- Clinical Laboratory of China-Japan Union Hospital, Jilin University, Changchun, China
The liver performs crucial roles in energy metabolism, detoxification, and immune regulation. Hepatic diseases, including hepatitis, liver fibrosis, and liver cancer, have posed a significant threat to global health, emphasizing the critical need for the development of novel and effective treatment approaches. Nanotechnology, an emerging technology, has been extensively researched in medicine. Among the many types of nanomaterials, polymeric nanoparticles (NPs) are widely used in drug delivery systems. Compared to traditional therapies, they offer significant advantages in the treatment of liver disease by improving outcomes and reducing side effects. This review introduced the development of liver disease and discussed the application of natural polymers and synthetic polymers in their management. Furthermore, this paper reviewed the application of polymeric nanoparticles -mainly chitosan (CS), hyaluronic acid (HA), polyethylene glycol (PEG) and poly (lactic-co-glycolic acid) (PLGA)-in liver disease treatment, focusing on their use in various delivery systems for pure bioactive compounds of natural origin, drugs, nucleic acids, peptides, and others. Finally, the challenges and future perspectives of the NPs were discussed to provide guidance for further research directions, with the aim of promoting the clinical application of nanotherapeutics in treating hepatic diseases.
1 Introduction
The liver is one of the largest organs in the human body, playing crucial roles in energy metabolism, detoxification, and immune regulation (Nicole et al., 2019). Certain chemicals, drugs, food, and various infections (bacterial, fungal, or viral) might cause hepatic diseases such as hepatitis, liver fibrosis and liver cancer (Aydın and Akçalı, 2018; Sumeet et al., 2019; De Mattos et al., 2024). Hepatitis is characterized by widespread or patchy necrosis in liver inflammation, comprising acute and chronic forms. Acute hepatitis typically resolves spontaneously, while chronic hepatitis is characterized by persistent liver inflammation and hepatocellular necrosis lasting for at least 6 months, typically caused by hepatitis viruses. Hepatitis viruses primarily replicate in the liver, causing hepatocyte necrosis, oxidative stress, inflammatory responses, and immune activation. This triggers a cycle of liver cells injury and repair, alongside the activation of hepatic stellate cells (HSCs) and fibroblasts, which secrete collagen, cytokines, pro-fibrotic factors, and other extracellular matrix components, promoting excessive fibrosis. Severe fibrosis could lead to cirrhosis, liver cancer, and even death (Pei et al., 2023). Clinical treatment methods for hepatic diseases are diverse, including surgical interventions (hepatectomy and liver transplantation), ablation therapy, chemotherapy, radiotherapy, antiviral therapy, and immunotherapy. Despite significant advancements in the clinic, some challenges hinder the effective use of current hepatic disease treatments, such as a shortage of donor organs for liver transplants (Tapper and Parikh, 2023). Furthermore, some drugs are constrained in their therapeutic potential by factors such as limited distribution and bioavailability, low solubility, as well as potential toxicity and adverse effects (Yang et al., 2022). Nanomedicines could improve therapeutic efficacy and reduce adverse effects of drugs (e.g., pure bioactive compounds of natural origin, chemotherapeutic drugs, and nucleic acid-based medicines) as new drug delivery systems (DDS), thus providing a new paradigm to address the above challenges.
The explosive advancement of nanotechnology over the past few decades was significantly impacting the field of medicine. Nanomaterials have obvious advantages not only in improving pharmacokinetics, prolonging blood circulation time and reducing drug toxicity, but also in targeting drug delivery, slowing drug release, and improving drug solubility. Many nanomaterials and micromaterials, such as polymeric nanoparticles, polymeric micelles, inorganic nanoparticles, dendrimers, nanogels and solid lipid nanoparticles have been explored in hepatic diseases treatment (Paolo and Pietro, 2019). Their delivery methods are usually categorized as passive targeting and active targeting. Passive targeting utilized the properties of nanoparticles, such as composition, particle size, and charge, to enable targeted delivery of drugs to specific areas within the organism. Active targeting utilized specific ligands to bind to cellular receptors for drug delivery. Passive and active targeting strategies could significantly enhance drug accumulation at liver disease sites, control drug release, reverse drug resistance, and reduce side effects (Yuxin et al., 2024). Among these, polymeric nanoparticles and solid lipid nanoparticles have been widely used in drug delivery systems. However, solid lipid nanoparticles were constrained by limited stability and challenges in lipid functionalization. In contrast, polymeric nanoparticles have been the most widely used due to their advantages to encapsulate diverse bioactive compounds, protect the encapsulated drugs, and enable targeted delivery (Beach et al., 2024). In view of the importance and growing interest in this field, we presented various types of polymers being used as drug delivery systems for hepatitis, liver fibrosis and liver cancer in this review (Table 1). Futhermore, this paper reviewed the application of polymeric nanoparticles-mainly chitosan (CS), hyaluronic acid (HA), polyethylene glycol (PEG) and poly (lactic-co-glycolic acid) (PLGA)-in liver disease treatment, focusing on their use in various delivery systems for pure bioactive compounds of natural origin, drugs, nucleic acids, peptides, and others (Figures 1, 2). And we hope that will trigger some new ideas and stimulate more efforts to promote the widespread use of nanotechnology intreating hepatic diseases.
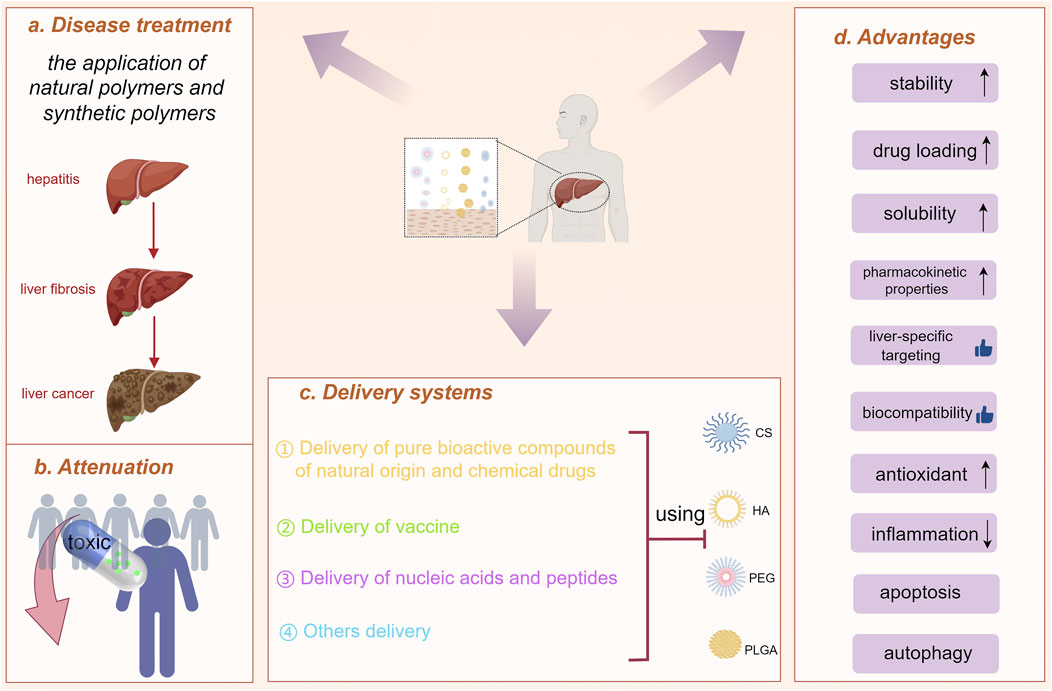
Figure 1. Polymeric nanoparticles (natural and synthetic polymers) used to treat liver diseases. (A) Disease treatment. (B) Attenuation. (C) The application of chitosan (CS), hyaluronic acid (HA), polyethylene glycol (PEG) and poly(lactic-co-glycolic acid) (PLGA) in liver disease treatment, focusing on their use in various delivery systems. (D) Advantages. (drawn by Figdraw, ID: UWPAY4434a).
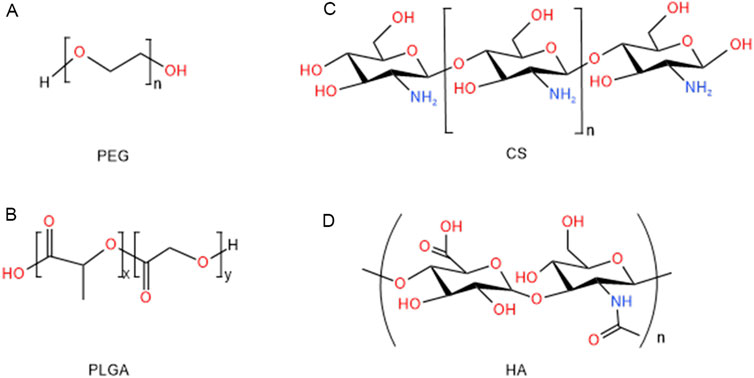
Figure 2. Structure of polymeric nanoparticles for the treatments of hepatic diseases: (A) PEG, (B) PLGA, (C) CS, (D) HA.
2 Polymeric nanoparticles
Polymeric nanoparticles have been employed in a range of traditional applications, including the manufacture of catheters, syringes, and implants for bone, cartilage, skin tissue, and blood vessels. In 1969, Speiser and his team first introduced the concept of polymeric nanoparticles for drug delivery (Shabir et al., 2017). Nowadays, numerous polymeric nanoparticles have been engineered for the targeted delivery of therapeutic agents, including vaccines, pure bioactive compounds of natural origin, nucleic acids, and small molecules, to the liver for the treatment of hepatic diseases. The focus of these advancements is on polymeric nanoparticles that exhibit favorable hydrophilicity, stability within the hematological system, protection of nucleic acids, and efficient endosomal/lysosomal escape, and appropriate surface charge for optimized drug delivery systems. Polymeric nanoparticles were typically divided into two main types according to their source: natural polymers and synthetic polymers. Natural polymers, known for their biocompatibility and biodegradability, were particularly suited for drug delivery and tissue engineering applications. In contrast, synthetic polymers, engineered to possess specific mechanical, chemical, and degradation properties, offered enhanced versatility for a wide range of biomedical and industrial uses (Galina et al., 2024).
2.1 Natural polymers
Natural polymers derive from biological sources such as plants, animals, or microorganisms. They are components of biological systems responsible for performing various essential functions (Bailey et al., 2023). There were many natural polymers frequently utilized for the development of polymeric nanoparticles, such as CS, HA, alginate, collagen, gelatin and cellulose.
CS, a linear β-1, 4-D-glucosamine, is a natural polysaccharide obtained from the deacetylation of chitin. It is the second most common natural polysaccharide in the world, after cellulose (Antonino et al., 2017). CS is non-toxicity and has no side effects, along with good moisturizing and adsorption properties. The United States. FDA has approved that CS is safe in the use of foods and drugs (Rizeq et al., 2019; Wang et al., 2020). CS has varied structures, sizes, and charges, and its chemical functional groups can be easily modified to meet the needs of specific applications and different modes of drug delivery (Rashki et al., 2021). CS has frequently been employed for the design of drug delivery systems due to its biocompatibility, natural degradability, mucosal adsorption, and other physicochemical properties, as well as its ability to demonstrate biological effects, including hypolipidemic, hypoglycemic, antioxidant, anti-inflammatory, antimicrobial, antibacterial, and immunomodulatory effects (Matalqah et al., 2020; Vohra et al., 2021). Meanwhile, CS has been used as a nano-adjuvant for vaccine and a carrier for drug delivery systems in liver disease.
HA is a glycosaminoglycan essential to the extracellular matrix (ECM). It is synthesized by hyaluronan synthases at the inner surface of the plasma membrane and is broken down through enzymatic hydrolysis of its b-1,4-glycosidic bonds by hyaluronidase (HAase) (Salih et al., 2024). It is found in large quantities in the vitreous humor, umbilical cord, dermis, synovial fluid, and heart valves. Approximately 80%–90% of HA is catabolized by the liver, while the remaining 10% is excreted via the kidneys in vivo (Saha and Rai, 2021; Matsumoto et al., 2022). Commercially, HA existed in three different molecular weight forms, high molecular weight (10,000 kD), medium molecular weight (500–800 kD) and low molecular weight (160–240 kD) HA. By modifying the molecular structure of HA, it was possible to create derivatives that were more resistant to mechanical and chemical forces while maintaining compatibility and biodegradability in biological environments. High molecular weight HA primarily exhibited immunosuppressive and anti-angiogenic properties, whereas medium and low molecular weight HA possessed pro-angiogenic and anti-apoptotic effects, induced the synthesis of heat shock proteins (HSP), and acted as effective immunostimulants (Zhu et al., 2020). The related literatures demonstrated that HA was non-toxic, biocompatible, and non-allergenic, promoting wound healing, tissue regeneration, anti-wrinkle effects, and anti-inflammatory responses. These findings might have implications for cancer prognosis (Ahmadian et al., 2019). Therefore, it was widely used in several medical fields, including orthopedics, dermatology, plastic surgery and cardiovascular surgery. In summary, HA’s diverse properties and versatile applications make it a valuable component in the development of therapeutic strategies for hepatic diseases and beyond.
Alginate is a natural polysaccharide primarily derived from brown algae, such as Laminaria, Ascophyllum, and Macrocystis. It has three advantages. First, the biodegradation of alginate under physiological conditions was demonstrated to occur via two distinct mechanisms: enzymatic cleavage by alginate lyase and acidic hydrolysis. Furthermore, modification of the chemical structure of alginate with functional groups or targeting ligands had the potential to enhance a number of properties, including stability, drug loading and liver-specific targeting (Piotr et al., 2021). And alginate readily formed gels in the presence of divalent cations like calcium ions (Ca2⁺), which facilitated the encapsulation of drugs for sustained or controlled release (Cassandra et al., 2022).
Collagen, the primary structural protein in connective tissues, was shown to enhance liver tissue repair by promoting hepatocyte adhesion, migration, and proliferation. It could deliver antifibrotic drugs directly to the liver, where it helped remodel the extracellular matrix and reduce fibrosis. Due to its structural similarity to the hepatic extracellular matrix, it tended to accumulate preferentially in liver tissue, thereby enhancing the efficiency of targeted drug delivery to the liver (Leora et al., 2019).
Gelatin, derived from collagen, a major component of the hepatic extracellular matrix, exhibited excellent biocompatibility. This property minimized immune responses, making it an ideal candidate for liver-targeted drug delivery. Gelatin was capable of encapsulating various therapeutic agents, such as anti-inflammatory, antifibrotic, and anticancer drugs (Yutao et al., 2024). In acidic lysosomal environments, gelatin degraded, enabling controlled drug release at the site of liver injury. Due to its water solubility, gelatin often required cross-linking during nanoparticle formulation to enhance stability and control release (Morán et al., 2015).
Cellulose is a polysaccharide found in plants cell walls (Pedersen et al., 2023). It exhibited both hydrophilic and hydrophobic properties, making them suitable for a wide range of biomedical applications. To enhance liver-specific drug delivery,it was functionalized with targeting ligands, a strategy that significantly improved their ability to selectively accumulate in hepatic tissues. This targeted delivery approach proved particularly valuable in the treatment of hepatic diseases, such as hepatitis and liver cancer, where localized drug release was essential for maximizing therapeutic efficacy while minimizing systemic side effects (Ansar et al., 2021).
