- 1Haihe Laboratory of Modern Chinese Medicine, Tianjin University of Traditional Chinese Medicine, Tianjin, China
- 2Engineering Research Center of Modern Chinese Medicine Discovery and Preparation Technique, Ministry of Education, Tianjin University of Traditional Chinese Medicine, Tianjin, China
- 3State Key Laboratory of Component-Based Chinese Medicine, Tianjin University of Traditional Chinese Medicine, Tianjin, China
- 4College of Chinese Medicine, Tianjin University of Chinese Medicine, Tianjin, China
Blue light induced eye damage (BLED) belongs to modern diseases. It is an ophthalmic disease caused by prolonged exposure to electronic devices or screens containing a large amount of high-energy short waves (blue light). Specific symptoms include dryness and discomfort in the eyes, blurred vision, headache, insomnia, and in severe cases, it may also cause various eye diseases such as cataracts and glaucoma. At present, the development of health products and drugs for eye blue light injury faces many difficulties. Therefore, further exploration and research are needed on the pathogenesis, pathophysiology, and pharmacological mechanisms of blue light injury. Natural medicine ingredients and preparations have unique advantages in targeting eye blue light injury fatigue products due to their multi-component synergistic effects, overall regulation, and mild and safe characteristics. Starting from the disease-related mechanisms and pathophysiological characteristics of eye blue light injury, this article elucidates the pharmacological mechanisms of various drugs for treating eye blue light injury. At the same time, it reviews the research on in vitro cultured cell and animal model conditions for blue light injury eyes, in order to provide reference for subsequent blue light injury modeling experiments. And explore future research directions to provide new ideas and methods for the prevention and treatment of BLED.
1 Introduction
In recent years, with the popularity of electronic devices such as computers and smartphones, people are increasingly exposed to artificial light sources (Ouyang et al., 2020), and prolonged exposure to light has become a major challenge affecting public visual health. The phototoxicity of visible light, especially blue light, has been extensively studied. Research has shown that short wave blue light between 400 and 460 nm is the most harmful (Wang and Li, 2021), as it can induce symptoms such as eye fatigue, dryness, pain, blurred vision, headache, insomnia, etc. Because it can penetrate the cornea and lens, directly reaching the retina, causing irreversible photochemical damage, known as blue light hazard (Kara-Junior et al., 2011; van Norren and Vos, 2016). However, endogenous pretreatment can to some extent alleviate the damage of blue light to biological tissues. It is a natural protective mechanism in organisms, which can reduce the damage of harmful light exposure to photoreceptors and RPE cells by synthesizing photoprotective pigments (such as melanin and lipofuscin) to absorb blue light, activate antioxidant enzymes, neutralize free radicals, etc. The subthreshold micropulse yellow laser (SMYL) treatment effectively treats macular edema by stimulating retinal pigment epithelial (RPE) cells with light, reducing the number of inflammatory cytokines and hyper-reflective retinal spots (HRS) (Bonfiglio et al., 2022).
Blue light damage to the eyes has led to an increase in various ophthalmic diseases such as age-related macular degeneration (AMD) (Jin and Jeong, 2022; Xia et al., 2019), cataracts (Xiang et al., 2020), and keratitis (Li et al., 2020). Epidemiological studies have shown that AMD is a human retinal degenerative disease affecting people aged 55 and above in industrialized countries, and is the main cause of blindness. Studies have shown that long-term accumulation of light exposure (especially blue light) can lead to retinal degeneration, causing damage to RPE cells and photoreceptor cells. AMD is mainly caused by functional impairment and loss of RPE, indicating that blue light is involved in the generation and development of AMD. Among patients aged 75 and above, the risk of early AMD is 25%, and the risk of late AMD is 8%. As the population ages, the absolute number of AMD patients worldwide will increase (Thomas et al., 2021). It is expected that by 2040, there will be 288 million AMD patients worldwide, with Asia having the highest number of disease patients (Keenan et al., 2021).
Many ophthalmic diseases caused by blue light damage can seriously affect people’s quality of life. To further investigate the pathogenesis and treatment methods of blue light damage, Based on the pathogenesis and pathophysiology of eye blue light injury, this article summarizes the pharmacological mechanisms of chemical small molecule drugs, natural drugs, and gene drugs related to the treatment of eye blue light injury. And summarize and organize the modeling conditions of cells and animals damaged by blue light, providing reference for subsequent blue light damage modeling experiments. Simultaneously exploring future research directions in order to lay the foundation for the application and development of natural medicine in the treatment of eye diseases.
2 Pathological characteristics and pathogenesis of blue light damage in the eyes
2.1 Pathophysiological characterization
The pathways of blue light damage mainly include oxidative stress, endoplasmic reticulum stress and oxidative DNA damage, inflammatory response, mitochondrial damage and cell apoptosis, lysosomal autophagy and vascular endothelial damage (Fan et al., 2022; Zhang H. et al., 2023). On the ocular surface, blue light irradiation can induce disordered autophagy levels in corneal stromal cells, affecting ocular surface function (Niwano et al., 2014). High energy and prolonged exposure to blue light can also penetrate the cornea and enter the lens, and the pathogenesis of cataracts may be closely related to oxidative damage to lens epithelial cells (Wang and Li, 2021; Liu, 2019). Blue light enters the retina, stimulating photoreceptors in the retina and photosensitive pigments in pigment epithelial cells, such as rhodopsin in photoreceptors and lipofuscin in pigment epithelial cells, producing a large amount of free radicals and reactive oxygen species (ROS). Lipid peroxidation is caused by ROS, which can lead to oxidative stress. Oxidative stress and photochemical damage can activate the apoptotic signaling pathway within cells, resulting in programmed cell death (Nan et al., 2023; Marie et al., 2018). In addition, the fluorescent group A2E of lipofuscin is activated by blue light, releasing free radicals and causing lipid peroxidation. This not only activates inflammatory reactions but also causes DNA breakage, while inhibiting the normal function of mitochondria and lysosomes (Moreira and Oliveira, 2011; Davies et al., 2001; Alaimo et al., 2019).
According to morphological observations, blue light irradiation can cause congestion and edema of corneal limbal blood vessels, dilation and thinning of the central part of the cornea, protrusion forward, and increased curvature. Long term exposure to blue light can cause the lens to become opaque, and the degree of opacity of the lens increases with prolonged exposure time. After blue light injury, the outer nuclear layer of the retina significantly thins, and due to degeneration and necrosis of some cells, the loss of outer nuclear layer cells decreases. Because ONL belongs to the photoreceptor cell layer, it often experiences a decrease in thickness and a decrease in the number of rod and cone cells when damaged, leading to problems such as decreased visual sensitivity, loss of field of view, and color vision abnormalities (Choi et al., 2016). Due to the release of pro-inflammatory cytokines, the permeability of blood vessels increases, and some harmful components in the blood can seep into the retina, leading to partial cell apoptosis. In addition, nuclear condensation and some irregular nuclei may occur, and fragmented nuclei can be seen (Shang et al., 2014). The pathological changes of blue light damage to the ocular surface, crystalline lens, and retina mentioned above may lead to various eye diseases, such as keratitis, dry eye syndrome, cataracts, myopia, and age-related macular degeneration (Figure 1).
2.2 Pathogenesis
2.2.1 Effects of blue light on cornea and lens
Research has shown that blue light toxicity is not limited to the retina, but can also damage the ocular surface through oxidative stress, inflammatory response, and cell apoptosis, and is associated with the formation of ocular surface inflammation and dry eye syndrome (Lee et al., 2016; Chong, 2022). High energy blue light is close to ultraviolet light in the spectrum, so excessive exposure to blue light poses a great risk to the ocular surface. Blue light has high energy and can penetrate the cornea and lens to reach the retina. Due to its location in the anterior part of the eyeball, the cornea is the first point of contact for light entering the eye (Zhao et al., 2018). Therefore, blue light can directly act on corneal epithelial cells and endothelial cells, leading to a decrease in cell survival rate (Marek et al., 2018). At the same time, the content of reactive oxygen species (ROS) and the secretion of interleukin IL-1 β in the cornea will also increase, further mediating cellular oxidative damage and inflammatory response. The transparency of the crystalline lens decreases with age, leading to a gradual increase in absorbance within the blue light spectrum (Lee et al., 2016). Certain structural proteins, protein metabolites, and enzymes in the crystalline lens can absorb blue light, which can induce the production of reactive oxygen species (ROS) in the mitochondria of lens epithelial cells and lead to cell apoptosis through the transforming growth factor - β/Smad3 signaling pathway. This can lead to the gradual darkening and yellowing of the crystalline lens, which in turn can trigger cataracts (Cougnard-Gregoire et al., 2023). In addition, blue light damage can also trigger inflammatory reactions on the ocular surface, with damaged cells releasing inflammatory factors that attract the infiltration of inflammatory cells, further exacerbating tissue damage (Ouyang et al., 2023).
2.2.2 Effects of blue light on the retina
Research has shown that the mechanism of retinal blue light damage is mainly related to the production of free radicals, lipid peroxidation, lipofuscin, rhodopsin, Ca2+levels, and other factors. The main ones involved are RPE cells and photoreceptor cells (Qin et al., 2019). Short term (minutes to hours) exposure to high irradiance blue light can cause damage to RPE cells (Tosini et al., 2016). Lipofuscin accumulates extensively in RPE cells, and its toxicity is determined by the total content of its main fluorescent group, N-subretinoyl-N-retinol-ethanolamine (A2E). Blue light can accelerate the oxidation of cells by A2E, leading to oxidative stress response and inducing RPE cell apoptosis. Researchers can search for effective candidate drugs that may treat blue light damage based on this mechanism (Conti et al., 2021). Lipofuscin generates a large amount of ROS under blue light irradiation, which triggers endoplasmic reticulum stress (ER stress) in RPE cells, manifested by upregulation of GRP 78 and CHOP protein expression, and then induces cell apoptosis through activation of Caspase-3 and decreased expression of Bcl-2 (Zhao, 2015). Blue light irradiation leads to dysfunction of lysosomes, decreased ability of lysosome phagocytosis and autophagy, accumulation of lipofuscin in cells, disruption of epithelial barrier, and ultimately resulting in cell apoptosis; Blue light can also affect the intracellular Ca2+concentration in RPE cells, causing changes in mitochondrial transmembrane potential and leading to cell apoptosis (Yang et al., 2023). In addition, blue light irradiation can also cause damage to retinal vascular endothelium. Hypoxia inducible factor 1 α (HIF-1 α) is significantly expressed in RPE, and as the main hypoxia sensor, it can regulate the expression of vascular endothelial growth factor (VEGF). VEGF plays a key role in angiogenesis and vascular permeability, but its overexpression can damage endothelial function and epithelial mesenchymal transition (Zhang Y. et al., 2023). Research has found that the expression of HIF-1 α and VEGF is elevated in the retina of rabbits with light damage, indicating that light exposure can induce endothelial dysfunction in the retina (Wang et al., 2016). Therefore, the mechanism of blue light induced RPE cell apoptosis is related to oxidative stress, endoplasmic reticulum stress, lysosomal dysfunction, and mitochondrial potential changes.
