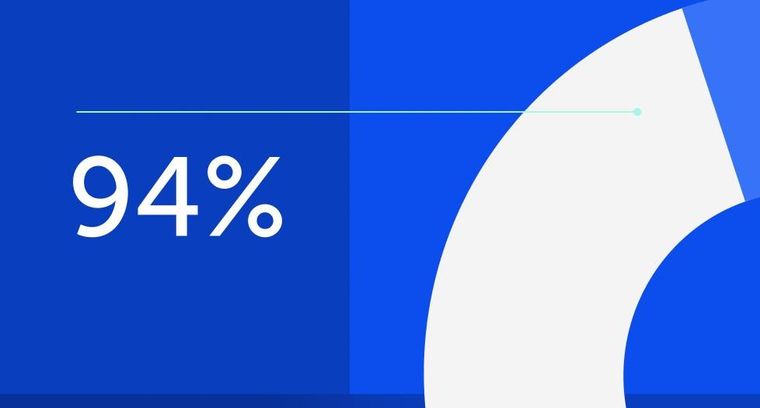
94% of researchers rate our articles as excellent or good
Learn more about the work of our research integrity team to safeguard the quality of each article we publish.
Find out more
REVIEW article
Front. Pharmacol., 30 January 2025
Sec. Pharmacology of Ion Channels and Channelopathies
Volume 15 - 2024 | https://doi.org/10.3389/fphar.2024.1536864
This article is part of the Research TopicEpithelial Transport: from fluid movement toward system organizationView all 3 articles
SLC26A6, a member of the SLC26 family of multifunctional anion transporters, has been particularly enigmatic because of its multiple modes of transport, its expression in organs that are difficult to study physiologically, and the lack of specific antibodies and inhibitors. This has recently changed. SLC26A6 is expressed in the human pancreas, kidney, intestine, heart and some other organs and is involved in fluid absorption, anion secretion, regulation of intracellular pH and elimination of waste products such as oxalate. This review will focus on three topics: Firstly, a molecular structure of human SLC26A6 has recently been obtained by cryo-electron microscopy. Structure-function studies of the reconstituted SLC26A6 in proteoliposomes suggested a 1:1 stoichiometry, resulting in electroneutral Cl−/HCO3− exchange and electrogenic Cl−/oxalate2− exchange. How do these data help to understand the published functional studies? Secondly, whole exon sequencing of a kidney stone cohort from the United Kingdom database revealed a dominant negative SLC26A6 mutation in a patient with enteric hyperoxaluria, oxalate kidney stones and a low calcium diet. How does this finding fit with previous genetic studies in mice and humans of SLC26A6 gene mutations? Thirdly, progress has been made in identifying specific inhibitors for SLC26A6. Where might this be of clinical relevance?
Solute carrier family 26 (SLC26) is a family of functionally diverse anion transporters found in all kingdoms of life. The human genome encodes 10 functional homologs, several of which are causally associated with major human diseases. The SLC26A6 gene from different species has been cloned in parallel by several researchers and has also been named CFEX and PAT-1 (Lohi et al., 2000; Knauf et al., 2001; Waldegger et al., 2001). SLC26A6 appears to be able to transport a wide range of mono- and divalent anions, including formate, sulphate, oxalate, nitrate, Cl− and HCO3−. SLC26A6 mRNA and protein expression was first reported in the pancreas (Lohi et al., 2000), the kidney (Knauf et al., 2001; Waldegger et al., 2001) and the intestine (Wang et al., 2002). The mRNA is found in many other organs, while the immunohistochemical data are less clear due to nonspecific binding of many anti-SLC26a6 antibodies (Seidler and Nikolovska, 2019; Wang et al., 2020). Slc26a6-deleted mice showed alterations in epithelial transport functions in the pancreatic ducts (Song et al., 2012), in the proximal intestine (Wang et al., 2005; Tuo et al., 2006; Singh et al., 2008a; Singh et al., 2008b; Singh et al., 2010; Knauf et al., 2011; Xia et al., 2014; Neumeier et al., 2020), and the salivary glands (Shcheynikov et al., 2008), without morphological abnormalities, as well as the development of kidney stone on a high oxalate diet (Jiang et al., 2006), and deficits in pHi-regulation in the heart (Sirish et al., 2017).
Early transport studies in Xenopus oocytes and in HEK293 cells by different groups suggested different stoichiometries, and therefore electroneutral vs. electrogenic Cl−/HCO3− exchange (Ko et al., 2002; Chernova et al., 2005; Shcheynikov et al., 2006). Because the aminoacid sequence similarity is unusally low between the human and mouse orthologs of SLC26A6 with 78% identity, functional studies have compared their transport characteristics when expressed in Xenopus oocytes: While human SLC26A6-mediated electroneutral (2?)Cl−/oxalate exchange2−, the exchange by murine Slc26a6 appeared electrogenic (Jiang et al., 2002; Chernova et al., 2005; Clark et al., 2008). The native tissue transport studies could not resolve these controversies, because Slc26a6 is coexpressed with, and its transport activity influenced by, many other transporters, which may influence the electrogenicity or–neutrality of a studied transport process. Since comprehensive reviews discuss these early experiments in detail (Ohana et al., 2009; Aronson, 2010; Ishiguro et al., 2012; Alper and Sharma, 2013; Ohana, 2015; Yamaguchi et al., 2017; Seidler and Nikolovska, 2019; Wang et al., 2020), I will focus on the recently published structure-function studies in reconstituted liposomes, on human disease causing mutations, and recent pharmacological developments.
The first member of the SLC26 family whose crystal structure was identified, was SLC26Dg, a prokaryotic proton-coupled fumarate transporter (Geertsma et al., 2015). SLC26Dg shares 46% residue similarity in the transmembrane (TM) region with human Prestin and 57% with the Escherichia coli transporter DauA. When reconstituted in lipid bilayers, the purified SLC26Dg forms a homodimer, which had already been predicted from previous studies for the whole family (Detro-Dassen et al., 2008; Compton et al., 2014). Using a specific method for protein expression and an anti-SLC26Dg nanobody to facilitate crystallisation (Chang and Geertsma, 2017), the group was able to crystallize SLC26Dg monomers to a resolution of 3.2 Å (Geertsma et al., 2015). The membrane-inserted domain consisted of two intertwined inverted repeats of seven transmembrane segments each (Figure 1A). The transmembrane part of SLC26Dg is organised into two parallel subdomains, with the helices 1–4 and 8–11 folding into a compact unit designated as the “transport” or “core” domain, and the Helices 5–7 and 12–14 forming an elongated structure called the “scaffold” or “gate” domain, which shields one side of the transport domain (Figure 1B). The anion binding site was proposed to be located in the center of the transport domain, close to the scaffold domain, and transport to occur by an “elevator-like” mechanism, in which the mobile transport domain moves against the rigid scaffold domain (Figure 1C). One of the best supporting experimental evidence for this “elevater mechanism” was provided by the groups of Jan-Philipp Machtens and Dominik Oliver during their studies on the transport mode of prestin (SLC26A5) (Kuwabara et al., 2023).
Figure 1. Structure and transport mechanism common for the SLC26A family. (A) Topology diagram of SLC26 proteins. TM3 and TM10, which form the substrate binding site, are colored blue and pink, respectively. (B) Membrane domain of a schematic SLC26A protomer viewed from the outside on the membrane. Transport and scaffold domains are shown in dark and light gray, respectively. (C) Side view in the plane of the membrane at the interface of the transport and scaffold domains, showing the opening of the SLC26A isoform to the extracellular space and the cytoplasm, respectively. The expected deformation of the lipid membrane as a result of the elevator movement of the transport domain is indicated. Asterisk indicates the transported anion. (D) Side view in the plane of the membrane on the human SLC26A9 dimer. One protomer is shown in a rainbow color scheme with the N- and C-terminus in blue and red, respectively. The opposite protomer is shown in the color scheme of panel (B). Dotted lines indicate intervening sequences (not structurally resolved) in the STAS domain.
This mode of transport was assumed to be representative for the whole SLC26 family, and this has so far been proven correct, with structure information having been published for several more of the mammalian, as well as prokaryotic members of the family (Wang et al., 2019; Chi et al., 2020; Bavi et al., 2021; Wang et al., 2021; Ge et al., 2021; Butan et al., 2022; Futamata et al., 2022; Li et al., 2023; Liu et al., 2023; Tippett et al., 2023; Wang et al., 2024). The first mammalian SLC26 member, for which a high resolution structure was published, was the murine Slc26a9 (Walter et al., 2019), shortly later followed by publication of the human homolog (Chi et al., 2020). This revealed the structure of the complete dimeric SLC26 assembly, showing that each membrane domain interacts with the cytoplasmatic Sulfate Transporter and Anti-Sigma factor antagonist (STAS) domain of the opposite protomer (Figure 1D).
The same group that analysed the structure and transport properties of mouse Slc26a9 (Walter et al., 2019) has recently published the functional and structural analysis of the human SLC26A6 isoform (Tippett et al., 2023). These very informative experiments will now be reviewed in detail: The group first expressed human SLC26A6 and murine Slc26a9 in HEK293 cells and performed whole-cell patch-clamp experiments, assuming that the reported electrogenic SLC26A6 (Shcheynikov et al., 2006; Ohana et al., 2009) could be studied by establishing a current-voltage relationship similar to that observed for Slc26a9. However, in contrast to Slc26a9, no specific current was measured at any voltage (Figure 2A), despite the fact that the membrane expression of SLC26A6 and Slc26a9 was similar. The group then expressed, purified and reconstituted the protein in proteoliposomes. The transport function was assessed using different fluorophores, always in comparison with that of Slc26a9t (a Slc26a9 with truncated IVS sequences for better membrane insertion). Using the pH-sensitive dye ACMA and the protonophore CCCP, the researchers were able to identify electrogenic anion influx. Electrogenic anion influx leads to the build-up of a negative potential. In the presence of the protonophore CCCP, this results in proton influx, reducing the pH inside the liposomes and quenching the ACMA fluorescence. In contrast to Slc26a9t-reconstituted proteoliposomes, external Cl− did not induce ACMA fluorescence quenching in SLC26A6-reconstituted proteoliposomes (Figure 2B). However, external oxalate (Ox2−) resulted in ACMA quenching in Cl− loaded SLC26A6-reconstituted proteoliposomes, suggesting that SLC26A6-mediated Ox2−/Cl− exchange is electrogenic (Figure 2C).
