- 1Department of Neurological Function Examination, The Fourth Affiliated Hospital of China Medical University, Shenyang, China
- 2Endoscopy Center, The Fourth Affiliated Hospital of China Medical University, Shenyang, China
- 3Fifth Department of General Surgery, The Fourth Affiliated Hospital of China Medical University, Shenyang, China
- 4Second Department of General Surgery, The Fourth Affiliated Hospital of China Medical University, Shenyang, China
Alzheimer’s disease (AD) is one of the most common diseases characterized by neurodegeneration and is becoming a major public health problem worldwide. AD is manifested mainly by progressive impairments in cognition, emotion, language and memory in the elderly population. Many treatment strategies have been explored for decades; however, there is still no effective way to address the root cause of AD pathogenesis, only to target symptoms to improve patient cognitive outcomes. Intracerebral administration is difficult because of the challenges posed by the blood‒brain barrier (BBB). NPs are materials with sizes between 1 and 100 nm that can improve biocompatibility, extend the half-life, transport macromolecules, be delivered across the BBB to the central nervous system, and exhibit good targeting capabilities. NPs can provide new ideas for the treatment of AD in terms of their antiaging, antineuroinflammatory, antioxidative, and nerve repair-promoting effects. In this manuscript, we first describe the relationship between AD and the BBB. Second, we introduce the application of nanoparticles for AD treatment. Finally, we summarize the challenges faced by nanoparticles in the treatment of AD.
1 Introduction
Alzheimer’s disease (AD) is a neurodegenerative disease characterized by progressive and irreversible degeneration of the brain, resulting in cognitive impairment and memory decline as the main clinical symptoms (Graff-Radford et al., 2021). The onset of AD usually involves excessive accumulation of β-amyloid (Aβ) and hyperphosphorylated tau protein in the brain, which ultimately leads to neuronal death and cognitive decline (Perneczky et al., 2024). AD is considered to be the most common and challenging mental disorder in society as a whole (Huang et al., 2024). As of 2020, approximately 40 million people worldwide are affected by AD, and this number is increasing annually (Zhang et al., 2023). AD is the leading cause of dementia in older adults, accounting for approximately two-thirds of cases in women compared with men (Zhang, 2023; Lopez-Lee et al., 2024). The onset of AD is thought to be age-related, especially after age 65, with studies showing that more than one in 10 people over the age of 65 have AD (Liu Y. et al., 2024). AD causes severe progressive cognitive impairment, neurobehavioral symptoms that are severe enough to have a significant functional impact on daily life, and patients with severe disease often lose independence (Scheltens et al., 2021). AD not only has a considerable social impact but also increases the economic burden on the global healthcare system to be of paramount importance to healthcare systems (Liu N. et al., 2024).
The treatment of AD is a long-term process, and the current medical community mainly uses drugs and psychotherapy to control the progression of the disease and delay the worsening of symptoms (Liu N. et al., 2024). Cholinesterase inhibitors (donepezil, rivastigmine) and glutamate receptor antagonists (memantine) are commonly used in clinical practice to treat cognitive symptoms (Buccellato et al., 2023). In addition, antipsychotics can also reduce symptoms in the treatment of AD. However, medical therapy can only temporarily relieve symptoms and cannot alter the natural history of AD patients (Aili et al., 2023). In recent years, novel antibody therapies targeting Aβ have been able to significantly reduce the production of Aβ and achieve tangible clinical benefits (Cummings et al., 2023). Some small molecule inhibitors, immunotherapies, and Tau-directed therapies also have potential trends in AD treatment (Liu N. et al., 2024). However, the blood‒brain barrier (BBB), which is composed of a network of capillaries that isolate the brain from blood circulation, prevents many drugs from crossing the BBB (Tosi et al., 2016). Drug treatment for AD is still challenging because of the restrictive nature of the blood‒brain barrier (BBB), which hinders the entry of drugs, thus affecting their efficacy and bioavailability (Elmahboub and Elkordy, 2024; Zhong et al., 2022; Rajendran and Krishnan, 2024). Currently, there is no better way to prevent cognitive decline, and treatment for AD is limited.
In recent years, nanomedicine has played an important role in the synthesis and delivery of novel drugs and has been widely used in the biomedical field (Xia et al., 2023a; Xia Y. et al., 2022; Liu et al., 2023). Nanoparticle-loaded drugs can be transported across the BBB to the affected site, increasing the concentration of the drug at the lesion site and thereby improving drug utilization and treatment efficiency (Liu et al., 2023). In this work, we introduce the pathogenesis of AD and introduce the different mechanisms of action of nanoparticles in the treatment of AD (Schemes 1, 2; Table 1), which may provide more opportunities for the therapeutic development of AD in the future.
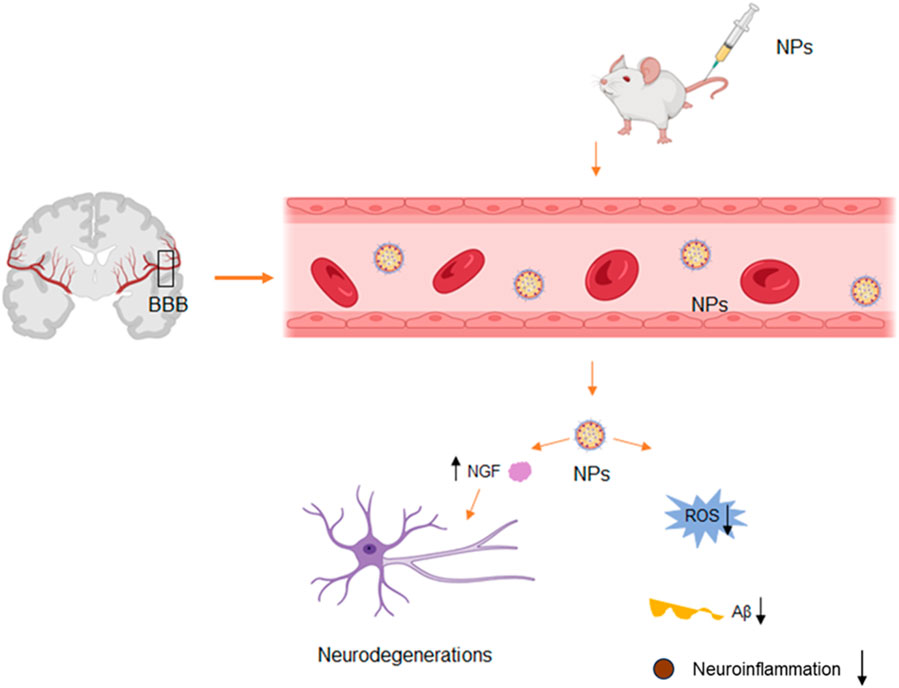
Scheme 1. Nanoparticles penetrate the blood-brain barrier to treat Alzheimer’s disease. Nanoparticles are injected into mice through the tail vein and can penetrate the BBB to the lesion site. Subsequently, it causes a decrease in local ROS, reduces nerve inflammation, secretes nerve regeneration factors to promote nerve regeneration, and decreases Aβ.

Scheme 2. The main pathogenesis of Alzheimer’s disease is the excessive accumulation of amyloid (Aβ) and hyperphosphorylated tau in the brain. Nanoparticles can reduce Aβ and tau protein phosphorylation through anti-aging, anti-inflammatory, antioxidant and promote nerve regeneration.
2 Alzheimer’s disease and the blood‒brain barrier
AD is a common chronic neurodegenerative disease characterized by widespread neuronal tangles and amyloid plaques in the brain (De Strooper and Karran, 2016). Aβ and hyperphosphorylated tau protein and neurofilament light chain (NfL) are promising biomarkers for the pathogenesis of AD (Cai et al., 2023). These dense and water-insoluble deposits are deposited in the brain, causing physical damage to surrounding cells (McManus and Latz, 2024). These plasma biomarkers can precisely distinguish AD patients from those who are normal or have other neurodegenerative diseases (Palmqvist et al., 2019). Aβ is formed by sequential cleavage of amyloid precursor proteins by β and γ secretases and is the first protein deposited in the brain (Rajmohan and Reddy, 2017). Aβ deposition triggers a cascade of reactions and progressively leads to Tau pathology, synaptic dysfunction, inflammation, neuronal loss, and eventually dementia (Benilova et al., 2012). With the deposition of large amounts of Aβ, Tau hyperphosphorylation occurs, and these isomers multiply widely to form amyloid plaques and produce neuronal tangles (Cao et al., 2024). These abnormal proteins block neurotransmitter signaling and gradually lead to neurodegeneration (Prajapati et al., 2024). AD is a slowly progressing neurological disease, and autophagy, inflammation, and neurovascular unit dysfunction have been reported to be closely related to the pathogenesis of AD (Prajapati et al., 2024).