Long term (several days to several weeks) exposure to low irradiance blue light can affect the wavelength activated photoreceptors (Tosini et al., 2016). High intensity blue light can cause irreversible inhibition of cytochrome oxidase, damage mitochondrial function and trigger cell apoptosis, while reducing sodium potassium ATPase activity, causing ion imbalance and increased osmotic pressure inside and outside the cell, leading to cell edema and organic damage, ultimately causing photoreceptor degeneration (Osborne et al., 2017). The degree of damage to photoreceptor cells (including cone cells and rod cells) exposed to blue light is higher, mainly due to the upregulation of retinal reactive oxygen species (ROS) production by blue light, which leads to mitochondrial damage in photoreceptor cells through oxidative stress. ROS induces the activation of mitogen activated kinase (MAPK), downregulates the phosphorylation level of extracellular regulatory protein kinase (p-ERK), upregulates activated nuclear transcription factor (NF-KB), activates autophagy pathway, and leads to apoptosis of retinal photoreceptor cells. After exposure to light, the expression level of pro-inflammatory factor miR-155 increases in the retina, while the expression level of anti-inflammatory factor SHIP1 decreases, leading to degeneration of retinal photoreceptors. Activation of NLRP3 inflammasome mediates the production of caspase-1 and 1L-1 β, leading to damage to retinal photoreceptor cells (Qin et al., 2019). Due to the presence of rhodopsin in rod cells and S-opsin in cone cells (Wheway et al., 2019), the conversion of rhodopsin by blue light can cause damage to both cone and rod cells, leading to blurred vision in the human eye. Due to the presence of rhodopsin, the photon capture ability of the retina is significantly enhanced under blue light irradiation, leading to an increase in the number of light induced cell deaths. Short wavelength LED light causes aggregation of short wavelength visual proteins, leading to degeneration of cone cells in the short term and severe damage to photoreceptor cells (Nakanishi et al., 2013). Blue light stimulation can activate the activity of prostaglandin synthase G/H. Prostaglandin synthase G/H is located in the inner and outer segments of rod and cone cells, promoting prostaglandin synthesis. It acts as a pigment group to absorb blue light, triggering oxidative reactions and producing a large amount of oxygen free radicals, leading to retinal damage and cell apoptosis (Xu and Wang, 2007). Therefore, the damage mechanism of blue light on photoreceptors is related to cytochrome oxidase, oxidative stress, mitochondrial damage, inflammatory response, and conversion of rhodopsin (Figure 2).
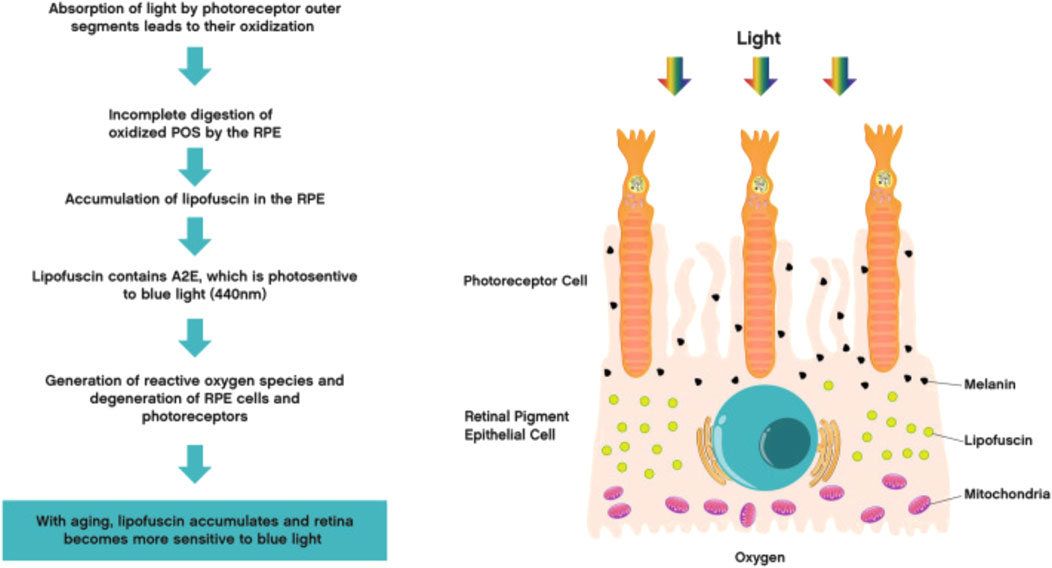
Figure 2. Mechanism of retinal blue light damage (Cougnard-Gregoire et al., 2023) (Quoted from Ophthalmology and therapy Journal).
3 Common models for research on blue light damage to eye diseases
3.1 Summary of cell modeling conditions
The replication of disease models through ex vivo cells has the advantages of short experimental time, convenient operation, easy observation, good controllability, and reduced use of experimental animals. Currently, most studies on the mechanism of blue light induced retinopathy and drug anti blue light activity also use in vitro experiments. Therefore, selecting a suitable cell model is crucial.
The retina plays an important role in visual transduction, with retinal photoreceptors and RPE cells involved in visual formation. Photoreceptors are divided into rod cells and cone cells. Rod cells contain rhodopsin, while cone cells contain opsin (Wheway et al., 2019). When exposed to blue light, both rhodopsin and opsin undergo varying degrees of damage, leading to retinal degeneration. RPE cells are crucial for vision, maintaining the vitality and function of photoreceptors by engulfing detached outer segments of photoreceptors (Hellinen et al., 2019). In addition, they have multiple functions such as supporting the neuroretina and choroid in the eye, as well as immune suppression (Sugita et al., 2021), and are commonly used in ophthalmic research and general epithelial cell research (Hazim et al., 2019).
Based on the physiological structure of the retina and the characteristics of blue light induced retinal lesions, mouse retinal photoreceptor cells (661W cells) and human retinal pigment epithelial cells (ARPE-19 cells) are commonly used cell models for light damage (Figure 3).
3.1.1 661W cell model
661W cells are derived from retinal tumors formed in transgenic mice expressing SV40 large T antigen under the control of the IRBP (photoreceptor retinol binding protein) promoter. They are currently widely used in the study of glaucoma (Sayyad et al., 2017), macular degeneration (Huang et al., 2022), intraretinal neurodegeneration (Kunimi et al., 2021), and blue light induced retinal lesions. A study used a 661W cell model to investigate the improvement of blue light induced subcellular damage by swiftgrass extract. After drug pretreatment for 1 h, 661W cells were exposed to blue light with a wavelength of 464 nm and a light intensity of 0.38 mW/cm2 for 24 h. It was found that swiftgrass extract protected cells from blue light induced cell death by inhibiting the increase in ROS production, and had a protective effect on mitochondria and lysosomes (Yamazaki et al., 2024); At the same time, ARPE-19 cells and 661W cells were used to investigate the anti blue light activity of Dendrobium polysaccharides. The modeling conditions were irradiated with 2500lux light for 3 h, 6 h, and 16 h. After 6 h of illumination, there was a significant difference in cell survival rate between the model group and the control group, indicating successful modeling (Hsu et al., 2024); The anti blue light activity of astaxanthin was investigated using a 661W cell model. LED blue light tubes with a wavelength of 450 ± 20 nm were selected and placed in an incubator. After pretreatment with astaxanthin at different concentrations for 1 h, the cells were exposed to a light intensity of 2000lux for 24 h. After modeling was completed, the cells were further cultured in a complete medium containing the drug for 24–48 h, and the anti blue light activity of astaxanthin was evaluated by measuring ROS levels and mitochondrial damage degree (Lin et al., 2020).
3.1.2 ARPE-19 cell model
The ARPE-19 cell line is derived from adult retinal pigment epithelial (RPE) explants, and ARPE-19 cells can be extensively expanded, providing a relatively stable source of retinal epithelial cells for functional and genetic research (Golconda et al., 2023). Lipofuscin is a byproduct of RPE cells engulfing the outer segment of photoreceptor cells, and its main fluorescent group is N-vinylidene retinyl-N-vinylethanolamine (A2E). When A2E is exposed to blue light, it is oxidized, inducing ROS production, oxidative stress, inflammatory response, etc., leading to RPE cell damage (Cho et al., 2023). Compared with 661W cells, ARPE-19 cells are more widely used in blue light damage research. Evaluate the anti blue light damage and retinal protective activity of the main active ingredient, hesperidin, in chrysanthemum using ARPE-19 cells. After pretreatment with A2E, expose the cells to blue light at a wavelength of 430 nm for 2 h, and the cell survival rate decreases by 50%, indicating the successful establishment of a light damage model (Feng et al., 2021); A study comparing two types of RPE cells, hTERT-RPE1 and ARPE-19, found that compared to hTERT-RPE1 cells, ARPE-19 cells have a stronger barrier function formed by tight junctions between cells. However, after being irradiated with blue light at a wavelength of 468 nm and a light intensity of 118.1 W/m2 for 4 h, the barrier function was impaired (Feng et al., 2021). The modeling conditions for the blue light damaged cell model are shown in (Table 1).
3.2 Summary of animal modeling conditions
Blue light damage animal modeling is the use of specific wavelengths of blue light to cause certain damage to animal eyes, in order to simulate the retinal damage that may occur in humans after prolonged exposure to blue light sources such as electronic screens. Its experimental technology is relatively mature and has a wide range of sources. The dorsal central region of the rat retina is considered a functional analogue of the human macular, which is the sharpest visual area (Gao et al., 2020). It has advantages such as simulation, controllability, repeatability, and safety. Therefore, through this model, researchers can better observe the impact of blue light on the visual system and explore methods for preventing or treating light damage, thereby providing scientific basis for protecting human visual health.
The animal model of blue light damage is generally selected from 5–6 week old SD rats, which need to undergo 7 days of adaptive feeding and dark maintenance before officially starting modeling. Because dark maintenance can eliminate the effects of other light sources on rat eyes and increase the photosensitivity of rat eyes. The blue light wavelength selected for the experiment is generally concentrated around 450 nm, and the blue light irradiation time is mostly 3 h, 6 h, 8 h, 12 h. Among them, the 12 h light exposure/12 h dark cycle is used for the 12 h light exposure, and the duration of irradiation depends on the light intensity of the selected lamp source. It is more appropriate to choose a duration of 2 weeks (14 days) for light exposure modeling. Studies have shown that blue light illumination within the range of 2000–10000 lux can cause damage to the retina of rats (Shang et al., 2014). There is a certain conversion relationship between blue light illumination and blue light irradiance, for example, at 450 nm blue light, 1W/m2 = 28lux, and color temperature is also related to blue light irradiance. During experiments, a thermometer can be placed inside the cage to investigate the temperature inside the cage. If the temperature is too high, certain temperature dispersion measures need to be taken. At present, animal experiments on blue light induced damage in rats are mostly based on blue light intensity. The distance from the light source to the rat’s eyes needs to be determined by considering the actual height of the rat cage and the magnitude of blue light intensity at different distances (Figure 4).
In addition, if a positive drug group is required to evaluate drug efficacy, the blue light irradiation positive drug group generally needs to be administered orally 1 week before modeling and continue until the end of light exposure. The relevant inspection indicators are tested after the end of light exposure, and in vivo intraocular examination can choose slit lamp microscopy, fundus photography, and ERG detection. After the completion of the live examination, blood samples are usually collected and euthanized from the rats 24 h later, and the eyeballs are removed to prepare retinal sections. The examination indicators generally include HE staining, immunohistochemistry, Western blot detection, TUNEL assay, oxidative stress (ROS), and transmission electron microscopy (TEM) (Shang et al., 2014), in order to analyze the protective effect of drugs on the retina of rats with blue light injury. The modeling conditions for the blue light damaged cell model are shown in (Table 2).
