cha et al., 2023). Conventional bone implants do not have an antimicrobial effect and are not resistant to bacterial invasion (Ballatori and Hinds, 2016). Once an infection occurs, it poses severe pain and risk of amputation (Kadhim et al., 2022). Yin et al. developed an antimicrobial hydrogel using methacrylate gel (GelMA) and bioinert sulfonated polyetheretherketone (SP) (Yin et al., 2020). Upon the addition of tobramycin (TOB) to the hydrogel, the SP@MX-TOB/GelMA implant exhibits potent antimicrobial properties against gram-negative/gram-positive bacteria. Studies have shown that SP@MX-TOB/GelMA has good cytocompatibility and bone-promoting ability, and can also kill osteosarcoma cells and gram-negative/gram-positive bacteria. The addition of bone morphogenetic protein (BMP)-2 to the hydrogel can also enhance the osteogenic effect of the hydrogel (Yi et al., 2020).
Based on the premise that alginate can produce reactive oxygen species and nitrogen to enhance anti-tumor activity, Albert et al. prepared HA and alginate hydrogels for the treatment of osteosarcoma (Espona-Noguera et al., 2024). Hydrogels not only have the ability to promote cell proliferation or induce cell death, but also promote bone regeneration. Liu et al. fabricated a double-network hydrogel by photoinitiator-initiated double bond polymerization and Schiff base reaction (Liu et al., 2023a). This hydrogel is made of methacrylic acid gelatin/oxidized dextran/montmorillonite-strontium/polypyrrole (GOMP) as the main material. Polypyrrole can provide conductivity and high photothermal conversion ability for hydrogels under the premise of photothermal therapy, and the network structure of hydrogels can be used to deliver DOX to enhance its anti-tumor effect. The addition of strontium can safely achieve the ability of hydrogels to promote bone tissue regeneration.
2.2 Gelatin stents
Gelatin stents can be designed in different shapes to provide physical support at osteosarcoma defects, and their pores can facilitate bone ingrowth and accelerate bone repair (Moghaddam et al., 2024; Chrungoo et al., 2024). Chen et al. utilized a decellularized periosteal scaffold to load DOX-gelatin microspheres (Chen et al., 2019). The periosteal scaffold is the physicochemical removal of cellular components, showing excellent cytocompatibility and the ability to promote fibroblast adhesion and growth (Li et al., 2023b). DOX-gelatin microspheres can reduce the toxicity of DOX while maintaining its structural and functional stability. Local acellular scaffolds can locally deliver osteosarcoma chemotherapy drugs, reduce osteosarcoma cell proliferation and provide physical support for bone regeneration. To enhance bone regeneration, Adler et al. utilized silane-modified gelatin-polystyrene sulfonates to modify polydopamine (PDA) scaffolds to make PDA-coated stents (Adler et al., 2023). The PDA-coated scaffold has uniform porosity (122 ± 39 μm) that contributes to the formation of mineralized bone by osteoblast activity after implantation (Han et al., 2019). The compressive stress and Young’s modulus of PDA-coated stents were (0.7 ± 0.3 MPa) and (57 ± 24 Mpa), respectively, and they were stable in cancellous bone. PDA-coated scaffolds are stable and can be degraded by 15% in 4 weeks in vivo. In addition, the PDA-coated scaffold can enhance the properties of the hydroxyapatite layer in vivo and promote the proliferation and differentiation of osteoblasts. Ghorbani et al. modified PDA scaffolds using alginate and gelatin (Ghorbani et al., 2022). Compared with the PDA stent, the improved PDA stent was more stable (compressive strength of 0.69 ± 0.02 MPa). The surface of the PDA scaffold has a porous structure (1.37 ± 0.12 mm), which can be loaded with osteocalcin and osteomodulin, which can promote biomineralization and accelerate osteoinduced regeneration. In addition, the PDA-coated ADA-GEL scaffold supports osteosarcoma cell adhesion and proliferation.
Titanium is commonly used in orthopedic and dental implants due to its excellent mechanical properties, chemical resistance, and biocompatibility (Elias et al., 2015). Studies have shown that Ti scaffolds with pore sizes between 350 and 1,500 μm can achieve stiffness similar to that of human bone (Nune et al., 2018). However, titanium, as an implant, is not biologically active and lacks osseointegration capacity (Jiang et al., 2018). Cai et al. designed and developed the 3D-printed Ti6Al4V lattice scaffold with hydrothermally induced, photothermal conversion TiO2/TiP coating (HR-Ti) (Cai et al., 2021). The scaffold is also infused with gelatin/hydroxyapatite nanocomposites to provide the basis for cell adhesion/bone regeneration. The gelatin/hydroxyapatite nanocomposites have an average pore size of 7.02 nm, which provides good space for drug delivery. The pore size of the 3D-printed gel scaffold is 50–600 μm, and its porous structure facilitates the ingrowth of bone tissue. The compressive strength of the stent is 17.8 ± 1.6 Mpa, and the elastic modulus is 8.8 ± 0.2 GPa. Stent loaded drugs are released within 2 days with >90% release and 28 days for complete release. In vitro experiments have demonstrated that hydrogel scaffolds can promote osseointegration and anti-tumor effects at 12 weeks after surgery.