The blood‒brain barrier (BBB) is the barrier between the walls of the cerebral capillaries and the plasma formed by glial cells and between brain cells and the plasma and cerebrospinal fluid formed by the choroid plexus; this barrier prevents certain substances (mostly harmful) from entering the brain tissue from the bloodstream (Zapata-Acevedo et al., 2024). The BBB blocks the entry of substances through four main mechanisms: (1) endothelial cells, which are the main functional components of the BBB; (2) enzyme barriers of metabolites; (3) efflux transporter barriers; and (4) immune cells, which form an immune barrier (Gao et al., 2023). More than 98% of small-molecule drugs, including recombinant proteins, peptides, nucleic acids, and monoclonal antibodies, cannot cross the BBB (Chen Y. X. et al., 2020). Therefore, crossing the BBB with drugs is a major challenge in the treatment of AD. There is a strong correlation between microvascular dysfunction in the brain and the pathogenesis of AD (Govindpani et al., 2019). Vascular abnormalities in the AD brain manifest themselves in the early stages of pathology and are characterized by altered vascular morphology, reduced cerebral blood flow (CBF), damage to neurovascular units (NVUs), inflammation of the vasculature, and cerebral amyloid angiopathy, which promote the infiltration of harmful molecules into the brain parenchyma, leading to neuroinflammation and neuronal dysfunction in AD (Lei et al., 2024). The BBB breakdown may precede cognitive decline and neurodegeneration, highlighting the critical need for further exploration of the BBB’s role in AD and its potential as a therapeutic target (Zlokovic, 2008). Because BBB destruction is associated with aging, it does not cause cognitive impairment in patients unless accompanied by inflammation (Alkhalifa et al., 2023). Therefore, the restoration of BBB function is essential for both improving AD pathology and restoring brain function.
3 Application of nanoparticles in AD
Nanomaterial delivery strategies have made significant progress in tissue engineering and regenerative medicine (Xia et al., 2023a; Xia Y. et al., 2022). Compared with other drug carriers, nanoparticles exhibit excellent biocompatibility, drug loading capacity, drug delivery efficiency via the BBB, and the ability to target brain damage (Passeri et al., 2022). In addition, nanoparticles have lower immunogenicity and better stability. Nanoparticles have been reported in the treatment of AD.
3.1 Anti-aging
Microglia are responsible for phagocytosis of the central nervous system and play important roles in maintaining homeostasis and protecting the brain from infection and damage (Fu et al., 2014). In the AD microenvironment, microglia not only recognize the Aβ peptide and initiate an immune response but also migrate to areas of amyloid deposition (Bolmont et al., 2008). The environment of AD can accelerate the senescence of microglia, which are dysplastic with abnormal loss, enhancement, and distortion of the cytoplasm, leading to a decrease in their phagocytic capacity (Grubman et al., 2021). Xiao et al. used polyD,L-lactic acid-glycolic acid copolymer (PLGA) nanoparticles targeting microglia to deliver p16ink4a siRNA to delay microglial senescence (Shin et al., 2024). PLGA nanoparticles have been reported to target microglia (Shin et al., 2018). Owing to their ability to phagocytose microglia, PLGA nanoparticles release siRNA after phagocytosis into cells, rejuvenating senescent microglia. The particle size of the PLGA nanoparticles was 240.4 ± 58.49 nm. The embedding efficiency was 31.8% ± 0.1%. In vivo experiments in rats revealed that the highest release rate was maintained from day 1 to day 5 after intravenous infusion of PLGA nanoparticles. Compared with those in the control group, the number of microglia in the PLGA nanoparticle group was 3.4-fold greater, and the phagocytic capacity of Aβ was increased.
Ji et al. synthesized senescence-specific killer compound 1 (SSK1), which can be hydrolyzed by β-galactosidase to induce apoptosis in senescent cells (Cai et al., 2020). Ji et al. used lipid nanoparticles to load SSK1 to form SSK1-NPs, which significantly reduced the expression of senescence-related genes, induced senescent cell elimination, and reduced lake Aβ accumulation (Ji et al., 2024). SSK1-NPs have a minimum diameter of 166 ± 5 nm and are able to cross the BBB and be deposited in the brain 1 h after intravenous administration. SSK1-NPs are able to release SSK1 when they enter the brain. Studies have shown that SSK1-NPs release 50% of SSK1 in the first 2 h and that 88% of SSK1 is gradually released after 24 h. After 8 weeks of intravenous infusion of SSK1-NPs into rats, SSK1 was induced to eliminate senescent cells in the brains of AD mice via the p38‒p21 axis (Gasek et al., 2021).
Aβ is produced by the amyloid precursor protein (APP), which is proteolytically cleaved by the β-site APP lyase 1 (BACE1) (El Andaloussi et al., 2013). SiRNAs targeting BACE1 can improve Aβ plaques and delay nerve damage in AD (Lane et al., 2018). However, the disadvantage of SiRNAs being unstable and very easy to lyse within cells limits their application (Aly and Waszczak, 2015). Li et al. developed mesenchymal stem cell (MSC)-derived exosomes for SiRNA delivery, while intranasal administration bypassed the BBB to achieve accumulation in the brain (Li et al., 2023). To increase the targeting of exosomes, Li et al. modified exosomes with poly-[(2-methacryloyl)ethyl (benzyl p-borate)dimethylammonium bromide] (BA-PDMAEMA (BAP)) to target ROS (BAP@siRNAs). BAP@siRNAs downregulated the levels of BACE1 and caspase-3 in AD-diseased cells, thereby reducing Aβ plaques and inhibiting neuronal apoptosis. The hydrodynamic diameter of the BAP@siRNAs was approximately 167.6 nm, and the potential was −6.85 mV. BAP@siRNAs are able to release siRNAs in a high-ROS environment. In vivo experiments in mice confirmed that BAP@siRNAs could significantly improve the cognitive ability of AD model mice 1 month after intranasal administration.
3.2 Reducing neuroinflammation
Although neuroinflammation is often not associated with the occurrence of AD, it can significantly worsen the disease by aggravating Aβ and tau pathologies (Chitnis and Weiner, 2017). Neuroinflammation has increasingly been recognized as a key factor in the pathogenesis of AD (Chen H. Y. et al., 2020). Neuroinflammation severely leads to BBB destruction during AD, increased ROS production, endothelial cell dysfunction, and stromal metalloproteinase (MMP) activation (Alkhalifa et al., 2023). Pro-inflammatory cytokine signaling plays a variety of roles in neurodegeneration and neuroprotection. The induction of pro-inflammatory signaling leads to the release of immune mediators, which affect the function of neurons and lead to cell death (Thakur et al., 2023). Glial cell activation is a central feature of CNS neuroinflammation. These glial cells can produce and release a variety of proinflammatory cytokines, chemokines, and reactive oxygen species (Singh, 2022). Chemokine (C-C motif) ligand 2 (CCL2) is a chemokine produced by astrocytes that can recruit cells expressing CCL2 receptor 2 (CCR2) around AD to the site of injury (Bose and Cho, 2013). 1-Trifluoromethoxyphenyl-3-(1-propionylpiperidin-4-yl)urea (TPPU) is a soluble epoxide hydrolase (sEH) inhibitor that has been reported to prevent glial cell reactivation and alleviate cognitive deficits in the brains of AD mice (Ghosh et al., 2020). Rapamycin is a potential small molecule that enhances autophagy in AD and accelerates the clearance of necrotic nerves (Majumder et al., 2011). Lin et al. developed platelet cell membrane liposomes carrying TPPU and rapamycin (TR@CPL) (Lin et al., 2024). Three months after TR@CPL injection into mice through the tail vein, there was less glial infiltration and reduced neuroinflammation. The transcription factor PU.1 is highly expressed in microglia and is positively correlated with the development of AD (Penney et al., 2020). Lowering PU.1 expression is considered an effective way to protect against AD (Pimenova et al., 2021). RNA therapeutics can ameliorate AD-associated transcriptional and translational changes in microglia. To increase siRNA protection and BBB permeability, William et al. utilized lipid nanoparticles to deliver PU.1 siRNAs (Ralvenius et al., 2024). Local intrathecal injection of liposomal nanoparticles enhances mRNA delivery within the mouse brain, achieving up to 92% ± 2% PU.1 transcriptional silencing within 12 h in mice. Thereby reducing the expression of neuroinflammatory factors in mice.