4 Research progress on drugs for preventing and treating eye diseases caused by blue light damage
The drug therapy for anti blue light damage can be divided into chemical drugs, natural drugs, and gene drugs. At present, a series of research results have been achieved on how to alleviate the damage of blue light to eye cells, such as RPE cells and photoreceptor cells, through drugs. Based on the pathogenesis of blue light damage, anti blue light damage drugs can include free radical scavengers, antioxidants, anti-inflammatory drugs, and gene therapy (Pan et al., 2023). Oxidative stress plays an important role in the harm caused by blue light. Research has shown that effective antioxidant extracts related to eliminating free radicals reduce oxidative damage caused by blue light, thereby improving clinical symptoms of ocular surface in dry eye mouse models (Zhu et al., 2022). These three types of drugs each have their own advantages and limitations. The research and application of chemical drugs are relatively extensive, but there may be certain side effects. Natural medicines provide a more natural option, but their effectiveness and safety require further research to confirm. The application of gene therapy in eye diseases is an emerging field, but its technical complexity and potential risks also require further research and evaluation.
4.1 Medication routes and obstacles for the treatment of eye diseases
The anterior segment of the eye is composed of the cornea, conjunctiva, iris, ciliary body, crystalline lens, and aqueous humor, while the posterior segment of the eye includes the posterior two-thirds of the eye, including the vitreous, retina, choroid, and optic nerve (Vaneev et al., 2021). The blood-ocular barrier (BOB) includes the blood-aqueous barrier (BAB) located in the anterior segment of the eye and the blood-retinal barrier (BRB) located in the posterior segment of the eye, which can restrict the entry of drugs from the blood into the eye and is the main obstacle in treating eye diseases (Lyu et al., 2024). Drugs enter the body orally, are absorbed through the gastrointestinal tract, enter the liver through the portal vein, and some drugs may be metabolized by the liver or bind with plasma proteins before entering the systemic circulation and ultimately reaching the eyes. Among them, drugs need to overcome the first pass effect of the liver and the blood-ocular barrier to reach the eye, so their bioavailability is relatively low. The routes of drug delivery to the eye include local, periocular, intravitreal, suprachoroidal, and subretinal. Intravitreal, suprachoroidal, and subretinal administration is an ideal choice for treating posterior ocular lesions (Varela-Fernandez et al., 2020), as they can bypass more anterior barriers such as tear film, cornea, and sclera, but may cause injection related complications. External use mainly involves applying medication to the surface of the cornea or conjunctiva through eye drops or ointment. Eye surface administration is the most commonly used route of administration, but drugs are easily washed away by tears and difficult to penetrate the corneal barrier, so their therapeutic effect on posterior ocular diseases is limited. The development of new ocular drug delivery systems, such as nanoparticles, liposomes, and hyper choroidal injection (Singh et al., 2024; Wu et al., 2024), can effectively deliver drugs to the posterior segment of the eye (Puglia et al., 2021), which is expected to improve the ocular bioavailability and therapeutic efficacy of drugs.
4.2 The efficacy and mechanism of chemical drugs
At present, the research and application of chemical drugs for the treatment of BLED have become quite popular. The mechanism of action of these drugs is mainly achieved through antioxidant effects and the clearance of free radicals to achieve therapeutic effects. Therefore, anti blue light damage chemical drugs mainly include free radical scavengers and antioxidants. In addition, enzyme activity protectants, optic nerve protectants, calcium ion antagonists, and hormone drugs have also played a positive role in protecting retinal cells. The summary of the pharmacological mechanisms of chemical drugs for preventing blue light damage is shown in (Table 3).
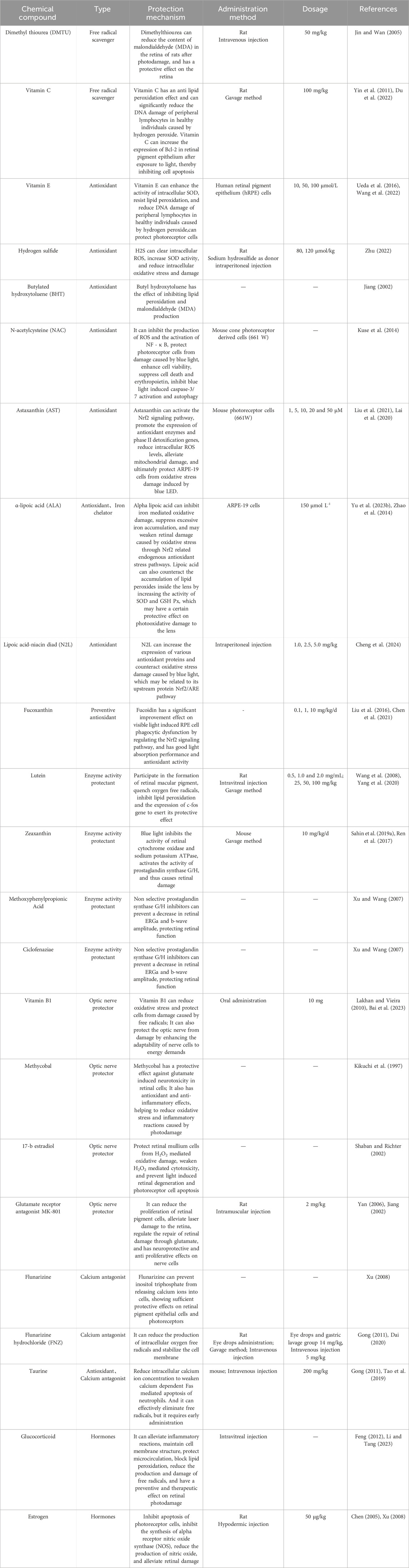
Table 3. Summary of the pharmacological mechanisms of chemical drugs for preventing blue light damage.
In addition, blue light damage is a cause of various eye diseases. Although current treatment methods are limited and mainly focus on relieving symptoms and delaying disease progression, researchers are actively exploring new treatment strategies. Research shows that both blue light damage and diabetes retinopathy (DR) involve the damage and dysfunction of retinal nerve cells. The locally administered NOX4 inhibitor GLX7013114 can effectively protect retinal neurons and amacrine cells, and alleviate oxidative stress and inflammatory reactions (Dionysopoulou et al., 2023). It may become a new drug for treating blue light damage. In addition to GLX7013114, lidocaine pretreatment has also been shown to prolong the lifespan and improve the function of retinal ganglion cells (RGCs) (Chou et al., 2018), which may provide another neuroprotective approach for treating blue light injury.
4.3 The efficacy and mechanism of natural medicine
At present, research on natural medicines for combating blue light damage is mostly focused on antioxidants and anti-inflammatory drugs. For example, caffeine, as an approved drug for clinical use, has anti-inflammatory and neuroprotective effects, and its mechanism of action is oxidative stress and inflammatory response. Currently, studies have shown that caffeine may be a potential candidate for the treatment of retinal degeneration (Conti et al., 2021). The research results indicate that natural medicine has significant therapeutic effects in preventing and treating eye diseases caused by blue light (Li C. et al., 2021). Natural medicine, with its multi-component and multi-target characteristics, can play a role in different stages. It can not only effectively eliminate free radicals and reduce oxidative damage, but also inhibit inflammatory reactions and protect the integrity of eye cells. The following summarizes the individual components of natural medicine for preventing blue light damage and their pharmacological mechanisms, in order to provide reference for research and application in related fields (Figure 5). The summary of the pharmacological mechanisms of natural medicine monomers for preventing blue light damage is shown in (Table 4); The summary of the pharmacological mechanism of natural medicine for preventing blue light damage is shown in (Table 5).
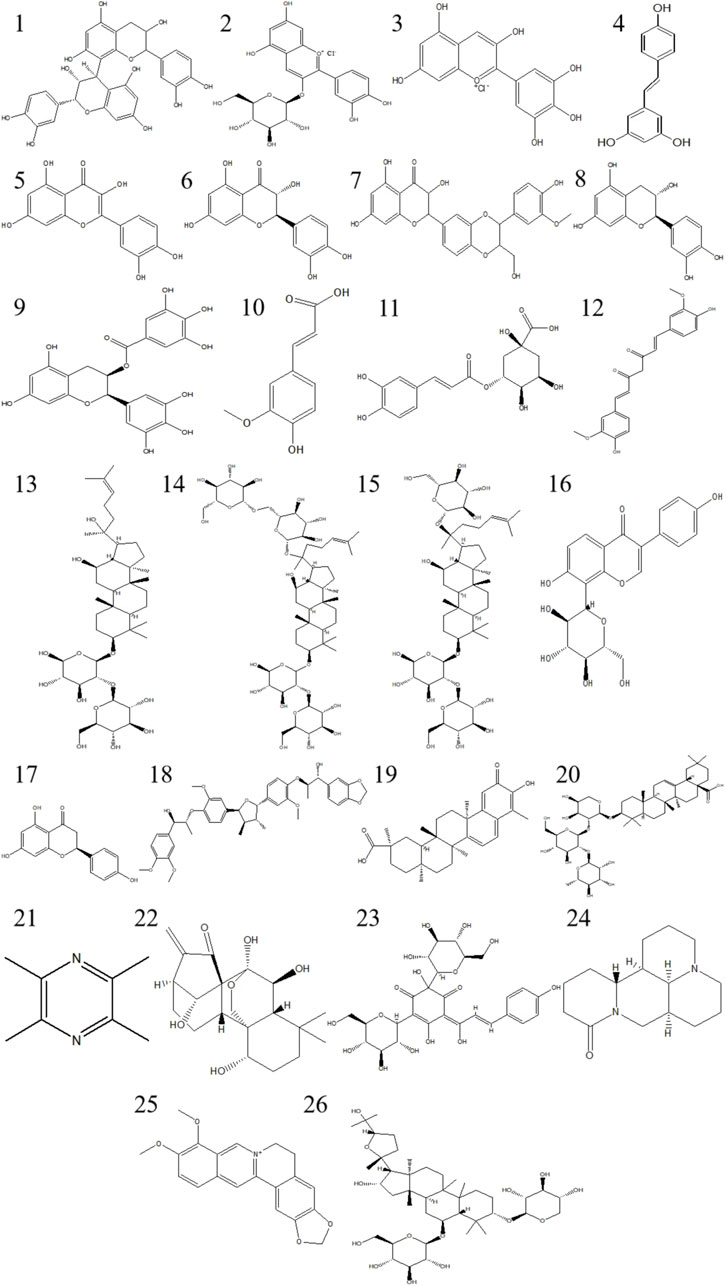
Figure 5. The compound structure of traditional Chinese medicine monomers includes:1 Procyanidin B2; 2 Cyanidin 3-O-glucoside chloride; 3 Delphinidin; 4 Resveratrol; 5 Quercetin; 6 Taxifolin; 7 Silymarin; 8 Catechin; 9 Epigallocatechin Gallate; 10 Ferulic acid; 11 Chlorogenic Acid; 12 Curcumin; 13 Ginsenoside Rg3; 14 Ginsenoside Rb1; 15 Ginsenoside Rd; 16 Puerarin; 17 Naringenin; 18 Manassantin B; 19 Celastrol; 20 Raddeanin A; 21 Tetramethylpyrazine; 22 Oridonin; 23 Hydroxysafflor Yellow A; 24 Matrine; 25 Berberine; 26 Astragaloside IV.