2.2 Synthetic polymers
Synthetic polymers, which could be easily adjusted in terms of monomer ratios, molecular weights, and chemical bonding, were characterized by their diversity. Due to their unique physicochemical properties, drugs could be efficiently encapsulated or conjugated by synthetic polymers, allowing for specific targeting of liver tissue and providing extended drug release at liver sites (Mohammed and Naif, 2022). Common synthetic polymers used in nanoparticle fabrication included PEG, PLGA, polycaprolactone (PCL), polymethyl methacrylate (PMMA), and others (Table 1). In recent years, the interest in synthetic polymers has grown exponentially due to their superiority. Researchers have made several adjustments to develop effective nanoparticles, including particle size, zeta-potential, size distribution, shapes and matrix composition (Kenward et al., 2021). This led to more predictable and reproducible outcomes in therapeutic applications. Moreover, synthetic polymers could be more easily functionalized to improve stability, targeting efficiency, and bioavailability.
PEG is a synthetic, highly water-soluble inert polymer (D’souza and Shegokar, 2016). Its chemical formula is H(OCH2CH2)n OH, where corresponds to the number of ethylene oxide units (Turecek Peter et al., 2016). Due to their non-toxicity, low immunogenicity, high safety, diverse physicochemical properties, biocompatibility and hydrophilicity, it was commonly used in pharmaceuticals, non-pharmaceutical products, and cosmetics (Yanjie et al., 2024). In 1990, the United States food and drug administration (FDA) approved the first PEGylated protein product for the treatment of severe combined immunodeficiency disease (Jevsevar et al., 2010; Haesun et al., 2022). PEGylation is generally defined as the covalent attachment of activated PEG to therapeutic agents. Since the first PEG modification of bovine serum albumin by Davis in 1977, PEGylation had been widely used in the research and applications to improve the physicochemical properties and biological activities of proteins (peptides), enzymes, antibodies and small molecule drugs. The solubility and flowability of various drugs could be significantly improved by chemical modification with PEG (PEGylation). For example, certain drugs had a short duration of action in vivo and were insoluble in water. PEG was utilized in the treatment of drugs to shield them from degradation by the human immune system and extend the drug’s pharmacokinetic profile in the body. Once the drug was treated with PEG, it acted like a layer of “camouflage” to avoid being eliminated by the human immune system as a foreign substance (Veronese and Pasut, 2005). At the same time, PEGylation of drugs reduced the dosage, improved the performance of existing drugs, prolonged the efficacy and reduced the cost of drugs. Therefore, PEG has been widely welcomed in the development of novel nanoparticles for the treatment of hepatic diseases.
PLGA is a biodegradable polymeric nanoparticles. It was approved by the United States. FDA and the European Medicines Agency (EMA) for medical applications, with several formulations such as Decapeptyl, Suprecur MP, and Lupron Depot receiving clinical approval (Makadia and Siegel, 2011). The excellent biocompatibility, biodegradability, and unique physicochemical properties of PLGA made it the most popular and effective drug delivery polymer (Mazen and José, 2022). PLGA was extensively studied for developing delivery vehicles for controlled release of small molecules, proteins, and other macromolecules (e.g., DNA, RNA, and peptides) for commercial (Bouissou et al., 2006; Essa et al., 2020). Since PLGA was biologically hydrolyzed into metabolite monomers (lactic acid and glycolic acid), which were then endogenously metabolized by the body via the citric acid cycle. Therefore, no systemic toxicity was associated with the utilization of PLGA for drug delivery. This positioned it as one of the most attractive polymer candidates in the field of drug delivery systems (Rosli et al., 2021).
PCL is a biodegradable polymer that has been proved to be particularly useful for the controlled and sustained release of drugs due to its slow degradation rate. It might be utilised as a method of encapsulating chemotherapeutic agents for the treatment of liver cancer (Moreira et al., 2022; Zhou et al., 2024). By functionalizing PCL nanoparticles with liver-specific ligands, selective delivery to liver tumors was achieved, enhancing the efficacy of the drugs while minimizing toxicity to healthy tissues (Bate et al., 2022). Additionally, it was metabolized into non-toxic degradation products, ensuring the safety of the microparticles for medical applications.
PMMA, a biocompatible and inertpolymer with high spinnability, is also a hydrophobic polymer (Srikar et al., 2007). It is widely used for the construction of medical devices such as bone cemen, drug delivery applications and microsensorst. It was commonly used for the long-term release of drugs, especially in sustained or extended-release applications. It was employed to encapsulate imaging agents, such as magnetic nano/microparticles or fluorescent dyes, for non-invasive liver cancer detection using MRI, PET, or fluorescence imaging techniques (Montri et al., 2013; Naoki et al., 2019).
Even though various natural and synthetic polymers were developed and tested in recent decades, indicators such as bioavailability, biocompatibility, biodegradability, toxicity, efficiency, and selectivity differed significantly. This was primarily due to the nature of microparticles and their physicochemical properties. The clinical development of the rest of nanoparticles was relatively limited compared to CS, HA, PEG, and PLGA. Currently, there is limited literature available regarding its application as a drug delivery system for liver diseases. Although these nanoparticles had shown promising results in preclinical studies, their clinical translation was slow, often due to challenges such as toxicity, biocompatibility, or difficulties in targeted delivery. In summary, in the following section, the effects of nanoparticles, using CS, HA, PEG, and PLGA as examples, on hepatitis, liver fibrosis, and liver cancer will be reviewed in detail. Hopefully, this review will stimulate further research into liver disease therapies.
3 The application of CS in treating hepatic diseases
3.1 Delivery of pure bioactive compounds of natural origin and chemical drugs using CS
The study showed that the combination of CS with pure bioactive compounds of natural origin and chemical drugs significantly improved the stability and bioavailability, offering potential benefits for hepatitis treatment while alleviating oxidative stress and inflammatory responses (Metkar et al., 2023). Specifically, it effectively ameliorated ibuprofen-induced elevations in serum aminotransferases, alkaline phosphatase, albumin, and total bilirubin, as well as elevated lipid peroxidation (MDA) and nitric oxide. It also significantly elevated glutathione, glutathione S-transferase, superoxide dismutase, interleukin-6 and B-cell lymphoma-2 (Bcl-2) levels, decreased interleukin-1β and nuclear factor κ-B (NF-κB) levels, enhanced the degradation of toxic effects during ibuprofen treatment, improved the antioxidant system and anti-inflammatory status, as well as increased serum mineral levels (AlKandari et al., 2024).
Compared to the use of drugs alone, combining CS with pure bioactive compounds of natural origin and chemical drugs significantly reduced liver fibrosis. For instance, Streptomyces malachiticus coated with curcumin-CS mixture (Elzoheiry et al., 2022), and CS loaded with Itraconazole (ITRCZ) (Elmorsy et al., 2024) or salvinorin B (Sal B) (Yao et al., 2024), could overcome issues of drug insolubility and permeability encountered with single-drug administration. They displayed potent antifibrotic effects, as indicated by the downregulation of transforming growth factor (TGF-β) and tissue inhibitor of metalloproteinases-1 (TIMP-1), as well as decreased hydroxyproline content and α-smooth muscle actin (α-SMA) immunoexpression. They also significantly improved oral bioavailability, while enhancing antioxidant and anti-inflammatory activities.
The pure bioactive compounds of natural origin and chemical drugs using CS for the treatment of liver cancer markedly enhanced the bioavailability and targeting ability of the drugs (Nimeet et al., 2023). Certain factors, such as cell apoptosis and autophagy activation, contributed to liver cancer treatment by modulating cellular processes involved in tumor progression. For instance, CS-coated ivosidenib- PLGA-nanoparticles exhibited higher stability in liver cancer treatment and significantly increased the expression of caspase-3, caspase-9, and p53 (Alsulays et al., 2024). These nanoparticles also inhibited liver cancer cell proliferation, and exhibited anti-migration and anti-angiogenesis properties, while down-regulating Bcl-2 and inducing apoptosis (Harakeh et al., 2023). Curcumin piggybacked niacin-CS could induce autophagy by activating the G-protein coupled receptor 109A (GPR109A)/amp-activated protein kinase (AMPK)/nuclear factor (erythroid-derived 2)-like 2 (Nrf2) signaling pathway, thereby enhancing the cellular degradation (Hong et al., 2017; Hanafy et al., 2023). These nanoparticles effectively targeted subcellular organelles by escaping endosomal and lysosomal cleavage, producing a proton sponge effect. After escaping from the endolysosomal pH, they effectively released rutin into the nucleus, thereby enhancing its cellular bioavailability (Wu et al., 2024). This targeted drug delivery system demonstrated strong performance. Additionally, CS could change the morphology of nanoparticles and improve the stability of nanosystems. The micro/nanoparticles with diameters ranging from 5 to 200 nm contribute to prolonging their circulation time in the bloodstream (Sun et al., 2017; Mondal et al., 2022).
3.2 Delivery of vaccine using CS
As an immunostimulant, CS can be used as vaccine adjuvants, improving vaccine efficacy by stimulating specific immunity. CS can induce sustained humoral and cellular immunity against infectious agents or non-infectious diseases. For example, CS has been demonstrated to serve as an effective carrier adjuvant system for interleukin-12 (IL-12) combined with hepatitis B surface antigen (HBsAg), which significantly enhanced HBV-specific CD8+ T and CD4+ T cell responses, achieving long-term memory against HBV (Zhao et al., 2021). Furthermore, the simultaneous administration of type I IFNs and CS resulted in enhanced serum immunoglobulin G (IgG) and immunoglobulin A (IgA) antibody responses, as well as increased the mucosal IgA antibody response and antitoxin titers (Bento et al., 2019; Maeyama et al., 2020). Meanwhile, nanovaccines were synthesized with precise size distribution and efficient encapsulation through utilizing charge complexation between CS and heparin to encapsulate recombinant hepatitis B virus surface antigen (rHBsAg) or core antigen (rHBcAg) (Qiao et al., 2021).
3.3 Delivery of nucleic acids and peptides using CS
CS has gained attention as a non-viral gene delivery system. It could be used as a DNA carrier for gene delivery, as it can condense nucleic acids into stable complexes ranging from 100 to 250 nm in size (Bolhassani et al., 2014). CS-based nanocarriers have been developed for the intracellular delivery of 10–23 DNAzyme, which is assembled into micelles in aqueous solution (Hong et al., 2019). They significantly improved delivery efficiency to hepatocytes and prolonged retention time in the liver (Fatouh et al., 2021). CS nanoparticles demonstrated lower cytotoxicity and high cellular uptake efficiency (Nimesh et al., 2010). Furthermore, CS is often selected as anti-liver fibrosis nucleic acid drug delivery carriers due to its affinity for collagen. CS significantly inhibited collagen over deposition in HSCs and promoted the delivery of anti-TGF-β siRNA through platelet-derived growth factor receptor-β (PDGFR-β) binding peptide modification. In vivo, CS successfully accumulated in fibrotic livers via collagen binding peptides (CBPs) modification and inhibited TGF-β1 expression, thus exerting antifibrotic effect (Azzam et al., 2020; El-Safy et al., 2020).
3.4 Others
In addition to the three delivery systems mentioned above, CS also demonstrated significant tumor-suppressive effects through the activation of the mitochondrial pathway and endoplasmic reticulum stress, leading to excessive ROS production and subsequent apoptosis in hepatocellular carcinoma cells (Kadry et al., 2018; Jiang et al., 2019). Concurrently, it could be engineered as hydrophilic nanoparticles with a neutral charge to minimize their uptake by phagocytic cells, such as macrophages, within the immune system (Jiang et al., 2022).
4 The application of HA in treating hepatic diseases
4.1 Delivery of pure bioactive compounds of natural origin and chemical drugs using HA
HA targeted cancer cells by binding to the CD44 receptor, improving drugs delivery efficiency and reducing side effects on normal tissues, thereby enhancing the therapeutic effect (Shao et al., 2024). Studies have shown that self-assembly of methoxy polyethylene glycol-poly lactic acid block copolymer (mPEGPLA) and hyaluronic acid-paclitaxel conjugate (HA-PTX) produced composite nanoparticles (mPPHP NPs) for effective cancer therapy. Paclitaxel (PTX) was rapidly released under the action of HA and esterase enzymes. mPPHP NPs exhibited selective cytotoxicity against A549 cells in vitro via CD44 receptor-mediated cellular uptake (Luo et al., 2020).
HA exhibits metabolic targeting. Drug-loaded HA reduced the supply of essential nutrients, such as glutamine and glucose, to cancer cells, thereby enhancing intracellular ROS production. Elevated levels of intracellular ROS trigger the activation of AMPK, resulting in the inhibition of protein kinase B (AKT) phosphorylation and ultimately inducing apoptosis in cancer cells (Ghosh et al., 2023). Varying molecular weights of HA can target solid tumor-associated macrophages, allowing for easy combinations of different components and ensuring that the molecular weight of the polymer or the preparation technique does not adversely affect the targeting ability (Fernandez-Marino et al., 2023).
4.2 Delivery of nucleic acids and peptides using HA
RNA interference (RNAi)-mediated gene silencing is frequently employed as an alternative therapeutic strategy for infectious diseases, including refractory hepatitis C virus (HCV) infection. A non-viral vector composed of HA, protamine, and a short hairpin RNA (shRNA74) was designed to target the internal ribosome entry site (IRES) of HCV. This vector demonstrated the capacity to inhibit the expression of the HCV IRES in Huh-7 cells, and it was subsequently rapidly and effectively internalized by the cells (Torrecilla et al., 2016). HA is biocompatible, biodegradable, and has the potential to target tissues and provide receptor-mediated uptake by specific cells, making it a valuable delivery agent for oligonucleotide-based therapies (Rao et al., 2016; Ossipov, 2019).
4.3 Others
HA plays a crucial role in the detection and treatment of viral hepatitis. HA-carbon nanotubes (CNTs) composites have been investigated as biofibers or electrode materials for bioelectrochemical applications. Cabral et al. developed a method for the detection of antibodies to hepatitis B core protein (anti-HBc). A nanohybrid surface assembled onto a glassy carbon electrode, consisting of amino-functionalized carbon nanotubes modified with hyaluronic acid, served as a sensing platform for anti-HBc detection. A large number of biomolecules were immobilized on the electrode surface with CNTs membranes, leading to high electron transfer and enabling label-free detection using redox probes (Cabral et al., 2016).
HA has a specific binding affinity for the CD44 receptor, which is highly expressed on the cell surface. During the development of liver fibrosis, activated HSCs (aHSCs) undergo proliferation, leading to a significant increase in CD44 expression on their surface (Kim and Seki, 2023). HA might not only improve drug efficiency but also specifically target aHSCs (Chen et al., 2016; Yu et al., 2024). This targeted approach resulted in the selective induction of apoptosis in aHSCs while sparing quiescent HSCs and hepatocytes (Gong et al., 2023).