Aβ vaccines reduce the long-term accumulation of Aβ by inducing the production of polyclonal anti-Aβ antibodies (Muhs et al., 2007). Regulatory T cells (Tregs) can attenuate neuroinflammation in AD by secreting anti-inflammatory cytokines such as transforming growth factor-β (TGF-β) and interleukin (IL)-10 (Kang et al., 2019). Zheng et al. developed therapeutic nanovaccine lipid nanoparticles (LNP-R/Aβs) loaded with rapamycin and Aβ (Jung et al., 2023). The nanovaccine efficiently delivers rapamycin and Aβ peptides to dendritic cells, produces anti-Aβ antibodies and Aβ-specific Treg cells, alleviates neuroinflammation, and inhibits cognitive impairment in mice. The size of LNP-R/Aβ is 232.7 ± 84.0 nm, and the zeta potential is 9.28 ± 4.74 mV. LNP-R/Aβ accumulates in the lymph nodes of mice 1 day after injection. In mice, LNP-R/Aβ treatment was confirmed to induce Aβ-specific Treg cells in AD, as high levels of Treg cell markers (Foxp3) and anti-inflammatory cytokines (TGF-β and IL-10) were detected, significantly reducing the number of Aβ plaques. However, the Aβ vaccine has achieved some efficacy in preclinical studies. However, United States FDA approval is controversial because the therapeutic effects on cognitive ability barely meet clinical endpoints and are limited to specific patient subpopulations. Therefore, Aβ vaccines still need to address a series of issues before they can be applied for clinical treatment.
3.3 Antioxidants
Aβ itself can induce oxidative stress, which also leads to increased production of Aβ (Miller et al., 2009). ROS are products of oxidative stress, which can cause mitochondrial dysfunction and induce neuroinflammation to play an important role in the development of AD (Bai et al., 2022). Elevated ROS concentrations promote tau phosphorylation. Tau protein is hyperphosphorylated, affecting mitochondrial function, causing synaptic dysfunction and even neuronal death which leads to decreased synaptic function, leading to apoptosis and subsequent neurodegeneration (Sen et al., 2018; Mashal et al., 2022). Studies have shown that elevated ROS often precedes Aβ deposition and neurofibrillary tangles (Butterfield and Boyd-Kimball, 2018). Lowering ROS is essential for slowing neurodegeneration in the brains of AD patients (Ionescu-Tucker and Cotman, 2021). Resveratrol (3,5,40-trihydroxystilbene, RES) has potential in the treatment of AD because of its anti-inflammatory, oxidative stress and immunomodulatory effects (Moussa et al., 2017). RES is poorly soluble in water and has low bioavailability, so it is difficult for RES to cross the BBB (Zhang et al., 2021). Cerium oxides (CeO2 and Ce2O3) have excellent biocompatibility and have antioxidant capacity (Bao et al., 2018). Yu et al. synthesized manganese-doped CeO2-doped mesoporous hollow nanoparticle (LMC-RES)-supported RES for the removal of ROS in an AD environment (Hu et al., 2023). The particle size of LMC-RES is approximately 120 nm, and the drug loading for RES is 39.8% ± 3.65. LMC-RES had a good sustained-release effect and good biocompatibility, and the drug release rate of LMC-RES at 24 h was 80.9% ± 2.25%. In a rat model of AD, LMC-RES can cross the BBB and be enriched in neurons, inhibiting oxidative stress and reducing ROS production through the Nrf-2/HO-1 signaling pathway.
The ability of Prussian blue (PB) to scavenge ROS is attributed to its affinity for hydroxyl radicals (Zhang et al., 2016). The ability of PB to scavenge ROS effectively has a potential neuroprotective effect on AD (Zhang et al., 2016). Poly(amide amine) (PAMAM) dendritic polymers can enhance the ability of PB to penetrate the BBB. Angiopep-2 (ANG-2), a ligand that targets low-density lipoprotein receptor-associated protein-1 (LPR-1) on the surface of cerebral capillary endothelial cells, can be coupled to functional groups on the surface of PAMAM to enhance the targeting of nanoparticles (Zou et al., 2020). Zhong et al. combined ANG-2-modified PAMAMs with PB nanoparticles to make PPA NPs improve the BBB transport efficiency of PB (Zhong et al., 2022). PPA NPs have a zeta potential of +19 mV and are noncytotoxic in mice. PPA NPs can be deposited in brain tissue via the BBB after 4 h of injection into mice via the tail vein. PPA NPs are able to activate mitophagy to eliminate abnormal mitochondria that produce large amounts of ROS. Breakthroughs have been made in the antioxidant nature of metal nanoparticles and their oxides, such as zinc oxide nanoparticles (El-Sayed et al., 2023). Zinc ions can reduce the incidence of infections as well as the production of inflammatory cytokines (Hussein et al., 2022). El-Sayed et al. reported that zinc oxide nanoparticles have a unique effect on acetylcholinesterase inhibition (El-Sayed et al., 2020). Elmonem et al. reported that zinc oxide nanoparticles ameliorate the neurotoxic effects of AD by decreasing IL-1β, TNF-α, and acetylcholinesterase activity and increasing glutathione production (Abd Elmonem et al., 2023).
Curcumin can activate Akt phosphorylation, which reduces Aβ production and plaque deposition, downregulate the production of AD-related cytokines (Ege, 2021). The poor bioavailability of curcumin limits its clinical application because of the difficulty of passing through the BBB (Xia et al., 2023a). PLGA is a copolymer with excellent biocompatibility and biodegradability (Xia Y. L. et al., 2022). Anila et al. developed curcumin-encapsulated PLGA nanoparticles for the treatment of AD (Mathew et al., 2012). PLGA-curcumin nanoparticles have particle sizes between 150 and 200 nm and zeta potentials in the range of −30 to −20 mV. PLGA-curcumin nanoparticles have nearly 60% free radical scavenging activity. Quercetin (QT) inhibits AchE, which prevents acetylcholine degradation and leads to a decrease in Aβ aggregate production that mitigates AD progression (Khan et al., 2020). QT is poorly bioavailable and often requires large doses to be effective (Ratnam et al., 2006). Sun et al. developed PLGA nanoparticles (PLGA@QT NPs) for QT encapsulation for the treatment of AD (Sun et al., 2016). PLGA@QT NPs have an average diameter in the range of approximately 100–150 nm and a QT loading rate of 35%. In vitro, the NPs were shown to release 100% of the QT within 2 h of PLGA@QT NP treatment. In mice, PLGA@QT NPs were shown to delay the progression of AD.