Figure 2. Transport properties of SLC26A6. (A) Current-Voltage relationships of HEK 293 cells expressing murine SLC26A9 (left graph) and human SLC26A6 (right graph). (B) Uncoupled Cl− transport mediated by either the modified murine construct SLC26A9T or SLC26A6 reconstituted into proteoliposomes, as monitored by the fluorescence change of the pH-sensitive fluorophore ACMA. Asterisk indicates addition of the protonophore CCCP, which allows counterion movement and electrogenic Cl− transport to proceed. (C) Electrogenic oxalate uptake followed by the fluorescence change of the pH-sensitive fluorophore ACMA. Traces show mean quenching of ACMA fluorescence in a time- and concentration-dependent manner for SLC26A6 proteoliposomes. Asterisk indicates addition of the protonophore CCCP, which allows counterion movement and electrogenic Cl− transport to proceed. (D) Coupled Cl−/HCO3− exchange monitored by the time- and concentration-dependent quenching of the fluorophore lucigenin trapped inside proteoliposomes containing SLC26A6. Hashtag indicates the addition of the assayed anion. (E) Coupled Cl−/HCO3− exchange monitored by the time- and concentration-dependent luminescence increase of the HCO3−-selective probe [Eu.L1+] trapped inside proteoliposomes containing SLC26A6. Hashtag indicates the addition of the assayed anion. For details regarding the methods and experimental protocol see Tippett et al. (2023).
SLC26A6-mediated Cl−o/HCO3−i exchange was assessed by measuring Cl− uptake into HCO3−- loaded proteoliposomes using the Cl−-sensitive dye lucigenin (Figure 2D). SLC26A6-mediated Cl−i/HCO3−o exchange was assessed by loading the proteoliposomes with the novel HCO3−-sensitive europium probe [Eu.Lv1+] (Figure 2E). In conclusion, the results suggested that SLC26A6 mediates electroneutral Cl−/HCO3− exchange, and electrogenic Ox2−/Cl− exchange.
The molecular structure of SLC26A6 was determined by cryo-electron microscopy in an inward-facing conformation. A data set of the purified protein at 3.3 Å allowed unambiguous interpretation by an atomic model. Although the transport mode of SLC26A9 and LC26A6 differ remarkably, the overall molecular structures of SLC26A6 and SLC26A6 were found to be quite similar (Figure 3A), (Tippett et al., 2023). This is also true for the other mammalian members of the SLC26A family whose structure was recently identified, implying a common molecular mechanisms underlying their diverse transport functions (Wang et al., 2019; Chi et al., 2020; Bavi et al., 2021; Wang et al., 2021; Ge et al., 2021; Butan et al., 2022; Futamata et al., 2022; Li et al., 2023; Liu et al., 2023; Tippett et al., 2023; Wang et al., 2024). The scaffold (gate) domains of SLC26A9 (Chi et al., 2020) and SLC26A (Tippett et al., 2023) form a rigid structure in the dimer together with the cytoplasmic STAS domains, which remain relatively static during transport. Since the STAS domains contain intervening sequences whose molecular structures are yet unresolved, it is not clear how much movement occurs during the transport process. The scaffold domains do not show pronounced differences between the two paralogs. However, the anion binding sites in the centre of the transport (core) domain facing the gate domain showed distinctly different features between SLC26A9 and SLC26A6. These differences were largely confined to the conformation of the α-helix 10, which forms direct interactions with the transported ions. A comparison of the amino acid sequence lining this short sequence of α10, as well as that of the side chains of α1 and α3 facing the binding pocket, revealed several amino acid substitutions (Figure 3B). Modelling of these different amino acids suggested that the anion binding pocket is considerably deeper in SLC26A9 compared to SLC26A6 (Figures 3C, D). The large arginine in R404, which is also present in most other SLC26 paralogs (all that are known to accept HCO3− in their binding site), but replaced by a valine in SLC26A9, interacts with the residues preceding α3, resulting in a more shallow pocket, and in addition likely also directly interacts with HCO3− and Ox2−.
Figure 3. Comparison of the molecular structure of SLC26A9 and SLC26A6. (A) Ribbon representation of the superimposed SLC26A6 (green, gray) and SLC26A9 (red, blue, PDBID: 7CH1) dimers (B) Sequence alignment of the region constituting the ion binding site of the ten functional human SLC26 paralogs. Conserved residues in the contact region between α1 and α10 are highlighted in green, residues involved in ion interactions in yellow. Deviating residues in SLC26A9 are highlighted in violet. Asterisk marks position that harbors a basic residue in all family members except for SLC26A9, where the residue is replaced by a valine. Whereas most paralogs, including the ones operating as HCO3− exchangers, carry an arginine at this site, the sulfate transporters SLC26A1 and 2 contain a smaller lysine. (C) Cα-representation of the contact region between α1 and α10 of (C) SLC26A6 and SLC26A9. The green ball represents the anion in the binding pocket (D). Slice across a surface of the TM domains of SLC26A6 (C) and SLC26A9 (D) viewed from within the membrane. The spacious aqueous cavity leading to the ion binding site from the cytoplasm is evident. Asterisk indicated the position of the transported ion. Arrows indicate possible movements of the core domain. For details, see Tippett et al. (2023).
In order to test this hypothesis, the investigators mutated the R404 to the amino acid found in SLC26A9. SLC26A6 mutant R404V did not interfere with the protein integrity, and displayed good expression levels in HEK293 cells. Since whole-cell Cl− currents were detectable but low compared to SLC26A9, the SLC26A6 mutant R404V was reconstituted into proteoliposomes. Using the pH-sensitive ACMA dye in the presence of the CCCP protonophore, the SLC26A6 mutant R404V displayed considerable uncoupled Cl− conductance. The Cl−/HCO3− exchange activity, assayed by loading the proteoliposomes with HCO3− and with the Cl− sensitive dye lucigenin, on the other hand, was markedly reduced. These data underpinned the importance of the Arg 404 for anion interactions and coupling, but also demonstrated that converting a SLC26 member with anion exchange characteristics into a fast Cl− conductor requires more structural changes that mutating a single amino acid residue.
Also likely to be involved in transport regulation, but of unknown importance, are the structurally not resolved regions of the “intervening sequence” that links secondary structure elements of the STAS domain. They may be important platforms for interaction with other proteins or with the inner leaflet of the plasma membrane, thus influencing many aspects of anion transport regulation. We do not currently know much about the transport regulation of SLC26A6, but knowledge of the structural basis of these proteins will help us to explore this topic (Tippett et al., 2023).
At the identical time of the publication of the structure-function studies of SLC26A6, the murine Slc26a4 molecular structure has been resolved (Liu et al., 2023). As predicted for all mammalian SLC26 members, Slc26a4 formed homodimers with the anion binding pocket between TMD3 and TMD10. As for SLC26A6, symmetric homodimers in an inwardly facing open state were visualized when the SLC26a4 molecules were frozen in either Cl− or in HCO3− solution. However when a mixture of the two anions was used, a portion of the homodimers were observed in an asymmetic state of inwardly and outwardly open configurations, underlining the function of a Cl−/HCO3− exchanger. Similar experiments may allow the study of the outwardly open configuration for SLC26A6 in the future.
The first investigations on the function of SLC266 were in vitro studies in heterologous expression systems and in isolated epithelia. The heterologous expression studies resulted in controversial results, with both electroneutral Cl−/HCO3− exchange and electrogenic 1Cl−/2HCO3− exchange described (Ko et al., 2002; Chernova et al., 2005; Alper et al., 2006; Shcheynikov et al., 2006). When the slc26a6−/− mouse became available, the first studies were performed in isolated duodenal and jejunal mucosa in so-called Ussing-chambers, in which the luminal solution is gassed with oxygen and the HCO3− output into the lumen is determined by so-called pH-stat microtitration. In this technique, very small additions of acid (in the case of a HCO3− secreting epithelium) by a pH-triggered microtitrator keep the luminal pH constant, and the amount of acid moieties added to do so are being recorded (Wang et al., 2005; Tuo et al., 2006). While there was a reduction in basal HCO3− output, the short circuit current (Ieq) was not different between slc26a6−/− and wt mucosa. In addition, the HCO3− secretory response (ΔJHCO3−) as well as the ΔIeq to forskolin was not significantly reduced in the absence of Slc26a6. In contrast, in cftr−/− duodenal and jejunal mucosa, both basal HCO3− output as well as basal Ieq were significantly reduced, and the ΔJHCO3− as well as the ΔIeq response to forskolin were virtually abolished (Seidler et al., 1997). In the case of Slc26a6 operating in a 1Cl− ion uptake against 2 HCO3− ions output mode, as postulated, one would expect a difference in the basal Ieq or at least in the forskolin-stimulated ΔIeq between the slc26a6−/− and WT tissue (Figure 4). The results therefore favour a Slc26a6-mediated 1Cl−/1HCO3− electroneutral exchange. An advantage of the method is that the driving force for HCO3− exit across the apical membrane is maximal, because the luminal bath is unbuffered and CO2-free, and the rapid gas lift prevents the buildup of unstirred layers. A (hypothetical) caveat is that the absence of CO2 in the luminal bath prevents the physiological CO2 absorption from the lumen, which membrane-bound and intracellular carbonic anhydrases quickly convert to H+ and HCO3−. The decrease of the subapical pHi due to CO2 recycling from the lumen stimulates apical Na+/H+ exchange, and the increase in HCO3− stimulates apical Cl−/HCO3− exchange (Furukawa et al., 2005).
Figure 4. Schematic diagrams of a salt and fluid absorptive and an anion secretory villous enterocyte, depicting the putative role of SLC26A6 in enterocyte anion and fluid absorption and secretion. Most of the immunohistochemical data regarding the location and their trafficking upon stimulation of anion secretion of the transporters are from rodents. (A) Villous enterocyte in the salt absorptive mode. The Na+/H+ exchanger NHE3 and the two Cl−/HCO3− exchangers SLC26A3 and SLC26A3 are located in the microvillar membrane. NHE3 absorbs Na+ in exchange for H+, as long as the sodium pump (ATPA (1–4) is active and intracellular Na+ concentration is low. The luminal exchange Na+/H+ exchange process is strongly stimulated by luminal CO2, which forms from the exported protons and existing HCO3− in the lumen, and results in lowering the pHi of the enterocyte (Xia et al., 2014; Tan et al., 2021). A driving force for the apical Cl−/HCO3− exchangers may be 1. The increase in subapical HCO3−, due to the NHE3-driven proton export, 2. A low intracellular Cl− concentration, established by a putative basolateral Cl− export mechanism (KCl cotransporter? Cl− channel?), 3. In the case of a 2HCO3−/1Cl− exchange mode for SLC26A6 and 2 Cl−/1HCO3− exchange mode for SLC26A3 (Shcheynikov et al., 2006), the negative membrane potential would favour SLC26A6-mediated 2HCO3−/1Cl− exchange. Basolateral transporter activity results in an export of Na+ and Cl− and an import HCO3− via the Na+/H+ exchanger NHE1, the Na+HCO3− cotransporter NBCe1 and possibly also via NBCn1. Water follows the osmotic gradient transcellularly and paracellularly; the latter can be visualized by the widening of the lateral spaces (Larsen et al., 2009). (B) Enterocyte in the anion and fluid secretory mode. Although the expression of the anion secretory machinery is expressed much more strongly in the cryptal area, CFTR and the Na+K+2Cl− cotransporter NKCC1 may also have an overlapping expression with the salt absorptive transporters in some parts of the rodent and human intestine (Jakab et al., 2012b; Tse et al., 2019; Nikolovska et al., 2022; Salari et al., 2023b). While secretory stimuli result in a decrease of microvillar NHE3 abundance, CFTR traffics into the luminal and NKCC1 and NBCe1 into the basolateral membrane (Jakab et al., 2011; Jakab et al. 2012a; Jakab et al. 2012b). AE2 also imports Cl− during anion secretion (Walker et al., 2002). The fast uptake of Cl− from the interstitium prevents a decrease in the intracellular Cl− concentration and cellular shrinkage in the colonic crypt epithelium, abolishing the necessity for luminal Cl− recycling via Slc26a6 and/or Slc26a3 (Bachmann et al., 2003). In the villous epithelium, however, cAMP-induced shrinkage has been reported (Gawenis et al., 2003), and a cell-specific and secretagogue-dependent functional coupling of CFTR and the SLC26 transporters is suggested.
