- 1School of Clinical Medical, Chengdu Medical College, Chengdu, China
- 2Department of Pharmacy, The First Affiliated Hospital of Chengdu Medical College, Chengdu, China
- 3Department of Respiratory and Critical Care Medicine, The First Affiliated Hospital of Chengdu Medical College, Chengdu, China
- 4College of Foreign Languages and Cultures, Sichuan University, Chengdu, China
Apigenin (C15H10O5, API) is a natural flavonoid widely found in vegetables, fruits, and plants such as celery, oranges, and chamomile. In recent years, API has attracted considerable attention as a dietary supplement due to its low toxicity, non-mutagenic properties and remarkable therapeutic efficacy in various diseases. In particular, evidence from a large number of preclinical studies suggests that API has promising effects in the prevention and treatment of a variety of liver diseases, including multifactorial liver injury, non-alcoholic fatty liver disease/non-alcoholic steatohepatitis, liver fibrosis and liver cancer. This paper provides a comprehensive review of the progress of research into the therapeutic applications of API in liver diseases as of August 2024, based on literature retrieved from databases such as Web of Science, PubMed, CNKI, Google Scholar and ScienceDirect. The hepatoprotective effects of API involve multiple molecular mechanisms, including inhibition of inflammation, alleviation of hepatic oxidative stress, amelioration of insulin resistance, promotion of fatty acid oxidation, inhibition of liver cancer cell proliferation and differentiation, and induction of tumour cell apoptosis. More importantly, signaling pathways such as Nrf2, NF-κB, PI3K/Akt/mTOR, NLRP3, Wnt/β-catenin, TGF-β1/Smad3, AMPK/SREBP, PPARα/γ, MAPKs, and Caspases are identified as key targets through which API exerts its beneficial effects in various liver diseases. Studies on its toxicity and pharmacokinetics indicate that API has low toxicity, is slowly metabolized and excreted in vivo, and has low oral bioavailability. In addition, the paper summarises and discusses the sources, physicochemical properties, new dosage forms, and current challenges and opportunities of API, with the aim of providing direction and rationale for the further development and clinical application of API in the food, pharmaceutical and nutraceutical fields.
1 Introduction
The liver is a crucial metabolic organ essential for maintaining normal physiological functions, including detoxification, protein synthesis, lipid metabolism, bile secretion, and glucose regulation (Kubes and Jenne, 2018; Zaefarian et al., 2019). Dysfunction of the liver not only disrupts the body’s metabolic balance but can also lead to systemic diseases (Ginès et al., 2022). Unfortunately, liver diseases have become a significant global health challenge, with pathologies ranging from non-alcoholic fatty liver disease (NAFLD) and alcoholic liver disease (ALD) to viral hepatitis, liver fibrosis, and hepatocellular carcinoma (HCC) (Ginès et al., 2022; Devarbhavi et al., 2023). Despite remarkable advances in modern medicine in the diagnosis and treatment of liver diseases, current therapeutic approaches still face limitations, including suboptimal efficacy, significant drug side effects and considerable inter-individual variability (Neshat et al., 2021). In chronic liver diseases, the interplay between inflammation, oxidative stress, and apoptosis often exacerbates the condition, leading to progressive deterioration (Szabo and Petrasek, 2015; Wang et al., 2021b; Allameh et al., 2023). Therefore, the development of safe, effective, and multi-targeted therapeutic strategies is of paramount importance.
Natural products, known for their diverse biological activities and low toxicity, have become a research focus in recent years (Wang et al., 2021a; Dai et al., 2023; Wang et al., 2023). Apigenin (API), a naturally occurring flavonoid, has attracted significant attention due to its broad pharmacological activities, particularly its potential in liver protection (Salehi et al., 2019). Widely distributed in various vegetables, medicinal plants, and fruits, API exhibits notable antioxidant, anti-inflammatory, anti-fibrotic, and anti-tumor activities, positioning it as a promising candidate for the treatment of liver diseases (Alshehri et al., 2019). Research has shown that API exerts its hepatoprotective effects through multiple mechanisms, including inhibition of inflammatory responses, alleviation of hepatic oxidative stress, improvement of insulin resistance, regulation of lipid metabolism, inhibition of liver cancer cell proliferation and differentiation, and induction of tumor cell apoptosis (Chiang et al., 2006; Wang et al., 2017; Goudarzi et al., 2021; Chou et al., 2024; Hsu et al., 2024). More importantly, studies have identified key signaling pathways involved in API-mediated liver protection, including nuclear factor erythroid 2-related factor 2 (Nrf2), nuclear factor kappa-B (NF-κB), phosphatidylinositide 3-kinase (PI3K)/protein kinase B (AKT)/mammalian target of rapamycin (mTOR), NOD-like receptor family pyrin domain containing 3 (NLRP3), Wnt/β-catenin, farnesoid X receptor (FXR), transforming growth factor-β1 (TGF-β1)/mothers against decapentaplegic homolog 3 (Smad3), and peroxisome proliferator-activated receptor alpha (PPARα) (Feng et al., 2017; Yang J. et al., 2018; Yue et al., 2020; Ji et al., 2021; Pan et al., 2021; Zheng et al., 2021; Meng et al., 2022).
However, there is currently a lack of systematic reviews on the sources, physicochemical properties, hepatoprotective effects, toxicity, pharmacokinetics, and novel formulations of API. Therefore, this paper aims to provide a comprehensive review of the research progress on API in the treatment of liver diseases up to August 2024, by searching databases such as Web of Science, PubMed, CNKI, Google Scholar, and ScienceDirect. In addition, this review will reflect on the limitations of existing research and propose future research directions to provide a solid theoretical foundation for the clinical application of API.
2 Sources and characteristics of API
API (C15H10O5, Figure 1) is a naturally occurring flavonoid, often referred to as a “phytoestrogen.” It is widely found in various plants, vegetables, and fruits, including parsley, celery, mint, chamomile, thyme, lettuce, asparagus, oranges, and grapefruit, with particularly high concentrations in parsley and celery (Figure 2) (Madunić et al., 2018; Alshehri et al., 2019). Studies have shown that API extracted from celery constitutes 17% of the total flavonoid content (Crozier et al., 1997). In recent years, API has garnered increasing attention as a dietary supplement due to its low toxicity, non-mutagenic properties, and significant therapeutic efficacy in various diseases.
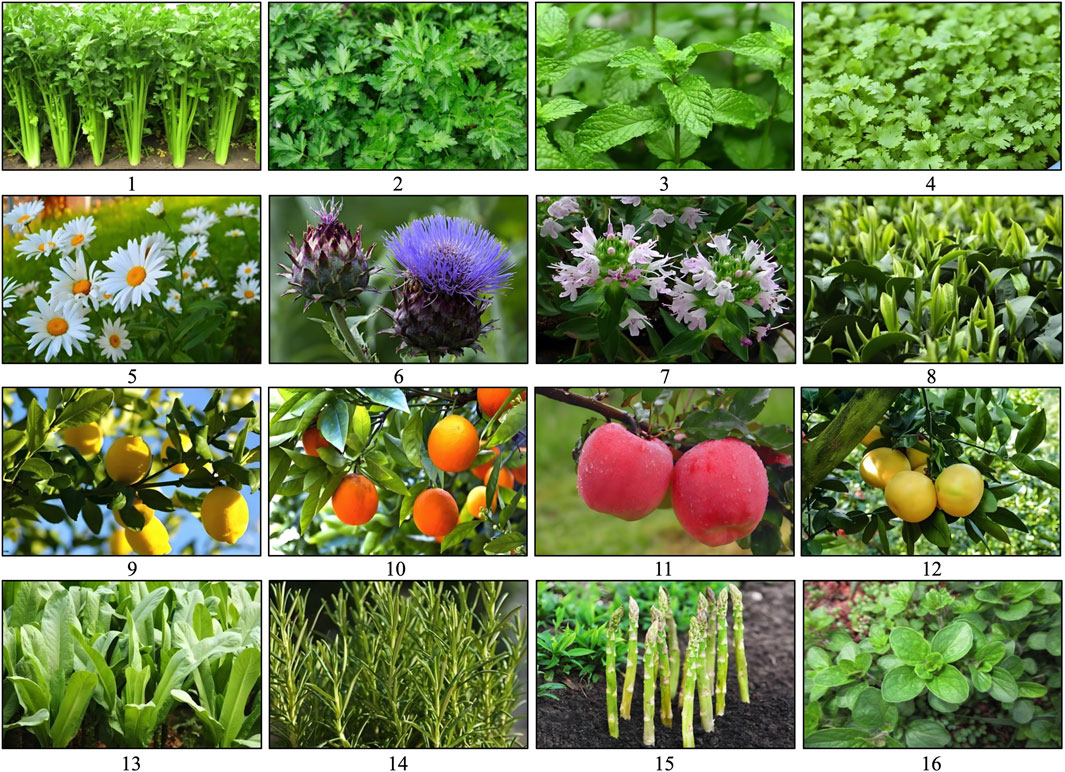
Figure 2. Sources of API. 1: Celery; 2: Parsley; 3. Mint; 4: Coriander; 5: Chamomile; 6: Artichoke; 7: Thyme; 8: Green tea; 9: Lemon; 10: Orange; 11. Apple; 12: Grapefruit; 13: Lettuce; 14: Rosemary; 15: Asparagus; 16: Oregano. Referring to the picture is from the website: http://www.gbif.org; https://image.baidu.com.