3.4 Induction of nerve repair
Nerve growth factor (NGF) promotes cranial nerve repair, prevents nerve damage and restores nerve cell function (Xia et al., 2023b). NGF is widely used in neuroregenerative diseases (Wang et al., 2022). However, NGF alone is readily degraded by biological enzymes during delivery and has difficulty crossing the blood‒brain barrier (BBB) to reach the lesion to function (Kuo et al., 2017). The brain-targeted peptide RVG has been widely used to improve the BBB penetration efficiency of drugs. Yuan et al. utilized a diselenide-rich ROS-responsive nanoplatform (R@NGF-Se-Se-Ru) drug delivery system to deliver NGF (Yuan et al., 2021). Under near-infrared (NIR) irradiation, R@NGF-Se-Se-Ru has good photothermal properties and decomposes nanoclusters into small nanoparticles. Due to the targeting ability of RVG, these nanoparticles can penetrate the BBB, which can effectively inhibit the aggregation of Aβ and promote neurite outgrowth, thereby delaying the progression of AD. R@NGF-Se-Se-Ru has an average particle size of 80 nm and loads of 22.2 and 13 mmol/mg for RVG and NGF, respectively. Some degree of neuronal recovery was detected after the R@NGF-Se-Se-Ru tail vein was injected into the mice, which were then subjected to NIR irradiation for 4 days. Metal‒organic frameworks (MOFs) have emerged as drug carriers for the delivery of organic molecules, biomacromolecules, and nanoparticles (Chen et al., 2018). Yu et al. developed nanoparticles using the antioxidant cerium for codelivery of siSOX9 and retinoic acid (RA) into NSCs for nerve repair in AD (Yu et al., 2020). The SOX9 protein is an essential transcription factor that benefits gliogenesis, and siSOX9 can downregulate the expression of the SOX9 protein (Doerks et al., 2002). RA has the ability to upregulate neuronal gene expression. The diameter of the nanocomposite is approximately 100–200 nm, and the loading efficiency of siSOX9 is as high as 90.1% (Doerks et al., 2002). In vitro use confirmed that 40% of the RA was released from the nanoparticles within 5 h. Cerium dioxide nanoparticles can act as ROS scavengers and can significantly reduce the intracellular levels of ROS. At week 5 in the annotated mice, the nanocomplexes effectively promoted the differentiation of NSCs into neurons and led to alleviation of oxidative stress, decreased neuronal apoptosis, and increased neurite length (Doerks et al., 2002).
Huang et al. genetically engineered neural stem cells (NSCs) to stably express the Aβ-degrading protease NEP, which is expressed on the cell membrane of NSCs and released into exosomes (Huang et al., 2021). To increase muscle delivery efficiency, Huang et al. designed a highly efficient gene and drug delivery nanopreparation (PPAR-siSOX9) using PBAE-PLGA to improve the neuronal differentiation efficiency of NEP-NSCs in the pathological AD microenvironment. Decreased SOX9 expression inhibits the degradation of β-catenin in cells, which further activates the Wnt/β-catenin pathway and promotes the differentiation of NSCs into neurons (Iwata et al., 2000). The nanoformulation has a spherical morphology with a diameter of ≈150 nm and a zeta potential of +23.6 mV (Iwata et al., 2000). Compared with the blank control group, the nanopreparative group differentiated and produced more neurons (69.4%) after 10 days in vitro. In one-month-old mice, nanopreparation significantly improved cognitive function to a greater degree (Iwata et al., 2000).
Naringenin is a ketone widely found in fruits, which has antioxidant, free radical scavenging, anti-cancer, and anti-inflammatory effects (Motallebi et al., 2022). Fakhrul et al. demonstrated that Naringenin has a neuroprotective effect and can treat cognitive impairment in AD (Khan et al., 2012). Shadab et al. developed the naringenin nanoemulsion with Naringenin (Md et al., 2018). The study showed that the nanoemulsion formulation loaded with naringenin had a droplet size of 113.83 nm. In mice, it was confirmed that naringenin nanoemulsion loaded with Naringenin significantly reduced Aβ-induced neurotoxicity and effectively induced nerve repair. Li et al. designed a composite nanomaterial of Prussian blue nanoparticles (PB NPs/MM) encapsulated in macrophage membranes (Li et al., 2025). Macrophage membranes have stronger targeting capabilities and more efficient immune evasion than nanoliposomes (Xi et al., 2022). The excellent photothermal capability of PB NPs can briefly open the BBB under near-infrared laser irradiation, thereby enhancing the transmission efficiency of PB NPs/MM in the BBB and ablating Aβ deposits (Liu et al., 2021). Under the condition of photothermal treatment, PB NPs/MM not only cleared the surrounding inflammation by the enrichment of the lesion site, but also promoted the nerve repair function of the mouse model of advanced AD.
4 Facing challenges
Neurological symptoms due to AD continue to be a challenge for clinicians. Drug therapy has controlled the progression of AD to some extent (Pena et al., 2020). Drug delivery to the brain remains a challenge because of the presence of the BBB, lipophilicity, high molecular weight of the drug, and other factors. Therefore, nanoparticle-mediated delivery of targeted drugs to the brain to delay AD progression has been explored in recent years. As a promising strategy, nanoparticles can reduce enzymatic degradation, endothelial cell clearance, and peripheral side effects while improving targeting and bioavailability and helping to overcome BBB barriers (Liu et al., 2023). Despite these potential advantages, there are still some challenging aspects of nanocarrier-mediated drug delivery, such as the toxicity of nanoparticles (Lamptey et al., 2022). The toxicity of nanoparticles depends mainly on size, surface charge, ion dissolution, and shape (Zielińska et al., 2020). First, choosing the optimal NP size for AD treatment is difficult. Studies have shown that nanoparticles with a particle size between 50 and 100 nm are more suitable for the treatment of AD, and the larger the size is, the lower the drug loading and BBB permeability. The smaller the size is, the greater the accumulation and toxicity (Liu and Shen, 2023; Sonavane et al., 2008). Rafael et al. demonstrated in the study of nanoparticle-targeted AD that smaller and negatively charged NPs can effectively cross the BBB and exhibit more neurosuppressive effects (Martín-Rapun et al., 2017). Jasjeet et al., in a review of nanoparticles on AD, concluded that the toxicity of naturally loaded nanoparticles is greatly reduced, this has only been reported in preclinical studies, and further verification of their toxicity in vivo is still needed (Sahni et al., 2011). In addition, the surface charge, ion dissolution, and shape of nanoparticles also affect their targeting and drug-loaded pharmacokinetics (Iqbal et al., 2024). Although improved nanoparticles can remedy best problems, their safety in vivo has not been clearly verified (Bi et al., 2024). Although nanoparticles have achieved good results in preclinical studies, there are some challenges to their clinical translation. There are still no clinical trials of nanoparticles for the treatment of AD.
5 Conclusion
AD is one of the most dangerous diseases that threatens the physical and mental health of older adults. As the world’s population ages, the number of older people is increasing. However, existing treatment strategies are far from satisfying the need for treatment by merely improving symptoms. The presence of the BBB prevents most drugs from penetrating the brain to exert their effects. The most important strategy for AD treatment is to develop effective drugs that can work in the brain through the BBB. Over the past few decades, therapeutic nanomedicine has also begun to gain momentum in the field of neuroscience. Owing to their natural advantages, nanoparticles have the potential to achieve targeted intracerebral drug delivery for the diagnosis, prevention, and treatment of neurological diseases. Nanomaterials can be improved in a controlled manner through internal and surface chemical modifications, with increased drug loading efficiency and targeted function, which can safely and effectively deliver loaded drugs to the lesion site. In addition, nanomaterials can provide new ideas for the treatment of AD through anti-aging, anti-neuroinflammatory, antioxidative, and nerve repair effects. However, the use of nanoparticles for clinical applications in the treatment of AD has rarely been reported. Medical protocols for the approval and validation of brain-targeted nanomedicines are still in their infancy. The clinical translation of nanoparticles faces some challenges, such as nanotoxicity, safe production and other factors. Although most nanoreports exist only in preclinical studies, we believe that more nanoparticle-based AD therapies are expected to be available in the near future.
Author contributions
JJ: Conceptualization, Writing – original draft, Writing – review and editing. SZ: Investigation, Project administration, Writing – original draft, Writing – review and editing. JZ: Data curation, Methodology, Writing – original draft, Writing – review and editing. YG: Conceptualization, Project administration, Writing – original draft, Writing – review and editing.
Funding
The author(s) declare that no financial support was received for the research and/or publication of this article.
Acknowledgments
We would like to express our appreciation to everyone who was involved in the drafting and preparation of the manuscript.
Conflict of interest
The authors declare that the research was conducted in the absence of any commercial or financial relationships that could be construed as a potential conflict of interest.