A method to measure HCO3− efflux across the apical membrane without the need to remove the CO2/HCO3− from the luminal bath was developed by the group of Lane Clarke (Simpson et al., 2005). They mounted murine duodenal mucosa into a perfusion chamber that allowed separate perfusion of the luminal and serosal baths, placed the chamber on the stage of a fluorescence microscope, loaded the villi with the pH-sensitive ratiometric dye BCECF, and measured the change of the pHi upon apical removal and replenishment of Cl− in the luminal bath, while the Cl− concentration in the serosal bath was reduced to prevent the replenishment of Cl− from the basolateral side. Calculation of the actual HCO3− flux across the apical membrane requires determination of the intracellular buffer capacity, which is subject to uncertainties in a multicellular tissue layer. Nevertheless, the group used this elegant technique to measure apical Cl−/HCO3− exchange activity in slc26a6−/− and WT mice (Simpson et al., 2007; Simpson et al., 2010), as well as in slc26a3−/− (Walker et al., 2011) and in cftr−/− mice (Simpson et al., 2005). The data suggest that Slc26a6 imports HCO3− during nutrient-induced enterocyte acidification (Simpson et al., 2010), and that membrane depolarisation does not influence Slc26a6-mediated Cl−/HCO3− exchange rate in the absence of CFTR, supporting the concept of electroneutral transport by Slc26a6 (Walker et al., 2011).
Slc26a6 was clearly involved in jejunal Cl− absorption from the luminal bath in isolated mouse jejunal mucosa, and its deletion also resulted in reduced sodium absorption, suggesting a coupling of the apical NHE3 and SLC26a6 during jejunal salt and water absorption (Seidler et al., 2008). In vivo studies supported the role of Slc26a6 in jejunal salt and fluid absorption and, under conditions that acidify the enterocytes, also in HCO3− absorption (Singh et al., 2008a; Xia et al., 2014). During single-pass perfusion of corresponding segments of the upper jejunum of anesthetised slc26a6−/− and WT littermates, the fluid absorptive rates were significantly reduced in the slc26a6−/− mice, as was the case in slc26a3−/− and in slc9a3−/− (nhe3−/−) mice. However, when unbuffered saline titrated to pH 7.4 prior to entry into the intestinal segment was perfused and the pH of the outflow was measured immediately after exit, the genetic deletion of Slc26a6 resulted in a decrease in jejunal fluid absorption, but not in a decrease in luminal pH, as was the case when Slc26a3 was deleted (Figure 5; Table 1) (Xia et al., 2014). This suggests that, under basal conditions of neutral intracellular and luminal pH, Slc26a6 predominantly exchanges luminal Cl− against another entity than HCO3− in vivo. This is most likely oxalate, although additional metabolic waste products or metabolic pathway intermediates cannot be excluded (Figure 4 displays hypothetical models of an absorptive and a secretory enterocyte).
Figure 5. Jejunal fluid absorption rates in (A) NHE3-, (B) SLC26a3- (DRA) and (C) Slc26a6- (PAT-1) deficient mice and their WT littermates. The left bargraphs show the fluid absorption rates in a perfused segment of the upper jejunum in anesthetised WT and gene-deleted mice during perfusion with a prewarmed, unbuffered isoosmolar saline perfusion of pH 7.4. The right bargraphs show the fluid absorption rates after switching the luminal solution to a 95%O2/5% CO2-gassed, HCO3− buffered saline, pH 7.4. CO2 enters the enterocyte, lowers the pHi, but increases both the intracellular proton as well as the HCO3− concentration and stimulates fluid absorption in a NHE3-, DRA- and PAT1-dependent fashion. The subsequent data in the publication show that PAT1 (Slc26a6) presumably imports HCO3− in conditions in which enterocyte pHi is acidic (by CO2 recycling from the lumen) and the luminal HCO3− concentration is high, while SLC26A3 imports Cl−, both in the absence and more so in the presence of luminal CO2/HCO3−. See Xia et al. (2014) for more information.
Table 1. Fluid absorption rates and pH-change between inflow and effluent in NHE3-, DRA- and PAT-1-deficient jejunum during single-pass perfusion experiments with unbuffered saline, pH 7.4 While the outflow is significantly more alkaline in nhe3−/− compared to WT jejunum, as expected when an apical proton extrusion mechanism is missing, and is significantly more acidic in slc26a3−/− (DRA) compared to WT, as expected when an apical HCO3− export mechanism is missing. However, in the slc26a6−/− (PAT-1) compared to WT jejunum, the outflow was slightly less acidic. This suggests that Slc26a6 predominantly exchanges extracellular Cl− against an anion other than HCO3−, most likely oxalate, under the experimental conditions. See Xia et al. (2014) for more information.
In contrast, under conditions of a high luminal CO2 tension and a high luminal HCO3− concentration, for example, after the alkaline pancreatic juice enters the acidic lumen of the duodenum after a meal, Slc26a6 may even import HCO3− into the enterocyte, although we do not know which intracellular anion is exchanged, possibly Cl− in addition to Ox2−, depending on the concentration gradients for the exchangeable anions across the apical membrane (Xia et al., 2014). Indeed, electrogenic oxalate secretion is favoured by the high affinity of oxalate to Slc26a6 (Jiang et al., 2002) and the negative membrane potential of the enterocytes. These in vivo data in the slc26a6-knockout mouse confirmed earlier in vitro data showing that Slc26a6 may function as an oxalate secretory mechanism across the small intestinal mucosa (Knauf et al., 2011). Indeed, slc26a6−/− mice on an oxalate-rich diet develop oxalate kidney stones (Jiang et al., 2006).
In contrast, deletion of Slc26a3, another Cl−/HCO3− exchanger expressed in the brush border membrane of enterocytes, which has a similar expression level and villous-predominant distribution as Slc26a6 in the upper small intestine (Liu et al., 2015; Seidler and Nikolovska, 2019), resulted in an approximately equal reduction of jejunal fluid absorption in vivo than the deletion of Slc26a6, but also resulted in a strong acidification of the effluent of the perfused jejunal segment (Table 1; Xia et al., 2014). Alkalinisation following luminal acid challenge in the duodenum in vivo was also predominantly dependent on SLC26a3 and CFTR expression, but not on Slc26a6 expression (Singh et al., 2008b; Singh et al., 2013). This suggests that in the mouse in vivo, Slc26a3 is the major transport mechanism for luminal alkalinisation in both the small and large intestine in the basal state and after a luminal acid load, but that both Slc26a3 and Slc26a6 are involved in small intestinal fluid absorption. The predominant transport mode for Slc26a3 in the mouse small intestine in vivo appears to be electroneutral Cl−/HCO3− exchange and that of Slc26a6 to be electrogenic Cl−/Ox2− exchange, but the latter may be complemented by electroneutral Slc26a6-dependent Cl−/HCO3− exchange depending on the acid/base status of the lumen the blood and the epithelium (Figure 4). Human SLC26A3 also acts as a major alkalinization mechanism in the human ileum and colon (Holmberg et al., 1975). The role for SLC26A6 is the human gut is not well known, but may at least in part also be anion/oxalate exchange, based on the recent findings about a human SLC26A6 transport defect (Figure 4) (Corniere et al., 2022). Why this is so is presently not clear, given the fact that current data favour an electroneutral Cl−/HCO3− exchange for both human and murine Slc26a6 and Slc26a3 orthologs, an affinity for oxalate for both transporters, and a similar localisation for Slc26a6 and Slc26a3 in the small intestine.
Taken together, a role for SLC26A6 in small intestinal Cl− absorption, and, under situations of high intracellular HCO3− concentration also HCO3−, has been established in vitro and in vivo, and the counterions may be oxalate as well as HCO3−. The role of Slc26a6 for secretagogue-stimulated mall intestinal HCO3− and fluid secretion is less well established. CFTR-mediated Cl− secretion into the lumen of the intestinal crypts and by the high CFTR expressor cells in the rat and human jejunal villi leads to a rapid increase in the uptake of Cl− via the basolateral uptake mechanisms NKCC1 and AE2. This process is enhanced by trafficking of CFTR and NKCC1 into the apical and basolateral membrane, respectively (Jakab et al., 2011; Jakab et al., 2012a; Jakab et al., 2012b). Basolateral Cl− uptake by AE2 will decrease the intracellular HCO3− concentration and favour Cl− secretion over HCO3− secretion via CFTR. A CFTR-dependent Cl− recycling via apical Cl−/HCO3− exchangers Slc26a6 and Slc26a3 may occur to a minor degree in the mouse small intestinal villous area and colonic cryptal mouth area, but the majority of stimulation-associated HCO3− secretion, which is always much lower than the stimulation-associated Cl− secretion, is probably due to the bicarbonate permeability of the CFTR channel (Figure 4). Slc26a6 has been reported to traffick into the rat proximal colonic brush border membrane (BBM) upon cAMP-dependent stimulation by lubiprostone, while Slc26a3 was internalized (Jakab et al., 2012b). Clearly, uncertainties related to antibody specificity, potential species differences, segmental differences that have not been unraveled completely, and as yet incomplete differentiation of intestinal organoids in vitro hamper the applicability of the schematic cartoons constructed for Figure 4, which summarize functional, molecular biological and immunohistochemical data from many different reports. More structure-function studies and the new organoid technology may pave the way for rapid progress in understanding the remaining questions related to the role and the molecular mechanisms of SLC26A6 in intestinal acid-base transport.