Physically, API is a yellow crystalline powder with a molecular weight of 270.24 g/mol and a density of 1.548 g/cm³. API has very low solubility in water. In contrast, it has good solubility in organic solvents such as ethanol, potassium hydroxide, and dimethyl sulfoxide (Tang et al., 2017). These physical properties limit its application in aqueous systems. However, its biological activity can be fully utilized through proper solvent selection. Chemically, API contains three phenolic hydroxyl groups and two carbon-carbon double bonds, which impart strong antioxidant activity (Zhou et al., 2023). API effectively scavenges free radicals, inhibits lipid peroxidation, and demonstrates significant anti-inflammatory, anticancer, and antimicrobial potential (Wang et al., 2014; Yang J. et al., 2018; Zarei et al., 2021). Moreover, the phenolic hydroxyl groups of API can chelate metal ions, further enhancing its antioxidant capacity (Spiegel and Sroka, 2023).
In summary, API shows potential for a wide range of applications in the food, pharmaceutical and nutraceutical fields due to its excellent antioxidant and other bioactive activities. Detailed data on the physical and chemical properties of API are presented in Table 1. Data obtained from https://china.guidechem.com.
3 The hepatoprotective effects and mechanisms of API in liver injury
3.1 Drug-induced liver injury
3.1.1 Acetaminophen
Acetaminophen (APAP), also known as N-acetyl-p-aminophenol, is a widely used analgesic and antipyretic drug globally (Ishitsuka et al., 2020). However, improper or excessive use of APAP can lead to severe liver damage. Mitochondrial oxidative stress is considered a key mechanism in APAP-induced liver injury (Yan et al., 2018). Typically, APAP is metabolized in the liver and detoxified via glucuronidation and sulfation pathways (Yan et al., 2018). However, when consumed in excess, these pathways become saturated, leading to the formation of the toxic metabolite N-acetyl-p-benzoquinone imine (NAPQI) via the cytochrome P450 enzyme system (Jaeschke and Ramachandran, 2024). The accumulation of NAPQI within hepatocytes causes cellular damage and necrosis, ultimately leading to liver failure (Jaeschke and Ramachandran, 2024). Therefore, it is crucial to identify effective clinical antidotes to mitigate APAP-induced acute liver injury.
In 2013, Yang et al. (2013) first reported that API (100, 200 mg/kg) exhibited significant protective effects against APAP-induced acute liver injury in mice. This beneficial effect may be attributed to the increased activity of glutathione reductase (GR), which in turn elevated hepatic glutathione (GSH) levels (Yang et al., 2013). Similarly, Rašković et al. (2017) found that API (10 mg/kg) attenuated APAP-induced liver injury in rats by inhibiting lipid peroxidation levels and enhancing enzymatic antioxidant defense mechanisms. Subsequently, Zhao et al. (2020) and Zhang et al. (2020) further confirmed the ameliorative effects of API in APAP-induced liver injury through both in vivo and in vitro experiments. On the one hand, API can alleviate APAP-induced liver injury by promoting autophagy through the regulation of the SIRT1-p53 axis, thus reducing inflammation and oxidative stress (Zhao et al., 2020). On the other hand, API can reverse APAP-induced liver injury by activating the AMPK/GSK-3β signaling pathway, promoting CPT1A activity, and activating the Nrf2 antioxidant pathway (Zhang et al., 2020).
3.1.2 Methotrexate
Methotrexate (MTX) is an antimetabolite drug widely used in the treatment of cancer, autoimmune diseases, and chronic inflammatory conditions (Mahmoud et al., 2023). However, high doses or prolonged use of MTX can lead to liver injury through various mechanisms, including inflammatory responses, oxidative stress, mitochondrial dysfunction, and metabolic disturbances, making hepatotoxicity one of the main side effects in clinical applications (Shetty et al., 2017). Therefore, to enhance the safety of MTX therapy, it is essential to adjust the dosage based on the patient’s specific condition or consider adjunctive treatments.
Studies have shown that pretreatment with API (20 mg/kg) significantly improves the levels of hepatic antioxidant markers (GSH, CAT, GPX and SOD) in mice with MTX-induced liver injury, and reduces levels of liver function indicators (AST, ALT and ALP) and inflammatory factors (TNF-α and IL-1β) (Goudarzi et al., 2021). Another study also found that pretreatment with API (3 mg/kg) alleviated MTX-induced liver injury by restoring the antioxidant defense system (MDA, SOD, CAT, GSH-Px and GSH), and reducing the expression of apoptotic factors (Caspase-3) and inflammatory factors (CRP, G-CSF and iNOS) (Sahindokuyucu-Kocasari et al., 2021). In summary, API can prevent MTX-induced liver injury by reducing inflammation and oxidative stress.
3.1.3 Cyclophosphamide
Cyclophosphamide (CYC) is an alkylating agent widely used in the treatment of cancer and autoimmune diseases (Ahlmann and Hempel, 2016). However, its clinical use is limited by dose-dependent toxicity, particularly hepatotoxicity (Emadi et al., 2009). CYC-induced liver injury is mainly mediated by its metabolites, phosphoramide mustard and acrolein, which can trigger oxidative stress, lipid peroxidation, and inflammatory responses, leading to hepatocyte apoptosis and necrosis, thereby exacerbating liver injury (Moore, 1991; Üsküdar Cansu et al., 2019). Therefore, in clinical practice, to reduce CYC-induced liver injury, it is necessary to regularly monitor liver function and consider the use of antioxidants or other protective agents for adjunctive therapy. Fortunately, Al-Amarat et al. (2022) have shown that pretreatment with API (20 and 40 mg/kg) significantly ameliorates CYC-induced liver injury in rats, reducing levels of ALT, AST, ALP, and LDH, and inhibiting the expression of ROS, LPO, NF-κB, pro-inflammatory mediators (TNF-α, IL-6 and iNOS), and apoptotic markers (Bax and Caspase-3). Mechanistically, the hepatoprotective effect of API is associated with the upregulation of the Nrf2/HO-1 signaling pathway and enhanced antioxidant defense (Al-Amarat et al., 2022).
3.2 Chemical liver injury
3.2.1 Furan
Furan (Fu) is a highly hepatotoxic industrial chemical and food contaminant, with liver injury primarily mediated by oxidative stress and inflammatory responses induced by its metabolites (Batool et al., 2021). In the liver, Fu is metabolized by the cytochrome P450 enzyme system to form reactive intermediates that react with cellular biomolecules, leading to lipid peroxidation, protein, and DNA damage (de Conti et al., 2017). Interestingly, Wang et al. (2014) demonstrated that API (5, 10, and 20 mg/kg) effectively alleviated Fu-induced liver and kidney injury. On the one hand, API increased the activity of GSH, GST and SOD, and reduced the levels of MPO, MDA and ROS (Wang et al., 2014). On the other hand, API decreased levels of pro-inflammatory cytokines IL-1β, IL-6 and TNF-α, and increased the level of the anti-inflammatory cytokine IL-10 (Wang et al., 2014). Mechanistically, the protective effects of API are mainly attributed to its excellent free radical scavenging ability and inhibition of lipid peroxidation (Wang et al., 2014). Therefore, consuming API-rich foods or using it as a dietary supplement could be significant for individuals at risk of Fu toxicity.
3.2.2 Carbon tetrachloride
Carbon tetrachloride (CCl4) is a potent hepatotoxic substance widely used in experimental models to induce liver injury, liver fibrosis, cirrhosis, and liver cancer (Scholten et al., 2015). In the body, CCl4 is metabolized by microsomal enzymes in hepatocytes into trichloromethyl radicals (·CCl3) and trichloromethyl peroxy radicals (·CCl3O2) (Weber et al., 2003). These reactive intermediates can react with cellular membrane lipids, proteins, and DNA, leading to lipid peroxidation, membrane damage, and DNA breaks (Unsal et al., 2021). In a mouse model of CCl4-induced acute liver injury, API (50, 100, 200 mg/kg) significantly alleviated lipid peroxidation, as evidenced by increased levels of SOD, GSH, GSH-Px and CAT, and decreased levels of MDA (Yue et al., 2020). Additionally, API reduced the inflammatory response by decreasing the secretion of TNF-α and IL-6, and increasing the secretion of IL-10 (Yue et al., 2020). Similarly, in an H2O2-induced HepG2 cell model, API (10, 20, and 40 µM) reversed the imbalance between SOD, GSH activity and excessive ROS, and reduced the expression of IL-6 and TNF-α (Yue et al., 2020). In summary, API may alleviate liver injury by inhibiting the non-canonical NF-κB pathway, thereby reducing inflammation and oxidative stress (Yue et al., 2020).