After surgical removal of osteosarcoma, postoperative bacterial infection triggers an inflammatory response and hinders the bone repair process. Therefore, local antimicrobial is also an important method for surgical resection of osteosarcoma. Huang et al. used N-acryloylglycanamide (NAGA)/methacrylic acid gelatin (Gel-MA) to make multifunctional hydrogels (GMNGs) (Huang et al., 2023). The combination of NAGA and Gel-MA endows GMNG with mechanical properties and controllable degradation ability, and GMNG has excellent superior tumor cell and antimicrobial ability. GMNG can effectively promote the formation of new bone in the body.
2.3 Nanoparticles/liposomes
Hydroxyapatite nanoparticles are widely used in orthopedic grafts due to their high surface-to-volume ratio, strong absorption capacity and excellent biocompatibility (Xia et al., 2022c). Liu et al. fabricated nanoparticles (DOX@nHAp NP) for DOX delivery using hydroxyapatite nanoparticles, gelatin, and polylactic acid fiber membranes (Liu et al., 2023b). DOX@nHAp NPs have an average size of 188.7 nm and have a 40% load efficiency for DOX. Polylactic acid membranes are able to give nanoparticles a stretching effect. DOX@nHAp NPs remain degraded in vivo for 20 days and continue to release DOX into the tumor microenvironment. DOX@nHAp NPs not only reduce the hepatorenal toxicity of DOX, but also promote osteoblast bone mineralization and enhance bone regeneration. Liao et al. developed a long-space nanoparticle for the treatment of osteosarcoma using gelatin methacrylate/chondroitin methacrylate, chondroitin sulfate, hydrogels, hybrid gold nanorods (GNRs) and nanohydroxyapatite (nHA) (Liao et al., 2021). Nanoparticles have good cytocompatibility and can enhance the photothermal therapy effect of osteosarcoma in vivo. Under light conditions, nanoparticles can penetrate tumor cell membranes faster without affecting surrounding tissues and cells. In vivo experiments have shown that nanoparticles can delay the growth of osteosarcoma cells and reduce their lung metastasis rate. The addition of nHA enhanced the bone mineralization capacity of the nanoparticles, which produced 65% more mineralized extracellular matrix (ECM) than the control group. Teotia et al. incorporated osteosarcoma cell-derived bone morphogenetic protein (BMP) and bisphosphonate (zoledronic acid) into gelatin cement to make gelatin biomaterials that promote cell proliferation and bone regeneration (Teotia et al., 2016). The particle size of gelatin cement is less than 40 μm, and the compressive strength is 6.9 ± 0.5 Mpa. Around tumors, the release of BMP from gelatin cement promotes bone regeneration. In vivo, gelatin cement promoted osteoblast adhesion on day 28 and detected Runt-related transcription factor-2 (RUNX2); collagen type I (COLI); bone sialoprotein (BSP); osteocalcin (OCN); Expression of glyceraldehyde-3-phosphate dehydrogenase (GAPDH). Within 16 weeks, it can promote trabecular bone regeneration.
The rapid degradation properties of gelatin are enhanced by the formation of gelatin modified by photo- or chemically induced polymerization of methacrylates (Yue et al., 2015). GelMA is widely used in biomedicine due to its biodegradability and variable physical properties (Xin et al., 2017). Wu et al. used GelMA to make nanoliposomes for the treatment of osteosarcoma (Wu et al., 2018). The average diameter of GelMA nanoliposomes is 119.6 ± 2.3 nm, and the compressive strength is 30.82 ± 1.49 N. Studies have shown that GelMA nanoliposomes are fully degraded in vivo for 21 days, and gemcitabine can be released (94.97% ± 2.20%) after 100 h in vivo. In an in vivo tumor-bearing mouse model, the growth of GelMA nanoliposome-treated mouse tumors at 14 days was significantly lower than that of the control group. Although this experiment did not verify whether GelMA nanoliposomes have sufficient compressive strength and support around osteosarcoma. However, in another study, the 3D-printed GelMA hydrogel can provide a solid foundation for bone regeneration, and the addition of angiogenesis factor and matrix metalloproteinase to the hydrogel can prevent mechanistic signaling and promote angiogenesis (Lin et al., 2023). However, it is important to note that an elevated MMP2/MMP9 activity ratio is associated with a poor response to chemotherapy in osteosarcoma (Kunz et al., 2016).