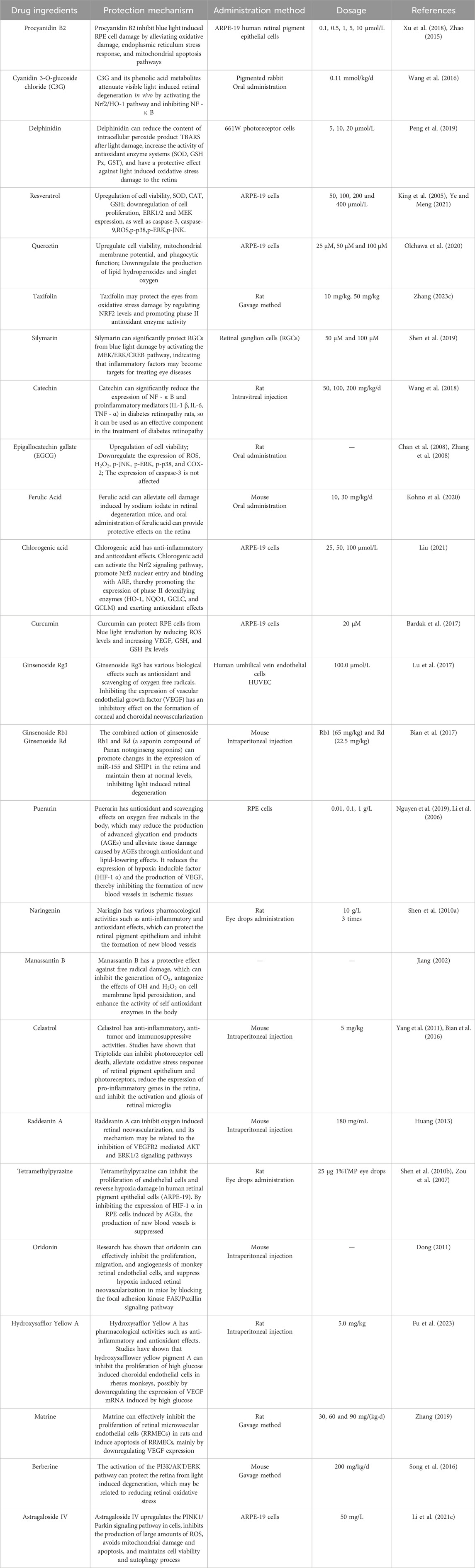
Table 4. Summary of pharmacological mechanisms of monomers in natural medicine for preventing blue light damage.
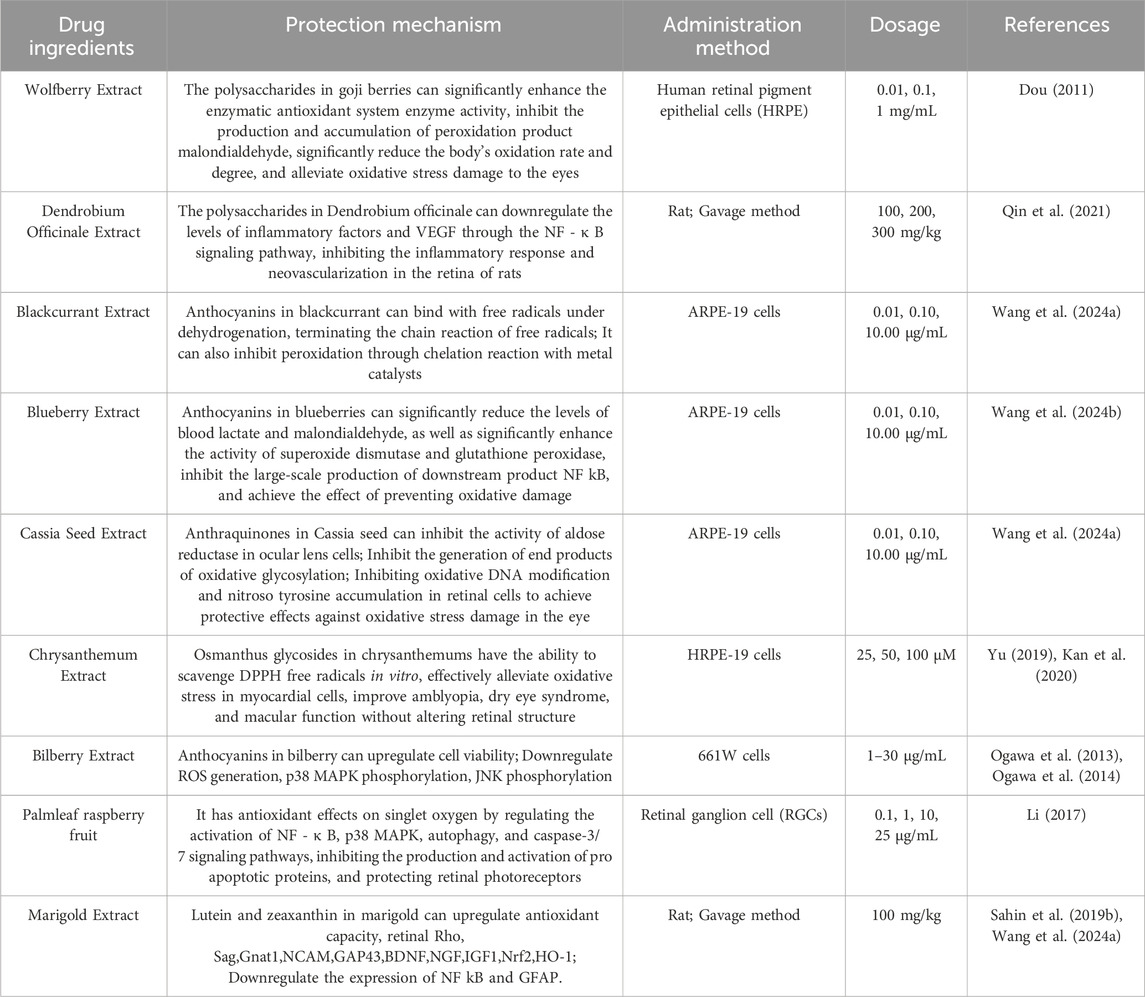
Table 5. Summary of the pharmacological mechanisms of traditional Chinese medicine for preventing blue light damage.
4.4 Efficacy mechanism of gene drug therapy
At present, some gene drugs can effectively treat BLED by enhancing cellular antioxidant capacity, regulating endoplasmic reticulum stress, and fundamentally reducing the damage of blue light to eye cells, thereby achieving good therapeutic effects. The mechanism of action of gene drugs is clear, and they can target eye cells with precise drug delivery and action, reducing side effects on other tissues and organs. The summary of the pharmacological mechanisms of gene therapy for preventing blue light damage is shown in (Table 6).
5 Future perspectives
With the popularization of electronic equipment, the incidence rate of eye blue light injury is expected to continue to rise, especially among young and elderly people. It is predicted that by 2050, the incidence rate of eye diseases such as myopia, diabetes retinopathy, macular degeneration and glaucoma will increase significantly (Antemie et al., 2023; Landreneau et al., 2021). Therefore, future research directions may focus more on the role of blue light damage in ophthalmic diseases in young and elderly people. In the field of ocular pathophysiology, research will utilize more advanced imaging techniques to achieve real-time monitoring of the damage process of blue light to ocular tissues. The study may reveal new associations between blue light damage and genetics, environment, and lifestyle, and explore how these factors collectively affect eye health. Experimental modeling will focus more on simulating the actual human exposure to blue light, including factors such as light intensity, wavelength, and duration. It is also possible to develop models that are closer to human pathophysiological characteristics, such as using gene editing techniques to create animal models with specific genetic backgrounds.
Although some drugs and supplements have been used to treat blue light damage, their efficacy and safety still need further validation. Natural medicine has unique advantages in treating BLED due to its multi-component synergistic effect, overall regulation, and mild and safe characteristics. Therefore, it is necessary to explore the mechanism of action of natural medicine components in preventing and treating blue light damage, as well as how to integrate them with modern medicine. Meanwhile, delving deeper into the molecular mechanisms of blue light damage can help discover new therapeutic targets. At present, RNA nanomedicine has been used to achieve specific targets in the clinical treatment of eye diseases (Zhang et al., 2024). In order to improve the therapeutic effect, it is necessary to develop new drugs and formulations to enhance the permeability and bioavailability of drugs in the eye. It is pointed out that hydrogels can prolong the residence time of drugs in the eye and control drug release, thus improving the bioavailability of drugs (Han et al., 2024). These research findings provide new directions for future drug development.
In addition, the ocular microbiome is a dynamic ecosystem that plays a crucial but yet to be fully explored role in human health. The immune pardon mechanism of the eye includes the blood aqueous barrier, blood retinal barrier, and anti-inflammatory cytokines, all of which are important components. Recent studies have begun to explore the role of the ocular surface in the lung eye axis, emphasizing the bidirectional relationship between the respiratory system and eye health, suggesting that respiratory diseases may have an impact on eye diseases (Allam et al., 2024). Other studies have shown that gut microbiota is not only involved in the occurrence and development of various extraintestinal diseases, but also closely related to ophthalmic diseases such as uveitis, age-related macular degeneration, and glaucoma. The concept of gut eye axis can be used to explain the impact of gut microbiota imbalance on eye health (Zhang H. et al., 2023; Napolitano et al., 2021). Although research on the gut eye axis is currently insufficient and controversial, and cannot fully explain all existing issues, elucidating the pathological mechanisms related to gut microbiota and common eye diseases may provide new ideas for disease diagnosis and treatment.
Author contributions
YuY: Writing–original draft, Writing–review and editing, Visualization. YW: Writing–original draft, Writing–review and editing, Visualization. YZ: Writing–review and editing. YaY: Writing–review and editing. GA: Writing–review and editing. ZL: Supervision, Writing–review and editing. DQ: Conceptualization, Supervision, Writing–original draft, Writing–review and editing.
Funding
The author(s) declare that financial support was received for the research, authorship, and/or publication of this article. This work is supported by the Science and Technology Project of Haihe Laboratory of Modern Chinese Medicine (Grant number: 24HHZYSS00007).
Conflict of interest
The authors declare that the research was conducted in the absence of any commercial or financial relationships that could be construed as a potential conflict of interest.
Generative AI statement
The author(s) declare that no Generative AI was used in the creation of this manuscript.
Publisher’s note
All claims expressed in this article are solely those of the authors and do not necessarily represent those of their affiliated organizations, or those of the publisher, the editors and the reviewers. Any product that may be evaluated in this article, or claim that may be made by its manufacturer, is not guaranteed or endorsed by the publisher.
References
Alaimo, A., Linares, G. G., Bujjamer, J. M., Gorojod, R. M., Alcon, S. P., Martinez, J. H., et al. (2019). Toxicity of blue led light and A2E is associated to mitochondrial dynamics impairment in ARPE-19 cells: implications for age-related macular degeneration. Arch. Toxicol. 93 (5), 1401–1415. doi:10.1007/s00204-019-02409-6
Allam, V., Patel, V. K., De Rubis, G., Paudel, K. R., Gupta, G., Chellappan, D. K., et al. (2024). Exploring the role of the ocular surface in the lung-eye axis: insights into respiratory disease pathogenesis. Life Sci. 349, 122730. doi:10.1016/j.lfs.2024.122730
An, N. (2022). Effect of lentivirus mediated ERRα gene overexpression on blue light induced apoptosis of retinal pigment epithelial cells. Master’s thesis. Guizhou Medical University.