3.2.3 Di (2-ethylhexyl) phthalate
Di (2-ethylhexyl) phthalate (DEHP) is a widely used plasticizer commonly found in various plastic products (Zhang X. et al., 2022). Unfortunately, DEHP exhibits significant hepatotoxicity and can infiltrate daily life through various routes, posing potential harm to the liver (Martínez-Razo et al., 2021). In a DEHP-induced AML12 cell model, DEHP induced ferroptosis by increasing ROS levels, disrupting iron homeostasis, triggering lipid peroxidation, and regulating the expression of ferroptosis-related genes (Han et al., 2022). Notably, API (2.7 × 10−3 μg/mL) significantly mitigated these adverse changes by modulating GPX4 activity and inhibiting intracellular iron accumulation (Han et al., 2022). These results suggest that API may serve as a potential detoxifying agent with protective effects against DEHP-induced liver injury.
3.2.4 Alcohol
Chronic or excessive alcohol consumption can significantly damage the liver, leading to the development of ALD (Mackowiak et al., 2024). Currently, alcohol-induced liver injury is one of the leading causes of liver diseases worldwide, with mechanisms involving steatosis, lipid metabolism disorders, oxidative stress, inflammatory responses, apoptosis and fibrosis (Lackner and Tiniakos, 2019; Chen M. et al., 2023). Interestingly, Wang et al. (2017), Wang et al. (2018) were the first to demonstrate, through both in vivo and in vitro experiments, the protective effects of API against alcohol-induced liver injury. On the one hand, API (150, 300 mg/kg) alleviated alcohol-induced liver damage by promoting the degradation of acetaldehyde, a toxic metabolite of alcohol, improving PPARα-mediated lipid metabolism pathways, and regulating CYP2E1-mediated oxidative stress in the liver (Wang et al., 2017). On the other hand, API (6, 12, and 24 µM) modulated the protein expression of NF-κB and IκB-α, reducing the production of inflammatory cytokines and thereby mitigating alcohol-induced inflammatory damage to hepatocytes (Wang et al., 2018). These findings indicate that API may prevent or reverse alcohol-induced liver injury by acting as a GR activator and CYP2E1 inhibitor, providing a theoretical foundation for the development of new drugs to treat ALD.
3.2.5 Lead acetate
Lead acetate (PbAc) is commonly used as a catalyst or reagent in organic synthesis but poses serious health and environmental hazards due to the toxicity of its lead ions (Lakka et al., 2023). Remarkably, in a rat model of PbAc-induced liver and kidney injury, API demonstrated significant protective effects (Fehaid et al., 2023). Specifically, API (20 mg/kg) inhibited oxidative reactions by upregulating the Nrf2/HO-1 signaling pathway and activating downstream antioxidant enzymes, while significantly reducing the production of pro-oxidants (Fehaid et al., 2023). Additionally, API lowered the expression of pro-inflammatory cytokines, effectively suppressing apoptosis in hepatocytes and renal cells induced by PbAc (Fehaid et al., 2023). Overall, due to its potent antioxidant, anti-inflammatory, and anti-apoptotic properties, API holds promise as a potential therapeutic agent to alleviate liver and kidney damage associated with lead exposure.
3.2.6 N-nitrosodiethylamine
N-nitrosodiethylamine (NDEA) is a nitrosamine compound commonly found in industrial waste, tobacco smoke, and certain contaminated foods and drinking water, and it exhibits high hepatotoxicity and carcinogenicity (Janmeda et al., 2024). NDEA severely damages liver structure and function through multiple mechanisms, including metabolic activation, oxidative stress, inflammatory responses, apoptosis, and necrosis, which may ultimately lead to liver fibrosis and even liver cancer (Cahyani et al., 2021). Remarkably, Ali et al. (2014) demonstrated that API could mitigate NDEA-induced hepatotoxicity in rats. Specifically, API (10, 20, 40 mg/kg) dose-dependently reduced serum levels of ALT, AST, ALP and LDH, as well as the levels of lipid peroxidation and protein carbonyl content (Ali et al., 2014). Furthermore, comet assays revealed that API treatment significantly reduced DNA damage in hepatocytes, blood lymphocytes and bone marrow cells (Ali et al., 2014). In conclusion, API is not only protective against NDEA-induced liver injury, but also exhibits anti-genotoxic potential.
3.3 Immune-mediated liver injury
Lipopolysaccharide (LPS), an endotoxin found in the outer membrane of gram-negative bacteria, is widely used to establish models of immune-mediated liver injury (Pfalzgraff and Weindl, 2019). LPS-induced liver injury primarily occurs through the activation of Kupffer cells, leading to the release of large amounts of inflammatory mediators such as IL-1β, IL-6, and TNF-α, thereby triggering immune-related liver damage (Rathinam et al., 2019). In a mouse model of LPS-induced liver injury, API (100, 200 mg/kg) exhibited significant hepatoprotective effects by inhibiting NF-κB and MAPK signaling cascades, enhancing both enzymatic and non-enzymatic antioxidant levels, and effectively reducing oxidative stress, neutrophil infiltration and inflammatory responses (Berköz et al., 2021). These findings suggest that API is a potential therapeutic agent for preventing liver injury caused by endotoxemia and sepsis.
D-Galactosamine (D-GalN) is an amino sugar compound that specifically interferes with RNA and protein synthesis in hepatocytes, leading to apoptosis and necrosis, as well as severe hepatitis and liver dysfunction (Maes et al., 2016). When combined with LPS, D-GalN significantly enhances the toxic response in hepatocytes, making this combination commonly used to establish animal models of immune-mediated liver injury for screening hepatoprotective drugs and exploring the mechanisms of liver injury (Kim et al., 2014; Farghali et al., 2016). Zhou et al. (2017), Zhou et al. (2020) confirmed, through both in vivo and in vitro experiments, the protective effects of API against D-GalN/LPS-induced liver injury. Mechanistically, API (100, 200 mg/kg) enhanced antioxidant capacity by increasing Nrf2-mediated antioxidant enzyme levels, including SOD, CAT, GST and GR (Zhou et al., 2017). Additionally, API (2.5, 5, 10, and 20 µM) suppressed the NF-κB signaling pathway by increasing the expression of PPARγ protein, thereby reducing the production of inflammatory cytokines (Zhou et al., 2020).
3.4 Ischemia-reperfusion liver injury
Ischemia-reperfusion (I/R) liver injury refers to significant liver tissue damage that occurs during the process of temporary interruption and subsequent restoration of blood supply to the liver (Wu et al., 2018). The initial ischemic phase leads to hypoxia and metabolic stress, which are further exacerbated by oxidative stress, inflammatory responses, and apoptosis during the reperfusion phase, thereby aggravating liver injury (Jiménez-Castro et al., 2019; Bardallo et al., 2022). Kupffer cells in the liver play a key role in mediating inflammatory responses, while neutrophil activation and ROS production exacerbate liver tissue damage (Jiménez-Castro et al., 2019). Unfortunately, I/R liver injury is a common clinical problem today, particularly during liver surgery and transplantation. Therefore, it is of great importance to find effective drugs to treat I/R liver injury.
Fortunately, Tsalkidou et al. (2014) and Tsaroucha et al. (2016) have confirmed that API exhibits significant protective effects in I/R liver injury. Specifically, API (15 mg/kg) reduced the activity of pro-apoptotic factors by modulating the expression of B-cell lymphoma-2 (Bcl-2) and Bcl-2-associated X (Bax) genes and decreased ICAM-1 levels, thereby reducing the release of inflammatory mediators and improving I/R -induced liver injury (Tsaroucha et al., 2016). Additionally, API (15 mg/kg) significantly reduced the expression levels of the Fas gene in hepatocytes during reperfusion (Tsalkidou et al., 2014). Notably, the Fas gene encodes the Fas receptor, a critical protein that initiates the apoptotic signaling pathway (Khan et al., 2017). This suggests that API may exert its protective effects against hepatic I/R injury by inhibiting the Fas/FasL-mediated apoptotic pathway (Tsalkidou et al., 2014).
3.5 Others
Nickel oxide nanoparticles (NiONPs) are transition metal oxide nanomaterials widely used in medical sensors, battery electrodes, catalysts and other fields (Berhe and Gebreslassie, 2023). Since NiONPs induce oxidative stress and reduce antioxidant capacity, leading to liver damage, it is imperative to find ways to protect individuals working in related industries from occupational exposure-induced liver damage (Saquib et al., 2018). Interestingly, Ali et al. (2021) confirmed, through hematological, biochemical, histopathological and metal analyses, the protective effects of API (25 mg/kg) against NiONPs-induced liver injury in rats, with specific mechanisms including the inhibition of oxidative stress and inflammatory responses. Moreover, API (0.625, 1.25, and 2.5 µM) also demonstrated protective effects against palmitic acid (PA)-induced damage in HepG2 cells and primary mouse hepatocytes (Meng et al., 2022). The beneficial effects of API may be attributed to its ability to alleviate PA-induced pyroptosis by inhibiting NLRP3 inflammasome activation through the activation of autophagy (Meng et al., 2022). Notably, this study reveals a novel link between autophagy and pyroptosis, providing new insights and targets for the use of functional factors in food to alleviate liver damage. The hepatoprotective effects and mechanisms of API in liver injury are presented in Table 2. The therapeutic role of API in liver injury is shown schematically in Figure 3.