The use of HA-coated micelles for drug delivery represents a promising approach for the clinical treatment of liver fibrosis (Li et al., 2020). HA-coated micelles exhibited a core-shell structure comprising a self-assembled biodegradable poly (L-lysine)-b-poly (lactic acid) AB-diblock copolymer (PLys+-b-PLLA) core and an exterior coating of HA. The coating was formed through polyion complexation via electrostatic interaction between the anionic HAs and cationic PLys segments. HA-coated micelles exhibited specific cellular uptake of LX-2 cells in vitro (Yoshizaki et al., 2023). Furthermore, HA micelles facilitate the increased delivery of chlorosartan, being specifically taken up by HSCs (Thomas et al., 2015).
5 The application of PEG in treating hepatic diseases
5.1 Delivery of pure bioactive compounds of natural origin and chemical drugs using PEG
PEGylation improved the pharmacological properties of small molecule therapeutics (Hooda et al., 2023). PEGylated interferon (IFN) or nucleoside analogs (lamivudine, adefovir, ribavirin, etc.) were currently important drugs for the treatment of chronic viral hepatitis. Among them, PEGylated IFN-α included PEGylated IFN α-2b (12 kD) and PEGylated IFN α-2a (40 kD), which reduced the clearance of this protein and effectively prolonged the T1/2. However, the latter is more stable in blood concentration and had a longer half-life (T1/2) due to the greater molecular weight of PEG and better protection against interferon, and its drug distribution was more concentrated (mainly in the blood and liver). PEGylated IFN exhibited a toxicity profile comparable to that of regular α-IFN, while offering a more advantageous pharmacokinetic profile and requiring less frequent administration. Additionally, it enhanced sustained efficacy by approximately 10% relative to regular α-IFN (Tang et al., 2018; Koh et al., 2019; Huang et al., 2022; Etzion et al., 2023; Huang et al., 2023; Wen et al., 2023; Jiang et al., 2024). Meanwhile, PEGylated ribavirin treated patients with chronic HCV infection with an SVR of up to 56% (Abd Ellah Noura et al., 2018; Pawlowski et al., 2024).
Besides, PEGylated drugs could enhance solubility, stability, and pharmacokinetic properties, extending T1/2 and reducing side effects (Li et al., 2020). PEGylated IFN α-2a (PEG-IFN-α-2a) (Chen et al., 2021), Pegbelfermin (PGBF), and short-chain PEG-modified curcumin derivative ameliorated the progression of liver fibrosis. These compounds not only increased drug concentrations in the blood but also maintain their anti-inflammatory activity. Meanwhile, they could effectively inhibit the expansion of scar-associated macrophage subpopulations oxidative stress (ROS) and scar-producing myofibroblasts in the damaged liver and remodel the fibrotic ecological niche by modulating ligand-receptor interactions, including platelet-derived growth factor-β (PDGF-β)/platelet-derived growth factor receptor-alpha (PDGFR-α) (Cheng et al., 2018; Xiao et al., 2021). Li F. et al. developed the β-D-Galactose-PEGylated bilirubin nanomedicine (GBRNP) for the treatment of liver fibrosis. It was introduced to target hepatocytes, scavenge ROS. The GBRNP micelles encapsulating selonsertib (Sel@GBRNPs) improved the solubility of the drug and afforded its targeted delivery to aGVwYXRvY3l0ZXMuU2VsQEdCUk5Qcw== could synergically protect hepatocytes from H2O2-induced damage, by decreasing signs of apoptosis and ROS levels. Meanwhile, riociguat combined with Sel@GBRNPs robustly attenuated hepatocyte apoptosis and progression of liver fibrosis in the CCl4-induced fibrosis model compared to riociguat + Sel treatment (Li F. et al., 2023).
Pure bioactive compounds of natural origin and chemical drugs loaded in PEG microparticles provided excellent targeted delivery and anti-cancer efficacy for liver cancer (Li et al., 2021). Paclitaxel (PTX) was attached to PEG to form nanodrugs. Due to the oxidation of the core cross-linking structure via sulfhydryl groups, the nanoparticles could be selectively uncoupled by disulfide bond reduction in the reducing microenvironment inside the tumor. This process released the loaded PTX to kill cancer cells while maintaining a high level of stability under physiological conditions outside the tumor (Cai et al., 2021). These nanoparticles were relatively uniform in size, approximately 200 nm, and exhibit a spherical shape. They demonstrated slow-release properties in vitro, with a high T1/2 and area under the curve (AUC) values, as well as a prolonged circulation time in vivo, thus increasing bioavailability of PTX (Qin et al., 2023). The introduction of hydrophilic PEG chain segments into hydrophobic PLGA molecules enhanced their hydrophilic properties, improved stability and anti-enzymatic activities, and enhanced interaction with cellular components. This modification also prolonged circulation time and reduced systemic clearance. The galactosylated chitosan (GC)-coated PEG-PLGA microparticles (GC@NPs) were developed for curcumin (CUR) delivery, which specifically bound to cancer cells that highly expressed the asialoglycoprotein receptor and precisely released CUR. The designed and synthesized microparticles significantly enhanced the drug retention time in vivo (Wang et al., 2020; Huang et al., 2023; Huang et al., 2024). Artesunate (ART)-loaded and glycyrrhizin (GA)-modified PEG-PLGA promoted the hepatic targeting distribution of ART, increased retention time, and promoted anti-tumor effects (Pan et al., 2020; Pan et al., 2023). Meanwhile, PEG could promote the anti-tumor effects of natural phenolic antioxidants (Sasaki et al., 2023; Darguzyte et al., 2024). Furthermore, hypoxic tumor cells acted as a key target for treating tumor. For example, PEG with folic acid (FA) and 2-nitroimidazole self-assembled into micelles by chemical coupling, which enabled effective and prolonged drug release in hypoxia-sensitive areas and specifically killed hypoxic tumor cells (Li et al., 2021; Meng et al., 2022). Overall, PEG nanoparticles had been a promising nanocarrier with good biosafety, serving as carriers for the delivery of pure bioactive compounds of natural origin and chemical drugs.
5.2 Delivery of nucleic acids and peptides using PEG
As research on PEGylation progresses, its applications are expanding, including as delivery systems for siRNA, mRNA, and pDNA (Wu et al., 2019). No toxicity was detected in an in vivo model system using a variety of hepatic cell types and mice with reversed fibrosis. PEG loaded by siRNA was targeted almost exclusively to the liver by intravenous injection and were taken up by nearly 50% of activated HSCs and activated human myofibroblast HSCs (Kaps et al., 2015). PEGylation helps prevent particle aggregation and improves storage stability.
The miRNA nanotherapeutics, activated by a stepwise stimulation of acidity and reduction to mimic the tumor microenvironment, effectively enhanced liver-specific miR-122 expression, thereby increasing the feasibility of translating miR-122 therapy for use against liver cancer (Wu et al., 2019). The PEG has three important novelties: its broad applicability for oligonucleotide cargo delivery, demand-driven pharmacodynamic personalization, and distinguished biodegradability, providing additional possibilities for PEG in the treatment of liver cancer.
5.3 Others
Fibrosis hinders polymeric nanoparticles delivery to liver cells, but cationic nanoparticles have been demonstrated to enhance hepatocyte delivery by disrupting endosomal systems and increasing cellular interactions through their pH buffering capacity (Ezhilarasan, 2021). For example, PEG coating enabled nanoscale graphene oxide (NGO) to be predominantly retained in the liver, lungs, and spleen, with particle sizes ranging from 10 to 800 nm, which facilitated the clearance of NGO from these organs, thereby alleviating liver fibrosis (Li et al., 2014). PEG attenuates oxidative stress, the inflammatory response and cellular damage in the liver (Wang et al., 2020; Acar et al., 2022; Bertolini et al., 2024). PEG had good hematological and cytocompatibility, as well as pH-sensitive stability (Xiang et al., 2023). PEG nanoparticles were mixed with extracellular vesicles derived from mesenchymal stem cells (EVs) to form EV-encapsulated PEG hydrogels via a fast, biocompatible click reaction. It could extended the time of EVs targeting in liver fibrosis (Mardpour et al., 2019).
For liver cancer, PEG nanoparticles could utilize redox-responsive components to target cancer cells, and significantly reduce the inflammatory response (Arib et al., 2021; Nisha et al., 2021). More specifically, antioxidants were elevated in hepatocellular carcinoma cells. Therefore, the use of polymers with the anti-oxidant response element allowed them to actively target liver cancer cells and trigger the release of drugs (Powell et al., 2022). Typically, the reduction in blood component interactions induced activation of the complement system, leading to reduced blood clearance of the drug carrier. PEG was a non-ionic hydrophilic polymer that provided a so-called “stealth” coating, which, through spatial stabilization, reduces the tendency of nanoparticles to aggregate and affects the pharmacokinetic properties of the drug or carrier (Cui et al., 2019). PEG shielding or administration extended circulation time and increased the likelihood of the drug reaching the site of action. (Liu et al., 2021; Xiao et al., 2023). The passive targeting of large nanoparticles by the EPR effect represented a pivotal concept in the context of solid tumor targeting in cancer nanomedicine. It was noteworthy that smaller-sized PEGs (≤20 kD, 12 nm) demonstrated substantial tumor targeting with minimal to no nonspecific uptake, whereas larger-sized PEGs (>20 kD, 13 nm) exhibited a marked accumulation in major organs, including the lungs, liver, and pancreas (Kang et al., 2020). PEG microspheres were polymeric particles capable of absorbing organic compounds. They had good compressibility and elasticity and could be suspended for extended periods (Giammaria et al., 2019; Lucatelli et al., 2019). Therefore, it could be attributed to the fact that PEG can persist in liver disease by modulating oxidative stress and the inflammatory response, and control drug release in the form of PEG microspheres.
6 The application of PLGA in treating hepatic diseases
6.1 Delivery of pure bioactive compounds of natural origin and chemical drugs using PLGA
Herbal extracts from natural medicines have the advantage of low toxicity and fewer side effects. However, the bioavailability and bioactivity of these extracts are limited by poor water solubility and rapid metabolism. PLGA improved the solubility and bioavailability of melatonin, berberine (BBR), and natural metabolites of retinoids (e.g., all-trans retinoic acid), and also reduced potential side effects during systemic administration, with good targeting and biosafety. Furthermore, PLGA could be modified to change the structure, packing shape and drug release kinetics of the prepared nanoparticles to meet the needs of different nanomaterial applications (Qiao et al., 2018; Fan et al., 2020; Ji et al., 2020). This approach presents a promising strategy for alleviating liver injury.
Similarly, PLGA could also improve the disadvantages of narrow therapeutic window, severe toxicity, and poor water solubility of antitumor drugs (Li et al., 2024). It was loaded with plumbagin and dihydrotanshinone I (a phenanthraquinone compound of Salvia miltiorrhiza) significantly improving the T1/2 and tumor targeting of these two drugs in mice with in liver cancer, which yielded the therapeutic effect of reversing the immunosuppressive tumor microenvironment (Han et al., 2022). PLGA loaded with betulinic acid (B) or doxorubicin (DOX) exhibited an initial burst and sustained release (Gao et al., 2019; Kumar et al., 2022). These nanoparticles exhibited stronger cytotoxicity at lower concentrations compared to free drug and were well internalized by HepG2 cells (Upadhyay et al., 2020). Additionally, they were effective in prolonging the T1/2 of the drugs, with higher drug concentrations in the plasma and liver. PLGA utilizes EPR effects to passively target tumor, prolong circulation time in vivo and improve drug accumulation in the tumor (Wu et al., 2024).
6.2 Delivery of vaccine using PLGA
PLGA has the capability to encapsulate various antigens and deliver them to antigen-presenting cells (APCs) (Figure 3A) (Zheng et al., 2023). PLGA-based vaccines have been demonstrated to effectively target APCs, thereby inducing robust cellular and humoral immune responses (Zhang et al., 2019). As an adjuvant for a therapeutic vaccine against viral hepatitis, PLGA significantly induced secretion levels of IL-2 and IFN-γ, and increased the absolute number of CD4+ and CD8+ T cells, triggering a cell-mediated immune response in mice (Roopngam et al., 2016; Mohammed and Naif, 2022). Additionally, it can effectively reduce adverse reactions associated with vaccinations and lower the required dosage in immunized animals, thereby improving the overall immune effects (Allahyari and Mohit, 2016).
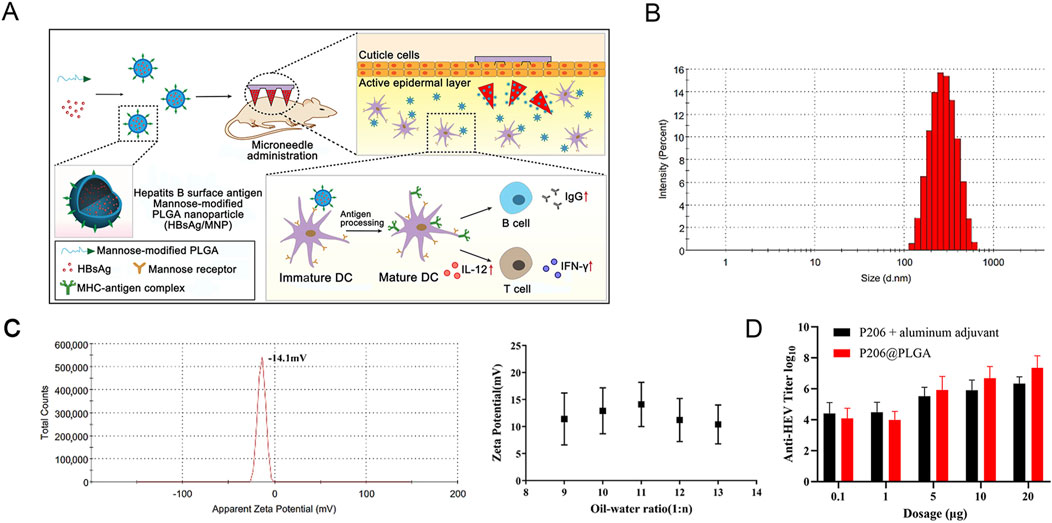
Figure 3. (A) The structure of PLGA nanoparticles and the immune response for nanoparticles in vivo. Reprinted with permission from (Zheng et al., 2023). Copyright 2024, Springer Nature. (B) P206@PLGA nanoparticles size and (C) zeta potential. (D) Determination of anti-HEV antibody titer in the serum after immunization with P206 plus aluminum adjuvant and P206@PLGA. The antibody titer induced by P206@PLGA in the 20 µg group was significantly higher than that in the P206 plus aluminum adjuvant group (p < 0.05). Reprinted with permission from (Yang et al., 2022). Copyright 2022, MDPI.
Moreover, PLGA was applied as a nanocarrier to encapsulate P206 (the immunogenic ecombinant protein), resulting in the preparation of P206@PLGA microparticles with a particle size of approximately 354 nm and a negatively charged surface. It was uniform in size, stable in quality, and could improve the bioavailability and prolong the duration of drug action. P206@PLGA was found to be more stable and induced higher antibody titers compared to traditional vaccines containing P206 and aluminum adjuvant (Figures 3B–D) (Yang et al., 2022). Once microparticles entered the body, they were rapidly engulfed by macrophages of the reticuloendothelial system, resulting in expedited removal from the circulation. This was observed within 30 min following intravenous administration. A substantial quantity (60%–90%) of antigen-loaded particles were subsequently distributed to the liver (Dai et al., 2017; Dewangan et al., 2018).