In the first description of SLC26A6 cloning and antibody generation, an immunohistochemical image of human pancreatic ducts was shown, with apical staining with an anti-SLC26A6 antibody (Lohi et al., 2000). mRNA expression for SLC26A6 was also found high in human pancreatic tissue. Subsequently, in vitro studies were performed in isolated pancreatic ducts from slc26a6−/− and WT mice (Wang et al., 2006; Ishiguro et al., 2007; Song et al., 2009) and guinea pigs (Stewart et al., 2009; Stewart et al., 2011). A sophisticated combination of electrophysiological and fluorometric techniques during ion substitution and inhibitor applications was used to study the electrogenicity of Cl−/HCO3− exchange across the luminal membrane of mouse interlobular ducts during cAMP stimulation (to open CFTR channels), and the results were interpreted as a demonstration of a 2 HCO3−/1 Cl− coupling of Slc26a6 and, in its absence, the appearance of a 1 HCO3−/2Cl−exchanger in the luminal membrane, probably Slc26a3 (Song et al., 2012). Although the results were carefully contained, I see alternative explanations for the data, based on scientific advances made in the last decade. Firstly, the pancreatic ductal epithelium, particularly of humans and guinea pigs, expresses high levels of CFTR in the luminal membrane, but low if any levels of the Na+K+2Cl− cotransporter NKCC1 in the basolateral membrane, in contrast to the situation in small intestinal and colonic crypts, which strongly express both CFTR and NKCC1 (Jakab et al., 2011; Yamaguchi et al., 2017; Salari et al., 2023a). Secondly, while intestinal crypts and, to a lesser extent, villous cells also express basolateral AE2, which can serve as an alternative Cl− uptake mechanism in exchange for intracellular HCO3−, the rat pancreatic duct cells express basolateral Na+HCO3− cotransporters and Na+/H+ exchangers, but do not express AE2 (Roussa et al., 2001). The situation in the human and guinea pig pancreatic ducts, which secrete an isotonic Na+(K+)-HCO3− solution, is completely different from that in the intestine, because the basolateral membrane lacks the Cl− absorptive transporters NKCC1 and AE2, and the strongly expressed basolateral NBCe1 favours the uptake of HCO3−, but not of Cl− (Ishiguro et al., 2012). Low intracellular Cl− stimulates the WNK pathway in pancreatic duct cells, resulting in an increase in the HCO3− conductance of the CFTR channel (Park et al., 2010). Recent computational modelling of the composition of ion transporters in the guinea pig (and presumably human) pancreatic ducts suggested that in the proximal small ducts, Slc26a6 exchanges luminal Cl− against intracellular HCO3− with either 1:1 or 1:2 coupling, but that the rising intraductal HCO3− and decreasing Cl− concentrations rapidly inhibit this exchange, and HCO3− is secreted via the CFTR channel. In order to achieve an isomolar cation-HCO3− concentration in the distal pancreatic duct, low activity of basolateral Cl− uptake pathways is a key requirement. This is in line with current evidence (Yamaguchi et al., 2017). A recent publication investigated the contribution of Slc26a6 to stimulated pancreatic juice secretion by the ex vivo mouse pancreas and found that the genetic deletion of Slc26a6 reduced the ex vivo fluid secretory rate by approximately 35% (Munemasa et al., 2019). The contribution to pancreatic fluid secretion was HCO3−-dependent. The authors also concluded that another major HCO3−-independent pathway is the primary driver of the fluid secretion process in the mouse pancreas. Interestingly, they localised Slc26a6 to the apical membrane of the pancreatic acini (Munemasa et al., 2019). This study also suggests that the other Slc26 members that mediate Cl−/HCO3− exchange and have been found expressed in pancreatic ducts (Andharia et al., 2018), are likely to be of minor importance for pancreatic ductal secretion in the mouse.
In contrast to mutations in SLC26A2, SLC26A3, and SLC26A4, that have been cloned during the search for the molecular cause of hereditable diseases in humans, variants in SLC26A6, although sought for in databases with genetic information on patient groups, have not been associated with human diseases until recently. An obvious search focus was on patient cohorts with pancreatic disease. However, no associations were found between the incidence of chronic pancreatitis and potentially disease causing variants in SLC26A6 (Balazs et al., 2015). At the time, this was surprising because Slc26a6 has been described as essential for CFTR-dependent pancreatic HCO3− secretion (Wang et al., 2006) and because the association of CFTR variants, even clinically mild ones, or those which only affected the CFTR-dependent HCO3− transport, with chronic idiopathic pancreatitis have been found in several cohorts (Bernardino et al., 2003; Lee et al., 2003; Weiss et al., 2005; LaRusch et al., 2014; Berke et al., 2022). One explanation may be that the rodent pancreas is not an ideal model for the assessment of human pancreatic fluid dynamics, as the rodent pancreas is capable of sustaining relatively high fluid secretory rates in the absence of HCO3− (Munemasa et al., 2019). In addition, the rodent pancreas does not develop severe pancreatic disease in the absence of CFTR expression, possibly due to the presence of alternative Cl− channels (Clarke et al., 1994). In contrast, human pancreatic fluid secretion, like that of the guinea pig, is strongly dependent on HCO3− availability and the ability of the CFTR channel to mediate HCO3− secretion.
A second focus for exploring the relevance of SLC26A6 mutations has been on kidney stone cohorts, or cohorts with a high risk of developing nephrolithiasis, because of the importance of Slc26a6 for intestinal secretion of oxalate in mice and the high affinity of oxalate for the human SLC26A6 protein. However, the first studies did not show an association between SLC26A6 variants and hyperoxaluria/hyperoxalemia or the risk of nephrolithiasis in these cohorts (Monico et al., 2008; Corbetta et al., 2009; Scherer et al., 2023). Recently however, a young female patient with very severe recurrent oxalate nephrolithiasis was identified in the Department of Metabolic and Renal Diseases of the University hospital in Amiens, France (Corniere et al., 2022). Biochemical analysis of the stone revealed it to be calcium oxalate. Urine analysis showed hyperoxaluria and mild hypocitraturia, and dietary history showed very low calcium intake but no excessive intake of oxalate-rich food. Whole exon sequencing identified a rare heterozygous missense mutation (c.1519C > T/p.R507W) in the SLC26A6 gene, but no mutations in other risk genes for oxalate nephrolithiasis. The father carried the same mutation and showed hyperoxaluria but not hypocitraturia and a very high daily fluid intake which may have prevented the formation of oxalate kidney stones. WT and the mutant SLC26A6 was expressed in OKP (opossum kidney) cells and the effect of the p.R507W mutation on the expression and function of human SLC26A6 was investigated. The mutant was less stable in the membrane and showed a reduced transport rate for Cl−/Ox2− exchange compared to WT Slc26A6. Interestingly, cotransfection studies showed strong dominant-negative effects of the mutant on the wild-type protein. This may explain why a heterozygous mutation results in a severe phenotype. In silico analysis of the mutant, based on the then published SLC26A9 structure (that of SLC26A6 not having been published yet), suggested that the mutation was situated in the last helix of the transmembrane domain close to the cytoplasmic STAS domain and may interfere with the optimal insertion of the SLC26A6 protein into the plasma membrane (Figure 6). This is the first strong evidence that mutations in the SLC26A6 gene that result in a functionally defective SLC26A6-mediated oxalate transport are strong risk factors for the development of oxalate nephropathy. Additional dietary factors were required for the stone phenotype, one of these factors being the low Ca2+ diet of this patient, since the change to a Ca2+-rich diet and sufficient fluid intake prevented further stone formation. The same is true for slc26a6−/− mice, which develop nephrolithiasis during high oxalate uptake, but not with a low oxalate diet (Seidler and Nikolovska, 2019).
Figure 6. In silico analysis of the putative effects of the R507W substitution on the SLC26A6 protein structure. Panel (A): Structural model of the human SLC26A6 protein. The two subunits are presented with one subunit colored in green and the other in orange (cartoon representation). The olive spheres represent the boundary of the lipid bilayer as computed with the PPM server. A zone predicted to interact with the Cl− ion as proposed in the mouse Slc26a9 experimental structure is highlighted in subunit A for orientation. R507 residues of both subunits are flagged; this residue is located on the last helix of the transmembrane domain. The insertion loop that could not be built is shown as a dashed line. The mutation is expected to destabilize the protein and to impede optimal insertion into the lipid bilayer. Panel (B): Analysis of the region surrounding R507. R507 makes several hydrogen bonds with it surrounding (magenta dashed lines), form a salt bridge with D682 (red dashed line) and has hydrophobic/aromatic contacts (brown dashed line) with, for example, F680. Panel (B), inset: Attempts to replace R507 on the computer screen by a tryptophan generate multiple steric clashes, illustrated here by several red disks. Alternative orientations of the W side chain would also create even more severe clashes, and certainly impede appropriate interaction with the other subunit, damage the oligomeric structure and the helical structure and could indirectly affect proper orientation/association with the membrane. See Corniere et al. (2022) for more information.
Other groups have also found polymorphisms in the SLC26A6 gene in kidney stone cohorts, but the functional significance of the individual polymorphisms for oxalate transport has not been elucidated in the same detail as in the study discussed above, and therefore the functional involvement of these variants in the development of the nephrolithiasis of these patients not certain (Monico et al., 2008; Corbetta et al., 2009; Lu et al., 2016). An interesting study from Israel also reported two polymorphisms in the STAS domain of SLC26A6 in two heterozygous carriers. These patients both had low urinary citrate concentrations (also a major risk factor for the development of Ca2+oxalate kidney stones), but only one patient developed Ca2+ oxalate stone. SLC26A6 mutants corresponding to the observed variants of the two patients were constructed by site-directed mutagenesis, expressed and functionally characterised in HEK293 cells. The one homolog mutation of the patient that had developed Ca2+ oxalate stones abolished the expression and function of SLC26A6 and impaired the regulation of SLC13-mediated citrate transport by SLC26A6. The SLC26A6 variant of the second patient showed reduced SLC26A6 protein expression and membrane trafficking, retained full transport activity and impaired the regulation of the citrate transporter (Shimshilashvili et al., 2020). The data follow on earlier work in heterologous expression systems and in mice that described a regulatory effect of SLC26A6 on the sodium-dicarboxylate cotransporter NaDC-1, which primarily mediates the co-transport of Na+ and tricarboxylic acid cycle intermediates, such as citrate and succinate (Ohana et al., 2013; Khamaysi et al., 2019). Taken together, the results from mutation screening of patient cohorts demonstrate the variable impact that SLC26A6 mutations may have on enteric and/or renal transport physiology. Future investigations of the anion transport defect (if any) associated with additional SLC26A6 mutations, by similar site directed mutagenesis and structure function studies as performed by Corniere et al., and Shimshilashvili et al., may broaden the spectrum of SLC6A6-associated human diseases.
One major experimental problem in unraveling the contributions of different anion transporters to a given physiological event was the lack of specific inhibitors. Both the chloride channels blockers, such as NPPB, and the anion transport inhibitors, such as the stilbene derivatives, were fairly nonspecific, inhibiting some, but not all, members of the SLC4 and SLC26 gene family, as well as some Cl− channels, but also less closely related biological structures such as the mitochondrial permeability transition pore (Boron, 2001; Sanders et al., 2012; Liu et al., 2016). Accordingly, nonwanted and nonspecific effects are problematic for the interpretation of the experimental data. Therefore, elucidating the regulation of SLC26a6 in the epithelia that endogenously express the transporter has been challenging, given the fact that epithelia may express different SLC26 isoforms in the same cell and even the same membrane. The few things that we know about the physiologic significance of endogenous Slc26a6 have been generated in slc26a6−/− mice (described and cited in the previous paragraphs), or in genetically manipulated cell lines with endogenous SLC26A6 expression (Freel et al., 2009; Anbazhagan et al., 2019; Arvans et al., 2023). In contrast, immunohistochemical data are often from rat intestine, because nonspecific effects are less problematic with polyclonal antibodies generated in rabbits, and monoclonal antibodies generated in mouse. In addition, the slc26a6−/− mouse may have adapted to the loss of this transporter by upregulation of other anion transport pathways, thus obscuring its biological significance.