4 The hepatoprotective effects and mechanisms of API in NAFLD/NASH
4.1 PPAR signaling pathway
PPARs are well-known for their critical role in the pathogenesis of NAFLD and NASH. Specifically, PPARα ameliorates hepatic steatosis by promoting fatty acid β-oxidation and reducing hepatic lipid accumulation (Khan et al., 2019). PPARγ plays a key role in adipocyte differentiation, lipid storage, and anti-inflammatory responses, and it reduces liver inflammation and fibrosis by modulating macrophage polarization (Chen H. et al., 2023). Additionally, PPARδ is significant in regulating insulin sensitivity and systemic lipid metabolism (Qiu et al., 2023). Therefore, therapeutic strategies targeting PPARs have shown potential efficacy and promising prospects in clinically improving NAFLD/NASH.
Interestingly, Shi et al. (2015) demonstrated that API alleviates hepatic steatosis and inflammatory necrosis in a high-fat diet (HFD)-induced NASH rat model by improving insulin resistance and glucose-lipid metabolism. The molecular mechanism involves the regulation of PPARα and PPARγ expression, suggesting that API might act as a dual agonist of PPARα/PPARγ (Shi et al., 2015). It combines the triglyceride-lowering effects of fibrates (PPARα agonists) with the insulin-sensitizing and lipid-regulating functions of thiazolidinediones (PPARγ agonists). Similarly, Hsu et al. (2021) found that API (20 µM) significantly increased the expression of proteins associated with the β-oxidation pathway, including PPARα, in oleic acid (OA)-treated Huh7 cells, thereby reducing intracellular lipid levels. Mechanistically, API may improve hepatic lipid accumulation by activating the autophagy-mitochondrial pathway (Hsu et al., 2021). These findings provide new insights into the potential of API in modulating dysregulated hepatic lipid metabolism.
Notably, PPARγ is also considered a major regulator of macrophage polarization (Wang et al., 2021b). Subsequent studies have shown that API (7.5 µM) significantly reverses M1 to M2 macrophage polarization in HFD and ob/ob mouse models, reducing inflammatory cell infiltration in the liver and lowering the secretion of pro-inflammatory cytokines, thereby alleviating obesity-associated inflammation (Feng et al., 2016). The underlying mechanism may involve the modulation of p65/PPARγ complex localization, altering macrophage functional polarization (Feng et al., 2016). Importantly, unlike the clinically used thiazolidinedione drug rosiglitazone, API does not cause side effects such as weight gain and osteoporosis (Feng et al., 2016). These results suggest that API may be a potential candidate for treating obesity-associated inflammation in the future.
4.2 Nrf2 signaling pathway
Oxidative stress is recognized as a key mechanism in the pathogenesis and progression of NAFLD/NASH (Chen et al., 2020). Numerous studies have shown that the Nrf2 signaling pathway plays a protective role in NAFLD (Xu et al., 2018; Bathish et al., 2022). Specifically, Nrf2 enhances cellular antioxidant defense by regulating the expression of a series of antioxidant enzymes, such as SOD, CAT and GST, thereby reducing the impact of oxidative damage on the liver (Xu et al., 2018). Additionally, Nrf2 can regulate inflammatory responses and lipid metabolism by inhibiting NF-κB activation and reducing the release of inflammatory mediators, thus lowering hepatic inflammation and lipid accumulation, ultimately helping to block the progression of NAFLD/NASH (Bathish et al., 2022). Therefore, activating the Nrf2 signaling pathway may represent a potential strategy for treating NAFLD/NASH.
Zhou (2016) and Feng et al. (2017) found that API (30 mg/kg) significantly inhibited lipid droplet formation in the liver, increased the expression of antioxidant genes, and suppressed the expression of lipogenic genes in an HFD-induced NAFLD mouse model. These effects were achieved by regulating the expression of downstream oxidative stress-related genes through the Nrf2 signaling pathway (Zhou, 2016; Feng et al., 2017). Further experiments demonstrated that API’s regulation of PPARγ target genes depended on Nrf2 activation, while Nrf2 activation offset the activation of PPARγ by API (Zhou, 2016; Feng et al., 2017). Moreover, Yang M. et al. (2018) showed that API (50 mg/kg) prevented multiple adverse metabolic effects induced by high fructose, including insulin resistance, dyslipidemia, and liver injury. The mechanism may involve the interaction between API and Keap1, blocking the binding of Keap1 to Nrf2, thereby increasing the transcriptional expression of Nrf2-targeted antioxidant genes (Yang M. et al., 2018). Collectively, these studies suggest that API, through the modulation of the Nrf2 signaling pathway, holds significant potential for mitigating NAFLD and improving metabolic syndrome.
4.3 NLRP3 signaling pathway
The NLRP3 inflammasome is a multiprotein complex whose activation triggers hepatic inflammatory responses, promoting hepatocyte injury and lipid accumulation (Fu and Wu, 2023). Specifically, upon activation, NLRP3 initiates downstream Caspase-1, leading to the release of pro-inflammatory cytokines such as IL-1β and IL-18 (Huang et al., 2021). The release of these cytokines not only exacerbates the inflammatory response in the liver, but also promotes hepatocyte injury and fibrosis, furthering the progression of NAFLD to NASH (de Carvalho Ribeiro and Szabo, 2022). Therefore, inhibiting the activity of the NLRP3 signaling pathway is considered a potential strategy for treating NAFLD/NASH.
Interestingly, Lv et al. (2019) reported that API (50 mg/kg) ameliorated hepatic lipid accumulation and inflammation, alleviating HFD-induced NAFLD. This beneficial effect may be attributed in part to the modulation of xanthine oxidase (XO) by API, which further inhibits the activation of NLRP3 inflammatory vesicles and the release of the inflammatory cytokines IL-1β and IL-18 (Lv et al., 2019). Similarly, Lu et al. (2023) confirmed through in vitro and in vivo experiments that API (5, 50 mg/kg) alleviated NAFLD by inhibiting the NLRP3 inflammasome. Furthermore, Meng et al. (2023) demonstrated that API (25, 50, and 100 mg/kg) reduced HFD-induced liver injury by activating mitophagy. Mechanistically, API may inhibit NLRP3 inflammasome activation by clearing ROS production, reducing lysosomal membrane permeability and cathepsin B (CTSB) release (Meng et al., 2023).
4.4 AMPK/SREBP signaling pathway
AMPK, as a cellular energy sensor, reduces hepatic lipid synthesis by inhibiting SREBP activation, thereby ameliorating NAFLD (Smith et al., 2016). Specifically, upon activation, AMPK inhibits the expression of fatty acid synthase (FAS) and acetyl-CoA carboxylase, enzymes that are induced by SREBP for lipid synthesis (Fang et al., 2022). Additionally, AMPK alleviates hepatic steatosis and inflammation by promoting fatty acid oxidation and reducing lipid accumulation (Fang et al., 2022). Therefore, the regulation of the AMPK/SREBP pathway is importance for the prevention and treatment of NAFLD.
In PA-induced HepG2 cells, API (10, 20, and 40 µM) significantly reduced total cholesterol (TC) and triglyceride (TG) levels (Lu et al., 2019). Moreover, API increased AMPK activity in a concentration-dependent manner while decreasing the expression of 3-hydroxy-3-methylglutaryl-CoA reductase, FAS and SREBP-1/2 (Lu et al., 2019). Mechanistically, API improves lipid metabolism by activating the AMPK/SREBP signaling pathway, thereby reducing excessive hepatic lipid accumulation (Lu et al., 2019).
4.5 JAK2-STAT3 signaling pathway
Studies have shown that excessive activation of the JAK2-STAT3 pathway leads to lipid accumulation and insulin resistance in hepatocytes, further exacerbating hepatic inflammation and steatosis (Chen B. et al., 2023). Conversely, inhibiting the activity of this signaling pathway can reduce hepatic inflammation and steatosis, thereby improving NAFLD symptoms (Huang et al., 2022). Therefore, the JAK2-STAT3 signaling pathway is considered a potential target for treating NAFLD, and regulating this pathway may effectively intervene and slow the disease’s progression.
Interestingly, Ma (Ma, 2019) explored the role and mechanism of API in anti-NAFLD through in vitro PA-induced LO2 cell models and in vivo HFD-induced NAFLD mouse models. The results of the cell experiments showed that API (25, 50, and 100 µM) improved lipid accumulation to varying degrees compared with the model group (Ma, 2019). The results of animal experiments further demonstrated that API (200 mg/kg) significantly ameliorated hepatic steatosis and reduced serum levels of TG, TC, GLU, LDL, AST, and ALT (Ma, 2019). Mechanistically, API may exert its anti-NAFLD effects by inhibiting the JAK2/STAT3 signaling pathway (Ma, 2019).