Nanoparticles alone cannot achieve on-demand and local delivery strategies (Patenaude and Hoare, 2012). Jalili et al. utilized GelMA, magnetic nanoparticles (MNPs), and poly (N-isopropylacrylamide-co-acrylamide) (poly (NIPAM-co-AM)) to make heat-responsive nanohydrogels for DOX delivery (Jalili et al., 2017). The loading rate of 150 nm of magnetic nanoparticle nanoparticles to DOX is 42.1% ± 8.1%. In vivo experiments have shown that within 24 h, magnetic nanoparticles can release 75.1% of DOX for chemotherapy for osteosarcoma. Within 48 h, tumor cell death can be significantly caused. Lin et al. synthesized GelMA for carrying photoinitiators to enhance antitumor activity (Lin et al., 2016). Studies have shown that glutathione can scavenge oxidative free radicals and protect cells in a viable state (Monostori et al., 2009). Albert et al. developed alginate-based hydrogels for the enhancement of peritumoral plasma-derived ROS and reactive nitrogen (Espona-Noguera et al., 2024). Low levels of reactive oxygen species and reactive nitrogen stimulate cell proliferation, while high concentrations of reactive oxygen species and reactive nitrogen are harmful (Privat-Maldonado et al., 2019). High doses of reactive oxygen species and reactive nitrogen have been shown to trigger cell death, endogenous RONS production, DNA damage, and lipid peroxidation in cancer cells (Malyavko et al., 2020). Alginate hydrogel solves the shortcomings of rapid and poorly controlled delivery of reactive oxygen species and reactive nitrogen that are easily diluted by body fluids, and can release a large amount of reactive oxygen species and reactive nitrogen in the local tumor. In tumor-bearing mice for 3 weeks, it was found that alginate hydrogel could greatly reduce tumor volume.
3 Conclusion
Osteosarcoma seriously affects the quality of life of adolescents. At present, there is no good clinical way to deal with the metastasis of osteosarcoma and the complications caused by surgery. Biomaterials have developed rapidly in tissue engineering and regenerative medicine in recent years, and have gradually become a novel modality in tumor treatment. As one of the important members of biomaterials, gelatin has good biocompatibility and has made phased progress in tumor treatment. The properties of gelatin make it an ideal biomaterial base, and the hydrogels, scaffolds, and nanoparticles/liposomes developed based on them have demonstrated a variety of functions in the treatment of osteosarcoma. Hydrogels release active substances, deliver drugs, antimicrobial and promote bone regeneration; The stent can provide physical support, promote bone growth, and load drugs to inhibit the tumor; Nanoparticles/liposomes are unique in drug delivery and enhanced therapeutic efficacy.
At present, its clinical application is rarely reported, but preclinical studies have demonstrated its potential. In the future, it is necessary to deeply explore the mechanism of action in vivo, compare and analyze different materials to optimize selection, strengthen standardized production and quality control, carry out multi-center clinical studies to accumulate data, and explore combination with existing therapies and personalized treatment strategies, and comprehensively consider cost-effectiveness. With the deepening of research, gelatin-based biomaterials are expected to break through the existing dilemma, bring a new dawn for the treatment of osteosarcoma, and significantly improve the prognosis and quality of life of patients.
Author contributions
QY: Writing–original draft, Writing–review and editing. XC: Methodology, Validation, Writing–original draft. JL: Validation, Writing–review and editing. YH: Resources, Supervision, Writing–review and editing.
Funding
The author(s) declare that no financial support was received for the research, authorship, and/or publication of this article.
Acknowledgments
We would like to express our appreciation to everyone involved in drafting and preparing the manuscript.
Conflict of interest
The authors declare that the research was conducted without any commercial or financial relationships that could be construed as a potential conflict of interest.
Generative AI statement
The author(s) declare that no Generative AI was used in the creation of this manuscript.
Publisher’s note
All claims expressed in this article are solely those of the authors and do not necessarily represent those of their affiliated organizations, or those of the publisher, the editors and the reviewers. Any product that may be evaluated in this article, or claim that may be made by its manufacturer, is not guaranteed or endorsed by the publisher.