Generative AI statement
The author(s) declare that no Generative AI was used in the creation of this manuscript.
Publisher’s note
All claims expressed in this article are solely those of the authors and do not necessarily represent those of their affiliated organizations, or those of the publisher, the editors and the reviewers. Any product that may be evaluated in this article, or claim that may be made by its manufacturer, is not guaranteed or endorsed by the publisher.
References
Abd Elmonem, H. A., Morsi, R. M., Mansour, D. S., and El-Sayed, E. R. (2023). Myco-fabricated ZnO nanoparticles ameliorate neurotoxicity in mice model of Alzheimer’s disease via acetylcholinesterase inhibition and oxidative stress reduction. Biometals 36, 1391–1404. doi:10.1007/s10534-023-00525-6
Aili, M., Zhou, K., Zhan, J., Zheng, H., and Luo, F. (2023). Anti-inflammatory role of gold nanoparticles in the prevention and treatment of Alzheimer’s disease. J. Mater. Chem. B 11, 8605–8621. doi:10.1039/D3TB01023F
Alkhalifa, A. E., Al-Ghraiybah, N. F., Odum, J., Shunnarah, J. G., Austin, N., and Kaddoumi, A. (2023). Blood-brain barrier breakdown in Alzheimer’s disease: mechanisms and targeted strategies. Int. J. Mol. Sci. 24, 16288. doi:10.3390/ijms242216288
Aly, A. E. E., and Waszczak, B. L. (2015). Intranasal gene delivery for treating Parkinson’s disease: overcoming the blood–brain barrier. Expert Opin. Drug Deliv. 12, 1923–1941. doi:10.1517/17425247.2015.1069815
Bai, R., Guo, J., Ye, X.-Y., Xie, Y., and Xie, T. (2022). Oxidative stress: the core pathogenesis and mechanism of Alzheimer’s disease. Ageing Res. Rev. 77, 101619. doi:10.1016/j.arr.2022.101619
Bao, Q., Hu, P., Xu, Y., Cheng, T., Wei, C., Pan, L., et al. (2018). Simultaneous blood–brain barrier crossing and protection for stroke treatment based on edaravone-loaded ceria nanoparticles. ACS Nano 12, 6794–6805. doi:10.1021/acsnano.8b01994
Benilova, I., Karran, E., and De Strooper, B. (2012). The toxic Aβ oligomer and Alzheimer’s disease: an emperor in need of clothes. Nat. Neurosci. 15, 349–357. doi:10.1038/nn.3028
Bi, X., Cao, N., and He, J. (2024). Recent advances in nanoenzymes for Alzheimer’s disease treatment. Colloids Surfaces B Biointerfaces 244, 114139. doi:10.1016/j.colsurfb.2024.114139
Bolmont, T., Haiss, F., Eicke, D., Radde, R., Mathis, C. A., Klunk, W. E., et al. (2008). Dynamics of the microglial/amyloid interaction indicate a role in plaque maintenance. J. Neurosci. 28, 4283–4292. doi:10.1523/JNEUROSCI.4814-07.2008
Bose, S., and Cho, J. (2013). Role of chemokine CCL2 and its receptor CCR2 in neurodegenerative diseases. Arch. Pharm. Res. 36, 1039–1050. doi:10.1007/s12272-013-0161-z
Buccellato, F. R., D’Anca, M., Tartaglia, G. M., Del Fabbro, M., Scarpini, E., and Galimberti, D. (2023). Treatment of Alzheimer’s disease: beyond symptomatic therapies. Int. J. Mol. Sci. 24, 13900. doi:10.3390/ijms241813900
Butterfield, D. A., and Boyd-Kimball, D. (2018). Oxidative stress, amyloid-β peptide, and altered key molecular pathways in the pathogenesis and progression of Alzheimer’s disease. J. Alzheimer’s Dis. 62, 1345–1367. doi:10.3233/JAD-170543
Cai, H., Pang, Y., Fu, X., Ren, Z., and Jia, L. (2023). Plasma biomarkers predict Alzheimer’s disease before clinical onset in Chinese cohorts. Nat. Commun. 14, 6747. doi:10.1038/s41467-023-42596-6
Cai, Y., Zhou, H., Zhu, Y., Sun, Q., Ji, Y., Xue, A., et al. (2020). Elimination of senescent cells by β-galactosidase-targeted prodrug attenuates inflammation and restores physical function in aged mice. Cell Res. 30, 574–589. doi:10.1038/s41422-020-0314-9
Cao, Z., Kong, F., Ding, J., Chen, C., He, F., and Deng, W. (2024). Promoting Alzheimer’s disease research and therapy with stem cell technology. Stem Cell Res. and Ther. 15, 136. doi:10.1186/s13287-024-03737-w
Chen, H. Y., Deng, J., Wang, Y., Wu, C. Q., Li, X., and Dai, H. W. (2020b). Hybrid cell membrane-coated nanoparticles: a multifunctional biomimetic platform for cancer diagnosis and therapy. Acta Biomater. 112, 1–13. doi:10.1016/j.actbio.2020.05.028
Chen, Y., Li, P., Modica, J. A., Drout, R. J., and Farha, O. K. (2018). Acid-resistant mesoporous metal–organic framework toward oral insulin delivery: protein encapsulation, protection, and release. J. Am. Chem. Soc. 140, 5678–5681. doi:10.1021/jacs.8b02089
Chen, Y. X., Wei, C. X., Lyu, Y. Q., Chen, H. Z., Jiang, G., and Gao, X. L. (2020a). Biomimetic drug-delivery systems for the management of brain diseases. Biomater. Sci. 8, 1073–1088. doi:10.1039/c9bm01395d
Chitnis, T., and Weiner, H. L. (2017). CNS inflammation and neurodegeneration. J. Clin. Invest. 127, 3577–3587. doi:10.1172/jci90609
Cummings, J. L., Osse, A. M. L., and Kinney, J. W. (2023). Alzheimer’s disease: novel targets and investigational drugs for disease modification. Drugs 83, 1387–1408. doi:10.1007/s40265-023-01938-w
De Strooper, B., and Karran, E. (2016). The cellular phase of Alzheimer’s disease. Cell 164, 603–615. doi:10.1016/j.cell.2015.12.056
Doerks, T., Copley, R. R., Schultz, J., Ponting, C. P., and Bork, P. (2002). Systematic identification of novel protein domain families associated with nuclear functions. Genome Res. 12, 47–56. doi:10.1101/gr.203201
Ege, D. (2021). Action mechanisms of curcumin in Alzheimer’s disease and its brain targeted delivery. Mater. (Basel) 14, 3332. doi:10.3390/ma14123332
El Andaloussi, S., Lakhal, S., Mäger, I., and Wood, M. J. (2013). Exosomes for targeted siRNA delivery across biological barriers. Adv. Drug Deliv. Rev. 65, 391–397. doi:10.1016/j.addr.2012.08.008
Elmahboub, Y. S. M., and Elkordy, A. A. (2024). Polymeric nanoparticles: a promising strategy for treatment of Alzheimer’s disease. J. Taibah Univ. Med. Sci. 19, 549–565. doi:10.1016/j.jtumed.2024.04.004
El-Sayed, E. R., Abdelhakim, H. K., and Zakaria, Z. (2020). Extracellular biosynthesis of cobalt ferrite nanoparticles by Monascus purpureus and their antioxidant, anticancer and antimicrobial activities: yield enhancement by gamma irradiation. Mater Sci. Eng. C Mater Biol. Appl. 107, 110318. doi:10.1016/j.msec.2019.110318
El-Sayed, E.-S. R., Mohamed, S. S., Mousa, S. A., El-Seoud, M. A. A., Elmehlawy, A. A., and Abdou, D. A. M. (2023). Bifunctional role of some biogenic nanoparticles in controlling wilt disease and promoting growth of common bean. Amb. Express 13, 41. doi:10.1186/s13568-023-01546-7
Fu, R., Shen, Q., Xu, P., Luo, J. J., and Tang, Y. (2014). Phagocytosis of microglia in the central nervous system diseases. Mol. Neurobiol. 49, 1422–1434. doi:10.1007/s12035-013-8620-6