4.6 Others
Furthermore, in HFD-induced mice, API (0.005% w/w) reduced plasma levels of free fatty acids, total cholesterol, apolipoprotein B, and liver function markers, while ameliorating hepatic steatosis and liver hypertrophy (Jung et al., 2016). This beneficial effect is partly attributed to API’s regulation of hepatic metabolic and transcriptional responses, including upregulation of genes related to fatty acid oxidation, the tricarboxylic acid cycle, oxidative phosphorylation, the electron transport chain, and cholesterol homeostasis, as well as downregulation of genes related to lipolysis and lipogenesis (Jung et al., 2016). It is worth noting that Fetuin-A, a glycoprotein synthesized and secreted by hepatocytes into the bloodstream, can impede insulin signaling activation by binding to the extracellular region of the insulin receptor, leading to insulin resistance (Lanthier et al., 2022). Interestingly, Hsu et al. (2024) reported that API (20 mg/kg) improved hepatic insulin resistance by targeting Fetuin-A in HFD-induced mice. This new finding expands the biomedical significance of API in the prevention and treatment of HFD-induced metabolic disorders. The hepatoprotective effects and mechanisms of API in NAFLD/NASH are presented in Table 3.
5 The hepatoprotective effects and mechanisms of API in liver fibrosis
Liver fibrosis is a pathological process induced by chronic liver injury, characterized by excessive deposition of extracellular matrix (ECM) within the liver (Aydın and Akçalı, 2018). It is commonly caused by chronic liver diseases such as viral hepatitis, ALD, NAFLD, and cholestatic liver diseases (Parola and Pinzani, 2019). During this process, hepatic stellate cells (HSCs) are activated and produce large amounts of ECM components, leading to the gradual destruction of liver structure and function (Higashi et al., 2017). The progression of liver fibrosis can range from reversible to irreversible. In the early stages, fibrosis can be significantly reversed through the removal of the causative factors or therapeutic interventions, while in the late stages, it may progress to irreversible cirrhosis, ultimately leading to liver failure and HCC (Parola and Pinzani, 2019). Current treatment strategies include the removal of the causative factors and the development of antifibrotic drugs, with research focusing on inhibiting HSC activation, reducing ECM production, promoting ECM degradation, and modulating related cellular signaling pathways (Roehlen et al., 2020; Zhang D. et al., 2022).
Fortunately, Ji et al. (2021) confirmed the protective effects of API against liver fibrosis. API effectively alleviates CCl4-and bile duct ligation (BDL)-induced liver fibrosis by reducing liver enzyme levels, inhibiting ECM production, and regulating the balance between matrix metalloproteinase 2 (MMP2) and tissue inhibitors of metalloproteinase 1 (TIMP1) (Ji et al., 2021). Mechanistically, API may exert its antifibrotic effects by inhibiting the activation of HSCs and autophagy through the TGF-β1/Smad3 and p38/PPARα signaling pathways (Ji et al., 2021). Similarly, Melaibari et al. (2023) and Sun et al. (2024) also evaluated the antifibrotic efficacy of API in CCl4-induced liver fibrosis mouse models. On the one hand, API significantly mitigates oxidative stress, inflammation, and pathological angiogenesis by restoring GSH levels and CAT activity, reducing lipid peroxidation, inhibiting the expression of pro-inflammatory cytokines IL-1β, IL-6 and TNF-α, and decreasing the expression of pro-angiogenic factors VEGF and CD34 (Melaibari et al., 2023). On the other hand, API exerts its remarkable hepatoprotective effects by inhibiting HSC activation through pathways such as the regulation of the EGFR-MEK1/2-ERK1/2 signaling pathway, inhibition of the PKM2-HIF-1α pathway, and mediation of oxidative stress (Sun et al., 2024).
Cholestasis is a disease caused by disturbances in bile secretion and metabolism, leading to the accumulation of toxic bile acids that can cause damage to hepatocytes and the entire body (Méndez-Sánchez et al., 2023). If left untreated, cholestasis can gradually progress to liver fibrosis and even cirrhosis (Zeng et al., 2023). Notably, studies have shown that API has protective effects against DDC-induced cholestatic liver disease (Zheng et al., 2021). Mechanistically, API enhances the antioxidant capacity of hepatocytes by upregulating the activity of antioxidant enzymes, and alleviates DDC-induced liver injury and fibrosis in mice by inhibiting the TLR4/NF-κB/TNF-α inflammatory signaling pathway and upregulating FXR expression to regulate bile acid metabolism (Zheng et al., 2021). These findings suggest that API holds potential as a therapeutic agent for cholestatic liver diseases, such as primary biliary cholangitis and primary sclerosing cholangitis.
In recent years, the application of transcriptomics in liver fibrosis research has significantly advanced this field. Through comprehensive analysis of gene expression profiles in fibrotic liver tissues, scientists have been able to uncover key genes and signaling pathways involved in the fibrotic process (Zhang and Zhang, 2020). In 2017, Hicks et al. (2017) identified API as a potential antifibrotic agent by querying transcriptomic databases. Subsequent studies demonstrated that API (2.5, 10, and 40 µM) reduces collagen I expression in a dose-dependent manner in the human HSC line TWNT-4 (Hicks et al., 2017). Mechanistically, API may exert its antifibrotic effects by regulating C1QTNF2, a secretory adipocytokine with hepatic metabolic effects (Hicks et al., 2017). Additionally, in a CCl4-induced liver fibrosis rat model, API (150, 300, and 600 mg/kg) significantly reduced various biochemical parameters and alleviated liver fibrosis and inflammation (Qiao et al., 2020). Further transcriptomic and proteomic analyses revealed that the protective effects of API on liver fibrosis in rats might be achieved through VEGF-mediated FAK phosphorylation and multiple signaling pathways, including MAPKs, PI3K/Akt, HIF-1, ROS and eNOS (Qiao et al., 2020).
Furthermore, Duan et al. (2024) established a transcription-based drug screening system and identified API from 283 natural compounds due to its ability to reverse gene expression patterns associated with PANoptosis and type I interferon (IFN-I) signaling. Subsequently, in BDL, Abcb4−/− and DDC-fed mouse models, API (25, 50 mg/kg) effectively alleviated liver injury, inflammation, and fibrosis, protected cholangiocytes from bile acid-induced PANoptosis, and inhibited IFN-I-induced inflammatory responses (Duan et al., 2024). Notably, this study not only demonstrated the pathogenic role of PANoptosis and IFN-I signaling in cholestatic liver fibrosis but also revealed a novel anti-inflammatory mechanism of API, making it a promising candidate for the treatment of cholestatic liver fibrosis (Duan et al., 2024). The hepatoprotective effects and mechanisms of API in liver fibrosis are presented in Table 4.
6 The hepatoprotective effects and mechanisms of API in liver cancer
6.1 Monotherapy
6.1.1 Inhibition of proliferation of HCC cells
In the treatment of HCC, inhibiting the proliferation of cancer cells is a crucial step. Numerous studies have demonstrated that API effectively inhibits the proliferation of various HCC cell lines (Chiang et al., 2006; Cui et al., 2018). For instance, API significantly suppresses the proliferation of HepG2 cells, Huh-7 cells, MHCC97 cells, MHCC97 cell-derived second-generation sphere cells, Hep3B cells, and PLC/PRF/5 cells, with IC50 values of 11.0 μg/mL, 12.0 μg/mL, 43.2 ± 2.3 μM, 18.4 ± 1.6 μM, 22.16 ± 0.67 μg/mL, and 22.55 ± 1.42 μg/mL, respectively (Chiang et al., 2006; Bhattacharya et al., 2018; Cui et al., 2018). Similarly, Chen et al. (2015) and Li et al. (2017) confirmed that API significantly inhibits the proliferation of HepG2 cells in a time- and concentration-dependent manner.
Notably, API has the potential to inhibit HCC cell proliferation by regulating the cell cycle. Wang (Wang, 2020) revealed that API (5, 10, and 20 mg/L) effectively arrests the cell cycle of Huh-7 cells at the G2/M phase, and this arresting effect is significantly enhanced with increasing concentrations of API. Further microRNA (miRNA) transcriptome sequencing analysis confirmed that API may mediate the cell cycle arrest of Huh-7 cells by regulating the differential expression of miRNAs (Wang, 2020). MED28, a subunit of the transcriptional activator, has been shown to play a key role in the occurrence and development of various malignancies (Cho et al., 2019). Interestingly, Chou et al. (2024) demonstrated that API could regulate the MED28/mTOR signaling pathway, leading to cell cycle arrest, affecting the nuclear translocation of SREBP-1, reducing lipid accumulation and ultimately inhibiting the development of HCC.
Research has shown that the high expression of KDM1A promotes the proliferation and invasion of HCC cells and is associated with tumor aggressiveness and poor prognosis (Ismail et al., 2018). Therefore, KDM1A is considered a potential target in HCC treatment. Zhang (2020) indicated that API (50, 100 µM) can inhibit the proliferation and growth of HCC cells by targeting KDM1A to regulate lipid metabolism. Moreover, the abnormal overexpression of long non-coding RNA H19 is closely related to the occurrence and progression of HCC (Zhang et al., 2019). Interestingly, Pan et al. (2021) revealed that API (50 mg/kg) can downregulate H19 and reduce the expression of β-catenin, leading to the inactivation of the Wnt/β-catenin signaling pathway, thereby inhibiting tumor growth. This study not only provides a new mechanism of tumor suppression mediated by API but also suggests that API may be a promising candidate drug for cancer patients.