Intravenous administration of PLGA nanovaccines [PLGA loaded with ovalbumin and invariant natural killer T (iNKT1) cell agonist] mainly targets the liver and spleen where iNKT1 cells are abundant and result in the highest serum IFN-γ levels, T cell cytotoxicity, and Th-1 type antibody responses (Dolen et al., 2020). This offers insights into the development of vaccines for anti-tumor applications.
6.3 Delivery of nucleic acids and peptides using PLGA
PLGA has also received widespread attention for delivering nucleic acids and proteins in the treatment of liver cancer. The small interfering RNA (siRNA) targeting alpha-fetoprotein (AFP) mRNA was found to induce apoptosis and cell death in HepG2 cells, highlighting its potential as a therapeutic agent. To deliver the AFP siRNAs into liver cancer cells, the siRNAs were loaded into the nanoparticles based on PLGA. It provided a sustained release of the drug, thereby maintaining high drug concentration at the target site while reducing systemic toxicity (Pho-Iam et al., 2021).
6.4 Others
The profibrogenic liver environment, characterized by fibrogenesis and chronic stimulation of HSCs, is formed by hypoxia and hydrogen peroxide (H2O2) accumulation. PLGA exhibits enhanced antifibrotic efficacy by eliminating excess H2O2 and alleviating hypoxic stress (Dong et al., 2023). This makes PLGA particularly relevant in the context of liver disease treatment. Choi et al. designed CO2 gas generating PLGA microsphere (MS) for the rapid release of tunicamycin in TACE treatment for liver cancer (Choi et al., 2021). Drugs were dispersed or dissolved in MSs and released slowly through various mechanisms such as self-diffusion, biodegradation, dissolution and osmotic pressure, which improved the efficacy and reduced side effects. Commonly used carrier materials include poly (lactic acid), poly (glycolic acid), and PLGA. PLGA is a co-polymer constituted by lactic acid and glycolic acid at a certain proportion. The hydrophilicity and degradation cycle of PLGA could be controlled by adjusting the molecular weight and the lactic-to-glycolic acid ratio, thereby optimizing their performance in drug delivery applications (Tian et al., 2018). In recent years, PLGA MSs have received widespread attention due to their excellent biocompatibility and biodegradability.
Biodegradable block copolymers play a crucial role in the field of nanoparticles, particularly for drug delivery applications. This is due to their efficient drug loading rate, good biocompatibility, and high bioavailability. Among them, PLGA-PEG is currently widely used (Mo et al., 2022; Lin et al., 2024). PLGA-PEG demonstrated good loading efficiency for BEZ235 (a PI3K/mTOR inhibitor) and high selectivity for Glypican-3-positive HepG2 cells (Tang et al., 2020). At the nominal concentration, they synergistically killed liver cancer cells with significantly higher efficiency than the free drugs. These characteristics make PLGA-PEG a promising candidate for improving the therapeutic efficacy of anticancer treatments.
7 Discussion
Hepatic diseases pose a serious threat to human health. The progression from hepatitis to liver fibrosis to liver cancer is also known as the “liver cancer trilogy”. Currently, drugs such as interferon, certain pure bioactive compounds of natural origin, sorafenib, and lenvatinib are primary treatment strategies for hepatic diseases in clinical practice. However, their use is limited due to low bioavailability, poor solubility, and the potential for drug resistance, and various side effects associated with long-term use. Liver transplantation is a successful treatment method for advanced liver cancer patients, yet it faces challenges such as organ shortages and high medical costs. In recent years, new drugs for liver disease have been developed continuously to improve the bioavailability and efficacy of delivered drugs. Polymeric nanoparticles offer many advantages as drug delivery systems, including regulated size and shape, highly specific targeting, stimuli responsiveness and high loading capacity (Patra et al., 2018). As an interdisciplinary field, nanotechnology has been widely used in researching liver disease treatment and has demonstrated promising therapeutic effects. Although well-appreciated results have been achieved, there are still many issues to be resolved.
First, Nanoparticles are more susceptible to aggregation, hygroscopic properties, contamination and degradation because of their high surface area and large nanoscale dimensions. Inappropriate storage conditions may lead to aggregation or degradation, necessitating rigorous standards for storage conditions and durations of nanomedicines (Muthu and Feng, 2009). Additionally, the preparation process for some polymeric nanoparticles is complex and has poor repeatability, making some nanoparticles difficult for large-scale production for clinical applications (Shepherd et al., 2021). Furthermore, the targeting and therapeutic efficacy of polymers is significantly constrained by the high complexity of both the liver and the cancer microenvironment. Since endogenous signals inside and outside cancer cells are difficult to control and vary between individuals, this variability can lead to erratic therapeutic effects. Several solutions address these issues. First, introducing microfluidic devices is one approach to solving the problem. It improves the scalability of polymeric nanoparticles and reduces production time and preparation steps. Additionally, it enables uniform control over the properties of drugs on a larger scale, which reduces the risk of aggregation or degradation (Valencia et al., 2020). Secondly, simple, rapid and affordable high-throughput screening methods can be employed to optimize various formulation parameters of nanoparticles. The design of experiments (DOE) is one of the most promising strategies (Jones et al., 2016; Ho et al., 2019). Furthermore, new manufacturing techniques and equipment for nanoparticles are being developed to produce low-toxicity polymeric nanoparticles, while simplified preparation processes facilitate large-scale production. Nanocarrier systems can be designed to contain multiple targeting mechanisms. Unlike single targeting approaches, these nanoparticles can carry two or more “guidance' signals simultaneously, increasing the accuracy and probability of identifying targeted tissue. Finally, it is also important to develop biomimetic in vitro test platforms and preclinical models that can effectively evaluate the properties of polymeric nanoparticles. Such assessments can more accurately predict their behavior in vivo. In the future, researchers may be able to provide personalized and precise drug delivery for patients by understanding the immunology of liver disease. For example, polymeric nanoparticles can target macrophages in the liver and improve the efficacy of treatments by modulating their immune response (Tacke, 2017). A rigorous biosafety assessment is required for polymeric nanoparticles, evaluating immunogenicity, the safety of degradation products, effects on liver function, and pharmacokinetic effects on the drug delivery system. Although several polymers for hepatic diseases are currently in clinical trials (Table 2), further efforts are needed to develop effective treatments for hepatic diseases. Therefore, it is essential to develop nanoparticles suitable for liver disease treatment and to further study the mechanisms of nanoparticles in hepatic diseases. Natural polymers and synthetic polymers each presented unique advantages and challenges in the treatment of hepatic diseases. Natural polymers excelled in biocompatibility and biodegradability, making them ideal for long-term therapies, while synthetic polymers offered greater control over drug release and targeting, making them suitable for precision medicine applications. The development of hybrid nanoparticle systems that combined the best features of both types of nanoparticles might hold the key to more effective and safer treatments for hepatic diseases in the future.
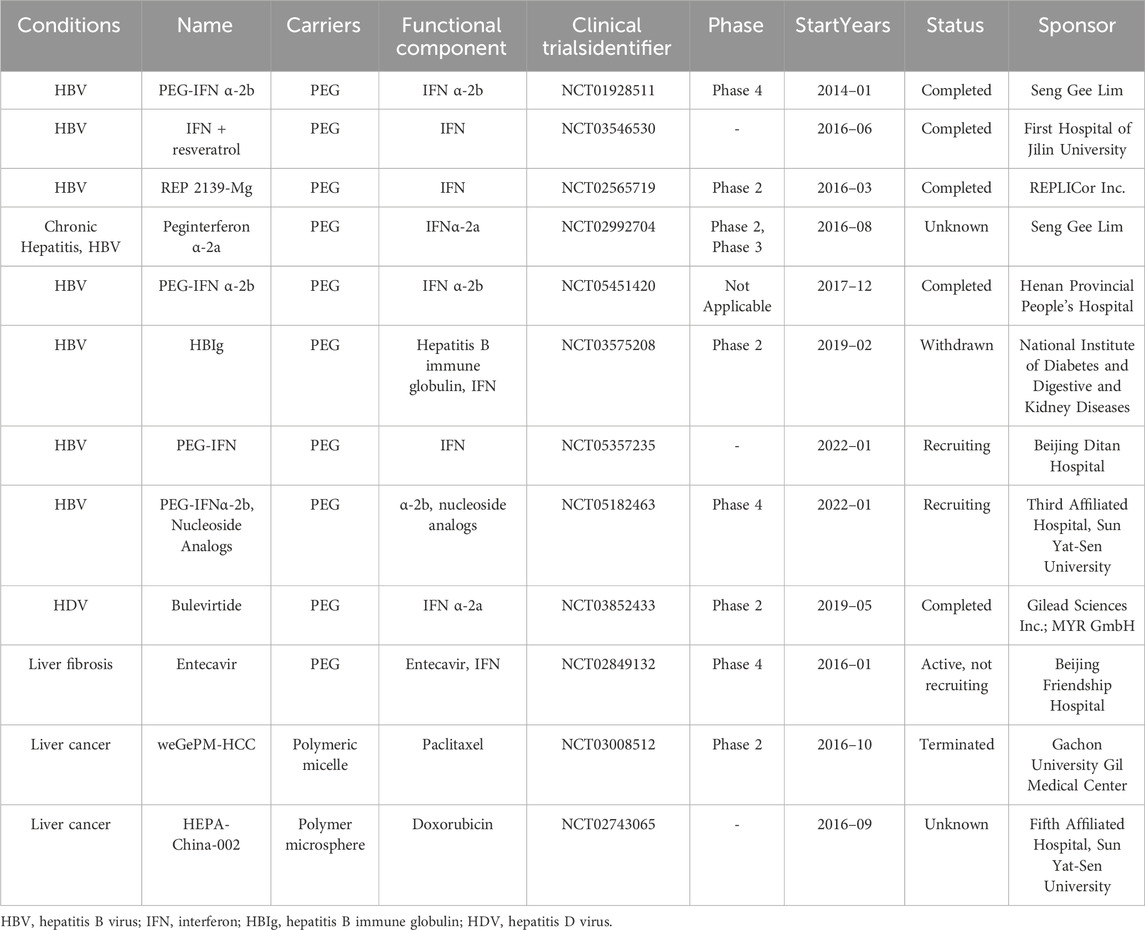
Table 2. List of clinical trials of polymers for hepatic diseases (clinicaltrials.gov).
In conclusion, this review discussed the advantages of natural polymers and synthetic polymers as drug delivery systems. Furthermore, the paper focused specifically on CS, HA, PEG and PLGA in the treatment of hepatitis (particularly viral hepatitis), liver fibrosis, and liver cancer, with the aim of exploring new strategies for the treatment of hepatic diseases. It also discussed various issues and future research directions. Despite the progress made in this field, significant challenges remain. Addressing these issues through targeted research is crucial for developing more cost-effective and practical nanomaterials that can enhance the clinical implementation of treatments for hepatic diseases. By focusing on these advancements, future studies can contribute to improved patient outcomes and more effective therapeutic options.
Author contributions
FG: Writing–original draft, Writing–review and editing. XF: Writing–original draft, Writing–review and editing. XL: Writing–original draft, Writing–review and editing.
Funding
The author(s) declare that no financial support was received for the research, authorship, and/or publication of this article.
Conflict of interest
The authors declare that the research was conducted in the absence of any commercial or financial relationships that could be construed as a potential conflict of interest.
Generative AI statement
The authors declare that no Generative AI was used in the creation of this manuscript.
Publisher’s note
All claims expressed in this article are solely those of the authors and do not necessarily represent those of their affiliated organizations, or those of the publisher, the editors and the reviewers. Any product that may be evaluated in this article, or claim that may be made by its manufacturer, is not guaranteed or endorsed by the publisher.