Antemie, R. G., Samoila, O. C., and Clichici, S. V. (2023). Blue light-ocular and systemic damaging effects: a narrative review. Int. J. Mol. Sci. 24 (6), 5998. doi:10.3390/ijms24065998
Bai, M., Miao, D., Li, Y., Lyu, J., Liu, Z., Li, Y., et al. (2023). Ginsenoside Rg1 injection combined with inosine tablets and vitamin B1 for the treatment of primary retinitis pigmentosa. Int. Eye Sci. 23 (12), 2035–2039. doi:10.3980/j.issn.1672-5123.2023.12.19
Bardak, H., Uguz, A. C., and Bardak, Y. (2017). Curcumin regulates intracellular calcium release and inhibits oxidative stress parameters, VEGF, and caspase-3/-9 levels in human retinal pigment epithelium cells. Physiol. Int. 104 (4), 301–315. doi:10.1556/2060.104.2017.4.3
Bian, M., Du, X., Cui, J., Wang, P., Wang, W., Zhu, W., et al. (2016). Celastrol protects mouse retinas from bright light-induced degeneration through inhibition of oxidative stress and inflammation. J. Neuroinflamm. 13, 50. doi:10.1186/s12974-016-0516-8
Bian, M., Du, X., Wang, P., Cui, J., Xu, J., Gu, J., et al. (2017). Combination of ginsenoside Rb1 and Rd protects the retina against bright light-induced degeneration. Sci. Rep. 7 (1), 6015. doi:10.1038/s41598-017-06471-x
Bin, Y. (2021). The protective effect and mechanism of Grx2 in blue light induced retinal light damage. Dissertation. Chongqing Medical University.
Bonfiglio, V., Rejdak, R., Nowomiejska, K., Zweifel, S. A., Justus, W. M., Romano, G. L., et al. (2022). Efficacy and safety of subthreshold micropulse yellow laser for persistent diabetic macular edema after vitrectomy: a pilot study. Front. Pharmacol. 13, 832448. doi:10.3389/fphar.2022.832448
Chan, C. M., Huang, J. H., Lin, H. H., Chiang, H. S., Chen, B. H., Hong, J. Y., et al. (2008). Protective effects of (-)-epigallocatechin gallate on UVA-induced damage in ARPE19 cells. Mol. Vis. 14, 2528–2534. doi:10.3390/ijms16035789
Chen, S. J., Lin, T. B., Peng, H. Y., Liu, H. J., Lee, A. S., Lin, C. H., et al. (2021). Cytoprotective potential of fucoxanthin in oxidative stress-induced age-related macular degeneration and retinal pigment epithelial cell senescence in vivo and in vitro. Mar. Drugs. 19 (2), 114. doi:10.3390/md19020114
Chen, W. J., Wu, C., Xu, Z., Kuse, Y., Hara, H., and Duh, E. J. (2017). Nrf2 protects photoreceptor cells from photo-oxidative stress induced by blue light. Exp. Eye Res. 154, 151–158. doi:10.1016/j.exer.2016.12.001
Chen, X. (2005). The protective effect of estrogen on retinal photodamage. Master’s thesis. Sichuan University.
Chen, Y. (2021). Study on the protective effect of Buyang Huanwu Decoction on retinal blue light damage in rats. Master’s thesis. Liaoning University of Traditional Chinese Medicine.
Cheng, T., Zou, Y., Jian, L., Zhang, M., and Dou, Y. (2024). Prevention and treatment of lipoic acid-niacin on blue-light-induced retinal damage in rats. Int. Eye Sci. 24 (02), 196–202. doi:10.3980/j.issn.1672-5123.2024.2.04
Cho, H. M., Lee, S. J., and Choung, S. Y. (2023). Protective effects of Panax ginseng berry extract on blue light-induced retinal damage in ARPE-19 cells and mouse retina. J. Ginseng Res. 47 (1), 65–73. doi:10.1016/j.jgr.2022.04.002
Choi, W., Lee, J. B., Cui, L., Li, Y., Li, Z., Choi, J. S., et al. (2016). Therapeutic efficacy of topically applied antioxidant medicinal plant extracts in a mouse model of experimental dry eye. Cell. Longev. 2016, 4727415. doi:10.1155/2016/4727415
Chong, J. (2022). Research progress of pathogenesis and surgical treatment of dry eye. Chin. Foreign Med. Res. 20 (33), 181–184. doi:10.14033/j.cnki.cfmr.2022.33.045
Chou, T. H., Musada, G. R., Romano, G. L., Bolton, E., and Porciatti, V. (2018). Anesthetic preconditioning as endogenous neuroprotection in glaucoma. Int. J. Mol. Sci. 19 (1), 237. doi:10.3390/ijms19010237
Conti, F., Lazzara, F., Romano, G. L., Platania, C., Drago, F., and Bucolo, C. (2021). Caffeine protects against retinal inflammation. Front. Pharmacol. 12, 824885. doi:10.3389/fphar.2021.824885
Cougnard-Gregoire, A., Merle, B., Aslam, T., Seddon, J. M., Aknin, I., Klaver, C., et al. (2023). Blue light exposure: ocular hazards and prevention-A narrative review. Ophthalmol. Ther. 12 (2), 755–788. doi:10.1007/s40123-023-00675-3
Dai, M. (2020). Preparation and evaluation of flunarizine hydrochloride ophtihalmic in situ organogel for systemic delivery. Master’s thesis. Anhui University of Chinese Medicine.
Davies, S., Elliott, M. H., Floor, E., Truscott, T. G., Zareba, M., Sarna, T., et al. (2001). Photocytotoxicity of lipofuscin in human retinal pigment epithelial cells. Free Radic. Biol. Med. 31 (2), 256–265. doi:10.1016/s0891-5849(01)00582-2
Dionysopoulou, S., Wikstrom, P., Bucolo, C., Romano, G. L., Micale, V., Svensson, R., et al. (2023). Topically administered NOX4 inhibitor, GLX7013114, is efficacious in treating the early pathological events of diabetic retinopathy. Diabetes 72 (5), 638–652. doi:10.2337/db22-0515
Dong, S., Liu, S., and Li, Q. (2018). Protective effects of light preconditioning on retinal photoreceptor cells aganist light damage. Recent Adv. Ophthalmol. 38 (05), 412–415+420. doi:10.13389/j.cnki.rao.2018.0096
Dong, Y. (2011). Research on inhibitory activity of small molecular compounds on tumor growth & metastasis via inhibiting angiogenesis and mechanism. Dissertation. East China Normal University.
Dou, R. (2011). Experimental Study of the lycium barbarum polysaccharide on the role of retinal pigment epithelial cells. Master’s thesis. Shandong University of Traditional Chinese Medicine.
Du, B., Xu, Y., and Wei, Z. (2022). Vitamin C alleviates macular degeneration in rats by regulating function of macrophages. J. Clin. Med. Pract. 26 (17), 130–134. doi:10.7619/jcmp.20220296
Fan, B., Zhang, C., Chi, J., Liang, Y., Bao, X., Cong, Y., et al. (2022). The molecular mechanism of retina light injury focusing on damage from short wavelength light. Oxidative Med. Cell. Longev. 2022, 8482149. doi:10.1155/2022/8482149
Feng, J., Lu, B., Zhu, H., Sun, X. J., and Sun, X. D. (2019). HSPA5 regulates endoplasmic reticulum stress for cell survival in retinal pigment epithelial cells. Recent Adv. Ophthalmol. 39 (12), 1111–1115. doi:10.13389/j.cnki.rao.2019.0255
Feng, J. H., Dong, X. W., Yu, H. L., Shen, W., Lv, X. Y., Wang, R., et al. (2021). Cynaroside protects the blue light-induced retinal degeneration through alleviating apoptosis and inducing autophagy in vitro and in vivo. Phytomedicine 88, 153604. doi:10.1016/j.phymed.2021.153604
Feng, M. (2012). Protective effect of saturated hydrogen saline against blue light-induced retinal damage in rats. Dissertation. Huazhong University of Science and Technology.
Fu, Y., Wang, T., Cao, J., Li, G., Zheng, L., Xu, W., et al. (2023). The protective effect of hydroxysafflor yellow A on light-induced retinal injury in rats. J. Otolaryngology Ophthalmol. Shandong Univ. 37 (05), 128–134. doi:10.6040/j.issn.1673-3770.0.2022.455
Gao, Y., Huang, J., and Yu, Y. (2020). Research progress on animal models of retinal photodamage. J. Mudanjiang Med. Univ. 41 (04), 133–135. doi:10.13799/j.cnki.mdjyxyxb.2020.04.036
Golconda, P., Andrade-Medina, M., and Oberstein, A. (2023). Subconfluent ARPE-19 cells display mesenchymal cell-state characteristics and behave like fibroblasts, rather than epithelial cells, in experimental HCMV infection studies. Viruses-Basel. 16 (1), 49. doi:10.3390/v16010049
Gong, X. (2011). Blue-light induced changes of L-type calcium channel subunit mRNA expression and free Calcium ion of Human Retinal Pigment epithelium Cells in vitro. Master’s thesis. Zunyi Medical University.
Han, J., Shu, H., Zhang, L., and Huang, S. (2024). Latest advances in hydrogel therapy for ocular diseases. Polymer 306, 127207. doi:10.1016/j.polymer.2024.127207
Hazim, R. A., Volland, S., Yen, A., Burgess, B. L., and Williams, D. S. (2019). Rapid differentiation of the human RPE cell line, ARPE-19, induced by nicotinamide. Exp. Eye Res. 179, 18–24. doi:10.1016/j.exer.2018.10.009
He, G. H., Zhang, W., Ma, Y. X., Yang, J., Chen, L., Song, J., et al. (2018). Mesenchymal stem cells-derived exosomes ameliorate blue light stimulation in retinal pigment epithelium cells and retinal laser injury by VEGF-dependent mechanism. Int. J. Ophthalmol. 11 (4), 559–566. doi:10.18240/ijo.2018.04.04
Hellinen, L., Hagstrom, M., Knuutila, H., Ruponen, M., Urtti, A., and Reinisalo, M. (2019). Characterization of artificially re-pigmented ARPE-19 retinal pigment epithelial cell model. Sci. Rep. 9 (1), 13761. doi:10.1038/s41598-019-50324-8
Hsu, W. H., Sangkhathat, C., Lu, M. K., Lin, W. Y., Liu, H. P., and Lin, Y. L. (2024). Dendrobium nobile polysaccharide attenuates blue light-induced injury in retinal cells and in vivo in Drosophila. Antioxidants 13 (5), 603. doi:10.3390/antiox13050603
Hu, Z., Zhang, Y., Wang, J., Mao, P., Lv, X., Yuan, S., et al. (2016). Knockout of Ccr2 alleviates photoreceptor cell death in rodent retina exposed to chronic blue light. Cell Death Dis. 7 (11), e2468. doi:10.1038/cddis.2016.363
Huang, L. (2013). Research on function and molecular mechanism of RaddeaninA in inhibition of ocular neovascularization. Master’s thesis. East China Normal University.