Recently, specific inhibitors have been generated for several Cl− channels, as well as for members of the SLC26 family, including SLC26A6. In 2021, the group of Alan Verkman first described the identification of a highly selective SLC26A6 inhibitor by high throughput screening, using as a readout the inhibition of Cl− efflux, in exchange for iodide, from anion-transporter-transfected FRT cells by fluorometric assessment of YFP quenching (Cil et al., 2021). A concentration that fully inhibited SLC26a6-mediated Cl− efflux did not affect Cl− efflux from FRT cells transfected with SLC26A3, A4, A9, and TMEM16a, and also did not affect the CFTR-mediated Isc-response to forskolin in a bronchial cell line. The group also tested the compound, named PAT1inh-B01, as well as the SLC26A3 (DRA)–inhibitor DRAinh-A270, in the murine intestine using an in vivo closed loop model, in which different segments of the intestine are tied off, filled with drug or vehicle-containing NaCl solution, excised and weighted after a certain time period. These results suggested that Slc26a6 was invovled to a similar degree in salt and water absorption as Slc26a3 in salt and water absorption as Slc26a3 in the murine jejunum, but was the major Cl− absorption pathway in the ileum, while Slc26a3 was the major absorption pathway in the distal colon (Figure 7). The group also tested the efficacy of PAT1inh-B01 in the ileum of mice with a F508del mutation in the CFTR gene, and found that the inhibitor completely blocked NaCl- and fluid absorption in this segment of the F508del mutant mouse, as did the NHE3 inhibitor tenapanor, whereas the Slc26a3-inhibitor DRAinh-A270 was without effect. The authors concluded that Slc26a6 and Slc26a3 mediate Cl− and water absorption in the murine intestine in a segment-specific manner. Based on the selective role of SLC26a6 in ileal fluid absorption in both WT and F508del mice, the authors speculate that the drug may be beneficial for the small intestinal obstructive problems in patients with cystic fibrosis. A more recent publication presented data on a more potent, also selective Slc26a6 inhibitor name PAT1inh-A0030 (Chu et al., 2023). The availability of these inhibitors may greatly facilitate the elucidation of the physiological role of Slc26a6 in cellular and organ function. However, a caveat against its long-term use, in particular in the kidney stone prone CF patient population, may come from a study by Xia et al. (2014), who studied the fluid absorptive and HCO3− secretion rates in anesthetised Slc26a6-, Slc26a3, NHE3-and NHE2-deleted mice by single-pass perfusion. They also found similar reductions in jejunal fluid absorption in slc26a6−/− and slc26a3−/− mice, as did Cil et al. (2021). However, a reduction in luminal HCO3− output, as expected for the deletion of an apical Cl−/HCO3− exchanger, was only observed in slc26a3−/− mice. This suggests that murine jejunal Slc26a6 exchanges luminal Cl− (because that was the only anion present in the luminal perfusate) for an anion other than HCO3−. The most likely candidate is oxalate (Jiang et al., 2002). The high oxalate but low Cl− affinity of the human compared to the mouse SLC26A6 paralogue suggests that the inhibition of intestinal SLC26A6 in human may increase the risk of hyperoxalemia in individuals at risk (Clark et al., 2008). On the other hand, if SLC26A6 does indeed predominantly exchanges luminal Cl− for HCO3− in the human ileum, its inhibition may be equally or more beneficial for prevention of distal ileal obstruction (DIOS) than the inhibition of NHE3-mediated luminal Na+/H+ exchange, which has been shown to both inhibit fluid absorption and increase HCO3− output in the small and large intestine in CFTR-deficient mice (Tan et al., 2021), as well as reduce the risk of intestinal obstructions in these mice when given orally for 3 weeks (Tan et al., 2022), and for which the intestine-specific, FDA-approved inhibitor tenapanor is available (Dominguez Rieg and Rieg, 2024). However, it has also been demonstrated in murine intestine and in cell culture, that NHE3 is involved in exporting protons that are absorbed by the proton-coupled peptide (PEPT-1), amino acid transporter SLC36A1, and short chain fatty acid absorbing monodecarboxylate transporter MCT-1, which all acidify the enterocyte during the absorption of the respective nutrient that they transport, raising concerns about negative effects of NHE3-inhibition on nutrient absorption (Tamai et al., 1995; Thwaites et al., 2002; Inigo et al., 2006; Chen et al., 2010). On the other hand, CF patients whose functional defect in the CFTR protein is amenable to CFTR-targeted therapy have shown positive effects on weight development by CFTR-corrector/potentiator therapy, suggesting that multiple options may soon be available to treat the defects in intestinal function in CF patients (Guimbellot et al., 2019; Regard et al., 2023). Careful observation on nutrient balance will be necessary when treating CF patients with inhibitors for acid/base and electrolyte transport in the intestine.
Figure 7. Region specific effects of the inhibitors for Slc26a6 (PAT1), Slc26a3 (DRA) and Slc9a3 (NHE3) in the mouse intestine. Upper panel shows the effect of luminal PAT1inh-B01 (30 μM), DRAinh-A270 (10 μM), and tenapanor (10 μM), individually and together, on loop weight-to-length ratio at 30 min in mouse (A) midjejunal closed loops, (B) ileal closed loops, and (C) distal colonic closed loops. (D) Cartoon schematically displays the differential effect of the inhibitors for PAT1, DRA and NHE3 in the mouse intestine. See Cil et al. (2021) for further details.
The multifunctional anion exchanger SLC26A6 was cloned 25 years ago, but its exact localisation, transport regulation and physiological significance at the organ, cellular and subcellular level are still being elucidated, and controversial data exist in the literature. In recent years, there have been major advances in the detailed understanding of the physiological functions of SLC26A6: Firstly, the elucidation of its structure at high resolution and the subsequent structure-function studies help to resolve the controversies regarding the transport mode of the different anions and allow a better interpretation of the published physiological experiments. Secondly, the first detailed functional analysis of a disease-causing SLC26A6 mutation detected in the genome of a patient with severe hyperoxaluria and nephrolithiasis has recently been described. Finally, selective inhibitors of SLC26A6 transport function have been identified. The new knowledge, combined with new tools, will pave the way for more detailed structure-function analyses andn more insight into the physiological role and pathological consequences of genetic defects in SLC26A6. Specific anti-SLC26A6 antibodies or/and an epitope-tagged SLC26A6 knock in mouse are urgently needed to gain insight into the cellular localisation of SLC26A6 throughout the body, to analyse its transport regulation and to find ways to target SLC26A6 for therapy.
US: Writing–original draft, Writing–review and editing.
The author(s) declare that financial support was received for the research, authorship, and/or publication of this article. The publication costs for this article will be covered by the funds allocated to publication fees by the DFG Forschergruppe FOR 5046.
The author is deeply grateful to Eric Geertsma, Raimund Dutzler, Dominique Eladari, Alan Verkman and Onur Cil for making Figures 1–3, 6, 7 available for reproduction in this review, and for reviewing and modifying the respective text and/or the figure legend. Furthermore, my deep thanks go to Mahdi Amiri for helping me with the figure and reference layout.
The author declares that the research was conducted in the absence of any commercial or financial relationships that could be construed as a potential conflict of interest.
The authors declare that no Generative AI was used in the creation of this manuscript.
All claims expressed in this article are solely those of the authors and do not necessarily represent those of their affiliated organizations, or those of the publisher, the editors and the reviewers. Any product that may be evaluated in this article, or claim that may be made by its manufacturer, is not guaranteed or endorsed by the publisher.
Alper, S. L., and Sharma, A. K. (2013). The SLC26 gene family of anion transporters and channels. Mol. Asp. Med. 34, 494–515. doi:10.1016/j.mam.2012.07.009
Alper, S. L., Stewart, A. K., Chernova, M. N., Zolotarev, A. S., Clark, J. S., and Vandorpe, D. H. (2006). Anion exchangers in flux: functional differences between human and mouse SLC26A6 polypeptides. Novartis Found. Symp. 273, 107–264.
Anbazhagan, A. N., Priyamvada, S., Borthakur, A., Saksena, S., Gill, R. K., Alrefai, W. A., et al. (2019). miR-125a-5p: a novel regulator of SLC26A6 expression in intestinal epithelial cells. Am. J. Physiol. Cell Physiol. 317, C200–C208. doi:10.1152/ajpcell.00068.2019
Andharia, N., Hayashi, M., and Matsuda, H. (2018). Electrophysiological properties of anion exchangers in the luminal membrane of Guinea pig pancreatic duct cells. Pflugers Arch. 470, 897–907. doi:10.1007/s00424-018-2116-1
Aronson, P. S. (2010). Role of SLC26A6-mediated Cl−-oxalate exchange in renal physiology and pathophysiology. J. Nephrol. 23 (Suppl. 16), S158–S164.
Arvans, D., Chang, C., Alshaikh, A., Tesar, C., Babnigg, G., Wolfgeher, D., et al. (2023). Sel1-like proteins and peptides are the major Oxalobacter formigenes-derived factors stimulating oxalate transport by human intestinal epithelial cells. Am. J. Physiol. Cell Physiol. 325, C344–C361. doi:10.1152/ajpcell.00466.2021
Bachmann, O., Wüchner, K., Rossmann, H., Leipziger, J., Osikowska, B., Colledge, W. H., et al. (2003). Expression and regulation of the Na+-K+-2Cl-cotransporter NKCC1 in the normal and CFTR-deficient murine colon. J. Physiol. 549 (Pt 2), 525–536. doi:10.1113/jphysiol.2002.030205
Balazs, A., Ruffert, C., Hegyi, E., Hritz, I., Czako, L., Takacs, T., et al. (2015). Genetic analysis of the bicarbonate secreting anion exchanger SLC26A6 in chronic pancreatitis. Pancreatology 15, 508–513. doi:10.1016/j.pan.2015.08.008
Bavi, N., Clark, M. D., Contreras, G. F., Shen, R., Reddy, B. G., Milewski, W., et al. (2021). The conformational cycle of prestin underlies outer-hair cell electromotility. Nature 600, 553–558. doi:10.1038/s41586-021-04152-4
Berke, G., Gede, N., Szadai, L., Ocskay, K., Hegyi, P., Sahin-Toth, M., et al. (2022). Bicarbonate defective CFTR variants increase risk for chronic pancreatitis: a meta-analysis. PLoS One 17, e0276397. doi:10.1371/journal.pone.0276397
Bernardino, A. L., Guarita, D. R., Mott, C. B., Pedroso, M. R., Machado, M. C., Laudanna, A. A., et al. (2003). CFTR, PRSS1 and SPINK1 mutations in the development of pancreatitis in Brazilian patients. JOP 4, 169–177.