References
Adler, C., Monavari, M., Abraham, G. A., Boccaccini, A. R., and Ghorbani, F. (2023). Mussel-inspired polydopamine decorated silane modified-electroconductive gelatin-PEDOT:PSS scaffolds for bone regeneration. RSC Adv. 13, 15960–15974. doi:10.1039/d3ra01311a
Ahmed, M. A., Al-Kahtani, H. A., Jaswir, I., AbuTarboush, H., and Ismail, E. A. (2020). Extraction and characterization of gelatin from camel skin (potential halal gelatin) and production of gelatin nanoparticles. Saudi J. Biol. Sci. 27, 1596–1601. doi:10.1016/j.sjbs.2020.03.022
Andreazza, R., Morales, A., Pieniz, S., and Labidi, J. (2023). Gelatin-based hydrogels: potential biomaterials for remediation. Polymers 15, 1026. doi:10.3390/polym15041026
Balakrishnan, B., Joshi, N., Jayakrishnan, A., and Banerjee, R. (2014). Self-crosslinked oxidized alginate/gelatin hydrogel as injectable, adhesive biomimetic scaffolds for cartilage regeneration. Acta biomater. 10, 3650–3663. doi:10.1016/j.actbio.2014.04.031
Ballatori, S. E., and Hinds, P. W. (2016). Osteosarcoma: prognosis plateau warrants retinoblastoma pathway targeted therapy. Signal Transduct. Target. Ther. 1, 16001. doi:10.1038/sigtrans.2016.1
Beird, H. C., Bielack, S. S., Flanagan, A. M., Gill, J., Heymann, D., Janeway, K. A., et al. (2022). Osteosarcoma. Nat. Rev. Dis. Prim. 8, 77. doi:10.1038/s41572-022-00409-y
Bian, J., Liu, Y., Zhao, X., Meng, C., Zhang, Y., Duan, Y., et al. (2023). Research progress in the mechanism and treatment of osteosarcoma. Chin. Med. J. 136, 2412–2420. doi:10.1097/cm9.0000000000002800
Cai, B., Huang, L., Wang, J., Sun, D., Zhu, C., Huang, Y., et al. (2021). 3D printed multifunctional Ti6Al4V-based hybrid scaffold for the management of osteosarcoma. Bioconjugate Chem. 32, 2184–2194. doi:10.1021/acs.bioconjchem.1c00367
Cha, H. S., Lee, H. K., Park, S. H., and Nam, M. J. (2023). Acetylshikonin induces apoptosis of human osteosarcoma U2OS cells by triggering ROS-dependent multiple signal pathways. Toxicol. In Vitro 86, 105521. doi:10.1016/j.tiv.2022.105521
Chauvin, J., Judée, F., Yousfi, M., Vicendo, P., and Merbahi, N. (2017). Analysis of reactive oxygen and nitrogen species generated in three liquid media by low temperature helium plasma jet. Sci. Rep. 7, 4562. doi:10.1038/s41598-017-04650-4
Chen, C., Dong, J., Chen, H., Wang, X., Mei, J., Wang, L., et al. (2019). Preparation of adriamycin gelatin microsphere-loaded decellularized periosteum that is cytotoxic to human osteosarcoma cells. J. Cell Physiol. 234, 10771–10781. doi:10.1002/jcp.27753
Chen, S., Wang, Y., Zhang, X., Ma, J., and Wang, M. (2022). Double-crosslinked bifunctional hydrogels with encapsulated anti-cancer drug for bone tumor cell ablation and bone tissue regeneration. Colloids Surfaces B Biointerfaces 213, 112364. doi:10.1016/j.colsurfb.2022.112364
Cheng, W., Nie, J., Xu, L., Liang, C., Peng, Y., Liu, G., et al. (2017). pH-sensitive delivery vehicle based on folic acid-conjugated polydopamine-modified mesoporous silica nanoparticles for targeted cancer therapy. ACS Appl. Mater. and interfaces 9, 18462–18473. doi:10.1021/acsami.7b02457
Chrungoo, S., Bharadwaj, T., and Verma, D. (2024). Nanofibrous polyelectrolyte complex incorporated BSA-alginate composite bioink for 3D bioprinting of bone mimicking constructs. Int. J. Biol. Macromol. 266, 131123. doi:10.1016/j.ijbiomac.2024.131123
Danışman-Kalındemirtaş, F., Kariper İ, A., Erdemir, G., Sert, E., and Erdem-Kuruca, S. (2022). Evaluation of anticancer effects of carboplatin-gelatin nanoparticles in different sizes synthesized with newly self-assembly method by exposure to IR light. Sci. Rep. 12, 10686. doi:10.1038/s41598-022-15051-7