Huang, Y., Chen, X., Jiang, Z., Luo, Q., Wan, L., Hou, X., et al. (2022). Transcriptome sequencing reveals tgf-β-mediated noncoding RNA regulatory mechanisms involved in DNA damage in the 661W photoreceptor cell line. Genes 13 (11), 2140. doi:10.3390/genes13112140
Iseli, H. P., Korber, N., Koch, C., Karl, A., Penk, A., Huster, D., et al. (2016). Scleral cross-linking by riboflavin and blue light application in young rabbits: damage threshold and eye growth inhibition. Graefes Arch. Clin. Exp. Ophthalmol. 254 (1), 109–122. doi:10.1007/s00417-015-3213-x
Jaadane, I., Boulenguez, P., Chahory, S., Carre, S., Savoldelli, M., Jonet, L., et al. (2015). Retinal damage induced by commercial light emitting diodes (LEDs). Free Radic. Biol. Med. 84, 373–384. doi:10.1016/j.freeradbiomed.2015.03.034
Jiang, Y. (2002). Research progress on prevention and treatment of retinal photodamage. Chin. J. Pract. Ophthalmology 03, 166–168. doi:10.3760/cma.j.issn.1006-4443.2002.03.002
Jin, H. L., and Jeong, K. W. (2022). Transcriptome analysis of long-term exposure to blue light in retinal pigment epithelial cells. Biomol. Ther. 30 (3), 291–297. doi:10.4062/biomolther.2021.155
Jin, X., and Wan, Q. (2005). The effect of dimethylthiourea(DMTU) on MDA levels in rats with retinal photodamage. Chin. J. Ophthalmol. Otorhinolaryngology 5 (02), 79–80. doi:10.14166/j.issn.1671-2420.2005.02.008
Kan, J., Wang, M., Liu, Y., Liu, H., Chen, L., Zhang, X., et al. (2020). A novel botanical formula improves eye fatigue and dry eye: a randomized, double-blind, placebo-controlled study. Am. J. Clin. Nutr. 112 (2), 334–342. doi:10.1093/ajcn/nqaa139
Kara-Junior, N., Espindola, R. F., Gomes, B. A., Ventura, B., Smadja, D., and Santhiago, M. R. (2011). Effects of blue light-filtering intraocular lenses on the macula, contrast sensitivity, and color vision after a long-term follow-up. J. Cataract. Refract. Surg. 37 (12), 2115–2119. doi:10.1016/j.jcrs.2011.06.024
Keenan, T., Cukras, C. A., and Chew, E. Y. (2021). Age-related macular degeneration: epidemiology and clinical aspects. Adv. Exp. Med. Biol. 1256, 1–31. doi:10.1007/978-3-030-66014-7_1
Kikuchi, M., Kashii, S., Honda, Y., Tamura, Y., Kaneda, K., and Akaike, A. (1997). Protective effects of methylcobalamin, a vitamin B12 analog, against glutamate-induced neurotoxicity in retinal cell culture. Invest. Ophthalmol. Vis. Sci. 38 (5), 848–854.
King, R. E., Kent, K. D., and Bomser, J. A. (2005). Resveratrol reduces oxidation and proliferation of human retinal pigment epithelial cells via extracellular signal-regulated kinase inhibition. Chem.-Biol. Interact. 151 (2), 143–149. doi:10.1016/j.cbi.2004.11.003
Kohno, M., Musashi, K., Ikeda, H. O., Horibe, T., Matsumoto, A., and Kawakami, K. (2020). Oral administration of ferulic acid or ethyl ferulate attenuates retinal damage in sodium iodate-induced retinal degeneration mice. Sci. Rep. 10 (1), 8688. doi:10.1038/s41598-020-65673-y
Kunimi, H., Lee, D., Ibuki, M., Katada, Y., Negishi, K., Tsubota, K., et al. (2021). Inhibition of the HIF-1α/BNIP3 pathway has a retinal neuroprotective effect. Faseb J. 35 (8), e21829. doi:10.1096/fj.202100572R
Kuse, Y., Ogawa, K., Tsuruma, K., Shimazawa, M., and Hara, H. (2014). Damage of photoreceptor-derived cells in culture induced by light emitting diode-derived blue light. Sci. Rep. 4, 5223. doi:10.1038/srep05223
Lai, T. T., Yang, C. M., and Yang, C. H. (2020). Astaxanthin protects retinal photoreceptor cells against high glucose-induced oxidative stress by induction of antioxidant enzymes via the PI3K/Akt/Nrf2 pathway. Antioxidants 9 (8), 729. doi:10.3390/antiox9080729
Lakhan, S. E., and Vieira, K. F. (2010). Nutritional and herbal supplements for anxiety and anxiety-related disorders: systematic review. Nutr. J. 9, 42. doi:10.1186/1475-2891-9-42
Landreneau, J. R., Hesemann, N. P., and Cardonell, M. A. (2021). Review on the myopia pandemic: epidemiology, risk factors, and prevention. Mo Med. 118 (2), 156–163.
Lee, H. S., Cui, L., Li, Y., Choi, J. S., Choi, J. H., Li, Z., et al. (2016). Correction: influence of light emitting diode-derived blue light overexposure on mouse ocular surface. PLoS One 11 (11), e0167671. doi:10.1371/journal.pone.0167671
Li, C., Li, Q., Hong, J., Chen, F., and Guo, C. (2021a). Protective effect of Astragaloside IV on the blue light-induced damage of retinal pigment epithelium and its mechanism. Recent Adv. Ophthalmol. 41 (11), 1006–1011. doi:10.13389/j.cnki.rao.2021.0212
Li, D., Zou, X., Chen, J., Xu, Z., Yu, Y., Zhou, W., et al. (2017). Protective effects of tissue factor targeting peptide on human retinal pigment epithelial cell damage induced by blue light. Chin. J. Exp. Ophthalmol. 35 (7), 7. doi:10.3760/cma.j.issn.2095-0160.2017.07.006
Li, G. (2017). Study on the protective effect of traditional Chinese medicine raspberry on cultured retinal ganglion cells in vitro. Master’s thesis. Liaoning University of Traditional Chinese Medicine.
Li, H., Zhang, M., Wang, D., Dong, G., Chen, Z., Li, S., et al. (2021b). Blue light from cell phones can cause chronic retinal light injury: the evidence from a clinical observational study and a SD rat model. Biomed. Res. Int. 2021, 3236892. doi:10.1155/2021/3236892
Li, M., and Tang, A. (2023). Advances in the application of intravitreal injection of glucocorticoids. J. Gannan Med. Univ. 43 (04), 429–434. doi:10.3969/j.issn.1001-5779.2023.04.020
Li, W., Jiang, D., Guo, L., Wang, P., and Zhang, L. (2006). Puerarin inhibits the proliferation of human retinal pigment epithelial cells and the expression of hypoxia-inducible factor-1α in human RPE cells induced by advanced glycation end products. Int. Eye Sci. (03), 580–583. doi:10.3969/j.issn.1672-5123.2006.03.020
Li, X., Jing, N., and Liu, X. (2021c). Research progress of traditional Chinese medicine in the treatment of ocular diseases. Int. Med. Health Guid. News 27 (16), 4. doi:10.1016/j.lfs.2024.123045
Li, Y., Zhang, P., Huang, C., and Wang, W. (2020). Dual effect of blue light on Fusariumsolani clinical corneal isolates in vitro. Lasers Med. Sci. 35 (6), 1299–1305. doi:10.1007/s10103-019-02911-4
Lin, C. W., Yang, C. M., and Yang, C. H. (2020). Protective effect of astaxanthin on blue light light-emitting diode-induced retinal cell damage via free radical scavenging and activation of PI3K/Akt/Nrf2 pathway in 661W cell model. Mar. Drugs. 18 (8), 387. doi:10.3390/md18080387
Lin, X. (2020). Role of estrogen-related receptor α in blue light-induced apoptosis of human retinal pigment epithelial cells. Master’s thesis. Jinan University.
Liu, T. (2019). Preliminary study of the effect of different wavelengths LED on lens. Dissertation. Tianjin Medical University.
Liu, Y. (2021). Protective role of chlorogenic acid against oxidative damage of ARPE-19 induced by blue light LED. Master’s thesis. Tianjin University of Science and Technology.