6.1.2 Induction of apoptosis of HCC cells
Apoptosis, a form of programmed cell death, plays a critical role in maintaining tissue health and stability by eliminating abnormal or damaged cells (Singh and Lim, 2022). In the treatment of HCC, inducing apoptosis of tumor cells is a major strategy, as it effectively reduces the number of cancer cells, inhibiting tumor growth and metastasis (Anwanwan et al., 2020). It is well known that Bcl-2, as a member of the apoptosis protein family, plays a role in inhibiting apoptosis, while Bax suppresses tumor growth by promoting apoptosis (Hafezi and Rahmani, 2021). Interestingly, Wang (Wang, 2020) demonstrated that API (50 μg/d) significantly reduces the expression of Bcl-2 while increasing the expression of Bax, thereby inducing apoptosis. Additionally, Khan and Sultana (2006) showed that API (25, 50 µM) can induce apoptosis in HepG2 cells by activating the Caspase pathway and promoting the production of TNF-α and IFN-γ. Similarly, Seydi et al. (2016) also confirmed that API (10, 20, and 40 µM) selectively induces apoptosis in HCC cells and inhibits tumor growth by directly targeting mitochondria.
API can also induce apoptosis of HCC cells by regulating multiple signaling pathways. The p53-WAF/p21 pathway is an important cell signaling pathway that mainly regulates the cell cycle and maintains genomic stability (Engeland, 2022). Interestingly, Chiang et al. (2006) revealed that API (4, 8, and 16 mg/mL) induces apoptosis in HepG2 cells, significantly increases p53 accumulation, and elevates p21/WAF1 levels. Mechanistically, API-induced apoptosis in HCC cells may be mediated through the p53-WAF/p21 pathway (Chiang et al., 2006). It is worth mentioning that API’s growth-inhibitory effect on HepG2 cells is comparable to the efficacy of the clinically used anti-HCC drug 5-fluorouracil.
Loss or dysfunction of PTEN leads to sustained activation of the downstream Akt signaling pathway, which plays a crucial role in the occurrence and development of HCC (Papa and Pandolfi, 2019). Wu et al. (2009) found that the apoptosis-inducing effect of API (20, 40, and 80 µM) in HepG2 cells is associated with the upregulation of PTEN protein expression and the reduction of phosphorylated Akt and phosphorylated Bad protein levels. Subsequently, Yang J. et al. (2018) also confirmed that API (10, 20, and 40 µM) induces apoptosis and autophagy in HCC cells by inhibiting the PI3K/Akt/mTOR signaling pathway. More importantly, inhibiting autophagy significantly enhanced the apoptotic effects of API in HepG2 cells and xenograft models, increasing its anticancer efficacy (Yang J. et al., 2018). This suggests that the combined use of API and autophagy inhibitors may represent a new strategy for treating HCC.
6.1.3 Inhibition of self-renewal of HCC stem cells
Studies have shown that the initiation, progression, local recurrence, distant metastasis, and failure of radiotherapy and chemotherapy in HCC are primarily driven by a small subset of tumor cells with stem cell-like properties, known as cancer stem cells (Lee et al., 2022). Therefore, the cancer stem cell hypothesis has spurred research and development of therapeutic strategies targeting HCC stem cells. Inhibiting the self-renewal ability of HCC stem cells can significantly reduce tumor proliferation and metastasis, thereby improving treatment outcomes (Liu et al., 2020). Fortunately, Jiang et al. (2017) revealed that API (5, 10, and 20 µM) can reduce the tumor sphere formation rate of HCC stem-like cells in a concentration-dependent manner. This inhibition of HCC stem-like cell self-renewal is attributed to the downregulation of CK2α expression by API (Jiang et al., 2017). Similarly, API (10, 20, 40 mg/L) can also reduce the sphere formation rate of MHCC97 cells in a concentration-dependent manner (Jiang et al., 2017). The mechanism is related to the upregulation of Src homology 2 domain-containing protein tyrosine phosphatase 1 (SHP-1) protein expression by API, which in turn reduces the phosphorylation level of STAT3 protein (Jiang et al., 2017). These findings provide strong evidence for API as a potential therapeutic approach targeting the inhibition of self-renewal in HCC stem cells.
6.1.4 Induction of differentiation of HCC cells
HCC cells are typically in an undifferentiated state, characterized by high proliferative capacity and invasiveness, which are major contributors to HCC progression and treatment resistance (Vogel et al., 2022). By inducing differentiation in these cells, their proliferative and invasive capacities can be weakened, and their sensitivity to conventional therapies increased, thereby inhibiting tumor growth and spread (Marquardt et al., 2015). Wen et al. (2007) demonstrated that API (10 µM) can induce differentiation of HepG2 cells from a tumorigenic to a more mature state, with notable changes in morphology and cytoskeletal structure, as well as a significant reduction in γ-GT activity and AFP secretion. It is worth noting that γ-GT is a marker enzyme for HCC, while AFP is a marker of HCC cell differentiation. Similarly, API (5 μm/L) can also induce differentiation in SMMC-7721 cells (Wang, 2018). Additionally, API can enhance the process of differentiation induced by the microenvironment of mouse embryonic liver cells at specific stages, with the synergistic effect peaking at 48 h (Wang, 2018). In summary, these studies suggest that API could serve as a lead compound with differentiation-inducing properties, and its chemical structure may be strategically modified to develop effective differentiation-inducing agents for HCC treatment.
6.1.5 Inhibition of angiogenesis of HCC cells
HCC is a highly vascularized tumor, with its growth and metastasis relying heavily on the process of angiogenesis, or the formation of new blood vessels (Morse et al., 2019). Inhibiting angiogenesis effectively cuts off the tumor’s nutrient supply, restricting its growth and spread, thereby improving patient survival and quality of life (Hu et al., 2022). Typically, the inhibition of angiogenesis is achieved by targeting key molecules such as VEGF and its receptors (Ghalehbandi et al., 2023). Interestingly, Kim et al. (2011) demonstrated that API (40, 80, and 120 µM) induces apoptosis in HCC cells and inhibits cell migration by reducing the expression of stromal proteins and type I collagen. More importantly, API exhibited significant anti-angiogenic effects (Kim et al., 2011). Further studies revealed that the anti-angiogenic activity of API is primarily dependent on the reduction of cell migration activity and the downregulation of VEGF and MMP8 release (Kim et al., 2011). In summary, stromal proteins may play a crucial role in API-induced apoptosis in HCC cells by mediating anti-angiogenic and anti-migratory activities.
Recent studies have indicated that extracellular vesicles (EVs) play a pivotal role in mediating intercellular communication within the tumor microenvironment, greatly influencing the progression of various tumors, including HCC (Kalluri and McAndrews, 2023). Therefore, the comprehensive inhibition of tumor-derived EVs’ production and release could emerge as a more effective strategy to halt tumor progression. Notably, Zhang et al. (2024) revealed that API can inhibit tumor angiogenesis by targeting ARHGEF1, thereby reducing the release of microvesicles and attenuating microvesicle-mediated angiogenesis, ultimately preventing the progression of HCC. This finding not only provides new insights into the anti-tumor mechanisms of API, but also opens up new avenues for curbing HCC progression by targeting and inhibiting the production and function of EVs.
6.1.6 Inhibition of invasion and migration of HCC cells
In anti-HCC therapy, inhibiting the invasion and migration of HCC cells is one of the key strategies (Clark and Vignjevic, 2015). By interfering with the motility and invasive properties of HCC cells, it is possible to effectively block their spread and metastasis. This not only helps in controlling the local spread of HCC but also prevents its metastasis to other organs, thereby improving the quality of life of patients. Qin et al. (2016) demonstrated that API (10, 20 µM) significantly inhibited the proliferation, migration, and invasion of PLC and Bel-7402 cells. Specifically, API reversed the increased levels of epithelial-mesenchymal transition (EMT) markers, enhanced cell adhesion, and regulated actin polymerization and cell migration (Qin et al., 2016). These effects are likely attributable to its inhibition of the NF-κB/Snail signaling pathway (Qin et al., 2016).
Additionally, API (5, 10, and 20 mg/L) was shown to inhibit the invasion of Huh-7 cells in a dose-dependent manner (Wang, 2020). Further transcriptomic sequencing results suggest that API may suppress cell invasion by modulating the differential expression of miRNAs (Wang, 2020). Zhou (Zhou, 2021) further elucidated the mechanisms by which API regulates HCC cell migration and invasion. The data indicate that API inhibits HCC cell migration and invasion, at least in part, through the downregulation of YAP, a downstream effector molecule of the Hippo pathway (Wang, 2020). These findings suggest that API may be an effective alternative therapy for treating refractory cancers and holds promise as an anti-metastatic candidate drug. The hepatoprotective effects and mechanisms of API in liver cancer (monotherapy) are presented in Table 5.