Dong, Z., Liao, Z., He, Y., Wu, C., Meng, Z., Qin, B., et al. (2022). Advances in the biological functions and mechanisms of miRNAs in the development of osteosarcoma. Technol. Cancer Res. and Treat. 21, 15330338221117386. doi:10.1177/15330338221117386
Echave, M. C., Sánchez, P., Pedraz, J. L., and Orive, G. (2017). Progress of gelatin-based 3D approaches for bone regeneration. J. Drug Deliv. Sci. Technol. 42, 63–74. doi:10.1016/j.jddst.2017.04.012
Elias, C. N., Fernandes, D. J., Resende, C. R. S., and Roestel, J. (2015). Mechanical properties, surface morphology and stability of a modified commercially pure high strength titanium alloy for dental implants. Dent. Mater. 31, e1–e13. doi:10.1016/j.dental.2014.10.002
Espona-Noguera, A., Tampieri, F., and Canal, C. (2024). Engineering alginate-based injectable hydrogels combined with bioactive polymers for targeted plasma-derived oxidative stress delivery in osteosarcoma therapy. Int. J. Biol. Macromol. 257, 128841. doi:10.1016/j.ijbiomac.2023.128841
Ghanbari, M., Salavati-Niasari, M., and Mohandes, F. (2021). Injectable hydrogels based on oxidized alginate-gelatin reinforced by carbon nitride quantum dots for tissue engineering. Int. J. Pharm. 602, 120660. doi:10.1016/j.ijpharm.2021.120660
Ghorbani, F., Kim, M., Monavari, M., Ghalandari, B., and Boccaccini, A. R. (2022). Mussel-inspired polydopamine decorated alginate dialdehyde-gelatin 3D printed scaffolds for bone tissue engineering application. Front. Bioeng. Biotechnol. 10, 940070. doi:10.3389/fbioe.2022.940070
Han, L., Jiang, Y., Lv, C., Gan, D., Wang, K., Ge, X., et al. (2019). Mussel-inspired hybrid coating functionalized porous hydroxyapatite scaffolds for bone tissue regeneration. Colloids surfaces. B, Biointerfaces 179, 470–478. doi:10.1016/j.colsurfb.2019.04.024
Hsu, M. J., Peng, S. F., Chueh, F. S., Tsai, C. H., Tsai, F. J., Huang, C. Y., et al. (2019). Lupeol suppresses migration and invasion via p38/MAPK and PI3K/Akt signaling pathways in human osteosarcoma U-2 OS cells. Biosci. Biotechnol. Biochem. 83, 1729–1739. doi:10.1080/09168451.2019.1606693
Huang, Y., Zhai, X., Ma, T., Zhang, M., Yang, H., Zhang, S., et al. (2023). A unified therapeutic-prophylactic tissue-engineering scaffold demonstrated to prevent tumor recurrence and overcoming infection toward bone remodeling. Adv. Mater. (Deerfield Beach, Fla.) 35, e2300313. doi:10.1002/adma.202300313
Hulmes, D. J. S., Miller, A., Parry, D. A. D., Piez, K. A., and Woodhead-Galloway, J. (1973). Analysis of the primary structure of collagen for the origins of molecular packing. J. Mol. Biol. 79, 137–148. doi:10.1016/0022-2836(73)90275-1
Jalili, N. A., Jaiswal, M. K., Peak, C. W., Cross, L. M., and Gaharwar, A. K. (2017). Injectable nanoengineered stimuli-responsive hydrogels for on-demand and localized therapeutic delivery. Nanoscale 9, 15379–15389. doi:10.1039/c7nr02327h
Jiang, N., Guo, Z., Sun, D., Li, Y., Yang, Y., Chen, C., et al. (2018). Promoting osseointegration of Ti implants through micro/nanoscaled hierarchical Ti phosphate/Ti oxide hybrid coating. ACS Nano 12, 7883–7891. doi:10.1021/acsnano.8b02227
Jiang, Y., and He, K. (2023). Nanobiotechnological approaches in osteosarcoma therapy: versatile (nano) platforms for theranostic applications. Environ. Res. 229, 115939. doi:10.1016/j.envres.2023.115939
Kadhim, M. M., Bokov, D. O., Ansari, M. J., Suksatan, W., Jawad, M. A., Chupradit, S., et al. (2022). Bone morphogenetic protein (BMP)-modified graphene oxide-reinforced polycaprolactone-gelatin nanofiber scaffolds for application in bone tissue engineering. Bioprocess Biosyst. Eng. 45, 981–997. doi:10.1007/s00449-022-02717-9
Kunz, P., Sähr, H., Lehner, B., Fischer, C., Seebach, E., and Fellenberg, J. (2016). Elevated ratio of MMP2/MMP9 activity is associated with poor response to chemotherapy in osteosarcoma. BMC Cancer 16, 223. doi:10.1186/s12885-016-2266-5