References
Abd Ellah Noura, H. T. H. M., John, J., and Hetta Helal, F. (2018). Nanomedicine as a future therapeutic approach for Hepatitis C virus. Nanomedicine 14 (11), 1471–1491. doi:10.2217/nnm-2018-0348
Acar, H., Sorgun, O., Yurtseve, G., Bora, E. S., and Erbas, O. (2022). Antifibrotic preventive effect of polyethylene glycol (PEG) 3350 in methotrexateinduced hepatoxicity model. Acta Cir. Bras. 37 (5), e370507–e370508. doi:10.1590/acb370507
Ahmadian, E., Dizaj, S. M., Eftekhari, A., Dalir, E., Samiei, M., Hasanzadeh, A., et al. (2019). The potential applications of hyaluronic acid hydrogels in biomedicine. Drug Res. 70 (1), 6–11. doi:10.1055/a-0991-7585
Albalawi, F., Hussein, M. Z., Fakurazi, S., and Masarudin, M. J. (2023). Fabrication and characterization of nanodelivery platform based on chitosan to improve the anticancer outcome of sorafenib in hepatocellular carcinoma. Sci. Rep. 13 (1), 12180. doi:10.1038/s41598-023-38054-4
AlKandari, F. M., Mohamed, H. S., Ahmed, S. A., Mahmoud, B., and Mahmoud, A. M. (2024). Protective effects of propolis and chitosan nanoparticles against ibuprofen-induced hepatotoxicity in albino rats. Diseases 12 (3), 49. doi:10.3390/diseases12030049
Allahyari, M., and Mohit, E. (2016). Peptide/protein vaccine delivery system based on PLGA particles. Hum. Vaccin. Immunother. 12 (3), 806–828. doi:10.1080/21645515.2015.1102804
Alshetaili, A., Ali, R., Qamar, W., Almohizea, S., and Anwer, M. (2023). Preparation, optimization, and characterization of chrysin-loaded TPGS-b-PCL micelles and assessment of their cytotoxic potential in human liver cancer (Hep G2) cell lines. Int. J. Biol. Macromol. 246, 125679. doi:10.1016/j.ijbiomac.2023.125679
Alsulays, B. B., Aodah, A. H., Ahmed, M. M., and Anwer, M. K. (2024). Preparation and evaluation of chitosan coated PLGA nanoparticles encapsulating ivosidenib with enhanced cytotoxicity against human liver cancer cells. Int. J. Nanomedicine 19, 3461–3473. doi:10.2147/IJN.S452989
Ansar, K., Bahman, Y., Farzin, S., Farideh, F., Hossein, N., and Hadi, P. (2021). Synthesis of biocompatible nanocrystalline cellulose against folate receptors as a novel carrier for targeted delivery of doxorubicin. Chem. Biol. Interact. 351 (0), 109731. doi:10.1016/j.cbi.2021.109731
Antonino, R. D. Q., Fook, B. R. P. L., Lima, V. A. d.O., Rached, R. C. D. F., Fook, M. V. L., da Silva Lima, R. J., et al. (2017). Preparation and characterization of chitosan obtained from shells of shrimp (Litopenaeus vannamei boone). Mar. Drugs 15 (5), 141. doi:10.3390/md15050141
Arib, C., Liu, H., Liu, Q., Cieutat, A. M., Paleni, D., Li, X., et al. (2021). A pegylated flavin adenine dinucleotide PEG complex to boost immunogenic and therapeutic effects in a liver cancer model. Nanotheranostics 5 (4), 405–416. doi:10.7150/ntno.59290
Aydın, M. M., and Akçalı, K. C. (2018). Liver fibrosis. Turk. J. Gastroenterol. 29 (1), 14–21. doi:10.5152/tjg.2018.17330
Azzam, M., El Safy, S., Abdelgelil, S. A., Weiskirchen, R., Asimakopoulou, A., de Lorenzi, F., et al. (2020). Targeting activated hepatic stellate cells using collagen-binding chitosan nanoparticles for siRNA delivery to fibrotic livers. Pharmaceutics 12 (6), 590. doi:10.3390/pharmaceutics12060590
Bailey, J. S., Eric D, S., and Suzanne, W. (2023). Mechanism of D-alanine transfer to teichoic acids shows how bacteria acylate cell envelope polymers. Nat. Microbiol. 8 (7), 1318–1329. doi:10.1038/s41564-023-01411-0
Bate, T., Shanahan, W., Casillo, J., Grant, R., Forbes, S., and Callanan, A. (2022). Rat liver ECM incorporated into electrospun polycaprolactone scaffolds as a platform for hepatocyte culture. J. Biomed. Mat. Res. 110 (12), 2612–2623. doi:10.1002/jbm.b.35115
Beach, M., Nayanathara, U., Gao, Y., Zhang, C., Xiong, Y., Wang, Y., et al. (2024). Polymeric nanoparticles for drug delivery. Chem. Rev. 124 (9), 5505–5616. doi:10.1021/acs.chemrev.3c00705
Bento, D., Jesus, S., Lebre, F., Goncalves, T., and Borges, O. (2019). Chitosan plus compound 48/80: formulation and preliminary evaluation as a hepatitis B vaccine adjuvant. Pharmaceutics 11 (2), 72. doi:10.3390/pharmaceutics11020072
Bertolini, A., Bloks, V. W., Wilmink, M., Bos, E., van de Peppel, I. P., Eilers, R., et al. (2024). Treatment of intestinal and liver features in cystic fibrosis mice by the osmotic laxative polyethylene glycol. J. Cyst. Fibros. 23 (3), 461–473. doi:10.1016/j.jcf.2023.09.015
Bolhassani, A., Javanzad, S., Saleh, T., Hashemi, M., Aghasadeghi, M. R., and Sadat, S. M. (2014). Polymeric nanoparticles: potent vectors for vaccine delivery targeting cancer and infectious diseases. Hum. Vaccin. Immunother. 10 (2), 321–332. doi:10.4161/hv.26796
Bouissou, C., Rouse, J. J., Price, R., and Walle, C. F. V. D. (2006). The influence of surfactant on PLGA microsphere glass transition and water sorption: remodeling the surface morphology to attenuate the burst release. Pharm. Res. 23 (6), 1295–1305. doi:10.1007/s11095-006-0180-2
Cabral, D. G., Lima, E. C., Moura, P., and Dutra, R. F. (2016). A label-free electrochemical immunosensor for hepatitis B based on hyaluronic acid-carbon nanotube hybrid film. Talanta 148, 209–215. doi:10.1016/j.talanta.2015.10.083
Cai, Q., Jiang, J., Zhang, H., Ge, P., Yang, L., and Zhu, W. (2021). Reduction-responsive anticancer nanodrug using a full poly(ethylene glycol) carrier. ACS Appl. Mater Interfaces 13 (16), 19387–19397. doi:10.1021/acsami.1c04648
Caputo, T. M., Cusano, A. M., Principe, S., Cicatiello, P., Celetti, G., Aliberti, A., et al. (2023). Sorafenib-loaded PLGA carriers for enhanced drug delivery and cellular uptake in liver cancer cells. Int. J. Nanomedicine 18, 4121–4142. doi:10.2147/IJN.S415968
Cassandra, L. R., David, M. K., Lexie, R. C., Connor, J. S., Aiyana, G. F., and David, T. C. (2022). Viscoelastic properties of bioprinted alginate microbeads compared to their bulk hydrogel analogs. J. Biomech. Eng. 145 (3), 1–11. doi:10.1115/1.4055757
Chen, S., Ouyang, H., He, D., Liu, D., Wang, X., Chen, H., et al. (2022). Functionalized PAMAM-based nanoformulation for targeted delivery of 5-fluorouracil in hepatocellular carcinoma. Curr. Pharm. Des. 28 (25), 2113–2125. doi:10.2174/1381612828666220506111918
Chen, S., Zhou, J., Wu, X., Meng, T., Wang, B., Liu, H., et al. (2021). Comparison of fibrosis regression of entecavir alone or combined with pegylated interferon alpha2a in patients with chronic hepatitis B. Hepatol. Int. 15 (3), 611–620. doi:10.1007/s12072-021-10162-1
Chen, Y. N., Hsu, S. L., Liao, M. Y., Liu, Y. T., Lai, C. H., Chen, J. F., et al. (2016a). Ameliorative effect of curcumin-encapsulated hyaluronic acid-PLA nanoparticles on thioacetamide-induced murine hepatic fibrosis. Int. J. Environ. Res. Public Health 14 (1), 11–16. doi:10.3390/ijerph14010011
Chen, Z., He, N., Chen, M., Zhao, L., and Li, X. (2016b). Tunable conjugation densities of camptothecin on hyaluronic acid for tumor targeting and reduction-triggered release. Acta Biomater. 43, 195–207. doi:10.1016/j.actbio.2016.07.020
Cheng, F., Chen, Y., Zhan, Z., Liu, Y., Hu, P., Ren, H., et al. (2018). Curc-mPEG454, a PEGylated curcumin derivative, improves anti-inflammatory and antioxidant activities: a comparative study. Inflammation 41 (2), 579–594. doi:10.1007/s10753-017-0714-2
Choi, J. W., Lee, S. Y., Cho, E. J., Jeong, D. I., Kim, D. D., Kim, H. C., et al. (2021). Gas generating microspheres for immediate release of Hsp90 inhibitor aiming at postembolization hypoxia in transarterial chemoembolization therapy of hepatocellular carcinoma. Int. J. Pharm. 607, 120988. doi:10.1016/j.ijpharm.2021.120988
Cui, J., Alt, K., Ju, Y., Gunawan, S. T., Braunger, J. A., Wang, T. Y., et al. (2019). Ligand-functionalized poly(ethylene glycol) particles for tumor targeting and intracellular uptake. Biomacromolecules 20 (9), 3592–3600. doi:10.1021/acs.biomac.9b00925
Dai, X., He, J., Zhang, R., Wu, G., Xiong, F., and Zhao, B. (2017). Co-delivery of polyinosinic:polycytidylic acid and flagellin by poly(lactic-co-glycolic acid) MPs synergistically enhances immune response elicited by intranasally delivered hepatitis B surface antigen. Int. J. Nanomedicine 12, 6617–6632. doi:10.2147/IJN.S146912
Darguzyte, M., Rama, E., Rix, A., Baier, J., Hermann, J., Rezvantalab, S., et al. (2024). Riboflavin-targeted polymers improve tolerance of paclitaxel while maintaining therapeutic efficacy. Nanomedicine 58, 102751–102765. doi:10.1016/j.nano.2024.102751
De Mattos, A., Bombassaro, I., Vogel, A., and Debes, J. (2024). Hepatocellular carcinoma-the role of the underlying liver disease in clinical practice. World J. Gastroenterol. 30 (19), 2488–2495. doi:10.3748/wjg.v30.i19.2488
Dewangan, H. K., Singh, S., Maurya, L., and Srivastava, A. (2018). Hepatitis B antigen loaded biodegradable polymeric nanoparticles: formulation optimization and in-vivo immunization in BALB/c mice. Curr. Drug Deliv. 15 (8), 1204–1215. doi:10.2174/1567201815666180604110457
Dolen, Y., Valente, M., Tagit, O., Jager, E., Van Dinther, E. A. W., van Riessen, N. K., et al. (2020). Nanovaccine administration route is critical to obtain pertinent iNKt cell help for robust anti-tumor T and B cell responses. Oncoimmunology 9 (1), 1738813–1738814. doi:10.1080/2162402X.2020.1738813
Dong, H., Han, X., Hao, M., Yang, Q., Lyu, Q., Tang, D., et al. (2023). Nanodrug rescues liver fibrosis via synergistic therapy with H(2)O(2) depletion and Saikosaponin b1 sustained release. Commun. Biol. 6 (1), 184. doi:10.1038/s42003-023-04473-2
D’souza, A. A., and Shegokar, R. (2016). Polyethylene glycol (PEG): a versatile polymer for pharmaceutical applications. Expert Opin. Drug Deliv. 13 (9), 1257–1275. doi:10.1080/17425247.2016.1182485
Elmorsy, E. A., Saber, S., Kira, A. Y., Alghasham, A., Abdel-Hamed, M. R., Amer, M. M., et al. (2024). Hedgehog signaling is a promising target for the treatment of hepatic fibrogenesis: a new management strategy using itraconazole-loaded nanoparticles. Front. Pharmacol. 15, 1377980. doi:10.3389/fphar.2024.1377980
El-Safy, S., Tammam, S. N., Abdel-Halim, M., Ali, M. E., Youshia, J., Shetab Boushehri, M. A., et al. (2020). Collagenase loaded chitosan nanoparticles for digestion of the collagenous scar in liver fibrosis: the effect of chitosan intrinsic collagen binding on the success of targeting. Eur. J. Pharm. Biopharm. 148, 54–66. doi:10.1016/j.ejpb.2020.01.003
Elzoheiry, A., Ayad, E., Omar, N., Elbakry, K., and Hyder, A. (2022). Anti-liver fibrosis activity of curcumin/chitosan-coated green silver nanoparticles. Sci. Rep. 12 (1), 18403. doi:10.1038/s41598-022-23276-9
Essa, D., Kondiah, P. P. D., Choonara, Y. E., and Pillay, V. (2020). The design of poly(lactide-co-glycolide) nanocarriers for medical applications. Front. Bioeng. Biotechnol. 8, 48–20. doi:10.3389/fbioe.2020.00048
Etzion, O., Hamid, S., Lurie, Y., Gane, E. J., Yardeni, D., Duehren, S., et al. (2023). Treatment of chronic hepatitis D with peginterferon lambda-the phase 2 LIMT-1 clinical trial. Hepatology 77 (6), 2093–2103. doi:10.1097/HEP.0000000000000309
Ezhilarasan, D. (2021). Advantages and challenges in nanomedicines for chronic liver diseases: a hepatologist's perspectives. Eur. J. Pharmacol. 893, 173832. doi:10.1016/j.ejphar.2020.173832
Fan, Q. Q., Zhang, C. L., Qiao, J. B., Cui, P. F., Xing, L., Oh, Y. K., et al. (2020). Extracellular matrix-penetrating nanodrill micelles for liver fibrosis therapy. Biomaterials 230, 119616–119640. doi:10.1016/j.biomaterials.2019.119616
Fatouh, A. M., Elshafeey, A. H., and Abdelbary, A. (2021). Galactosylated chitosan coated liposomes of ledipasvir for liver targeting: chemical synthesis, statistical optimization, in-vitro and in-vivo evaluation. J. Pharm. Sci. 110 (3), 1148–1159. doi:10.1016/j.xphs.2020.10.002
Feng, A., Xi, L., Yan, T., Zebo, J., Fan, Y., Jingpei, G., et al. (2024). STING agonist-based hydrogel enhances immune activation in synergy with radiofrequency ablation for hepatocellular carcinoma treatment. J. Control. Release 369, 296–308. doi:10.1016/j.jconrel.2024.01.048
Fernandez-Marino, I., Anfray, C., Crecente-Campo, J., Maeda, A., Ummarino, A., Teijeiro-Valino, C., et al. (2023). Mannose-modified hyaluronic acid nanocapsules for the targeting of tumor-associated macrophages. Drug Deliv. Transl. Res. 13 (7), 1896–1911. doi:10.1007/s13346-022-01265-9
Galina, S., Slavena, D., and Petar D, P. (2024). Natural and synthetic polymers for biomedical and environmental applications. Polym. (Basel) 16 (8), 1159. doi:10.3390/polym16081159
Gao, L., Li, Q., Zhang, J., Huang, Y., Deng, L., Li, C., et al. (2019). Local penetration of doxorubicin via intrahepatic implantation of PLGA based doxorubicin-loaded implants. Drug Deliv. 26 (1), 1049–1057. doi:10.1080/10717544.2019.1676842