Liu, Y., Liu, M., Zhang, X., Chen, Q., Chen, H., Sun, L., et al. (2016). Protective effect of fucoxanthin isolated from laminaria japonica against visible light-induced retinal damage both in vitro and in vivo. J. Agric. Food Chem. 64 (2), 416–424. doi:10.1021/acs.jafc.5b05436
Liu, Y., Ma, N., Liu, Y., Guo, Y., Li, H., Shan, Y., et al. (2021). Astaxanthin protects retinal pigment epithelial cells from oxidative stress induced by blue light emitting diodes. Food Sci. 42 (21), 128–136. doi:10.7506/spkx1002-6630-20201017-154
Lu, Y., Cai, L., Zhu, C., Liu, G., Zhang, Y., Huang, J., et al. (2017). Inhibitory effect of ginsenoside RG3 on choroidal neovascularization. Recent Adv. Ophthalmol. 37 (10), 922–925. doi:10.13389/j.cnki.rao.2017.0234
Lyu, J., Chen, F., and Yu, Y. (2024). Advances in novel ocular drug delivery systems. Chin. J. Mod. Appl. Pharm. 41 (03), 398–407. doi:10.13748/j.cnki.issn1007-7693.20221054
Marek, V., Melik-Parsadaniantz, S., Villette, T., Montoya, F., Baudouin, C., Brignole-Baudouin, F., et al. (2018). Blue light phototoxicity toward human corneal and conjunctival epithelial cells in basal and hyperosmolar conditions. Free Radic. Biol. Med. 126, 27–40. doi:10.1016/j.freeradbiomed.2018.07.012
Marie, M., Bigot, K., Angebault, C., Barrau, C., Gondouin, P., Pagan, D., et al. (2018). Light action spectrum on oxidative stress and mitochondrial damage in A2E-loaded retinal pigment epithelium cells. Cell Death Dis. 9 (3), 287. doi:10.1038/s41419-018-0331-5
Moreira, P. I., and Oliveira, C. R. (2011). Mitochondria as potential targets in antidiabetic therapy. Handb. Exp. Pharmacol. 203, 331–356. doi:10.1007/978-3-642-17214-4_14
Nakanishi, T., Shimazawa, M., Sugitani, S., Kudo, T., Imai, S., Inokuchi, Y., et al. (2013). Role of endoplasmic reticulum stress in light-induced photoreceptor degeneration in mice. J. Neurochem. 125 (1), 111–124. doi:10.1111/jnc.12116
Nan, L., Zhang, Y., Song, H., Ye, Y., Jiang, Z., and Zhao, S. (2023). Influence of light-EmittingDiode-derived blue light overexposure on rat ocular surface. J. Ophthalmol. 2023, 1097704. doi:10.1155/2023/1097704
Napolitano, P., Filippelli, M., Davinelli, S., Bartollino, S., Dell'Omo, R., and Costagliola, C. (2021). Influence of gut microbiota on eye diseases: an overview. Ann. Med. 53 (1), 750–761. doi:10.1080/07853890.2021.1925150
Nguyen, N. L. M., Wen, Y. T., Ho, Y. C., Kapupara, K., and Tsai, R. K. (2019). Therapeutic effects of Puerarin against anterior ischemic optic neuropathy through antiapoptotic and anti-inflammatory actions. Invest. Ophthalmol. Vis. Sci. 60 (10), 3481–3491. doi:10.1167/iovs.19-27129
Niwano, Y., Kanno, T., Iwasawa, A., Ayaki, M., and Tsubota, K. (2014). Blue light injures corneal epithelial cells in the mitotic phase in vitro. Br. J. Ophthalmol. 98 (7), 990–992. doi:10.1136/bjophthalmol-2014-305205
Ogawa, K., Kuse, Y., Tsuruma, K., Kobayashi, S., Shimazawa, M., and Hara, H. (2014). Protective effects of bilberry and lingonberry extracts against blue light-emitting diode light-induced retinal photoreceptor cell damage in vitro. BMC Complement. Altern. Med. 14, 120. doi:10.1186/1472-6882-14-120
Ogawa, K., Tsuruma, K., Tanaka, J., Kakino, M., Kobayashi, S., Shimazawa, M., et al. (2013). The protective effects of bilberry and lingonberry extracts against UV light-induced retinal photoreceptor cell damage in vitro. J. Agric. Food Chem. 61 (43), 10345–10353. doi:10.1021/jf402772h
Olchawa, M. M., Krzysztynska-Kuleta, O. I., Mokrzynski, K. T., Sarna, P. M., and Sarna, T. J. (2020). Quercetin protects ARPE-19 cells against photic stress mediated by the products of rhodopsin photobleaching. Photochem. Photobiol. Sci. 19 (8), 1022–1034. doi:10.1039/d0pp00165a
Osborne, N. N., Nunez-Alvarez, C., Del, O. S., and Merrayo-Lloves, J. (2017). Visual light effects on mitochondria: the potential implications in relation to glaucoma. Mitochondrion 36, 29–35. doi:10.1016/j.mito.2016.11.009
Ouyang, W., Wang, S., Yan, D., Wu, J., Zhang, Y., Li, W., et al. (2023). The cGAS-STING pathway-dependent sensing of mitochondrial DNA mediates ocular surface inflammation. Signal Transduct. Target. Ther. 8 (1), 371. doi:10.1038/s41392-023-01624-z
Ouyang, X., Yang, J., Hong, Z., Wu, Y., Xie, Y., and Wang, G. (2020). Mechanisms of blue light-induced eye hazard and protective measures: a review. Biomed. Pharmacother. 130, 110577. doi:10.1016/j.biopha.2020.110577
Ozkaya, E. K., Anderson, G., Dhillon, B., and Bagnaninchi, P. O. (2019). Blue-light induced breakdown of barrier function on human retinal epithelial cells is mediated by PKC-zeta over-activation and oxidative stress. Exp. Eye Res. 189, 107817. doi:10.1016/j.exer.2019.107817
Pan, Y., Mou, Z., and Shao, Y. (2023). Research progress on the mechanism of ocular damage caused by blue light. Int. Eye Sci. 23 (02), 208–211. doi:10.3980/j.issn.1672-5123.2023.2.05
Peng, J., Du, J., Zhong, Q., Liu, T., Yang, L., Wu, A., et al. (2019). Protect effect of Delphinidin on light induced oxidative damage of retina. Int. Eye Sci. 19 (10), 1657–1662. doi:10.3980/j.issn.1672-5123.2019.10.05
Puglia, C., Santonocito, D., Romeo, G., Intagliata, S., Romano, G. L., Strettoi, E., et al. (2021). Lipid nanoparticles traverse non-corneal path to reach the posterior eye segment: in vivo evidence. Molecules 26 (15), 4673. doi:10.3390/molecules26154673
Qin, S., Lu, Y., and Qin, B. (2019). Mechanism and protection of retina injured by blue light. Int. Eye Sci. 19 (10), 1696–1699. doi:10.3980/j.issn.1672-5123.2019.10.14
Qin, W., Guo, M., Li, X., Li, Y., Sun, X., Jin, J., et al. (2021). Research progress of Chinese herb monomers in the treatment of diabetic retinopathy. Int. Eye Sci. 21 (08), 1373–1377. doi:10.3980/j.issn.1672-5123.2021.8.12
Ren, D., Zhang, H., Wang, X., Xue, L., and Wu, W. (2017). Study on the synergistic antioxidant activity of lutein and zeaxanthin. Sci. Technol. Food Industry 38 (17), 296–299+304. doi:10.13386/j.issn1002-0306.2017.17.058
Sahin, K., Akdemir, F., Orhan, C., Tuzcu, M., Gencoglu, H., Sahin, N., et al. (2019a). (3R, 3'R)-zeaxanthin protects the retina from photo-oxidative damage via modulating the inflammation and visual health molecular markers. Cutan. Ocul. Toxicol. 38 (2), 161–168. doi:10.1080/15569527.2018.1554667
Sahin, K., Gencoglu, H., Akdemir, F., Orhan, C., Tuzcu, M., Sahin, N., et al. (2019b). Lutein and zeaxanthin isomers may attenuate photo-oxidative retinal damage via modulation of G protein-coupled receptors and growth factors in rats. Biochem. Biophys. Res. Commun. 516 (1), 163–170. doi:10.1016/j.bbrc.2019.06.032
Sayyad, Z., Sirohi, K., Radha, V., and Swarup, G. (2017). 661W is a retinal ganglion precursor-like cell line in which glaucoma-associated optineurin mutants induce cell death selectively. Sci. Rep. 7 (1), 16855. doi:10.1038/s41598-017-17241-0
Shaban, H., and Richter, C. (2002). A2E and blue light in the retina: the paradigm of age-related macular degeneration. Biol. Chem. 383 (3-4), 537–545. doi:10.1515/BC.2002.054
Shang, Y. M., Wang, G. S., Sliney, D., Yang, C. H., and Lee, L. L. (2014). White light-emitting diodes (LEDs) at domestic lighting levels and retinal injury in a rat model. Environ. Health Perspect. 122 (3), 269–276. doi:10.1289/ehp.1307294
Shen, Y., Zhang, W. Y., and Chiou, G. C. (2010a). Effect of naringenin on NaIO(3)-induced retinal pigment epithelium degeneration and laser-induced choroidal neovascularization in rats. Int. J. Ophthalmol. 3 (1), 5–8. doi:10.3980/j.issn.2222-3959.2010.01.02
Shen, Y., Zhao, H., Wang, Z., Guan, W., Kang, X., Tai, X., et al. (2019). Silibinin declines blue light-induced apoptosis and inflammation through MEK/ERK/CREB of retinal ganglion cells. Artif. Cell. Nanomed. Biotechnol. 47 (1), 4059–4065. doi:10.1080/21691401.2019.1671430
Shen, Y., Zhuang, P., Lin, B. Q., Zhang, W. Y., and Cy, C. G. (2010b). Effect of Tetramethylpyrazine on RPE degeneration, choroidal blood flow and oxidative stress of RPE cells. Int. J. Ophthalmol. 3 (3), 205–210. doi:10.3980/j.issn.2222-3959.2010.03.06
Shimizu, H., Takayama, K., Yamada, K., Suzumura, A., Sato, T., Nishio, Y., et al. (2022). Dimethyl fumarate protects retinal pigment epithelium from blue light-induced oxidative damage via the Nrf2 pathway. Antioxidants 12 (1), 45. doi:10.3390/antiox12010045
Singh, M., Negi, R., Vinayagam, R., Kang, S. G., and Shukla, P. (2024). Age-related macular degeneration (AMD): pathophysiology, drug targeting approaches, and recent developments in nanotherapeutics. Med. Lith. 60 (10), 1647. doi:10.3390/medicina60101647
Song, D., Song, J., Wang, C., Li, Y., and Dunaief, J. L. (2016). Berberine protects against light-induced photoreceptor degeneration in the mouse retina. Exp. Eye Res. 145, 1–9. doi:10.1016/j.exer.2015.10.005
Sugita, S., Mandai, M., Kamao, H., and Takahashi, M. (2021). Immunological aspects of RPE cell transplantation. Prog. Retin. Eye Res. 84, 100950. doi:10.1016/j.preteyeres.2021.100950
Tao, Y., He, M., Yang, Q., Ma, Z., Qu, Y., Chen, W., et al. (2019). Systemic taurine treatment provides neuroprotection against retinal photoreceptor degeneration and visual function impairments. Drug Des. Devel Ther. 13, 2689–2702. doi:10.2147/DDDT.S194169
Thomas, C. J., Mirza, R. G., and Gill, M. K. (2021). Age-related macular degeneration. Med. Clin. N. Am. 105 (3), 473–491. doi:10.1016/j.mcna.2021.01.003
Tosini, G., Ferguson, I., and Tsubota, K. (2016). Effects of blue light on the circadian system and eye physiology. Mol. Vis. 22, 61–72.
Ueda, K., Zhao, J., Kim, H. J., and Sparrow, J. R. (2016). Photodegradation of retinal bisretinoids in mouse models and implications for macular degeneration. Proc. Natl. Acad. Sci. U. S. A. 113 (25), 6904–6909. doi:10.1073/pnas.1524774113
Vaneev, A., Tikhomirova, V., Chesnokova, N., Popova, E., Beznos, O., Kost, O., et al. (2021). Nanotechnology for topical drug delivery to the anterior segment of the eye. Int. J. Mol. Sci. 22 (22), 12368. doi:10.3390/ijms222212368
van Norren, D., and Vos, J. J. (2016). Light damage to the retina: an historical approach. Eye 30 (2), 169–172. doi:10.1038/eye.2015.218
Varela-Fernandez, R., Diaz-Tome, V., Luaces-Rodriguez, A., Conde-Penedo, A., Garcia-Otero, X., Luzardo-Alvarez, A., et al. (2020). Drug delivery to the posterior segment of the eye: biopharmaceutic and pharmacokinetic considerations. Pharmaceutics 12 (3), 269. doi:10.3390/pharmaceutics12030269
Wang, G., Yu, F., Tao, Z., and Deng, S. (2024a). Research progresses on xanthophyll of marigold. J. Trop. Subtropical Bot., 1–10. doi:10.11926/jtsb.4893
Wang, H., Wang, M., Liu, Z., He, Y., Shi, J., and Zou, S. (2024b). Protective effect of a complex of blueberry, blackcurrant, medlar and Cassia obtusifolia against oxidative stress injury in retinal epithelial cells. Mod. Food Sci. Technol. 40 (01), 47–53. doi:10.13982/j.mfst.1673-9078.2024.1.1613
Wang, J. (2023). The role and mechanism of METTL7B in blue light induced apoptosis in retinal RPE cells. Master’s thesis. Guizhou Medical University.
Wang, L., Yu, X., Zhang, D., Wen, Y., Zhang, L., Xia, Y., et al. (2023). Long-term blue light exposure impairs mitochondrial dynamics in the retina in light-induced retinal degeneration in vivo and in vitro. J. Photochem. Photobiol. B-Biol. 240, 112654. doi:10.1016/j.jphotobiol.2023.112654
Wang, M., Zhang, C., and Lin, X. (2008). Protective effect of lutein against blue light-induced retinal damage in rat. J. Hyg. Res. 37 (04), 409–412. doi:10.3969/j.issn.1000-8020.2008.04.006
Wang, S., Li, Y., Zhang, Y., Chen, H., and Zhang, W. (2022). Effect of vitamin E on retinal pigment epithelial cells injured by high dose blue light. Int. Eye Sci. 22 (02), 189–193. doi:10.3980/j.issn.1672-5123.2022.2.03
Wang, W., Zhang, Y., Jin, W., Xing, Y., and Yang, A. (2018). Catechin weakens diabetic retinopathy by inhibiting the expression of NF-κB signaling pathway-mediated inflammatory factors. Ann. Clin. Lab. Sci. 48 (5), 594–600.