Labay, C., Roldán, M., Tampieri, F., Stancampiano, A., Bocanegra, P. E., Ginebra, M.-P., et al. (2020). Enhanced generation of reactive species by cold plasma in gelatin solutions for selective cancer cell death. ACS Appl. Mater. and interfaces 12, 47256–47269. doi:10.1021/acsami.0c12930
Le Nail, L.-R., Brennan, M., Rosset, P., Deschaseaux, F., Piloquet, P., Pichon, O., et al. (2018). Comparison of tumor- and bone marrow-derived mesenchymal stromal/stem cells from patients with high-grade osteosarcoma. Int. J. Mol. Sci. 19, 707. doi:10.3390/ijms19030707
Li, J., He, D., Hu, L., Li, S., Zhang, C., Yin, X., et al. (2023b). Decellularized periosteum promotes guided bone regeneration via manipulation of macrophage polarization. Biotechnol. J. 18, e2300094. doi:10.1002/biot.202300094
Li, S., Zhang, H., Liu, J., and Shang, G. (2023a). Targeted therapy for osteosarcoma: a review. J. cancer Res. Clin. Oncol. 149, 6785–6797. doi:10.1007/s00432-023-04614-4
Li, T., Li, L., Wu, X., Tian, K., and Wang, Y. (2019). The oncogenic role of GNL3 in the progression and metastasis of osteosarcoma. Cancer Manag. Res. 11, 2179–2188. doi:10.2147/cmar.S195360
Liao, J., Shi, K., Jia, Y., Wu, Y., and Qian, Z. (2021). Gold nanorods and nanohydroxyapatite hybrid hydrogel for preventing bone tumor recurrence via postoperative photothermal therapy and bone regeneration promotion. Bioact. Mater. 6, 2221–2230. doi:10.1016/j.bioactmat.2021.01.006
Lin, C. H., Lin, K. F., Mar, K., Lee, S. Y., and Lin, Y. M. (2016). Antioxidant N-acetylcysteine and glutathione increase the viability and proliferation of MG63 cells encapsulated in the gelatin methacrylate/VA-086/blue light hydrogel system. Tissue Eng. Part C Methods 22, 792–800. doi:10.1089/ten.TEC.2016.0025
Lin, Y., Yuan, K., Yang, Y., Yang, S., Huang, K., Yu, Z., et al. (2023). Osteosarcoma progression in biomimetic matrix with different stiffness: insights from a three-dimensional printed gelatin methacrylamide hydrogel. Int. J. Biol. Macromol. 252, 126391. doi:10.1016/j.ijbiomac.2023.126391
Liu, J., Lin, S., Dang, J., Wang, S., Cheng, W., Ran, Z., et al. (2023a). Anticancer and bone-enhanced nano-hydroxyapatite/gelatin/polylactic acid fibrous membrane with dual drug delivery and sequential release for osteosarcoma. Int. J. Biol. Macromol. 240, 124406. doi:10.1016/j.ijbiomac.2023.124406
Liu, X., Zhang, Y., Wu, H., Tang, J., Zhou, J., Zhao, J., et al. (2023b). A conductive gelatin methacrylamide hydrogel for synergistic therapy of osteosarcoma and potential bone regeneration. Int. J. Biol. Macromol. 228, 111–122. doi:10.1016/j.ijbiomac.2022.12.185
Loi, G., Stucchi, G., Scocozza, F., Cansolino, L., Cadamuro, F., Delgrosso, E., et al. (2023). Characterization of a bioink combining extracellular matrix-like hydrogel with osteosarcoma cells: preliminary results. Gels (Basel, Switzerland) 9, 129. doi:10.3390/gels9020129
Lukin, I., Erezuma, I., Maeso, L., Zarate, J., Desimone, M. F., Al-Tel, T. H., et al. (2022). Progress in gelatin as biomaterial for tissue engineering. Pharmaceutics 14, 1177. doi:10.3390/pharmaceutics14061177
Maji, S., Agarwal, T., Das, J., and Maiti, T. K. (2018). Development of gelatin/carboxymethyl chitosan/nano-hydroxyapatite composite 3D macroporous scaffold for bone tissue engineering applications. Carbohydr. Polym. 189, 115–125. doi:10.1016/j.carbpol.2018.01.104
Malyavko, A., Yan, D., Wang, Q., Klein, A. L., Patel, K. C., Sherman, J. H., et al. (2020). Cold atmospheric plasma cancer treatment, direct versus indirect approaches. Mater. Adv. 1, 1494–1505. doi:10.1039/D0MA00329H
Marina, N., Gebhardt, M., Teot, L., and Gorlick, R. (2004). Biology and therapeutic advances for pediatric osteosarcoma. Oncol. 9, 422–441. doi:10.1634/theoncologist.9-4-422