Butan, C., Song, Q., Bai, J. P., Tan, W. J. T., Navaratnam, D., and Santos-Sacchi, J. (2022). Single particle cryo-EM structure of the outer hair cell motor protein prestin. Nat. Commun. 13, 290. doi:10.1038/s41467-021-27915-z
Chang, Y. N., and Geertsma, E. R. (2017). The novel class of seven transmembrane segment inverted repeat carriers. Biol. Chem. 398, 165–174. doi:10.1515/hsz-2016-0254
Chen, M., Singh, A., Xiao, F., Dringenberg, U., Wang, J., Engelhardt, R., et al. (2010). Gene ablation for PEPT1 in mice abolishes the effects of dipeptides on small intestinal fluid absorption, short-circuit current, and intracellular pH. Am. J. Physiol. Gastrointest. Liver Physiol. 299, G265–G274. doi:10.1152/ajpgi.00055.2010
Chernova, M. N., Jiang, L., Friedman, D. J., Darman, R. B., Lohi, H., Kere, J., et al. (2005). Functional comparison of mouse slc26a6 anion exchanger with human SLC26A6 polypeptide variants: differences in anion selectivity, regulation, and electrogenicity. J. Biol. Chem. 280, 8564–8580. doi:10.1074/jbc.M411703200
Chi, X., Jin, X., Chen, Y., Lu, X., Tu, X., Li, X., et al. (2020). Structural insights into the gating mechanism of human SLC26A9 mediated by its C-terminal sequence. Cell Discov. 6, 55. doi:10.1038/s41421-020-00193-7
Chu, T., Karmakar, J., Haggie, P. M., Tan, J. A., Master, R., Ramaswamy, K., et al. (2023). Selective isoxazolopyrimidine PAT1 (SLC26A6) inhibitors for therapy of intestinal disorders. RSC Med. Chem. 14, 2342–2347. doi:10.1039/d3md00302g
Cil, O., Haggie, P. M., Tan, J. T., Rivera, A. A., and Verkman, A. S. (2021). SLC26A6-selective inhibitor identified in a small-molecule screen blocks fluid absorption in small intestine. JCI Insight 6, e147699. doi:10.1172/jci.insight.147699
Clark, J. S., Vandorpe, D. H., Chernova, M. N., Heneghan, J. F., Stewart, A. K., and Alper, S. L. (2008). Species differences in Cl-affinity and in electrogenicity of SLC26A6-mediated oxalate/Cl-exchange correlate with the distinct human and mouse susceptibilities to nephrolithiasis. J. Physiol. 586, 1291–1306. doi:10.1113/jphysiol.2007.143222
Clarke, L. L., Grubb, B. R., Yankaskas, J. R., Cotton, C. U., Mckenzie, A., and Boucher, R. C. (1994). Relationship of a non-cystic fibrosis transmembrane conductance regulator-mediated chloride conductance to organ-level disease in Cftr(-/-) mice. Proc. Natl. Acad. Sci. U. S. A. 91, 479–483. doi:10.1073/pnas.91.2.479
Compton, E. L., Page, K., Findlay, H. E., Haertlein, M., Moulin, M., Zachariae, U., et al. (2014). Conserved structure and domain organization among bacterial Slc26 transporters. Biochem. J. 463, 297–307. doi:10.1042/BJ20130619
Corbetta, S., Eller-Vainicher, C., Frigerio, M., Valaperta, R., Costa, E., Vicentini, L., et al. (2009). Analysis of the 206M polymorphic variant of the SLC26A6 gene encoding a Cl-oxalate transporter in patients with primary hyperparathyroidism. Eur. J. Endocrinol. 160, 283–288. doi:10.1530/EJE-08-0623
Corniere, N., Thomson, R. B., Thauvin, S., Villoutreix, B. O., Karp, S., Dynia, D. W., et al. (2022). Dominant negative mutation in oxalate transporter SLC26A6 associated with enteric hyperoxaluria and nephrolithiasis. J. Med. Genet. 59, 1035–1043. doi:10.1136/jmedgenet-2021-108256
Detro-Dassen, S., Schanzler, M., Lauks, H., Martin, I., Zu Berstenhorst, S. M., Nothmann, D., et al. (2008). Conserved dimeric subunit stoichiometry of SLC26 multifunctional anion exchangers. J. Biol. Chem. 283, 4177–4188. doi:10.1074/jbc.M704924200
Dominguez Rieg, J. A., and Rieg, T. (2024). New functions and roles of the Na(+)-H(+)-exchanger NHE3. Pflugers Arch. 476, 505–516. doi:10.1007/s00424-024-02938-9
Freel, R. W., Morozumi, M., and Hatch, M. (2009). Parsing apical oxalate exchange in Caco-2BBe1 monolayers: siRNA knockdown of SLC26A6 reveals the role and properties of PAT-1. Am. J. Physiol. Gastrointest. Liver Physiol. 297, G918–G929. doi:10.1152/ajpgi.00251.2009
Furukawa, O., Hirokawa, M., Zhang, L., Takeuchi, T., Bi, L. C., Guth, P. H., et al. (2005). Mechanism of augmented duodenal HCO(3)(-) secretion after elevation of luminal CO(2). Am. J. Physiol. Gastrointest. Liver Physiol. 288, G557–G563. doi:10.1152/ajpgi.00344.2004
Futamata, H., Fukuda, M., Umeda, R., Yamashita, K., Tomita, A., Takahashi, S., et al. (2022). Author Correction: cryo-EM structures of thermostabilized prestin provide mechanistic insights underlying outer hair cell electromotility. Nat. Commun. 13, 7337. doi:10.1038/s41467-022-34985-0
Gawenis, L. R., Franklin, C. L., Simpson, J. E., Palmer, B. A., Walker, N. M., Wiggins, T. M., et al. (2003). cAMP inhibition of murine intestinal Na/H exchange requires CFTR-mediated cell shrinkage of villus epithelium. Gastroenterology 125 (4), 1148–1163. doi:10.1016/s0016-5085(03)01212-5
Ge, J., Elferich, J., Dehghani-Ghahnaviyeh, S., Zhao, Z., Meadows, M., von Gersdorff, H., et al. (2021). Molecular mechanism of prestin electromotive signal amplification. Cell 184 (18), 4669–4679.e13. doi:10.1016/j.cell.2021.07.034
Geertsma, E. R., Chang, Y. N., Shaik, F. R., Neldner, Y., Pardon, E., Steyaert, J., et al. (2015). Structure of a prokaryotic fumarate transporter reveals the architecture of the SLC26 family. Nat. Struct. Mol. Biol. 22, 803–808. doi:10.1038/nsmb.3091
Guimbellot, J., Solomon, G. M., Baines, A., Heltshe, S. L., Vandalfsen, J., Joseloff, E., et al. (2019). Effectiveness of ivacaftor in cystic fibrosis patients with non-G551D gating mutations. J. Cyst. Fibros. 18, 102–109. doi:10.1016/j.jcf.2018.04.004
Holmberg, C., Perheentupa, J., and Launiala, K. (1975). Colonic electrolyte transport in health and in congenital chloride diarrhea. J. Clin. investigation 56 (2), 302–310. doi:10.1172/JCI108094
Inigo, C., Barber, A., and Lostao, M. P. (2006). Na+ and pH dependence of proline and beta-alanine absorption in rat small intestine. Acta Physiol. (Oxf) 186, 271–278. doi:10.1111/j.1748-1716.2006.01538.x
Ishiguro, H., Namkung, W., Yamamoto, A., Wang, Z., Worrell, R. T., Xu, J., et al. (2007). Effect of Slc26a6 deletion on apical Cl-/HCO3- exchanger activity and cAMP-stimulated bicarbonate secretion in pancreatic duct. Am. J. Physiol. Gastrointest. Liver Physiol. 292, G447–G455. doi:10.1152/ajpgi.00286.2006
Ishiguro, H., Yamamoto, A., Nakakuki, M., Yi, L., Ishiguro, M., Yamaguchi, M., et al. (2012). Physiology and pathophysiology of bicarbonate secretion by pancreatic duct epithelium. Nagoya J. Med. Sci. 74, 1–18.