Gao, C., Liu, Y., Zhang, T. L., Luo, Y., Gao, J., Chu, J. J., et al. (2023). Biomembrane-derived nanoparticles in Alzheimer’s disease therapy: a comprehensive review of synthetic lipid nanoparticles and natural cell-derived vesicles. Int. J. Nanomedicine 18, 7441–7468. doi:10.2147/ijn.S436774
Gasek, N. S., Kuchel, G. A., Kirkland, J. L., and Xu, M. (2021). Strategies for targeting senescent cells in human disease. Nat. Aging 1, 870–879. doi:10.1038/s43587-021-00121-8
Ghosh, A., Comerota, M. M., Wan, D., Chen, F., Propson, N. E., Hwang, S. H., et al. (2020). An epoxide hydrolase inhibitor reduces neuroinflammation in a mouse model of Alzheimer's disease. Sci. Transl. Med. 12, eabb1206. doi:10.1126/scitranslmed.abb1206
Govindpani, K., McNamara, L. G., Smith, N. R., Vinnakota, C., Waldvogel, H. J., Faull, R. L., et al. (2019). Vascular dysfunction in Alzheimer’s disease: a prelude to the pathological process or a consequence of it? J. Clin. Med. 8, 651. doi:10.3390/jcm8050651
Graff-Radford, J., Yong, K. X. X., Apostolova, L. G., Bouwman, F. H., Carrillo, M., Dickerson, B. C., et al. (2021). New insights into atypical Alzheimer’s disease in the era of biomarkers. Lancet Neurology 20, 222–234. doi:10.1016/S1474-4422(20)30440-3
Grubman, A., Choo, X. Y., Chew, G., Ouyang, J. F., Sun, G., Croft, N. P., et al. (2021). Transcriptional signature in microglia associated with Aβ plaque phagocytosis. Nat. Commun. 12, 3015. doi:10.1038/s41467-021-23111-1
Hu, Y., Guo, H., Cheng, S., Sun, J., Du, J., Liu, X., et al. (2023). Functionalized cerium dioxide nanoparticles with antioxidative neuroprotection for Alzheimer’s disease. Int. J. Nanomedicine 18, 6797–6812. doi:10.2147/ijn.S434873
Huang, D., Cao, Y., Yang, X., Liu, Y., Zhang, Y., Li, C., et al. (2021). A nanoformulation-mediated multifunctional stem cell therapy with improved beta-amyloid clearance and neural regeneration for Alzheimer's disease. Adv. Mater 33, e2006357. doi:10.1002/adma.202006357
Huang, Y. Y., Gan, Y. H., Yang, L., Cheng, W., and Yu, J. T. (2024). Depression in Alzheimer’s disease: epidemiology, mechanisms, and treatment. Biol. Psychiatry 95, 992–1005. doi:10.1016/j.biopsych.2023.10.008
Hussein, H. G., El-Sayed, E.-S. R., Younis, N. A., Hamdy, A. E. H. A., and Easa, S. M. (2022). Harnessing endophytic fungi for biosynthesis of selenium nanoparticles and exploring their bioactivities. Amb. Express 12, 68. doi:10.1186/s13568-022-01408-8
Ionescu-Tucker, A., and Cotman, C. W. (2021). Emerging roles of oxidative stress in brain aging and Alzheimer’s disease. Neurobiol. Aging 107, 86–95. doi:10.1016/j.neurobiolaging.2021.07.014
Iqbal, I., Saqib, F., Mubarak, Z., Latif, M. F., Wahid, M., Nasir, B., et al. (2024). Alzheimer’s disease and drug delivery across the blood-brain barrier: approaches and challenges. Eur. J. Med. Res. 29, 313. doi:10.1186/s40001-024-01915-3
Iwata, N., Tsubuki, S., Takaki, Y., Watanabe, K., Sekiguchi, M., Hosoki, E., et al. (2000). Identification of the major Abeta1-42-degrading catabolic pathway in brain parenchyma: suppression leads to biochemical and pathological deposition. Nat. Med. 6, 143–150. doi:10.1038/72237
Ji, W., Zhou, H., Liang, W., Zhang, W., Gong, B., Yin, T., et al. (2024). SSK1-Loaded neurotransmitter-derived nanoparticles for Alzheimer’s disease therapy via clearance of senescent cells. Small 20, 2308574. doi:10.1002/smll.202308574
Jung, M., Lee, S., Park, S., Hong, J., Kim, C., Cho, I., et al. (2023). A therapeutic nanovaccine that generates anti-amyloid antibodies and amyloid-specific regulatory T cells for Alzheimer’s disease. Adv. Mater. 35, 2207719. doi:10.1002/adma.202207719
Kang, T. H., Park, J. H., Shin, D., Choi, H., Kim, J., and Lee, M. C. (2019). Brown adipocyte and splenocyte Co-culture maintains regulatory T cell subset in intermittent hypobaric conditions. Tissue Eng. Regen. Med. 16, 539–548. doi:10.1007/s13770-019-00205-y
Khan, H., Ullah, H., Aschner, M., Cheang, W. S., and Akkol, E. K. (2020). Neuroprotective effects of quercetin in Alzheimer’s disease. Biomolecules 10, 59. doi:10.3390/biom10010059
Khan, M. B., Khan, M. M., Khan, A., Ahmed, M. E., Ishrat, T., Tabassum, R., et al. (2012). Naringenin ameliorates Alzheimer’s disease (AD)-type neurodegeneration with cognitive impairment (AD-TNDCI) caused by the intracerebroventricular-streptozotocin in rat model. Neurochem. Int. 61, 1081–1093. doi:10.1016/j.neuint.2012.07.025
Kuo, Y. C., Lin, C. Y., Li, J. S., and Lou, Y. I. (2017). Wheat germ agglutinin-conjugated liposomes incorporated with cardiolipin to improve neuronal survival in Alzheimer’s disease treatment. Int. J. Nanomedicine 12, 1757–1774. doi:10.2147/ijn.S128396
Lamptey, R. N. L., Chaulagain, B., Trivedi, R., Gothwal, A., Layek, B., and Singh, J. (2022). A review of the common neurodegenerative disorders: current therapeutic approaches and the potential role of nanotherapeutics. Int. J. Mol. Sci. 23, 1851. doi:10.3390/ijms23031851
Lane, C. A., Hardy, J., and Schott, J. M. (2018). Alzheimer’s disease. Eur. J. Neurology 25, 59–70. doi:10.1111/ene.13439
Lei, T., Yang, Z., Li, H., Qin, M., and Gao, H. (2024). Interactions between nanoparticles and pathological changes of vascular in Alzheimer’s disease. Adv. Drug Deliv. Rev. 207, 115219. doi:10.1016/j.addr.2024.115219
Li, J., Peng, H., Zhang, W., Li, M., Wang, N., Peng, C., et al. (2023). Enhanced nose-to-brain delivery of combined small interfering RNAs using lesion-recognizing nanoparticles for the synergistic therapy of alzheimer’s disease. ACS Appl. Mater. and Interfaces 15, 53177–53188. doi:10.1021/acsami.3c08756
Li, L., Zhang, J., Zhang, Y., Zhao, R., Yang, F., Yan, Y., et al. (2025). Biofilm-modified Prussian blue improves memory function in late-stage Alzheimer’s disease mice with triple therapy. Int. J. Pharm. 670, 125112. doi:10.1016/j.ijpharm.2024.125112
Lin, R. R., Jin, L. L., Xue, Y. Y., Zhang, Z. S., Huang, H. F., Chen, D. F., et al. (2024). Hybrid membrane-coated nanoparticles for precise targeting and synergistic therapy in Alzheimer’s disease. Adv. Sci. (Weinheim, Baden-Wurttemberg, Ger.) 11, e2306675. doi:10.1002/advs.202306675
Liu, N., Liang, X., Chen, Y., and Xie, L. (2024b). Recent trends in treatment strategies for Alzheimer’s disease and the challenges: a topical advancement. Ageing Res. Rev. 94, 102199. doi:10.1016/j.arr.2024.102199