Mirabello, L., Troisi, R. J., and Savage, S. A. (2009). Osteosarcoma incidence and survival rates from 1973 to 2004: data from the surveillance, epidemiology, and end results program. Cancer 115, 1531–1543. doi:10.1002/cncr.24121
Moghaddam, M. M., Jooybar, E., Imani, R., and Ehrbar, M. (2024). Development of injectable microgel-based scaffolds via enzymatic cross-linking of hyaluronic acid-tyramine/gelatin-tyramine for potential bone tissue engineering. Int. J. Biol. Macromol. 279, 135176. doi:10.1016/j.ijbiomac.2024.135176
Monostori, P., Wittmann, G., Karg, E., and Túri, S. (2009). Determination of glutathione and glutathione disulfide in biological samples: an in-depth review. J. Chromatogr. B Anal. Technol. Biomed. Life Sci. 877, 3331–3346. doi:10.1016/j.jchromb.2009.06.016
Ning, B., Liu, Y., Huang, T., and Wei, Y. (2023). Autophagy and its role in osteosarcoma. Cancer Med. 12, 5676–5687. doi:10.1002/cam4.5407
Nune, K. C., Li, S., and Misra, R. D. K. (2018). Advancements in three-dimensional titanium alloy mesh scaffolds fabricated by electron beam melting for biomedical devices: mechanical and biological aspects. Sci. China Mater. 61, 455–474. doi:10.1007/s40843-017-9134-x
Patenaude, M., and Hoare, T. (2012). Injectable, degradable thermoresponsive poly (N-isopropylacrylamide) hydrogels. ACS Macro Lett. 1, 409–413. doi:10.1021/mz200121k
Privat-Maldonado, A., Schmidt, A., Lin, A., Weltmann, K. D., Wende, K., Bogaerts, A., et al. (2019). ROS from physical plasmas: redox chemistry for biomedical therapy. Oxid. Med. Cell Longev. 2019, 9062098. doi:10.1155/2019/9062098
Seliktar, D. (2012). Designing cell-compatible hydrogels for biomedical applications. Sci. (New York, N.Y.) 336, 1124–1128. doi:10.1126/science.1214804
Shi, P., Cheng, Z., Zhao, K., Chen, Y., Zhang, A., Gan, W., et al. (2023). Active targeting schemes for nano-drug delivery systems in osteosarcoma therapeutics. J. nanobiotechnology 21, 103. doi:10.1186/s12951-023-01826-1
Simpson, E., and Brown, H. L. (2018). Understanding osteosarcomas. JAAPA 31, 15–19. doi:10.1097/01.jaa.0000541477.24116.8d
Su, K., and Wang, C. (2015). Recent advances in the use of gelatin in biomedical research. Biotechnol. Lett. 37, 2139–2145. doi:10.1007/s10529-015-1907-0
Tan, Y., Zi, Y., Peng, J., Shi, C., Zheng, Y., and Zhong, J. (2023). Gelatin as a bioactive nanodelivery system for functional food applications. Food Chem. 423, 136265. doi:10.1016/j.foodchem.2023.136265
Teotia, A. K., Gupta, A., Raina, D. B., Lidgren, L., and Kumar, A. (2016). Gelatin-modified bone substitute with bioactive molecules enhance cellular interactions and bone regeneration. ACS Appl. Mater. and interfaces 8, 10775–10787. doi:10.1021/acsami.6b02145
Van Boxem, W., Van der Paal, J., Gorbanev, Y., Vanuytsel, S., Smits, E., Dewilde, S., et al. (2017). Anti-cancer capacity of plasma-treated PBS: effect of chemical composition on cancer cell cytotoxicity. Sci. Rep. 7, 16478. doi:10.1038/s41598-017-16758-8
Wu, W., Dai, Y., Liu, H., Cheng, R., Ni, Q., Ye, T., et al. (2018). Local release of gemcitabine via in situ UV-crosslinked lipid-strengthened hydrogel for inhibiting osteosarcoma. Drug Deliv. 25, 1642–1651. doi:10.1080/10717544.2018.1497105
Xia, Y., Wang, H., Yang, R., Hou, Y., Li, Y., Zhu, J., et al. (2023). Biomaterials delivery strategies to repair degenerated intervertebral discs by regulating the inflammatory microenvironment. Front. Immunol. 14, 1051606. doi:10.3389/fimmu.2023.1051606
Xia, Y., Yang, R., Hou, Y., Wang, H., Li, Y., Zhu, J., et al. (2022c). Application of mesenchymal stem cell-derived exosomes from different sources in intervertebral disc degeneration. Front. Bioeng. Biotechnol. 10, 1019437. doi:10.3389/fbioe.2022.1019437