Wang, X., and Li, Z. (2021). Research progress of eye damage caused by short wave blue light. Med. Recapitulate 27 (01), 116–120. doi:10.3969/j.issn.1006-2084.2021.01.022
Wang, Y., Huo, Y., Zhao, L., Lu, F., Wang, O., Yang, X., et al. (2016). Cyanidin-3-glucoside and its phenolic acid metabolites attenuate visible light-induced retinal degeneration in vivo via activation of Nrf2/HO-1 pathway and NF-κB suppression. Mol. Nutr. Food Res. 60 (7), 1564–1577. doi:10.1002/mnfr.201501048
Wheway, G., Nazlamova, L., Turner, D., and Cross, S. (2019). 661W photoreceptor cell line as a cell model for studying retinal ciliopathies. Front. Genet. 10, 308. doi:10.3389/fgene.2019.00308
Wu, K. Y., Gao, A., Giunta, M., and Tran, S. D. (2024). What's new in ocular drug delivery: advances in suprachoroidal injection since 2023. Pharmaceuticals 17 (8), 1007. doi:10.3390/ph17081007
Xia, H., Hu, Q., Li, L., Tang, X., Zou, J., Huang, L., et al. (2019). Protective effects of autophagy against blue light-induced retinal degeneration in aged mice. Sci. China-Life Sci. 62 (2), 244–256. doi:10.1007/s11427-018-9357-y
Xiang, Y., Zou, M., Zhang, Y., Jin, R., and Nie, Y. (2020). Drug-loaded and blue-ray filtered hydrogel as a potential intraocular lens for cataract treatment. Pharm. Nanotechnol. 8 (4), 302–312. doi:10.2174/2211738508666200313144112
Xie, T., Cai, J., Yao, Y., Sun, C., Yang, Q., Wu, M., et al. (2021). LXA4 protects against blue-light induced retinal degeneration in human A2E-laden RPE cells and Balb-c mice. Ann. Transl. Med. 9 (15), 1249. doi:10.21037/atm-21-3390
Xu, J. (2008). The effect of artificial light on the eye and biothythm and traditional Chinese medicine intervention function. Dissertation. Nanjing University of Chinese Medicine.
Xu, J., and Wang, Y. (2007). Progress in the study of blue light injury of the retina. Int. Eye Sci. (04), 1107–1109. doi:10.3969/j.issn.1672-5123.2007.04.065
Xu, Z., Jiang, Y., Zhao, Z., Li, W., and Sun, X. (2018). Photooxidation-induced damage in retinal pigment epithelium and protective effect of procyanidins B2. J. Shanghai Jiaot. Univ. Sci. 36 (01), 36–43. doi:10.3969/j.issn.1671-9964.2018.01.007
Yamazaki, K., Ishida, K., Otsu, W., Muramatsu, A., Nakamura, S., Yamada, W., et al. (2024). Delphinidins from Maqui Berry (Aristotelia chilensis) ameliorate the subcellular organelle damage induced by blue light exposure in murine photoreceptor-derived cells. BMC Complement. Med. Ther. 24 (1), 3. doi:10.1186/s12906-023-04322-z
Yan, W. (2006). Injurious effect of low level laser irradiation and therapeutic effect of MK-801 in retinal laser injury. Master’s thesis. Academy of Military Science.
Yang, J., Peng, H., Chen, Q., and Jiang, Y. (2011). Effect of tripterine on expression of VEGF and PEDF of retinal endothelial cells in mouse with high glucose. Recent Adv. Ophthalmol. 31 (04), 321–323+331. doi:10.13389/j.cnki.rao.2011.04.006
Yang, J., Zhang, Y., Zhang, L., Liao, Z., Hou, Y., Zhan, Z., et al. (2020). The protective effect of lutein against retinal damage induced by blue light emitting diodes(LEDs) in rats. Food Nutr. China. 26 (06), 54–58. doi:10.19870/j.cnki.11-3716/ts.2020.06.013
Yang, S., Wang, C., and Qi, S. (2023). Research progress on the mechanism of retinal light injury. Int. Eye Sci. 23 (06), 938–942. doi:10.3980/j.issn.1672-5123.2023.6.11
Ye, M. J., and Meng, N. (2021). Resveratrol acts via the mitogen-activated protein kinase (MAPK) pathway to protect retinal ganglion cells from apoptosis induced by hydrogen peroxide. Bioengineered 12 (1), 4878–4886. doi:10.1080/21655979.2021.1954742
Yin, J., Thomas, F., Lang, J. C., and Chaum, E. (2011). Modulation of oxidative stress responses in the human retinal pigment epithelium following treatment with vitamin C. J. Cell. Physiol. 226 (8), 2025–2032. doi:10.1002/jcp.22532
Yu, H. (2019). Effect of drugs on retinal pigment epithelial cell damage injury Models. Master’s thesis. Southeast University.
Yu, Y., Cheng, T., Zou, X., Zhang, M., Yu, Y., Zou, Y., et al. (2023a). Experimental study of chronic retinal damage induced by blue light exposure in Brown Norway rats. Tianjin Med. J. 51 (11), 1193–1198. doi:10.11958/20230030
Yu, Y., Zhou, W., Yu, Z., Zhang, M., Cheng, T., Zou, X., et al. (2023b). Protective effects of α-lipoic acid on blue light-induced damage in ARPE-19 cells. Recent Adv. Ophthalmol. 43 (10), 770–774. doi:10.13389/j.cnki.rao.2023.0155
Zhang, B., Rusciano, D., and Osborne, N. N. (2008). Orally administered epigallocatechin gallate attenuates retinal neuronal death in vivo and light-induced apoptosis in vitro. Brain Res. 1198, 141–152. doi:10.1016/j.brainres.2007.12.015
Zhang, C. (2023a). Comparative study of retinal damage induced by laser irradiation at wavelengths of 650 nm and 450 nm and the role of PARP-1 in blue light damage. Dissertation. Jilin University.
Zhang, C., Chen, Q., Zhou, W., Li, Z., Yu, Y., Deng, Q., et al. (2022). Protective effect and mechanism of miR-22-3p on rat retinal ganglion cells exposed to blue light. Recent Adv. Ophthalmol. 42 (10), 780–785. doi:10.13389/j.cnki.rao.2022.0160
Zhang, H., Song, T., Kang, R., Ren, F., Liu, J., and Wang, J. (2023a). Plant bioactive compounds alleviate photoinduced retinal damage and asthenopia: mechanisms, synergies, and bioavailability. Nutr. Res. 120, 115–134. doi:10.1016/j.nutres.2023.10.003
Zhang, P. (2023b). Exploring the efficacy and mechanism of taxifolin on blue light induced dry age-related macular degeneration. Master’s thesis. Jiangxi University of Chinese Medicine.
Zhang, S. (2023c). Inhibiting PARP-1 can suppress mitophagy and synergistically protect the retina from light damage. Dissertation. Jilin University.
Zhang, Y., Shi, Y., Khan, M. M., Xiao, F., Chen, W., Tao, W., et al. (2024). Ocular RNA nanomedicine: engineered delivery nanoplatforms in treating eye diseases. Trends Biotechnol. 42, 1439–1452. doi:10.1016/j.tibtech.2024.05.002
Zhang, Y., Wang, T., Wan, Z., Bai, J., Xue, Y., Dai, R., et al. (2023b). Alterations of the intestinal microbiota in age-related macular degeneration. Front. Microbiol. 14, 1069325. doi:10.3389/fmicb.2023.1069325
Zhang, Z. (2019). Effect of matrine on proliferation of retinal microvascular endothelial cell and expression of vascular endothelial growth factor in rats with diabetic retinopathy. Eval. Analysis Drug-Use Hosp. China 19 (04), 422–425+428. doi:10.14009/j.issn.1672-2124.2019.04.011
Zhao, L., Wang, C., Song, D., Li, Y., Song, Y., Su, G., et al. (2014). Systemic administration of the antioxidant/iron chelator α-lipoic acid protects against light-induced photoreceptor degeneration in the mouse retina. Invest. Ophthalmol. Vis. Sci. 55 (9), 5979–5988. doi:10.1167/iovs.14-15025
Zhao, Z. (2015). Grape procyanidins B2 protect RPE cells against photooxidative damage. Master’s thesis. Shanghai Jiao Tong University.
Zhao, Z. C., Zhou, Y., Tan, G., and Li, J. (2018). Research progress about the effect and prevention of blue light on eyes. Int. J. Ophthalmol. 11 (12), 1999–2003. doi:10.18240/ijo.2018.12.20
Zhu, J., Inomata, T., Shih, K. C., Okumura, Y., Fujio, K., Huang, T., et al. (2022). Application of animal models in interpreting dry eye disease. Front. Med. 9, 830592. doi:10.3389/fmed.2022.830592
Zhu, S. (2022). Protective effects of hydrogen sulfide/lycium barbarum polysaccharide on blue light/PM2.5-induced oxidative damage. Master’s thesis. Lanzhou University.
Zhu, Y. (2021). Study on the light damage of SD rat's retina by different wavelength of LED lights. Master’s thesis. Tianjin Medical University.
Zhuang, H., Wu, Z., Chen, X., and Li, C. (2022). CERKL attenuates blue light-induced oxidative stress in human retinal pigment epithelial cells by activating the SIRT1/E2F1 axis. Int. Eye Sci. 22 (08), 1245–1251. doi:10.3980/j.issn.1672-5123.2022.8.02
Ziolkowska, N., Lewczuk, B., Szyrynska, N., Rawicka, A., and Vyniarska, A. (2023). Low-intensity blue light exposure reduces melanopsin expression in intrinsically photosensitive retinal ganglion cells and damages mitochondria in retinal ganglion cells in wistar rats. Cells 12 (7), 1014. doi:10.3390/cells12071014
Keywords: eye, blue light damage, pharmacological mechanism, modeling conditions, research direction
Citation: Yan Y, Wu Y, Zhao Y, Yang Y, An G, Liu Z and Qi D (2025) A review on eye diseases induced by blue light: pathology, model, active ingredients and mechanisms. Front. Pharmacol. 16:1513406. doi: 10.3389/fphar.2025.1513406
Received: 18 October 2024; Accepted: 02 January 2025;
Published: 23 January 2025.
Edited by:
Hua Yang, China Pharmaceutical University, ChinaReviewed by:
Giovanni Luca Romano, Kore University of Enna, ItalyXinggang Yang, Shenyang Pharmaceutical University, China
Copyright © 2025 Yan, Wu, Zhao, Yang, An, Liu and Qi. This is an open-access article distributed under the terms of the Creative Commons Attribution License (CC BY). The use, distribution or reproduction in other forums is permitted, provided the original author(s) and the copyright owner(s) are credited and that the original publication in this journal is cited, in accordance with accepted academic practice. No use, distribution or reproduction is permitted which does not comply with these terms.
*Correspondence: Zhidong Liu, bGl1emhpZG9uZ0B0anV0Y20uZWR1LmNu; Dongli Qi, cWlkb25nbGlAdGp1dGNtLmVkdS5jbg==
†These authors have contributed equally to this work and share first authorship