Liu, N., Ruan, J., Li, H., and Fu, J. (2023). Nanoparticles loaded with natural medicines for the treatment of Alzheimer’s disease. Front. Neurosci. 17, 1112435. doi:10.3389/fnins.2023.1112435
Liu, W., Dong, X., Liu, Y., and Sun, Y. (2021). Photoresponsive materials for intensified modulation of Alzheimer’s amyloid-β protein aggregation: a review. Acta Biomater. 123, 93–109. doi:10.1016/j.actbio.2021.01.018
Liu, Y., and Shen, Y. (2023). Applications of nanoparticles in Alzheimer’s disease. J. Alzheimers Dis. 96, 459–471. doi:10.3233/jad-230098
Liu, Y., Tan, Y., Zhang, Z., Yi, M., Zhu, L., and Peng, W. (2024a). The interaction between ageing and Alzheimer’s disease: insights from the hallmarks of ageing. Transl. Neurodegener. 13, 7. doi:10.1186/s40035-024-00397-x
Lopez-Lee, C., Torres, E. R. S., Carling, G., and Gan, L. (2024). Mechanisms of sex differences in Alzheimer’s disease. Neuron 112, 1208–1221. doi:10.1016/j.neuron.2024.01.024
Majumder, S., Richardson, A., Strong, R., and Oddo, S. (2011). Inducing autophagy by rapamycin before, but not after, the formation of plaques and tangles ameliorates cognitive deficits. PLoS One 6, e25416. doi:10.1371/journal.pone.0025416
Martín-Rapun, R., De Matteis, L., Ambrosone, A., Garcia-Embid, S., Gutierrez, L., and de la Fuente, J. M. (2017). Targeted nanoparticles for the treatment of Alzheimer’s disease. Curr. Pharm. Des. 23, 1927–1952. doi:10.2174/1381612822666161226151011
Mashal, Y., Abdelhady, H., and Iyer, A. K. (2022). Comparison of tau and amyloid-β targeted immunotherapy nanoparticles for Alzheimer’s disease. Biomolecules 12, 1001. doi:10.3390/biom12071001
Mathew, A., Fukuda, T., Nagaoka, Y., Hasumura, T., Morimoto, H., Yoshida, Y., et al. (2012). Curcumin loaded-PLGA nanoparticles conjugated with Tet-1 peptide for potential use in Alzheimer’s disease. PLoS One 7, e32616. doi:10.1371/journal.pone.0032616
McManus, R. M., and Latz, E. (2024). NLRP3 inflammasome signalling in Alzheimer’s disease. Neuropharmacology 252, 109941. doi:10.1016/j.neuropharm.2024.109941
Md, S., Gan, S. Y., Haw, Y. H., Ho, C. L., Wong, S., and Choudhury, H. (2018). In vitro neuroprotective effects of naringenin nanoemulsion against β-amyloid toxicity through the regulation of amyloidogenesis and tau phosphorylation. Int. J. Biol. Macromol. 118, 1211–1219. doi:10.1016/j.ijbiomac.2018.06.190
Miller, V. M., Lawrence, D. A., Mondal, T. K., and Seegal, R. F. (2009). Reduced glutathione is highly expressed in white matter and neurons in the unperturbed mouse brain--implications for oxidative stress associated with neurodegeneration. Brain Res. 1276, 22–30. doi:10.1016/j.brainres.2009.04.029
Motallebi, M., Bhia, M., Rajani, H. F., Bhia, I., Tabarraei, H., Mohammadkhani, N., et al. (2022). Naringenin: a potential flavonoid phytochemical for cancer therapy. Life Sci. 305, 120752. doi:10.1016/j.lfs.2022.120752
Moussa, C., Hebron, M., Huang, X., Ahn, J., Rissman, R. A., Aisen, P. S., et al. (2017). Resveratrol regulates neuro-inflammation and induces adaptive immunity in Alzheimer’s disease. J. neuroinflammation 14, 1. doi:10.1186/s12974-016-0779-0
Muhs, A., Hickman, D. T., Pihlgren, M., Chuard, N., Giriens, V., Meerschman, C., et al. (2007). Liposomal vaccines with conformation-specific amyloid peptide antigens define immune response and efficacy in APP transgenic mice. Proc. Natl. Acad. Sci. U. S. A. 104, 9810–9815. doi:10.1073/pnas.0703137104
Palmqvist, S., Janelidze, S., Stomrud, E., Zetterberg, H., Karl, J., Zink, K., et al. (2019). Performance of fully automated plasma assays as screening tests for alzheimer disease–related β-amyloid status. JAMA Neurol. 76, 1060–1069. doi:10.1001/jamaneurol.2019.1632
Passeri, E., Elkhoury, K., Morsink, M., Broersen, K., Linder, M., Tamayol, A., et al. (2022). Alzheimer’s disease: treatment strategies and their limitations. Int. J. Mol. Sci. 23, 13954. doi:10.3390/ijms232213954
Pena, S. A., Iyengar, R., Eshraghi, R. S., Bencie, N., Mittal, J., Aljohani, A., et al. (2020). Gene therapy for neurological disorders: challenges and recent advancements. J. Drug Target 28, 111–128. doi:10.1080/1061186x.2019.1630415
Penney, J., Ralvenius, W. T., and Tsai, L. H. (2020). Modeling Alzheimer’s disease with iPSC-derived brain cells. Mol. Psychiatry 25, 148–167. doi:10.1038/s41380-019-0468-3
Perneczky, R., Dom, G., Chan, A., Falkai, P., and Bassetti, C. (2024). Anti-amyloid antibody treatments for Alzheimer’s disease. Eur. J. Neurol. 31, e16049. doi:10.1111/ene.16049
Pimenova, A. A., Herbinet, M., Gupta, I., Machlovi, S. I., Bowles, K. R., Marcora, E., et al. (2021). Alzheimer’s-associated PU.1 expression levels regulate microglial inflammatory response. Neurobiol. Dis. 148, 105217. doi:10.1016/j.nbd.2020.105217
Prajapati, S. K., Pathak, A., and Samaiya, P. K. (2024). Alzheimer’s disease: from early pathogenesis to novel therapeutic approaches. Metab. Brain Dis. 39, 1231–1254. doi:10.1007/s11011-024-01389-6
Rajendran, K., and Krishnan, U. M. (2024). Mechanistic insights and emerging therapeutic stratagems for Alzheimer’s disease. Ageing Res. Rev. 97, 102309. doi:10.1016/j.arr.2024.102309
Rajmohan, R., and Reddy, P. H. (2017). Amyloid-beta and phosphorylated tau accumulations cause abnormalities at synapses of Alzheimer’s disease neurons. J. Alzheimer's Dis. 57, 975–999. doi:10.3233/JAD-160612
Ralvenius, W. T., Andresen, J. L., Huston, M. M., Penney, J., Bonner, J. M., Fenton, O. S., et al. (2024). Nanoparticle-mediated delivery of anti-PU.1 siRNA via localized intracisternal administration reduces neuroinflammation. Adv. Mater. 36, 2309225. doi:10.1002/adma.202309225
Ratnam, D. V., Ankola, D. D., Bhardwaj, V., Sahana, D. K., and Kumar, M. N. V. R. (2006). Role of antioxidants in prophylaxis and therapy: a pharmaceutical perspective. J. Control. Release 113, 189–207. doi:10.1016/j.jconrel.2006.04.015
Sahni, J. K., Doggui, S., Ali, J., Baboota, S., Dao, L., and Ramassamy, C. (2011). Neurotherapeutic applications of nanoparticles in Alzheimer’s disease. J. Control. Release 152, 208–231. doi:10.1016/j.jconrel.2010.11.033
Scheltens, P., De Strooper, B., Kivipelto, M., Holstege, H., Chételat, G., Teunissen, C. E., et al. (2021). Alzheimer’s disease. Lancet 397, 1577–1590. doi:10.1016/s0140-6736(20)32205-4
Sen, A., Nelson, T. J., Alkon, D. L., and Hongpaisan, J. (2018). Loss in PKC epsilon causes downregulation of MnSOD and BDNF expression in neurons of Alzheimer’s disease Hippocampus. J. Alzheimer’s Dis. 63, 1173–1189. doi:10.3233/JAD-171008