Xia, Y., Yang, R., Wang, H., Hou, Y., Li, Y., Zhu, J., et al. (2022a). Biomaterials delivery strategies to repair spinal cord injury by modulating macrophage phenotypes. J. tissue Eng. 13, 20417314221143059. doi:10.1177/20417314221143059
Xia, Y., Yang, R., Zhu, J., Wang, H., Li, Y., Fan, J., et al. (2022b). Engineered nanomaterials trigger abscopal effect in immunotherapy of metastatic cancers. Front. Bioeng. Biotechnol. 10, 890257. doi:10.3389/fbioe.2022.890257
Xin, T., Gu, Y., Cheng, R., Tang, J., Sun, Z., Cui, W., et al. (2017). Inorganic strengthened hydrogel membrane as regenerative periosteum. ACS Appl. Mater. and interfaces 9, 41168–41180. doi:10.1021/acsami.7b13167
Yang, C., Tian, Y., Zhao, F., Chen, Z., Su, P., Li, Y., et al. (2020). Bone microenvironment and osteosarcoma metastasis. Int. J. Mol. Sci. 21, 6985. doi:10.3390/ijms21196985
Yi, M. H., Lee, J. E., Kim, C. B., Lee, K. W., and Lee, K. H. (2020). Locally controlled diffusive release of bone morphogenetic protein-2 using micropatterned gelatin methacrylate hydrogel carriers. Biochip J. 14, 405–420. doi:10.1007/s13206-020-4411-0
Yin, J., Han, Q., Zhang, J., Liu, Y., Gan, X., Xie, K., et al. (2020). MXene-based hydrogels endow polyetheretherketone with effective osteogenicity and combined treatment of osteosarcoma and bacterial infection. ACS Appl. Mater. and interfaces 12, 45891–45903. doi:10.1021/acsami.0c14752
Yuan, B., Shi, K., Zha, J., Cai, Y., Gu, Y., Huang, K., et al. (2023b). Nuclear receptor modulators inhibit osteosarcoma cell proliferation and tumour growth by regulating the mTOR signaling pathway. Cell death and Dis. 14, 51. doi:10.1038/s41419-022-05545-7
Yuan, P., Min, Y., and Zhao, Z. (2023a). Multifunctional nanoparticles for the treatment and diagnosis of osteosarcoma. Biomater. Adv. 151, 213466. doi:10.1016/j.bioadv.2023.213466
Yue, K., Trujillo-de Santiago, G., Alvarez, M. M., Tamayol, A., Annabi, N., and Khademhosseini, A. (2015). Synthesis, properties, and biomedical applications of gelatin methacryloyl (GelMA) hydrogels. Biomaterials 73, 254–271. doi:10.1016/j.biomaterials.2015.08.045
Zhai, X., Wu, Y., and Tan, H. (2023). Gelatin-based targeted delivery systems for tissue engineering. Curr. drug targets 24, 673–687. doi:10.2174/1389450124666230605150303
Zhang, H., Wu, S., Chen, W., Hu, Y., Geng, Z., and Su, J. (2023). Bone/cartilage targeted hydrogel: strategies and applications. Bioact. Mater. 23, 156–169. doi:10.1016/j.bioactmat.2022.10.028
Zhang, Y., Yang, J., Zhao, N., Wang, C., Kamar, S., Zhou, Y., et al. (2018). Progress in the chemotherapeutic treatment of osteosarcoma. Oncol. Lett. 16, 6228–6237. doi:10.3892/ol.2018.9434
Keywords: osteosarcoma, gelatin, osteoblasts, osteoclasts, bone defects
Citation: Yang Q, Chen X, Liu J and He Y (2025) Gelatin-based biomaterials as a delivery strategy for osteosarcoma treatment. Front. Pharmacol. 16:1537695. doi: 10.3389/fphar.2025.1537695
Received: 01 December 2024; Accepted: 13 January 2025;
Published: 28 January 2025.
Edited by:
Zhiyu Zhang, Fourth Affiliated Hospital of China Medical University, ChinaReviewed by:
Duoyi Zhao, Fourth Affiliated Hospital of China Medical University, ChinaDaqian Wan, Tongji University, China
Copyright © 2025 Yang, Chen, Liu and He. This is an open-access article distributed under the terms of the Creative Commons Attribution License (CC BY). The use, distribution or reproduction in other forums is permitted, provided the original author(s) and the copyright owner(s) are credited and that the original publication in this journal is cited, in accordance with accepted academic practice. No use, distribution or reproduction is permitted which does not comply with these terms.
*Correspondence: Yeteng He, aHl0ZW5nNTcyOThAMTYzLmNvbQ==