Ghosh, A., Ghosh, A. K., Zaman, A., and Das, P. K. (2023). Metformin-loaded hyaluronic acid-derived carbon dots for targeted therapy against hepatocellular carcinoma by glutamine metabolic reprogramming. Mol. Pharm. 20 (12), 6391–6406. doi:10.1021/acs.molpharmaceut.3c00772
Giammaria, F., Donatella, S., Riccardo, C., Luca, M., Cinzia, M., Roberto, C., et al. (2019). A review discussing the use of polyethylene glycol microspheres in the treatment of hepatocellular carcinoma. Future Oncol. 15 (7), 695–704. doi:10.2217/fon-2018-0425
Gong, L., Zhou, H., Zhang, Y., Wang, C., Fu, K., Ma, C., et al. (2023). Preparation of Phillygenin-Hyaluronic acid composite milk-derived exosomes and its anti-hepatic fibrosis effect. Mater Today Bio 23, 100804. doi:10.1016/j.mtbio.2023.100804
GutAyoub, M. M., Elantouny, N. G., El-Nahas, H. M., and Ghazy, F. E. S. (2018). Injectable PLGA Adefovir microspheres; the way for long term therapy of chronic hepatitis-B. Eur. J. Pharm. Sci. 118, 24–31. doi:10.1016/j.ejps.2018.03.016
Haesun, P., Andrew, O., and Kinam, P. (2022). Evolution of drug delivery systems: from 1950 to 2020 and beyond. J. Control. Release 342, 53–65. doi:10.1016/j.jconrel.2021.12.030
Haigang, W., Qiuju, H., Huajun, Z., Xu, D., and Zhang, J. (2018). Single dose HBsAg CS-γ-PGA nanogels induce potent protective immune responses against HBV infection. Eur. J. Pharm. Biopharm. 124, 82–88. doi:10.1016/j.ejpb.2017.12.003
Han, L., Wang, Y., Huang, X., Liu, B., Hu, L., Ma, C., et al. (2008). A stage-specific cancer chemotherapy strategy through flexible combination of reduction-activated charge-conversional core-shell nanoparticles. Theranostics 9 (22), 6532–6549. doi:10.7150/thno.35057
Han, S., Bi, S., Guo, T., Sun, D., Zou, Y., Wang, L., et al. (2022). Nano co-delivery of Plumbagin and Dihydrotanshinone I reverses immunosuppressive TME of liver cancer. J. Control. Release 348, 250–263. doi:10.1016/j.jconrel.2022.05.057
Hanafy, N. A. N., Sheashaa, R. F., Moussa, E. A., and Mahfouz, M. E. (2023). Potential of curcumin and niacin-loaded targeted chitosan coated liposomes to activate autophagy in hepatocellular carcinoma cells: an in vitro evaluation in HePG2 cell line. Int. J. Biol. Macromol. 245, 125572. doi:10.1016/j.ijbiomac.2023.125572
Harakeh, S., Saber, S. H., Al-Raddadi, R., Alamri, T., Al-Jaouni, S., Qari, M., et al. (2023). Novel curcumin nanoformulation induces apoptosis, and reduces migration and angiogenesis in liver cancer cells. Artif. Cells Nanomed Biotechnol. 51 (1), 361–370. doi:10.1080/21691401.2023.2238756
Ho, D., Wang, P., and Kee, T. (2019). Artificial intelligence in nanomedicine. Nanoscale Horiz. 4 (2), 365–377. doi:10.1039/c8nh00233a
Hong, S. C., Yoo, S. Y., Kim, H., and Lee, J. (2017). Chitosan-based multifunctional platforms for local delivery of therapeutics. Mar. Drugs 15 (3), 60. doi:10.3390/md15030060
Hong, Y., Mao, D., Wu, R., Gao, Z., Meng, T., Wang, R., et al. (2019). Hepatitis B virus S gene therapy with 10-23 DNAzyme delivered by chitosan-g-stearic acid micelles. RSC Adv. 9 (27), 15196–15204. doi:10.1039/c9ra00330d
Hooda, P., Al-Dosari, M., Sinha, N., Parvez, M. K., and Sehgal, D. (2023). Inhibition of HEV replication by FDA-approved RdRp inhibitors. ACS Omega 8 (44), 41570–41578. doi:10.1021/acsomega.3c05637
Hu, Q., Su, Y., Ma, S., Wei, P., He, C., Yang, D., et al. (2024a). Integrin-targeted theranostic nanoparticles for clinical MRI-traceable treatment of liver fibrosis. ACS Appl. Mater Interfaces 16 (2), 2012–2026. doi:10.1021/acsami.3c12776
Hu, Y., Nie, Q., Cong, X., Wu, W., Wu, Q., Liu, Q., et al. (2024b). PEN-coated superparamagnetic iron-mediated delivery of siSnail2 to inhibit metastasis and promote ferroptosis in the treatment of cancer. Int. J. Pharm. 650, 123728. doi:10.1016/j.ijpharm.2023.123728
Huang, L., Zhang, H., Kang, X., Chen, Z., Wang, L., and Zeng, Y. (2023a). Efficacy of pegylated interferon alpha-2b plus entecavir therapy and predictors of treatment success in children with chronic hepatitis B. Front. Immunol. 14, 1–8. doi:10.3389/fimmu.2023.1282922
Huang, M., Liu, J., Fan, Y., Sun, J., Cheng, J. X., Zhang, X. F., et al. (2023b). Development of curcumin-loaded galactosylated chitosan-coated nanoparticles for targeted delivery of hepatocellular carcinoma. Int. J. Biol. Macromol. 253 (6), 127219–127231. doi:10.1016/j.ijbiomac.2023.127219
Huang, Y., Wu, S., Li, J., He, C., Cheng, Y., Li, N., et al. (2024). Self-amplified pH/ROS dual-responsive Co-delivery nano-system with chemo-photodynamic combination therapy in hepatic carcinoma treatment. Int. J. Nanomedicine 19, 3737–3751. doi:10.2147/IJN.S453199
Huang, Y. W., Qin, A., Tsai, C. Y., and Chen, P. J. (2022). Novel pegylated interferon for the treatment of chronic viral hepatitis. Viruses 14 (6), 1128–1142. doi:10.3390/v14061128
Jevsevar, S., Kunstelj, M. I., and Porekar, V. G. (2010). PEGylation of therapeutic proteins. Biotechnol. J. 5 (1), 113–128. doi:10.1002/biot.200900218
Ji, D., Wang, Q., Zhao, Q., Tong, H., Yu, M., Wang, M., et al. (2020). Co-delivery of miR-29b and germacrone based on cyclic RGD-modified nanoparticles for liver fibrosis therapy. J. Nanobiotechnology 18 (1), 86–106. doi:10.1186/s12951-020-00645-y
Jiang, L., Wang, Y., Wei, X., Yang, L., Liu, S., Wang, Y., et al. (2022). Improvement in phenotype homeostasis of macrophages by chitosan nanoparticles and subsequent impacts on liver injury and tumor treatment. Carbohydr. Polym. 277, 118891. doi:10.1016/j.carbpol.2021.118891
Jiang, S., Guo, S., Huang, Y., Yin, Y., Feng, J., Zhou, H., et al. (2024). Predictors of HBsAg seroclearance in patients with chronic HBV infection treated with pegylated interferon-α: a systematic review and meta-analysis. Hepatol. Int. 18 (3), 892–903. doi:10.1007/s12072-024-10648-8
Jiang, Y., Yu, X., Su, C., Zhao, L., and Shi, Y. (2019). Chitosan nanoparticles induced the antitumor effect in hepatocellular carcinoma cells by regulating ROS-mediated mitochondrial damage and endoplasmic reticulum stress. Artif. Cells Nanomed Biotechnol. 47 (1), 747–756. doi:10.1080/21691401.2019.1577876
Jones, D. E., Ghandehari, H., and Facelli, J. C. (2016). A review of the applications of data mining and machine learning for the prediction of biomedical properties of nanoparticles. Comput. Methods Programs Biomed. 132, 93–103. doi:10.1016/j.cmpb.2016.04.025
Kadry, M. O., Abdel-Megeed, R. M., El-Meliegy, E., and Abdel-Hamid, A. Z. (2018). Crosstalk between GSK-3, c-Fos, NFκB and TNF-α signaling pathways play an ambitious role in Chitosan Nanoparticles Cancer Therapy. Toxicol. Rep. 5, 723–727. doi:10.1016/j.toxrep.2018.06.002
Kang, H., Rho, S., Stiles, W. R., Hu, S., Baek, Y., Hwang, D. W., et al. (2020). Size-dependent EPR effect of polymeric nanoparticles on tumor targeting. Adv. Healthc. Mater 9 (1), 1–15. doi:10.1002/adhm.201901223
Kaps, L., Nuhn, L., Aslam, M., Brose, A., Foerster, F., Rosigkeit, S., et al. (2015). In vivo gene-silencing in fibrotic liver by siRNA-loaded cationic nanohydrogel particles. Adv. Healthc. Mater 4 (18), 2809–2815. doi:10.1002/adhm.201500826
Kenward, J., Nathaniel, C., Edgar, H. H. W., and Cyrille, B. (2021). Bioactive synthetic polymers. Adv. Mat. 34 (2), 1–28. doi:10.1002/adma.202105063
Kim, J., and Seki, E. (2023). Hyaluronan in liver fibrosis: basic mechanisms, clinical implications, and therapeutic targets. Hepatol. Commun. 7 (4), 1–11. doi:10.1097/HC9.0000000000000083
Koh, C., Da, B. L., and Glenn, J. S. (2019). HBV/HDV coinfection: a challenge for therapeutics. Clin. Liver Dis. 23 (3), 557–572. doi:10.1016/j.cld.2019.04.005
Kumar, P., Gautam, A. K., Kumar, U., Bhadauria, A. S., Singh, A. K., Kumar, D., et al. (2022). Mechanistic exploration of the activities of poly(lactic-co-glycolic acid)-loaded nanoparticles of betulinic acid against hepatocellular carcinoma at cellular and molecular levels. Arch. Physiol. Biochem. 128 (3), 836–848. doi:10.1080/13813455.2020.1733024
Kurniawan, D. W., Jajoriya, A. K., Dhawan, G., Mishra, D., Argemi, J., Bataller, R., et al. (2018). Therapeutic inhibition of spleen tyrosine kinase in inflammatory macrophages using PLGA nanoparticles for the treatment of non-alcoholic steatohepatitis. J. Control. Release 288, 227–238. doi:10.1016/j.jconrel.2018.09.004
Lee, S. Y., Choi, J. W., Lee, J. Y., Kim, D. D., Kim, H. C., and Cho, H. J. (2018). Hyaluronic acid/doxorubicin nanoassembly-releasing microspheres for the transarterial chemoembolization of a liver tumor. Drug Deliv. 25 (1), 1472–1483. doi:10.1080/10717544.2018.1480673
Leora, G.-H., Dake, H., and Aijun, W. (2019). Developing regenerative treatments for developmental defects, injuries, and diseases using extracellular matrix collagen-targeting peptides. Int. J. Mol. Sci. 20 (17), 4072. doi:10.3390/ijms20174072
Li, B., Zhang, X. Y., Yang, J. Z., Zhang, Y. J., Li, W. X., Fan, C. H., et al. (2014). Influence of polyethylene glycol coating on biodistribution and toxicity of nanoscale graphene oxide in mice after intravenous injection. Int. J. Nanomedicine 9, 4697–4707. doi:10.2147/IJN.S66591
Li, D., Liu, S., Zhu, J., Shen, L., Zhang, Q. Y., and Zhu, H. (2021a). Folic acid modified TPGS as a novel nano-micelle for delivery of nitidine chloride to improve apoptosis induction in Huh7 human hepatocellular carcinoma. BMC Pharmacol. Toxicol. 22 (1), 1–11. doi:10.1186/s40360-020-00461-y
Li, F., Cheng, Z., Sun, J., Cheng, X., Li, C., Wu, Z., et al. (2023). The combination of sinusoidal perfusion enhancement and apoptosis inhibition by riociguat plus a galactose-PEGylated bilirubin multiplexing nanomedicine ameliorates liver fibrosis progression. Nano Lett. 23 (10), 4126–4135. doi:10.1021/acs.nanolett.2c04726
Li, J., Liu, W., Li, Z., Hu, Y., Yang, J., and Li, J. (2021b). PEGylated AIEgen molecular probe for hypoxia-mediated tumor imaging and photodynamic therapy. Chem. Commun. (Camb.) 57 (38), 4710–4713. doi:10.1039/d1cc00967b
Li, L., Liang, N., Wang, D., Yan, P., Kawashima, Y., Cui, F., et al. (2018). Amphiphilic polymeric micelles based on deoxycholic acid and folic acid modified chitosan for the delivery of paclitaxel. Int. J. Mol. Sci. 19 (10), 3132. doi:10.3390/ijms19103132
Li, R., Li, Z., Feng, Y., Yang, H., Shi, Q., Tao, Z., et al. (2020a). PDGFRβ-targeted TRAIL specifically induces apoptosis of activated hepatic stellate cells and ameliorates liver fibrosis. Apoptosis 25 (1-2), 105–119. doi:10.1007/s10495-019-01583-3
Li, W., Zhou, C., Fu, Y., Chen, T., Liu, X., Zhang, Z., et al. (2020b). Targeted delivery of hyaluronic acid nanomicelles to hepatic stellate cells in hepatic fibrosis rats. Acta Pharm. Sin. B 10 (4), 693–710. doi:10.1016/j.apsb.2019.07.003
Li, X., Wang, X., Jiao, Q., Shao, Y., Luo, R., Zhang, J., et al. (2023). Selective inhibition of glycolysis in hepatic stellate cells and suppression of liver fibrogenesis with vitamin A-derivative decorated camptothecin micelles. Acta Biomater. 168, 497–514. doi:10.1016/j.actbio.2023.07.035
Li, Z., Lan, J., Wu, Y., Ding, Y., and Zhang, T. (2024). Homotypic cell membrane-camouflaged biomimetic PLGA nanoparticle loading triptolide for the treatment of hepatocellular carcinoma. Drug Deliv. 31 (1), 2354687. doi:10.1080/10717544.2024.2354687
Liang, Y., Wang, B., Chen, Q., Fu, X., Jiang, C., Lin, Z., et al. (2023). Systemic delivery of glycosylated-PEG-masked oncolytic virus enhances targeting of antitumor immuno-virotherapy and modulates T and NK cell infiltration. Theranostics 13 (15), 5452–5468. doi:10.7150/thno.87498
Liao, K., Chen, S., Yang, G., Huang, X., Wang, T., Long, S., et al. (2024). Black phosphorus-based nanoparticles induce liver cancer cell mitochondrial apoptosis and immune cell tumor infiltration for enhancing dendritic cell therapy. Heliyon 10 (5), e27234. doi:10.1016/j.heliyon.2024.e27234
Lin, S., Jing, H., Du, X., Yang, X., and Wang, J. (2024). Optimization of lipid assisted polymeric nanoparticles for siRNA delivery and cancer immunotherapy. Biomater. Sci. 12 (8), 2057–2066. doi:10.1039/d3bm02071a
Liu, Y., Kong, T., Yang, Z., Zhang, Y., Lei, J., and Zhao, P. (2021). Self-assembled folic acid-targeted pectin-multi-arm polyethylene glycol nanoparticles for tumor intracellular chemotherapy. ACS Omega 6 (2), 1223–1234. doi:10.1021/acsomega.0c04350
Lucatelli, P., Ginnani Corradini, L., De Rubeis, G., Rocco, B., Basilico, F., Cannavale, A., et al. (2019). Balloon-occluded transcatheter arterial chemoembolization (b-TACE) for hepatocellular carcinoma performed with polyethylene-glycol epirubicin-loaded drug-eluting embolics: safety and preliminary results. Cardiovasc. Interv. Radiol. 42 (6), 853–862. doi:10.1007/s00270-019-02192-y