Jakab, R. L., Collaco, A. M., and Ameen, N. A. (2011). Physiological relevance of cell-specific distribution patterns of CFTR, NKCC1, NBCe1, and NHE3 along the crypt-villus axis in the intestine. Am. J. Physiol. Gastrointest. Liver Physiol. 300, G82–G98. doi:10.1152/ajpgi.00245.2010
Jakab, R. L., Collaco, A. M., and Ameen, N. A. (2012a). Cell-specific effects of luminal acid, bicarbonate, cAMP, and carbachol on transporter trafficking in the intestine. Am. J. physiology. Gastrointest. liver physiology 303 (8), G937–G950. doi:10.1152/ajpgi.00452.2011
Jakab, R. L., Collaco, A. M., and Ameen, N. A. (2012b). Lubiprostone targets prostanoid signaling and promotes ion transporter trafficking, mucus exocytosis, and contractility. Dig. Dis. Sci. 57 (11), 2826–2845. doi:10.1007/s10620-012-2352-8
Jiang, Z., Asplin, J. R., Evan, A. P., Rajendran, V. M., Velazquez, H., Nottoli, T. P., et al. (2006). Calcium oxalate urolithiasis in mice lacking anion transporter Slc26a6. Nat. Genet. 38, 474–478. doi:10.1038/ng1762
Jiang, Z., Grichtchenko, I., Boron, W. F., and Aronson, P. S. (2002). Specificity of anion exchange mediated by mouse Slc26a6. J. Biol. Chem. 277, 33963–33967. doi:10.1074/jbc.M202660200
Khamaysi, A., Anbtawee-Jomaa, S., Fremder, M., Eini-Rider, H., Shimshilashvili, L., Aharon, S., et al. (2019). Systemic succinate homeostasis and local succinate signaling affect blood pressure and modify risks for calcium oxalate lithogenesis. J. Am. Soc. Nephrol. 30, 381–392. doi:10.1681/ASN.2018030277
Knauf, F., Ko, N., Jiang, Z., Robertson, W. G., Van Itallie, C. M., Anderson, J. M., et al. (2011). Net intestinal transport of oxalate reflects passive absorption and SLC26A6-mediated secretion. J. Am. Soc. Nephrol. 22, 2247–2255. doi:10.1681/ASN.2011040433
Knauf, F., Yang, C. L., Thomson, R. B., Mentone, S. A., Giebisch, G., and Aronson, P. S. (2001). Identification of a chloride-formate exchanger expressed on the brush border membrane of renal proximal tubule cells. Proc. Natl. Acad. Sci. U. S. A. 98, 9425–9430. doi:10.1073/pnas.141241098
Ko, S. B., Shcheynikov, N., Choi, J. Y., Luo, X., Ishibashi, K., Thomas, P. J., et al. (2002). A molecular mechanism for aberrant CFTR-dependent HCO(3)(-) transport in cystic fibrosis. EMBO J. 21, 5662–5672. doi:10.1093/emboj/cdf580
Kuwabara, M. F., Haddad, B. G., Lenz-Schwab, D., Hartmann, J., Longo, P., Huckschlag, B. M., et al. (2023). Elevator-like movements of prestin mediate outer hair cell electromotility. Nat. Commun. 14 (1), 7145. doi:10.1038/s41467-023-42489-8
Larsen, E. H., Willumsen, N. J., Møbjerg, N., and Sørensen, J. N. (2009). The lateral intercellular space as osmotic coupling compartment in isotonic transport. Acta physiol. Oxf. Engl. 195 (1), 171–186. doi:10.1111/j.1748-1716.2008.01930.x
Larusch, J., Jung, J., General, I. J., Lewis, M. D., Park, H. W., Brand, R. E., et al. (2014). Mechanisms of CFTR functional variants that impair regulated bicarbonate permeation and increase risk for pancreatitis but not for cystic fibrosis. PLoS Genet. 10, e1004376. doi:10.1371/journal.pgen.1004376
Lee, J. H., Choi, J. H., Namkung, W., Hanrahan, J. W., Chang, J., Song, S. Y., et al. (2003). A haplotype-based molecular analysis of CFTR mutations associated with respiratory and pancreatic diseases. Hum. Mol. Genet. 12, 2321–2332. doi:10.1093/hmg/ddg243
Li, J., Huang, S., Liu, S., Liao, X., Yan, S., and Liu, Q. (2023). SLC26 family: a new insight for kidney stone disease. Front. Physiol. 14, 1118342. doi:10.3389/fphys.2023.1118342
Liu, G., Wang, Z. K., Wang, Z. Y., Yang, D. B., Liu, Z. P., and Wang, L. (2016). Mitochondrial permeability transition and its regulatory components are implicated in apoptosis of primary cultures of rat proximal tubular cells exposed to lead. Arch. Toxicol. 90, 1193–1209. doi:10.1007/s00204-015-1547-0
Liu, Q., Zhang, X., Huang, H., Chen, Y., Wang, F., Hao, A., et al. (2023). Asymmetric pendrin homodimer reveals its molecular mechanism as anion exchanger. Nat. Commun. 14 (1), 3012. doi:10.1038/s41467-023-38303-0
Liu, X., Li, T., Riederer, B., Lenzen, H., Ludolph, L., Yeruva, S., et al. (2015). Loss of Slc26a9 anion transporter alters intestinal electrolyte and HCO3− transport and reduces survival in CFTR-deficient mice. Pflugers Arch. 467, 1261–1275. doi:10.1007/s00424-014-1543-x
Lohi, H., Kujala, M., Kerkela, E., Saarialho-Kere, U., Kestila, M., and Kere, J. (2000). Mapping of five new putative anion transporter genes in human and characterization of SLC26A6, a candidate gene for pancreatic anion exchanger. Genomics 70, 102–112. doi:10.1006/geno.2000.6355
Lu, X., Sun, D., Xu, B., Pan, J., Wei, Y., Mao, X., et al. (2016). In silico screening and molecular dynamic study of nonsynonymous single nucleotide polymorphisms associated with kidney stones in the SLC26A6 gene. J. Urol. 196, 118–123. doi:10.1016/j.juro.2016.01.093
Monico, C. G., Weinstein, A., Jiang, Z., Rohlinger, A. L., Cogal, A. G., Bjornson, B. B., et al. (2008). Phenotypic and functional analysis of human SLC26A6 variants in patients with familial hyperoxaluria and calcium oxalate nephrolithiasis. Am. J. Kidney Dis. 52, 1096–1103. doi:10.1053/j.ajkd.2008.07.041
Munemasa, T., Mukaibo, T., and Melvin, J. E. (2019). Slc26a6 is an apical membrane anion exchanger that drives HCO3−-dependent fluid secretion in murine pancreatic acinar cells. Am. J. Physiol. Cell Physiol. 317, C1153–C1160. doi:10.1152/ajpcell.00257.2019
Neumeier, L. I., Thomson, R. B., Reichel, M., Eckardt, K. U., Aronson, P. S., and Knauf, F. (2020). Enteric oxalate secretion mediated by Slc26a6 defends against hyperoxalemia in murine models of chronic kidney disease. J. Am. Soc. Nephrol. 31, 1987–1995. doi:10.1681/ASN.2020010105
Nikolovska, K., Cao, L., Hensel, I., Di Stefano, G., Seidler, A. E., Zhou, K., et al. (2022). Sodium/hydrogen-exchanger-2 modulates colonocyte lineage differentiation. Acta physiol. Oxf. Engl. 234 (3), e13774. doi:10.1111/apha.13774
Ohana, E. (2015). Transepithelial ion transport across duct cells of the salivary gland. Oral Dis. 21, 826–835. doi:10.1111/odi.12201
Ohana, E., Shcheynikov, N., Moe, O. W., and Muallem, S. (2013). SLC26A6 and NaDC-1 transporters interact to regulate oxalate and citrate homeostasis. J. Am. Soc. Nephrol. 24, 1617–1626. doi:10.1681/ASN.2013010080
Ohana, E., Yang, D., Shcheynikov, N., and Muallem, S. (2009). Diverse transport modes by the solute carrier 26 family of anion transporters. J. Physiol. 587, 2179–2185. doi:10.1113/jphysiol.2008.164863
Park, H. W., Nam, J. H., Kim, J. Y., Namkung, W., Yoon, J. S., Lee, J. S., et al. (2010). Dynamic regulation of CFTR bicarbonate permeability by [Cl-]i and its role in pancreatic bicarbonate secretion. Gastroenterology 139, 620–631. doi:10.1053/j.gastro.2010.04.004
Regard, L., Martin, C., Da Silva, J., and Burgel, P. R. (2023). CFTR modulators: current status and evolving knowledge. Semin. Respir. Crit. Care Med. 44, 186–195. doi:10.1055/s-0042-1758851
Roussa, E., Alper, S. L., and Thevenod, F. (2001). Immunolocalization of anion exchanger AE2, Na(+)/H(+) exchangers NHE1 and NHE4, and vacuolar type H(+)-ATPase in rat pancreas. J. Histochem Cytochem 49, 463–474. doi:10.1177/002215540104900406
Salari, A., Xiu, R., Amiri, M., Pallenberg, S. T., Schreiber, R., Dittrich, A. M., et al. (2023b). The anion channel TMEM16a/Ano1 modulates CFTR activity, but does not function as an apical anion channel in colonic epithelium from cystic fibrosis patients and healthy individuals. Int. J. Mol. Sci. 24, 14214. doi:10.3390/ijms241814214
Salari, A., Zhou, K., Nikolovska, K., Seidler, U., and Amiri, M. (2023a). Human colonoid-myofibroblast coculture for study of apical Na+/H+ exchangers of the lower cryptal neck region. Int. J. Mol. Sci. 24 (5), 4266. doi:10.3390/ijms24054266
Sanders, K. M., Zhu, M. H., Britton, F., Koh, S. D., and Ward, S. M. (2012). Anoctamins and gastrointestinal smooth muscle excitability. Exp. Physiol. 97, 200–206. doi:10.1113/expphysiol.2011.058248
Scherer, L., Schonauer, R., Nemitz-Kliemchen, M., Hagemann, T., Hantmann, E., De Fallois, J., et al. (2023). Delta weight loss unlike genetic variation associates with hyperoxaluria after malabsorptive bariatric surgery. Sci. Rep. 13, 9029. doi:10.1038/s41598-023-35941-8
Seidler, U., Blumenstein, I., Kretz, A., Viellard-Baron, D., Rossmann, H., Colledge, W. H., et al. (1997). A functional CFTR protein is required for mouse intestinal cAMP-cGMP- and Ca(2+)-dependent HCO3- secretion. J. Physiol. 505 (Pt 2), 411–423. doi:10.1111/j.1469-7793.1997.411bb.x
Seidler, U., and Nikolovska, K. (2019). Slc26 family of anion transporters in the gastrointestinal tract: expression, function, regulation, and role in disease. Compr. Physiol. 9, 839–872. doi:10.1002/cphy.c180027
Seidler, U., Rottinghaus, I., Hillesheim, J., Chen, M., Riederer, B., Krabbenhoft, A., et al. (2008). Sodium and chloride absorptive defects in the small intestine in Slc26a6 null mice. Pflugers Arch. 455, 757–766. doi:10.1007/s00424-007-0318-z
Shcheynikov, N., Wang, Y., Park, M., Ko, S. B., Dorwart, M., Naruse, S., et al. (2006). Coupling modes and stoichiometry of Cl−/HCO3− exchange by slc26a3 and slc26a6. J. Gen. Physiol. 127, 511–524. doi:10.1085/jgp.200509392
Shcheynikov, N., Yang, D., Wang, Y., Zeng, W., Karniski, L. P., So, I., et al. (2008). The Slc26a4 transporter functions as an electroneutral Cl−/I-/HCO3− exchanger: role of Slc26a4 and Slc26a6 in I- and HCO3− secretion and in regulation of CFTR in the parotid duct. J. Physiol. 586, 3813–3824. doi:10.1113/jphysiol.2008.154468
Shimshilashvili, L., Aharon, S., Moe, O. W., and Ohana, E. (2020). Novel human polymorphisms define a key role for the SLC26A6-STAS domain in protection from Ca2+-oxalate lithogenesis. Front. Pharmacol. 11, 405. doi:10.3389/fphar.2020.00405