6.2 Combination therapy
6.2.1 With sorafenib
Sorafenib (C21H16ClF3N4O3, SOR) is a multi-targeted oral anticancer drug widely used in the treatment of advanced HCC, advanced renal cell carcinoma, and differentiated thyroid carcinoma (Escudier et al., 2019). It exerts its antitumor effects by inhibiting various intracellular and extracellular kinases, thereby interfering with tumor cell proliferation and angiogenesis, which inhibits tumor growth and metastasis (Xia et al., 2020). Despite its remarkable efficacy in cancer treatment, SOR is associated with adverse effects that cannot be ignored, including rash, hand-foot syndrome, hypertension, and diarrhea (Gauthier and Ho, 2013; Keating, 2017). Consequently, researchers are exploring combination therapy strategies to overcome SOR resistance and reduce its adverse effects.
Interestingly, Şirin et al. (2020) were the first to investigate the effect of combining SOR with API on HepG2 cells. The data demonstrated that combination therapy significantly decreased cell viability, increased apoptosis, and inhibited cell migration and invasion (Şirin et al., 2020). These findings suggest that API accelerates and enhances the anticancer effects of SOR while exhibiting lower toxicity to healthy cells, potentially helping to overcome SOR resistance (Şirin et al., 2020). However, further research is needed to determine the optimal dosage and method of this combination therapy. Similarly, Singh et al. (2024) also demonstrated that the combination of SOR and API was more effective than SOR alone, exhibiting stronger cytotoxicity, apoptosis induction, tumor cell cycle arrest, and tumor growth inhibition. Overall, API emerges as a promising adjuvant, and further studies will help to deepen our understanding of its mechanism of action.
6.2.2 With doxorubicin
Doxorubicin (C27H29NO11, DOX) is an anthracycline antibiotic widely used to treat various cancers, including breast cancer, ovarian cancer, bladder cancer, lung cancer, and lymphomas (Kciuk et al., 2023). Its mechanism of action involves intercalating into the DNA double helix, interfering with DNA and RNA synthesis, thereby inhibiting cell proliferation and inducing apoptosis (van der Zanden et al., 2021). Despite its significant antitumor effects in clinical applications, DOX use is associated with severe side effects, particularly cardiotoxicity (Sheibani et al., 2022). Therefore, researchers have been striving to enhance the therapeutic efficacy of DOX while reducing its side effects.
Studies have shown that API can enhance the sensitivity of DOX-resistant BEL-7402 (BEL-7402/ADM) cells to DOX (Gao et al., 2013). Specifically, in a BEL-7402 xenograft model, the combination of API and DOX significantly inhibited tumor growth, reduced cell proliferation, and induced apoptosis compared to DOX alone (Gao et al., 2013). This beneficial effect may be attributed to the inhibition of the PI3K/Akt/Nrf2 signaling pathway, thereby reversing the resistant phenotype (Gao et al., 2013). Four years later, the research team further discovered that API could sensitize BEL-7402/ADM cells to DOX by modulating the miR-520b/ATG7-related autophagy pathway in HCC (Gao et al., 2018). These findings not only help to understand the potential molecular mechanisms of chemotherapy resistance but also provide new therapeutic strategies to overcome drug resistance in liver cancer.
6.2.3 With paclitaxel
Paclitaxel (C47H51NO14, PTX) is a widely used clinical anticancer drug primarily used to treat various solid tumors, including breast cancer, ovarian cancer, non-small cell lung cancer, and pancreatic cancer (Liu et al., 2023). Its primary mechanism of action involves promoting the polymerization of tubulin, stabilizing microtubule structures, thereby preventing the normal depolymerization of microtubules during cell division, leading to cell cycle arrest at mitosis, and ultimately inducing apoptosis (Zhu and Chen, 2019). However, PTX resistance remains a significant challenge in treatment. Interestingly, Li et al. (2020) revealed that API significantly reduced hypoxia-induced resistance to PTX in HepG2 cells and tumor-bearing mouse models. Mechanistically, API enhanced the anticancer activity of PTX in hypoxic tumors by inhibiting the Akt/p-Akt pathway and HSP90 expression, thereby reducing HIF-1α expression (Li et al., 2020). These results not only confirm the synergistic effect of PTX and API but also highlight the potential of using API in combination with other anticancer drugs as adjuvant therapy.
6.2.4 With cisplatin
Cisplatin (PtCl2(NH3)2, CDDP) is a platinum-based anticancer drug widely used in clinical practice to treat various solid tumors, including testicular cancer, ovarian cancer, bladder cancer, head and neck cancers, and lung cancer (Ghosh, 2019). The mechanism of action is to induce apoptosis and programmed cell death through the formation of CDDP-DNA adducts, leading to intra- and interstrand cross-linking of DNA and interference with DNA replication and transcription processes (Rocha et al., 2018). Despite its broad-spectrum antitumor activity, CDDP use is also associated with side effects, including nephrotoxicity, ototoxicity, and neurotoxicity (McSweeney et al., 2021). Moreover, CDDP resistance is an issue that needs to be addressed. Studies have shown that API can enhance the chemotherapeutic effects of CDDP in liver cancer cells, including HepG2, Hep3B, and Huh7 cells (Papachristou et al., 2021). Specifically, API enhanced the genotoxicity, cytotoxicity, anti-invasive, and anti-migratory effects of CDDP in HCC cell lines (Papachristou et al., 2021). These findings provide direction for future exploration of the precise mechanisms of action when using CDDP and API in combination.
6.2.5 With 5-fluorouracil
5-Fluorouracil (C4H3FN2O2, 5-FU) is a chemotherapeutic drug widely used in the treatment of various malignancies, including colorectal cancer, gastric cancer, pancreatic cancer, and breast cancer (Hu et al., 2023). Its mechanism of action involves inhibiting the key enzyme in the pyrimidine metabolic pathway—thymidylate synthase—thereby interfering with DNA and RNA synthesis, leading to the inhibition of cancer cell proliferation and growth (Sethy and Kundu, 2021). However, 5-FU is also associated with certain side effects, including bone marrow suppression, gastrointestinal reactions, and mucositis (Wei et al., 2018). Therefore, it is often used in combination with other chemotherapeutic drugs (such as oxaliplatin, irinotecan) or targeted therapies (such as bevacizumab, ramucirumab) to enhance therapeutic efficacy and reduce resistance.
Research indicates that the combination of API with 5-FU may be an effective chemotherapy strategy for HCC (Hu et al., 2015). Specifically, the combination treatment significantly increased reactive ROS levels in liver cancer cells, leading to a decrease in mitochondrial membrane potential and activation of the mitochondrial apoptosis pathway (Hu et al., 2015). Mechanistically, API may enhance the chemosensitivity of liver cancer cells to 5-FU by activating the intrinsic apoptosis pathway mediated by mitochondrial membrane potential (Hu et al., 2015). This discovery provides a new avenue for optimizing chemotherapy regimens for HCC and overcoming chemotherapy resistance.
6.2.6 With chrysin
Chrysin (C15H10O4, CHR) is a naturally occurring flavonoid compound found in propolis, bee pollen, and various plants, garnering attention for its multiple biological activities, including antioxidant, anti-inflammatory, anticancer, and neuroprotective effects (Mani and Natesan, 2018). Of particular importance is CHR’s anticancer activity, which involves mechanisms such as inducing apoptosis, inhibiting proliferation, and preventing invasion and metastasis of cancer cells (Salari et al., 2022). Interestingly, Huang et al. (2016) demonstrated that the combination of API and CHR significantly reduced the viability of HepG2 and MDA-MB-231 cells, induced apoptosis, and reduced the migratory capacity of MDA-MB-231 cells. Moreover, combination therapy effectively inhibited the growth of MDA-MB-231 xenograft tumors (Huang et al., 2016). These results indicate that the combination of CHR and API has potential anticancer effects, providing a solid foundation for the research of new drug targets. The hepatoprotective effects and mechanisms of API in liver cancer (combination therapy) are presented in Table 6. The schematic diagram of API improvement of liver cancer is shown in Figure 4.
7 Toxicity of API
Most studies have reported that API exhibits no significant toxicity to cells or experimental animals. For instance, Seydi et al. (2016) demonstrated that API exhibited no cytotoxicity to normal liver cells at concentrations ranging from 0 to 50 μM and showed only minimal cytotoxicity at 100 μM. Similarly, Vrhovac Madunić et al. (2018) found that API exhibited no apparent toxicity to human peripheral blood lymphocytes (HPBLs) at concentrations ranging from 0 to 100 μM, and regardless of concentration and exposure time, did not significantly increase the number of DNA strand breaks. It is noteworthy that Wang (Wang, 2022) explored the impact of API-casein nanoparticles on organ coefficients and liver toxicity in rats. The results showed that oral administration of API-casein nanoparticles (equivalent to API 100–1,000 mg/kg) for 2 weeks had no significant effect on the organ coefficients of the heart, liver, spleen, lungs, and kidneys, nor on the hepatic histological structure in rats, indicating good biosafety (Wang, 2022). Overall, these studies suggest that API exhibits extremely low toxicity and has a high degree of clinical safety, with broad prospects for development and application. However, systematic toxicological studies are still needed in the future to comprehensively assess the toxicity and mechanisms of API.