Shin, H. J., Kim, I. S., Choi, S. G., Lee, K., Park, H., Shin, J., et al. (2024). Rejuvenating aged microglia by p16(ink4a)-siRNA-loaded nanoparticles increases amyloid-β clearance in animal models of Alzheimer’s disease. Mol. Neurodegener. 19, 25. doi:10.1186/s13024-024-00715-x
Shin, J., Yin, Y., Park, H., Park, S., Triantafillu, U. L., Kim, Y., et al. (2018). p38 siRNA-encapsulated PLGA nanoparticles alleviate neuropathic pain behavior in rats by inhibiting microglia activation. Nanomedicine (Lond) 13, 1607–1621. doi:10.2217/nnm-2018-0054
Singh, D. (2022). Astrocytic and microglial cells as the modulators of neuroinflammation in Alzheimer’s disease. J. Neuroinflammation 19, 206. doi:10.1186/s12974-022-02565-0
Sonavane, G., Tomoda, K., and Makino, K. (2008). Biodistribution of colloidal gold nanoparticles after intravenous administration: effect of particle size. Colloids Surf. B Biointerfaces 66, 274–280. doi:10.1016/j.colsurfb.2008.07.004
Sun, D., Li, N., Zhang, W., Zhao, Z., Mou, Z., Huang, D., et al. (2016). Design of PLGA-functionalized quercetin nanoparticles for potential use in Alzheimer’s disease. Colloids Surfaces B Biointerfaces 148, 116–129. doi:10.1016/j.colsurfb.2016.08.052
Thakur, S., Dhapola, R., Sarma, P., Medhi, B., and Reddy, D. H. (2023). Neuroinflammation in Alzheimer’s disease: current progress in molecular signaling and therapeutics. Inflammation 46, 1–17. doi:10.1007/s10753-022-01721-1
Tosi, G., Musumeci, T., Ruozi, B., Carbone, C., Belletti, D., Pignatello, R., et al. (2016). The “fate” of polymeric and lipid nanoparticles for brain delivery and targeting: strategies and mechanism of blood–brain barrier crossing and trafficking into the central nervous system. J. Drug Deliv. Sci. Technol. 32, 66–76. doi:10.1016/j.jddst.2015.07.007
Wang, H., Xia, Y., Li, B., Li, Y., and Fu, C. (2022). Reverse adverse immune microenvironments by biomaterials enhance the repair of spinal cord injury. Front. Bioeng. Biotechnol. 10, 812340. doi:10.3389/fbioe.2022.812340
Xi, Y., Chen, Y., Jin, Y., Han, G., Song, M., Song, T., et al. (2022). Versatile nanomaterials for Alzheimer’s disease: pathogenesis inspired disease-modifying therapy. J. Control. Release 345, 38–61. doi:10.1016/j.jconrel.2022.02.034
Xia, Y., Wang, H., Yang, R., Hou, Y., Li, Y., Zhu, J., et al. (2023a). Biomaterials delivery strategies to repair degenerated intervertebral discs by regulating the inflammatory microenvironment. Front. Immunol. 14, 1051606. doi:10.3389/fimmu.2023.1051606
Xia, Y., Yang, R., Wang, H., Hou, Y., Li, Y., Zhu, J., et al. (2022a). Biomaterials delivery strategies to repair spinal cord injury by modulating macrophage phenotypes. J. Tissue Eng. 13, 20417314221143059. doi:10.1177/20417314221143059
Xia, Y., Zhu, J., Yang, R., Wang, H., Li, Y., and Fu, C. (2023b). Mesenchymal stem cells in the treatment of spinal cord injury: mechanisms, current advances and future challenges. Front. Immunol. 14, 1141601. doi:10.3389/fimmu.2023.1141601
Xia, Y. L., Yang, R. H., Wang, H. Y., Li, Y. H., and Fu, C. F. (2022b). Application of chitosan-based materials in surgical or postoperative hemostasis. Front. Mater. 9. doi:10.3389/fmats.2022.994265
Yu, D., Ma, M., Liu, Z., Pi, Z., Du, X., Ren, J., et al. (2020). MOF-encapsulated nanozyme enhanced siRNA combo: control neural stem cell differentiation and ameliorate cognitive impairments in Alzheimer’s disease model. Biomaterials 255, 120160. doi:10.1016/j.biomaterials.2020.120160
Yuan, X., Jia, Z., Li, J., Liu, Y., Huang, Y., Gong, Y., et al. (2021). A diselenide bond-containing ROS-responsive ruthenium nanoplatform delivers nerve growth factor for Alzheimer’s disease management by repairing and promoting neuron regeneration. J. Mater Chem. B 9, 7835–7847. doi:10.1039/d1tb01290h
Zapata-Acevedo, J. F., Mantilla-Galindo, A., Vargas-Sánchez, K., and González-Reyes, R. E. (2024). Blood-brain barrier biomarkers. Adv. Clin. Chem. 121, 1–88. doi:10.1016/bs.acc.2024.04.004
Zhang, C. (2023). Etiology of Alzheimer’s disease. Discov. Med. 35, 757–776. doi:10.24976/Discov.Med.202335178.71
Zhang, W., Hu, S., Yin, J.-J., He, W., Lu, W., Ma, M., et al. (2016). Prussian blue nanoparticles as multienzyme mimetics and reactive oxygen species scavengers. J. Am. Chem. Soc. 138, 5860–5865. doi:10.1021/jacs.5b12070
Zhang, W., Mehta, A., Tong, Z., Esser, L., and Voelcker, N. H. (2021). Development of polymeric nanoparticles for blood–brain barrier transfer—strategies and challenges. Adv. Sci. 8, 2003937. doi:10.1002/advs.202003937
Zhang, X. W., Zhu, X. X., Tang, D. S., and Lu, J. H. (2023). Targeting autophagy in Alzheimer’s disease: animal models and mechanisms. Zool. Res. 44, 1132–1145. doi:10.24272/j.issn.2095-8137.2023.294
Zhong, G., Long, H., Zhou, T., Liu, Y., Zhao, J., Han, J., et al. (2022). Blood-brain barrier Permeable nanoparticles for Alzheimer’s disease treatment by selective mitophagy of microglia. Biomaterials 288, 121690. doi:10.1016/j.biomaterials.2022.121690
Zielińska, A., Costa, B., Ferreira, M. V., Miguéis, D., Louros, J. M. S., Durazzo, A., et al. (2020). Nanotoxicology and nanosafety: safety-by-design and testing at a glance. Int. J. Environ. Res. Public Health 17, 4657. doi:10.3390/ijerph17134657
Zlokovic, B. V. (2008). The blood-brain barrier in health and chronic neurodegenerative disorders. Neuron 57, 178–201. doi:10.1016/j.neuron.2008.01.003
Keywords: nanoparticles, Alzheimer’s disease, blood-brain barrier, nerve regeneration, β amyloid
Citation: Jia J, Zhao S, Zhao J and Gao Y (2025) Engineered nanoparticles for the treatment of Alzheimer’s disease. Front. Pharmacol. 16:1510798. doi: 10.3389/fphar.2025.1510798
Received: 13 October 2024; Accepted: 21 March 2025;
Published: 03 April 2025.
Edited by:
Panoraia Siafaka, European University Cyprus, CyprusReviewed by:
Tapan A. Patel, University of Nebraska Medical Center, United StatesAdeline Yoke Yin Chia, Taylor’s University, Malaysia
Anil Kumar Mavi, University of Delhi, India
Copyright © 2025 Jia, Zhao, Zhao and Gao. This is an open-access article distributed under the terms of the Creative Commons Attribution License (CC BY). The use, distribution or reproduction in other forums is permitted, provided the original author(s) and the copyright owner(s) are credited and that the original publication in this journal is cited, in accordance with accepted academic practice. No use, distribution or reproduction is permitted which does not comply with these terms.
*Correspondence: Yun Gao, Nzc3MDQ2NDVAY211LmVkdS5jbg==
†These authors have contributed equally to this work