Luo, K., Yin, S., Zhang, R., Yu, H., Wang, G., and Li, J. (2020). Multifunctional composite nanoparticles based on hyaluronic acid-paclitaxel conjugates for enhanced cancer therapy. Int. J. Pharm. 589, 119870. doi:10.1016/j.ijpharm.2020.119870
Maeyama, J. I., Kurata-Iesato, Y., Isaka, M., Komiya, T., and Sakurai, S. (2020). Induction of antibody responses in mice immunized intranasally with Type I interferon as adjuvant and synergistic effect of chitosan. Microbiol. Immunol. 64 (9), 610–619. doi:10.1111/1348-0421.12832
Makadia, H. K., and Siegel, S. J. (2011). Poly lactic-co-glycolic acid (PLGA) as biodegradable controlled drug delivery carrier. Polym. (Basel) 3 (3), 1377–1397. doi:10.3390/polym3031377
Mandal, D., Shaw, T. K., Dey, G., Pal, M. M., Mukherjee, B., Bandyopadhyay, A. K., et al. (2018). Preferential hepatic uptake of paclitaxel-loaded poly-(d-l-lactide-co-glycolide) nanoparticles - a possibility for hepatic drug targeting: pharmacokinetics and biodistribution. Int. J. Biol. Macromol. 112, 818–830. doi:10.1016/j.ijbiomac.2018.02.021
Mardpour, S., Ghanian, M. H., Sadeghi-abandansari, H., Mardpour, S., Nazari, A., Shekari, F., et al. (2019). Hydrogel-mediated sustained systemic delivery of mesenchymal stem cell-derived extracellular vesicles improves hepatic regeneration in chronic liver failure. ACS Appl. Mat. Interfaces 11 (41), 37421–37433. doi:10.1021/acsami.9b10126
Matalqah, S. M., Aiedeh, K., Mhaidat, N. M., Alzoubi, K. H., Bustanji, Y., and Hamad, I. (2020). Chitosan nanoparticles as a novel drug delivery system: a review article. Curr. Drug Targets 21 (15), 1613–1624. doi:10.2174/1389450121666200711172536
Matsumoto, T., Aoki, T., Shimizu, T., Park, K. H., Shiraki, T., Sakuraoka, Y., et al. (2022). Prognostic significance of preoperative hyaluronic acid level in patients with hepatocellular carcinoma. HPB Oxf. 24 (4), 525–534. doi:10.1016/j.hpb.2021.09.001
Mazen, M. E.-H., and José, L. A. (2022). Recent advances in the surface functionalization of PLGA-based nanomedicines. Nanomater. (Basel) 12 (3), 1–34. doi:10.3390/nano12030354
Meng, H., Li, R., Xie, Y., Mo, Z., Zhai, H., Zhang, G., et al. (2023). Nanoparticles mediated circROBO1 silencing to inhibit hepatocellular carcinoma progression by modulating miR-130a-5p/CCNT2 Axis. Int. J. Nanomed. 18, 1677–1693. doi:10.2147/ijn.s399318
Meng, T., Li, Y., Tian, Y., Ma, M., Shi, K., Shang, X., et al. (2022). A hypoxia-sensitive drug delivery system constructed by nitroimidazole and its application in the treatment of hepatocellular carcinoma. AAPS PharmSciTech 23 (6), 167. doi:10.1208/s12249-022-02316-7
Metkar, S. P., Fernandes, G., Nikam, A. N., Soman, S., Birangal, S., Seetharam, R. N., et al. (2023). Mannosylated-chitosan-coated andrographolide nanoliposomes for the treatment of hepatitis: in vitro and in vivo evaluations. Membr. (Basel) 13 (2), 193. doi:10.3390/membranes13020193
Mo, J., Da, X., Li, Q., Huang, J., Lu, L., and Lu, H. (2022). The study of exosomes-encapsulated mPEG-PLGA polymer drug-loaded particles for targeted therapy of liver cancer. J. Oncol. 2022, 4234116. doi:10.1155/2022/4234116
Mohammed, M. M., and Naif, H. M. (2022). Poly(Lactide-co-Glycolide) nanoparticle-mediated vaccine delivery of encapsulated surface antigen protein of hepatitis B virus elicits effective immune response. Viral Immunol. 35 (2), 112–121. doi:10.1089/vim.2021.0058
Mondal, S., Das, S., Mahapatra, P. K., and Saha, K. D. (2022). Morin-VitaminE-β-CyclodextrinInclusionComplexLoadedChitosanNanoparticles (M-Vit.E-CD-CSNPs) ameliorate arsenic-induced hepatotoxicityina murine model. Molecules 27 (18), 5819. doi:10.3390/molecules27185819
Montri, R., Don Haeng, L., Panya, S., and Su-Geun, Y. (2013). Photo-cured PMMA/PEI core/shell nanoparticles surface-modified with Gd-DTPA for T1 MR imaging. J. Colloid Interface Sci. 415 (2014), 70–76. doi:10.1016/j.jcis.2013.09.049
Morán, M. C., Rosell, N., Ruano, G., Busquets, M. A., and Vinardell, M. P. (2015). Gelatin-based nanoparticles as DNA delivery systems: synthesis, physicochemical and biocompatible characterization. Colloids Surf. B. Biointerfaces 134 (0), 156–168. doi:10.1016/j.colsurfb.2015.07.009
Moreira, L., Chagas, A., Ames-Sibin, A., Pateis, V., Gonçalves, O., Silva-Comar, F., et al. (2022). Alpha-tocopherol-loaded polycaprolactone nanoparticles improve the inflammation and systemic oxidative stress of arthritic rats. J. Tradit. Complement. Med. 12 (4), 414–425. doi:10.1016/j.jtcme.2021.12.003
Muthu, M. S., and Feng, S. S. (2009). Pharmaceutical stability aspects of nanomedicines. Nanomedicine 4 (8), 857–860. doi:10.2217/nnm.09.75
Naoki, M., Kenichiro, M., Daisuke, A., Ryo, M., Seishin, T., Taeko, M., et al. (2019). Quantitative evaluation of image recognition performance of fiducial markers in real-time tumor-tracking radiation therapy. Phys. Med. 65 (0), 33–39. doi:10.1016/j.ejmp.2019.08.004
Nicole, P., Patricia, I., and Meritxell, H. (2019). Liver organoids: from basic research to therapeutic applications. Gut 68 (12), 2228–2237. doi:10.1136/gutjnl-2019-319256
Nie, X., Liu, Y., Li, M., Yu, X., Yuan, W., Huang, S., et al. (2020). SP94 peptide-functionalized PEG-PLGA nanoparticle loading with cryptotanshinone for targeting therapy of hepatocellular carcinoma. AAPS PharmSciTech 21 (4), 124. doi:10.1208/s12249-020-01655-7
Nimeet, D., Dhwani, R., Sagar, S., Raghav, G., Pranav, P., Bharathi, K., et al. (2023). Chitosan: a potential biopolymer in drug delivery and biomedical applications. Pharmaceutics 15 (4), 1313. doi:10.3390/pharmaceutics15041313
Nimesh, S., Thibault, M. M., Lavertu, M., and Buschmann, M. D. (2010). Enhanced gene delivery mediated by low molecular weight chitosan/DNA complexes: effect of pH and serum. Mol. Biotechnol. 46 (2), 182–196. doi:10.1007/s12033-010-9286-1
Nisha, R., Kumar, P., Gautam, A. K., Bera, H., Bhattacharya, B., Parashar, P., et al. (2021). Assessments of in vitro and in vivo antineoplastic potentials of β-sitosterol-loaded PEGylated niosomes against hepatocellular carcinoma. J. Liposome Res. 31 (3), 304–315. doi:10.1080/08982104.2020.1820520
Ossipov, D. A. (2019). Hyaluronan-based delivery of therapeutic oligonucleotides for treatment of human diseases. Expert Opin. Drug Deliv. 16 (6), 621–637. doi:10.1080/17425247.2019.1617693
Pan, X., Liu, S., Ju, L., Xi, J., He, R., Zhao, Y., et al. (2020). Preparation, evaluation, and in vitro cytotoxicity studies of artesunate-loaded glycyrrhetinic acid decorated PEG-PLGA nanoparticles. Drug Dev. Ind. Pharm. 46 (11), 1889–1897. doi:10.1080/03639045.2020.1825475
Pan, X. W., Huang, J. S., Liu, S. R., Shao, Y. D., Xi, J. J., He, R. Y., et al. (2023). Evaluation of the liver targeting and anti-liver cancer activity of artesunate-loaded and glycyrrhetinic acid-coated nanoparticles. Exp. Ther. Med. 26 (5), 516–530. doi:10.3892/etm.2023.12215
Paolo, C., and Pietro, M. (2019). Advances in drug delivery and biomaterials: facts and vision. Pharmaceutics 11 (1), 1–44. doi:10.3390/pharmaceutics11010048
Patra, J. K., Das, G., Fraceto, L. F., Campos, E. V. R., Shin, H. S., Acosta-Torres, L. S., et al. (2018). Nano based drug delivery systems: recent developments and future prospects. J. Nanobiotechnol. 16 (1), 71. doi:10.1186/s12951-018-0392-8
Pawlowski, T., Radkowski, M., Perlejewski, K., Laskus, T., and Malyszczak, K. (2024). The severity of depressive symptoms as an independent predictor of sustained virological response during treatment of hepatitis C with pegylated interferon-α2a and oral ribavirin. Psychosom. Med. 86 (2), 124–128. doi:10.1097/PSY.0000000000001274
Pedersen, G., Blaschek, L., Frandsen, K., Noack, L., and Persson, S. (2023). Cellulose synthesis in land plants. Mol. plant 16 (1), 206–231. doi:10.1016/j.molp.2022.12.015
Pei, Q., Yi, Q., and Tang, L. (2023). Liver fibrosis resolution: from molecular mechanisms to therapeutic opportunities. Int. J. Mol. Sci. 24 (11), 9671. doi:10.3390/ijms24119671
Pho-Iam, T., Punnakitikashem, P., Somboonyosdech, C., Sripinitchai, S., Masaratana, P., Sirivatanauksorn, V., et al. (2021). PLGA nanoparticles containing α-fetoprotein siRNA induce apoptosis and enhance the cytotoxic effects of doxorubicin in human liver cancer cell line. Biochem. Biophys. Res. Commun. 553, 191–197. doi:10.1016/j.bbrc.2021.03.086
Piman, P., Kawintra, T., Thanyathanya, R., Karnchanok, K., Tarapong, S., Somporn, K., et al. (2024). Pickering emulsion of oleoresin from dipterocarpus alatus roxb. Ex G. Don and its antiproliferation in colon (HCT116) and liver (HepG2) cancer cells. Molecules 29 (11), 2695. doi:10.3390/molecules29112695
Piotr, R., Ilona, L., Paulina, P., Witold, S., and Beata, K. (2021). Modification of alginates to modulate their physic-chemical properties and obtain biomaterials with different functional properties. Molecules 26 (23), 7264. doi:10.3390/molecules26237264
Powell, L. G., Alexander, C., Stone, V., Johnston, H. J., and Conte, C. (2022). An in vitro investigation of the hepatic toxicity of PEGylated polymeric redox responsive nanoparticles. RSC Adv. 12 (20), 12860–12870. doi:10.1039/d2ra00395c
Qiao, D., Chen, Y., and Liu, L. (2021). Engineered therapeutic nanovaccine against chronic hepatitis B virus infection. Biomaterials 269, 120674. doi:10.1016/j.biomaterials.2021.120674
Qiao, J. B., Fan, Q. Q., Xing, L., Cui, P. F., He, Y. J., Zhu, J. C., et al. (2018). Vitamin A-decorated biocompatible micelles for chemogene therapy of liver fibrosis. J. Control. Release 283, 113–125. doi:10.1016/j.jconrel.2018.05.032
Qin, S., Li, J., Pan, Z., Wang, C., and Zhang, B. F. (2023). Targeted paclitaxel prodrug nanoassemblies to improve therapeutic effects for liver cancer. Colloids Surf. B. Biointerfaces 226, 113285–111396. doi:10.1016/j.colsurfb.2023.113285
Rao, N. V., Yoon, H. Y., Han, H. S., Ko, H., Son, S., Lee, M., et al. (2016). Recent developments in hyaluronic acid-based nanomedicine for targeted cancer treatment. Expert Opin. Drug Deliv. 13 (2), 239–252. doi:10.1517/17425247.2016.1112374
Rashki, S., Asgarpour, K., Tarrahimofrad, H., Hashemipour, M., Ebrahimi, M. S., Fathizadeh, H., et al. (2021). Chitosan-based nanoparticles against bacterial infections. Carbohydr. Polym. 251 (2021), 117108–117112. doi:10.1016/j.carbpol.2020.117108
Rizeq, B. R., Younes, N. N., Rasool, K., and Nasrallah, G. K. (2019). Synthesis, bioapplications, and toxicity evaluation of chitosan-based nanoparticles. Int. J. Mol. Sci. 20 (22), 5776. doi:10.3390/ijms20225776
Roopngam, P., Liu, K., Mei, L., Zheng, Y., Zhu, X., Tsai, H. I., et al. (2016). Hepatitis C virus E2 protein encapsulation into poly d, l-lactic-co-glycolide microspheres could induce mice cytotoxic T-cell response. Int. J. Nanomedicine 11, 5361–5370. doi:10.2147/IJN.S109081
Rosli, N. A., Karamanlioglu, M., Kargarzadeh, H., and Ahmad, I. (2021). Comprehensive exploration of natural degradation of poly(lactic acid) blends in various degradation media: a review. Int. J. Biol. Macromol. 187, 732–741. doi:10.1016/j.ijbiomac.2021.07.196
Safer, A. M., Hanafy, N. A., Bharali, D. J., Cui, H., and Mousa, S. A. (2015). Effect of green tea extract encapsulated into chitosan nanoparticles on hepatic fibrosis collagen fibers assessed by atomic force microscopy in rat hepatic fibrosis model. J. Nanosci. Nanotechnol. 15 (9), 6452–6459. doi:10.1166/jnn.2015.10608
Saha, I., and Rai, V. (2021). Hyaluronic acid based microneedle array: recent applications in drug delivery and cosmetology. Carbohydr. Polym. 267, 118168. doi:10.1016/j.carbpol.2021.118168
Salih, A. R. C., Farooqi, H. M. U., Amin, H., Karn, P. R., Meghani, N., and Nagendran, S. (2024). Hyaluronic acid: comprehensive review of a multifunctional biopolymer. Futur. J. Pharm. Sci. 10 (1), 63–14. doi:10.1186/s43094-024-00636-y
Sasaki, D., Kusamori, K., and Nishikawa, M. (2023). Delivery of corn-derived nanoparticles with anticancer activity to tumor tissues by modification with polyethylene glycol for cancer therapy. Pharm. Res. 40 (4), 917–926. doi:10.1007/s11095-022-03431-7
Shabir, H., Gyan, P., Aycabal, O., Saghi, S., Mohammad Farhan, S., Jungmok, S., et al. (2017). Evolution and clinical translation of drug delivery nanomaterials. Nano Today 15 (0), 91–106. doi:10.1016/j.nantod.2017.06.008
Shafie, F., Nabavizadeh, F., Ardestani, M. S., Panahi, M., Ashabi, G., Samandari, H., et al. (2019). Sorafenib loaded PAMAM-dendrimer attenuates liver fibrosis and its complications in bile duct-ligated rats. Can. J. Physiol. Pharmacol. 97 (8), 691–698. doi:10.1139/cjpp-2019-0141
Shao, W., Yang, Y., Shen, W., Ren, L., Wenwen, W., and Zhu, P. (2024). Hyaluronic acid-conjugated methotrexate and 5-fluorouracil for targeted drug delivery. Int. J. Biol. Macromol. 273 (Pt 1), 132671. doi:10.1016/j.ijbiomac.2024.132671