Simpson, J. E., Gawenis, L. R., Walker, N. M., Boyle, K. T., and Clarke, L. L. (2005). Chloride conductance of CFTR facilitates basal Cl-/HCO3- exchange in the villous epithelium of intact murine duodenum. Am. J. Physiol. Gastrointest. Liver Physiol. 288, G1241–G1251. doi:10.1152/ajpgi.00493.2004
Simpson, J. E., Schweinfest, C. W., Shull, G. E., Gawenis, L. R., Walker, N. M., Boyle, K. T., et al. (2007). PAT-1 (Slc26a6) is the predominant apical membrane Cl−/HCO3− exchanger in the upper villous epithelium of the murine duodenum. Am. J. Physiol. Gastrointest. Liver Physiol. 292, G1079–G1088. doi:10.1152/ajpgi.00354.2006
Simpson, J. E., Walker, N. M., Supuran, C. T., Soleimani, M., and Clarke, L. L. (2010). Putative anion transporter-1 (Pat-1, Slc26a6) contributes to intracellular pH regulation during H+-dipeptide transport in duodenal villous epithelium. Am. J. Physiol. Gastrointest. Liver Physiol. 298, G683–G691. doi:10.1152/ajpgi.00293.2009
Singh, A. K., Amlal, H., Haas, P. J., Dringenberg, U., Fussell, S., Barone, S. L., et al. (2008a). Fructose-induced hypertension: essential role of chloride and fructose absorbing transporters PAT1 and Glut5. Kidney Int. 74, 438–447. doi:10.1038/ki.2008.184
Singh, A. K., Liu, Y., Riederer, B., Engelhardt, R., Thakur, B. K., Soleimani, M., et al. (2013). Molecular transport machinery involved in orchestrating luminal acid-induced duodenal bicarbonate secretion in vivo. J. Physiol. 591, 5377–5391. doi:10.1113/jphysiol.2013.254854
Singh, A. K., Riederer, B., Chen, M., Xiao, F., Krabbenhoft, A., Engelhardt, R., et al. (2010). The switch of intestinal Slc26 exchangers from anion absorptive to HCOFormula secretory mode is dependent on CFTR anion channel function. Am. J. Physiol. Cell Physiol. 298, C1057–C1065. doi:10.1152/ajpcell.00454.2009
Singh, A. K., Sjoblom, M., Zheng, W., Krabbenhoft, A., Riederer, B., Rausch, B., et al. (2008b). CFTR and its key role in in vivo resting and luminal acid-induced duodenal HCO3- secretion. Acta Physiol. (Oxf) 193, 357–365. doi:10.1111/j.1748-1716.2008.01854.x
Sirish, P., Ledford, H. A., Timofeyev, V., Thai, P. N., Ren, L., Kim, H. J., et al. (2017). Action potential shortening and impairment of cardiac function by ablation of Slc26a6. Circ. Arrhythm. Electrophysiol. 10, e005267. doi:10.1161/CIRCEP.117.005267
Song, Y., Ishiguro, H., Yamamoto, A., Jin, C. X., and Kondo, T. (2009). Effects of Slc26a6 deletion and CFTR inhibition on HCO3- secretion by mouse pancreatic duct. J. Med. Invest. 56 (Suppl. l), 332–335. doi:10.2152/jmi.56.332
Song, Y., Yamamoto, A., Steward, M. C., Ko, S. B., Stewart, A. K., Soleimani, M., et al. (2012). Deletion of Slc26a6 alters the stoichiometry of apical Cl-/HCO-3 exchange in mouse pancreatic duct. Am. J. Physiol. Cell Physiol. 303, C815–C824. doi:10.1152/ajpcell.00151.2012
Stewart, A. K., Shmukler, B. E., Vandorpe, D. H., Reimold, F., Heneghan, J. F., Nakakuki, M., et al. (2011). SLC26 anion exchangers of Guinea pig pancreatic duct: molecular cloning and functional characterization. Am. J. Physiol. Cell Physiol. 301, C289–C303. doi:10.1152/ajpcell.00089.2011
Stewart, A. K., Yamamoto, A., Nakakuki, M., Kondo, T., Alper, S. L., and Ishiguro, H. (2009). Functional coupling of apical Cl−/HCO3− exchange with CFTR in stimulated HCO3− secretion by Guinea pig interlobular pancreatic duct. Am. J. Physiol. Gastrointest. Liver Physiol. 296, G1307–G1317. doi:10.1152/ajpgi.90697.2008
Tamai, I., Takanaga, H., Maeda, H., Sai, Y., Ogihara, T., Higashida, H., et al. (1995). Participation of a proton-cotransporter, MCT1, in the intestinal transport of monocarboxylic acids. Biochem. Biophys. Res. Commun. 214, 482–489. doi:10.1006/bbrc.1995.2312
Tan, Q., Di Stefano, G., Tan, X., Renjie, X., Romermann, D., Talbot, S. R., et al. (2021). Inhibition of Na(+)/H(+) exchanger isoform 3 improves gut fluidity and alkalinity in cystic fibrosis transmembrane conductance regulator-deficient and F508del mutant mice. Br. J. Pharmacol. 178, 1018–1036. doi:10.1111/bph.15323
Tan, X., Kini, A., Romermann, D., and Seidler, U. (2022). The NHE3 inhibitor tenapanor prevents intestinal obstructions in CFTR-deleted mice. Int. J. Mol. Sci. 23, 9993. doi:10.3390/ijms23179993
Thwaites, D. T., Kennedy, D. J., Raldua, D., Anderson, C. M., Mendoza, M. E., Bladen, C. L., et al. (2002). H/dipeptide absorption across the human intestinal epithelium is controlled indirectly via a functional Na/H exchanger. Gastroenterology 122, 1322–1333. doi:10.1053/gast.2002.32992
Tippett, D. N., Breen, C., Butler, S. J., Sawicka, M., and Dutzler, R. (2023). Structural and functional properties of the transporter SLC26A6 reveal mechanism of coupled anion exchange. Elife 12, RP87178. doi:10.7554/eLife.87178
Tse, C. M., Yin, J., Singh, V., Sarker, R., Lin, R., Verkman, A. S., et al. (2019). cAMP stimulates SLC26A3 activity in human colon by a CFTR-dependent mechanism that does not require CFTR activity. Cell Mol. Gastroenterol. Hepatol. 7 (3), 641–653. doi:10.1016/j.jcmgh.2019.01.002
Tuo, B., Riederer, B., Wang, Z., Colledge, W. H., Soleimani, M., and Seidler, U. (2006). Involvement of the anion exchanger SLC26A6 in prostaglandin E2-but not forskolin-stimulated duodenal HCO3- secretion. Gastroenterology 130, 349–358. doi:10.1053/j.gastro.2005.10.017
Waldegger, S., Moschen, I., Ramirez, A., Smith, R. J., Ayadi, H., Lang, F., et al. (2001). Cloning and characterization of SLC26A6, a novel member of the solute carrier 26 gene family. Genomics 72, 43–50. doi:10.1006/geno.2000.6445
Walker, N. M., Flagella, M., Gawenis, L. R., Shull, G. E., and Clarke, L. L. (2002). An alternate pathway of cAMP-stimulated Cl secretion across the NKCC1-null murine duodenum. Gastroenterology 123 (2), 531–541. doi:10.1053/gast.2002.34757
Walker, N. M., Simpson, J. E., Hoover, E. E., Brazill, J. M., Schweinfest, C. W., Soleimani, M., et al. (2011). Functional activity of Pat-1 (Slc26a6) Cl−/HCO3− exchange in the lower villus epithelium of murine duodenum. Acta Physiol. (Oxf) 201, 21–31. doi:10.1111/j.1748-1716.2010.02210.x
Walter, J. D., Sawicka, M., and Dutzler, R. (2019). Cryo-EM structures and functional characterization of murine Slc26a9 reveal mechanism of uncoupled chloride transport. Elife 8, 46986. doi:10.7554/eLife.46986
Wang, C., Sun, B., Zhang, X., Huang, X., Zhang, M., Guo, H., et al. (2019). Structural mechanism of the active bicarbonate transporter from cyanobacteria. Nat. Plants 5, 1184–1193. doi:10.1038/s41477-019-0538-1
Wang, J., Wang, W., Wang, H., and Tuo, B. (2020). Physiological and pathological functions of SLC26A6. Front. Med. (Lausanne) 7, 618256. doi:10.3389/fmed.2020.618256
Wang, L., Chen, K., and Zhou, M. (2021). Structure and function of an Arabidopsis thaliana sulfate transporter. Nat. Commun. 12, 4455. doi:10.1038/s41467-021-24778-2
Wang, L., Hoang, A., Gil-Iturbe, E., Laganowsky, A., Quick, M., and Zhou, M. (2024). Mechanism of anion exchange and small-molecule inhibition of pendrin. Nat. Comm. 15 (1), 346. doi:10.1038/s41467-023-44612-1
Wang, Y., Soyombo, A. A., Shcheynikov, N., Zeng, W., Dorwart, M., Marino, C. R., et al. (2006). Slc26a6 regulates CFTR activity in vivo to determine pancreatic duct HCO3− secretion: relevance to cystic fibrosis. EMBO J. 25, 5049–5057. doi:10.1038/sj.emboj.7601387
Wang, Z., Petrovic, S., Mann, E., and Soleimani, M. (2002). Identification of an apical Cl−/HCO3− exchanger in the small intestine. Am. J. Physiol. Gastrointest. Liver Physiol. 282, G573–G579. doi:10.1152/ajpgi.00338.2001
Wang, Z., Wang, T., Petrovic, S., Tuo, B., Riederer, B., Barone, S., et al. (2005). Renal and intestinal transport defects in Slc26a6-null mice. Am. J. Physiol. Cell Physiol. 288, C957–C965. doi:10.1152/ajpcell.00505.2004
Weiss, F. U., Simon, P., Bogdanova, N., Mayerle, J., Dworniczak, B., Horst, J., et al. (2005). Complete cystic fibrosis transmembrane conductance regulator gene sequencing in patients with idiopathic chronic pancreatitis and controls. Gut 54, 1456–1460. doi:10.1136/gut.2005.064808
Xia, W., Yu, Q., Riederer, B., Singh, A. K., Engelhardt, R., Yeruva, S., et al. (2014). The distinct roles of anion transporters Slc26a3 (DRA) and Slc26a6 (PAT-1) in fluid and electrolyte absorption in the murine small intestine. Pflugers Arch. 466, 1541–1556. doi:10.1007/s00424-013-1381-2
Keywords: anion exchange, cryo-microscopy, SLC26 family, hyperuricemia, nephrolithiasis, pancreatic secretion, acid base balance, intestine
Citation: Seidler UE (2025) The enigmatic SLC26A6 multifunctional anion transporter: recent advances in structure-function relationship, pathophysiological significance and novel pharmacological inhibitors. Front. Pharmacol. 15:1536864. doi: 10.3389/fphar.2024.1536864
Received: 29 November 2024; Accepted: 31 December 2024;
Published: 30 January 2025.
Edited by:
Nicoletta Pedemonte, Giannina Gaslini Institute (IRCCS), ItalyReviewed by:
Marcelo Catalan, Austral University of Chile, ChileCopyright © 2025 Seidler. This is an open-access article distributed under the terms of the Creative Commons Attribution License (CC BY). The use, distribution or reproduction in other forums is permitted, provided the original author(s) and the copyright owner(s) are credited and that the original publication in this journal is cited, in accordance with accepted academic practice. No use, distribution or reproduction is permitted which does not comply with these terms.
*Correspondence: Ursula E. Seidler, c2VpZGxlci51cnN1bGFAbWgtaGFubm92ZXIuZGU=
Disclaimer: All claims expressed in this article are solely those of the authors and do not necessarily represent those of their affiliated organizations, or those of the publisher, the editors and the reviewers. Any product that may be evaluated in this article or claim that may be made by its manufacturer is not guaranteed or endorsed by the publisher.
Research integrity at Frontiers
Learn more about the work of our research integrity team to safeguard the quality of each article we publish.