Shepherd, S. J., Issadore, D., and Mitchell, M. J. (2021). Microfluidic formulation of nanoparticles for biomedical applications. Biomaterials 274, 120826. doi:10.1016/j.biomaterials.2021.120826
Shinn, J., Park, S., Lee, S., Park, N., Kim, S., Hwang, S., et al. (2024). Antioxidative hyaluronic acid-bilirubin nanomedicine targeting activated hepatic stellate cells for anti-hepatic-fibrosis therapy. ACS Nano 18 (6), 4704–4716. doi:10.1021/acsnano.3c06107
Siapoush, S., Mousazadeh, H., Rezaei, R., Hatami, B., Mazhari, S., Hashemi, N., et al. (2023). Oral targeted delivery of Imatinib by pH responsive copolymer modulates liver fibrosis in the mice model. Int. J. Pharm. 641, 123068. doi:10.1016/j.ijpharm.2023.123068
Srikar, R., Yarin, A. L., Megaridis, C. M., Bazilevsky, A. V., and Kelley, E. (2007). Desorption-limited mechanism of release from polymer nanofibers. Langmuir 24 (3), 965–974. doi:10.1021/la702449k
Sumeet, K. A., Harshad, D., John, E., and Patrick S, K. (2019). Burden of liver diseases in the world. J. Hepatol. 70 (1), 151–171. doi:10.1016/j.jhep.2018.09.014
Sun, Q., Zhou, Z., Qiu, N., and Shen, Y. (2017). Rational design of cancer nanomedicine: nanoproperty integration and synchronization. Adv. Mat. 29 (14), 1–18. doi:10.1002/adma.201606628
Tacke, F. (2017). Targeting hepatic macrophages to treat liver diseases. J. Hepatol. 66 (6), 1300–1312. doi:10.1016/j.jhep.2017.02.026
Tang, L. S. Y., Covert, E., Wilson, E., and Kottilil, S. (2018). Chronic hepatitis B infection: a review. Jama 319 (17), 1802–1813. doi:10.1001/jama.2018.3795
Tang, X., Li, A., Xie, C., Zhang, Y., Liu, X., Xie, Y., et al. (2020). The PI3K/mTOR dual inhibitor BEZ235 nanoparticles improve radiosensitization of hepatoma cells through apoptosis and regulation DNA repair pathway. Nanoscale Res. Lett. 15 (1), 63–16. doi:10.1186/s11671-020-3289-z
Tapper, E. B., and Parikh, N. D. (2023). Diagnosis and management of cirrhosis and its complications: a review. Jama 329 (18), 1589–1602. doi:10.1001/jama.2023.5997
Thomas, R. G., Moon, M. J., Kim, J. H., Lee, J. H., and Jeong, Y. Y. (2015). Effectiveness of losartan-loaded hyaluronic acid (HA) micelles for the reduction of advanced hepatic fibrosis in C3H/HeN mice model. PLoS One 10 (12), e0145512. doi:10.1371/journal.pone.0145512
Tian, L., Gao, J., Yang, Z., Zhang, Z., and Huang, G. (2018). Tamibarotene-loaded PLGA microspheres for intratumoral injection administration: preparation and evaluation. AAPS PharmSciTech 19 (1), 275–283. doi:10.1208/s12249-017-0827-9
Torrecilla, J., Del Pozo-Rodriguez, A., Solinis, M. A., Apaolaza, P. S., Berzal-Herranz, B., Romero-Lopez, C., et al. (2016). Silencing of hepatitis C virus replication by a non-viral vector based on solid lipid nanoparticles containing a shRNA targeted to the internal ribosome entry site (IRES). Colloids Surf. B. Biointerfaces 146, 808–817. doi:10.1016/j.colsurfb.2016.07.026
Turecek Peter, L., Bossard Mary, J. S. F., and Ivens Inge, A. (2016). PEGylation of biopharmaceuticals: a review of chemistry and nonclinical safety information of approved drugs. J. Pharm. Sci. 105 (2), 460–475. doi:10.1016/j.xphs.2015.11.015
Upadhyay, P., Bhattacharjee, M., Bhattacharya, S., Ahir, M., Adhikary, A., and Patra, P. (2020). Silymarin-loaded, lactobionic acid-conjugated porous PLGA nanoparticles induce apoptosis in liver cancer cells. ACS Appl. Bio Mater 3 (10), 7178–7192. doi:10.1021/acsabm.0c00987
Valencia, P. M., Farokhzad, O. C., Karnik, R., and Langer, R. (2020). Microfluidic technologies for accelerating the clinical translation of nanoparticles. Nat. Nanotechnol. 7 (10), 623–629. doi:10.1038/nnano.2012.168
Veronese, F. M., and Pasut, G. (2005). PEGylation, successful approach to drug delivery. Drug Discov. Today 10 (21), 1451–1458. doi:10.1016/S1359-6446(05)03575-0
Vohra, K., Mehta, M., Garg, V., Dua, K., and Dureja, H. (2021). Formulation, characterisation and in vitro cytotoxic effect of Lens culinarisMedikus seeds extract loaded chitosan microspheres. Curr. Mol. Pharmacol. 14 (3), 448–457. doi:10.2174/1874467214666210210124739
Wang, J., Li, Z., Chen, Y., Luo, N., and He, S. (2024). Gelatin nanocarriers assembled by a self-immolative cross-linker for targeted cancer therapy. Int. J. Biol. Macromol. 268, 131722. doi:10.1016/j.ijbiomac.2024.131722
Wang, J., Zhuo, J., Tao, Y., Xu, S., Chen, Z., Yang, F., et al. (2020a). Salinomycin-loaded small-molecule nanoprodrugs enhance anticancer activity in hepatocellular carcinoma. Int. J. Nanomedicine 15, 6839–6854. doi:10.2147/IJN.S236928
Wang, Q., Kim, So Y., Hirosh, M., Wang, Z., Vijay, P., Matsuda, M., et al. (2020). Oral administration of PEGylated TLR7 ligand ameliorates alcohol-associated liver disease via the induction of IL-22. Proc. Natl. Acad. Sci. U. S. A. 118 (1), 1–11. doi:10.1073/pnas.2020868118
Wang, W., Meng, Q., Li, Q., Liu, J., Zhou, M., Jin, Z., et al. (2020b). Chitosan derivatives and their application in biomedicine. Int. J. Mol. Sci. 21 (2), 487. doi:10.3390/ijms21020487
Wen, C., Wang, Y., Tian, H., Lei, Y., Wang, Z., Cai, D., et al. (2023). Clinical cure induced by pegylated interferon alpha-2b in the advantaged population of chronic hepatitis B virus infection: a retrospective cohort study. Front. Cell Infect. Microbiol. 13, 1–11. doi:10.3389/fcimb.2023.1332232
Wu, J., Huang, J., Kuang, S., Chen, J., Li, X., Chen, B., et al. (2019a). Synergistic MicroRNA therapy in liver fibrotic rat using MRI-visible nanocarrier targeting hepatic stellate cells. Adv. Sci. (Weinh) 6 (5), 1801809. doi:10.1002/advs.201801809
Wu, P., Wang, X., Yin, M., Zhu, W., Chen, Z., Zhang, Y., et al. (2024a). ULK1 mediated autophagy-promoting effects of rutin-loaded chitosan nanoparticles contribute to the activation of NF-κB signaling besides inhibiting EMT in Hep3B hepatoma cells. Int. J. Nanomedicine 19, 4465–4493. doi:10.2147/IJN.S443117
Wu, W., Hu, Q., Wang, M., Shao, S., Zhao, X., Bai, H., et al. (2019b). A PEGylated megamer-based microRNA delivery system activatable by stepwise microenvironment stimulation. Chem. Commun. (Camb.) 55 (63), 9363–9366. doi:10.1039/c9cc03846a
Wu, Y., Fan, Q., Zhou, J., Hu, H., Liao, Z., Tang, X., et al. (2024b). Biomimetic platelet-like nanoparticles enhance targeted hepatocellular carcinoma therapy. Colloids Surf. B. Biointerfaces 240 (2024), 113973. doi:10.1016/j.colsurfb.2024.113973
Wu, Y. J., Wu, Y. C., Chen, I. F., Wu, Y. L., Chuang, C. W., Huang, H. H., et al. (2018). Reparative effects of astaxanthin-hyaluronan nanoaggregates against retrorsine-CCl₄-induced liver fibrosis and necrosis. Molecules 23 (4), 726. doi:10.3390/molecules23040726
Wu, Z. C., Liu, X. Y., Liu, J. Y., Piao, J. S., and Piao, M. G. (2022). Preparation of betulinic acid galactosylated chitosan nanoparticles and their effect on liver fibrosis. Int. J. Nanomed. 17, 4195–4210. doi:10.2147/ijn.s373430
Xiang, L., Wang, X., Shao, Y., Jiao, Q., Cheng, J., Zheng, X., et al. (2023). Folate decoration supports the targeting of camptothecin micelles against activated hepatic stellate cells and the suppression of fibrogenesis. ACS Appl. Mater Interfaces 15 (1), 2030–2042. doi:10.1021/acsami.2c16616
Xiao, S., Deng, Y., Shen, N., Sun, Y., Tang, H., Hu, P., et al. (2021). Curc-mPEG454, a PEGylated curcumin derivative, as a multi-target anti-fibrotic prodrug. Int. Immunopharmacol. 101 (2021), 108166. doi:10.1016/j.intimp.2021.108166
Xiao, Z., Tan, Y., Cai, Y., Huang, J., Wang, X., Li, B., et al. (2023). Nanodrug removes physical barrier to promote T-cell infiltration for enhanced cancer immunotherapy. J. Control. Release 356, 360–372. doi:10.1016/j.jconrel.2023.02.029
Xiaolong, T., Longzhou, C., Amin, L., Shiyu, C., Yinci, Z., Xueke, L., et al. (2018). Anti-GPC3 antibody-modified sorafenib-loaded nanoparticles significantly inhibited HepG2 hepatocellular carcinoma. Drug Deliv. 25 (1), 1484–1494. doi:10.1080/10717544.2018.1477859
Xue, J., Hu, Y., Su, Y., Guo, T., and Li, Y. (2020). Studies on the inhibitory effects of nano-hydroxyapatite-loaded As₂O₃ on hepatoma cells. J. Nanosci. Nanotechnol. 20 (12), 7451–7456. doi:10.1166/jnn.2020.18715
Yang, S., Cai, C., Wang, H., Ma, X., Shao, A., Sheng, J., et al. (2022a). Drug delivery strategy in hepatocellular carcinoma therapy. Cell Commun. Signal. 20 (1), 26. doi:10.1186/s12964-021-00796-x
Yang, Y., Sun, Z., Li, H., Tian, J., Chen, M., and Liu, T. (2022b). Preparation and immune effect of HEV ORF2 P206@PLGA nanoparticles. Nanomater. (Basel) 12 (4), 595. doi:10.3390/nano12040595
Yanjie, S., Jiyoung, S., and Xiao-Ying, Y. (2024). ToF-SIMS evaluation of PEG-related mass peaks and applications in PEG detection in cosmetic products. Sci. Rep. 14 (1), 1–13. doi:10.1038/s41598-024-65504-4
Yao, H., Zhao, J., Wang, Z., Lv, J., Du, G., Jin, Y., et al. (2020). Enhanced anticancer efficacy of cantharidin by mPEG-PLGA micellar encapsulation: an effective strategy for application of a poisonous traditional Chinese medicine. Colloids Surf. B. Biointerfaces 196, 111285. doi:10.1016/j.colsurfb.2020.111285
Yao, Z., Du, M., Wang, Y., Zhu, H., Shu, L., You, X., et al. (2024). Pharmacodynamic study of chitosan in combination with salvianolic acid B in the treatment of CC1(4) -induced liver fibrosis in mice. Clin. Exp. Pharmacol. Physiol. 51 (1), 17–29. doi:10.1111/1440-1681.13828
Yoshizaki, Y., Yamasaki, M., Nagata, T., Suzuki, K., Yamada, R., Kato, T., et al. (2023). Drug delivery with hyaluronic acid-coated polymeric micelles in liver fibrosis therapy. ACS Biomater. Sci. Eng. 9 (6), 3414–3424. doi:10.1021/acsbiomaterials.3c00327
Yu, F., Liu, Z., Feng, J., Man, Y., Zhang, H., Shi, J., et al. (2024). Hyaluronic acid modified extracellular vesicles targeting hepatic stellate cells to attenuate hepatic fibrosis. Eur. J. Pharm. Sci. 198, 106783. doi:10.1016/j.ejps.2024.106783
Yutao, M., Zhihua, L., Yucheng, L., Yao, C., Le, M., Xiaoya, L., et al. (2024). Biodegradable microembolics with nanografted polyanions enable high-efficiency drug loading and sustained deep-tumor drug penetration for locoregional chemoembolization treatment. ACS Nano 18 (28), 18211–18229. doi:10.1021/acsnano.4c00047
Yuxin, H., Yifan, W., Lin, W., Wen, J., and Stefan, W. (2024). Understanding nanoparticle-liver interactions in nanomedicine. Expert Opin. Drug Deliv. 21 (6), 829–843. doi:10.1080/17425247.2024.2375400
Zhang, C., Wang, A., Wang, H., Yan, M., Liang, R., He, X., et al. (2019). Entecavir-loaded poly (lactic-co-glycolic acid) microspheres for long-term therapy of chronic hepatitis-B: preparation and in vitro and in vivo evaluation. Int. J. Pharm. 560, 27–34. doi:10.1016/j.ijpharm.2019.01.052
Zhang, J., Wang, Y., Jiang, Y., Liu, T., Luo, Y., Diao, E., et al. (2018). Enhanced cytotoxic and apoptotic potential in hepatic carcinoma cells of chitosan nanoparticles loaded with ginsenoside compound K. Carbohydr. Polym. 198, 537–545. doi:10.1016/j.carbpol.2018.06.121
Zhao, H., Wang, H., Hu, Y., Xu, D., Yin, C., Han, Q., et al. (2021). Chitosan nanovaccines as efficient carrier adjuvant system for IL-12 with enhanced protection against HBV. Int. J. Nanomedicine 16, 4913–4928. doi:10.2147/IJN.S317113
Zheng, X., Zhu, J., Zheng, C., Tan, Z., Ji, Z., Tao, J., et al. (2023). Dissolving microneedle arrays as a hepatitis B vaccine delivery system adjuvanted by APC-targeted poly (Lactic-co-Glycolic acid) (PLGA) nanoparticles. AAPS PharmSciTech 24 (1), 42–15. doi:10.1208/s12249-022-02473-9
Zhou, J., Chen, Y., Liu, Y., Huang, T., Xing, J., Ge, R., et al. (2024). Electrospun medicated gelatin/polycaprolactone Janus fibers for photothermal-chem combined therapy of liver cancer. Int. J. Biol. Macromol. 269 (0), 132113. doi:10.1016/j.ijbiomac.2024.132113
Keywords: hepatic diseases, liver cancer, nanomaterials, polymeric nanoparticles, drug delivery
Citation: Gao F, Feng X and Li X (2025) Recent advances in polymeric nanoparticles for the treatment of hepatic diseases. Front. Pharmacol. 16:1528752. doi: 10.3389/fphar.2025.1528752
Received: 15 November 2024; Accepted: 08 January 2025;
Published: 24 January 2025.
Edited by:
Jose J. G. Marin, University of Salamanca, SpainReviewed by:
Maitane Asensio, University of Salamanca, SpainOscar Briz, University of Salamanca, Spain
Copyright © 2025 Gao, Feng and Li. This is an open-access article distributed under the terms of the Creative Commons Attribution License (CC BY). The use, distribution or reproduction in other forums is permitted, provided the original author(s) and the copyright owner(s) are credited and that the original publication in this journal is cited, in accordance with accepted academic practice. No use, distribution or reproduction is permitted which does not comply with these terms.
*Correspondence: Xinyu Li, bGl4aW55dTE5ODdAamx1LmVkdS5jbg==