8 Pharmacokinetic properties of API
It is surprising that approximately 40% of failures in drug development can be attributed to inappropriate pharmacokinetic properties (Walker, 2004). Therefore, determining the pharmacokinetic characteristics of a drug in the early stages of development is crucial. Pharmacokinetic analysis allows for the prediction of individual differences in drug behavior, optimization of drug efficacy and safety, and reduction of adverse reactions (van den Anker et al., 2018). Additionally, pharmacokinetic data provide a scientific basis for drug interactions and dosing in special populations, thereby improving the effectiveness and safety of drug therapy (Koziolek et al., 2019). Currently, the pharmacokinetics of API are mainly studied using high-performance liquid chromatography-tandem mass spectrometry (HPLC-MS/MS) and ultra-performance liquid chromatography-tandem mass spectrometry (UPLC-MS/MS) to analyze plasma samples from rats and mice. Comparative pharmacokinetic parameters of API from seven studies are summarized in Table 7.
Ganguly et al. (2021) indicates that API exhibits slow distribution and elimination rates in rats. Following intravenous administration (2.49 mg/kg), the T1/2, Cmax, AUC(0-t), and CL of API were 26.60 ± 1.20 h, 0.08 ± 0.00 μg/mL, 1.41 ± 0.05 μg/mL·h, and 1.33 ± 0.03 L/h/kg, respectively (Ganguly et al., 2021). Additionally, another study reported that the terminal phase distribution volume (Vd) of API after intravenous administration (20 mg/kg) was 15.75 ± 11.73 L/kg, exceeding the total body water volume in rats, suggesting that API was well-distributed into tissues (Wan et al., 2007). Meanwhile, the total body clearance (CL) of API following intravenous administration (20 mg/kg) was 6.12 ± 0.79 L/h/kg, significantly higher than the hepatic blood flow rate in rats, indicating that API is cleared not only through hepatic metabolism but also via renal or other excretory pathways (Wan et al., 2007).
Currently, the elimination pathways of API have not been systematically explored. Existing studies suggest that the half-life (T1/2) of API remains uncertain. For oral doses ranging from 10 to 60 mg/kg, the T1/2 ranges from 2.11 to 91.8 h (Gradolatto et al., 2005; Teng et al., 2012; Ding et al., 2014). For injection doses of 2–20 mg/kg, the T1/2 ranges from 1.75 to 26.6 h (Wan et al., 2007; Ganguly et al., 2021; Dhara et al., 2023). Interestingly, another study also pointed out that API has a slow absorption and metabolism process in rats, primarily excreted through urine (Gradolatto et al., 2005). Importantly, a gender difference in the metabolic rate of API was observed, with female rats exhibiting a slightly higher metabolic rate than male rats, especially in the formation of glucuronide conjugates (Gradolatto et al., 2005). Therefore, future research should delve into the metabolic differences of API across different genders and physiological states to formulate personalized dosing regimens in clinical applications.
Due to the limitations in the absorption and bioavailability of API, some researchers have focused on enhancing its oral bioavailability through experimental approaches. For instance, Ding et al. (2014) developed a novel nanocarbon drug carrier, which significantly improved the dissolution rate and oral bioavailability of API. Specifically, the Cmax and AUC(0-t) values of API-SD were 2.45-fold and 1.83-fold higher than those of API, respectively, with a relative oral bioavailability increase of 183% (Ding et al., 2014). Similarly, Ganguly et al. (2021) developed galactose-modified PLGA nanoparticles (API-GAL-NPs) loaded with API for active liver-targeted treatment of HCC. Notably, the AUC(0-t), T1/2, and MRT(0-t) values of API-GAL-NPs were 2.12-fold, 3.47-fold, and 3.84-fold higher than those of API, respectively, indicating that API-GAL-NPs could maintain stable and significantly higher levels of API in the blood, offering superior anticancer effects compared to API alone (Ganguly et al., 2021). In conclusion, future studies should further explore the pharmacokinetic properties of API in various animal models and evaluate its long-term safety and potential toxicity.
9 New formulations of API
Despite the significant bioactivity and numerous health benefits of API, its clinical application is hindered by factors such as low bioavailability, poor water solubility, and drug interactions. Therefore, improving drug design and formulation technologies to overcome these limitations is of paramount importance. Interestingly, the development of novel drug delivery systems offers an effective approach to address these challenges associated with API.
9.1 Nanoparticles
9.1.1 Polymer nanoparticles
Polymeric nanoparticles (PNPs) are composed of natural or synthetic polymers and are widely used in drug delivery, gene therapy, and tissue engineering (Zielińska et al., 2020). Notably, polymeric nanoparticles can achieve controlled drug release in vivo by modulating parameters such as the composition, size, and surface charge of the nanoparticles (Elmowafy et al., 2023). Remarkably, polymeric micelles loaded with API, prepared by Zhai et al. (2013), effectively improved the solubility of API in water. In vitro drug release studies showed that nearly 84% of API was released from the micelles within 36 h, exhibiting significant sustained-release characteristics (Zhai et al., 2013). Further experiments demonstrated that polymeric micelles loaded with API exhibited significantly higher toxicity to HepG2 and MCF-7 cells compared to free API (Zhai et al., 2013). Subsequently, Das et al. (2013) developed API-loaded PLGA nanoparticles and found that they enhanced the anticancer effects in mice with skin tumors and mitochondrial dysfunction induced by Benzo [a]pyrene and UV-B radiation.
In recent years, numerous studies have demonstrated that API-loaded nanoparticles have significant anti-HCC effects. For instance, Bhattacharya et al. (2018) showed that API-loaded nanoparticles significantly increased the concentration of API in the blood and liver, substantially controlling the progression of HCC. This beneficial effect may be attributed to the enhanced permeability and retention (EPR) effect of the API-loaded nanoparticles in solid tumors (Bhattacharya et al., 2018). Additionally, Ganguly et al. (2021) confirmed that API-loaded galactose-modified PLGA nanoparticles (API-GAL-NPs) exhibited more pronounced therapeutic effects in rats with diethylnitrosamine (DEN)-induced liver cancer. This superior efficacy is likely achieved through the active targeting and improved internalization of API by API-GAL-NPs in liver cancer cells (Ganguly et al., 2021).
Interestingly, Dhara et al. (2023) developed a tumor-responsive phosphorothioated and amino-modified aptamer (AS1411)-conjugated stealth nanoliposome for encapsulating API, successfully achieving targeted drug distribution of API in the tumor. More importantly, this aptamer-modified nanoliposome (Apt-NLCs) significantly reduced the incidence of tumors and tumor-associated hepatic degenerative lesions, further validating its potential in the treatment of HCC (Dhara et al., 2023). These research findings indicate that the application of nanotechnology has significantly improved the drug delivery and therapeutic efficacy of API, providing a solid foundation for its clinical application.
9.1.2 Metal nanoparticles
Metal nanoparticles (MNPs) have shown great potential in areas such as biosensing, drug delivery, and cancer therapy due to their high surface area and unique optical properties (Sánchez-López et al., 2020). Among them, silver nanoparticles have gained attention for their powerful antibacterial properties, capable of exerting broad-spectrum antibacterial effects by releasing silver ions that disrupt bacterial cell membranes and DNA structures (Durán et al., 2016). One study demonstrated that silver nanoparticles synthesized with API (AP-SNPs) exhibited enhanced anticancer, antibacterial, and antioxidant activities (Zarei et al., 2021). Specifically, AP-SNPs improved liver function by modulating liver enzymes, lipid peroxidation, and increasing the expression of antioxidant enzymes (Zarei et al., 2021). Although AP-SNPs have shown great potential, further research and evaluation are needed to assess their potential biotoxicity and environmental impact to ensure their safe and effective clinical application.
9.1.3 Carbon-based nanoparticles
Carbon-based nanoparticles (CBNPs) hold an important position in nanotechnology and biomedicine, with major types including carbon nanotubes, graphene, fullerenes, and carbon nanopowder (CNP) (Patel et al., 2019). Carbon nanopowder has become a research hotspot as a drug carrier due to its high surface area, chemical stability, and biocompatibility. Its unique surface properties enable it to load various drugs through physical adsorption or chemical bonding, thereby enhancing drug solubility and bioavailability (Johnson et al., 2022). Ding et al. (2014) demonstrated that carbon nanopowder solid dispersions significantly increased the bioavailability of API by up to 183%. This effect was partly attributed to the increased dissolution and absorption rates in rats (Ding et al., 2014).
9.1.4 Other nanoparticles
Li (2008), Wang (2012); Wang (2022), and Huang et al. (2019b), among others, successfully prepared different types of API nanocarriers using various methods such as hot-melt ultrasonic dispersion, phase inversion, acid-base, and physical adsorption. These nanocarriers included API solid lipid nanoparticles, API liposome nanocapsules, API-casein nanoparticles, and API-loaded mesoporous silica nanoparticle solid dispersions (AP-MSN). These novel nanocarriers significantly improved the oral absorption and bioavailability of API, particularly the API-casein nanoparticles, which significantly enhanced the solubility of API in simulated gastric and intestinal fluids by 422.96-fold and 108.20-fold, respectively, compared to pure API (Wang, 2022). As the main natural protein in milk, casein has good biocompatibility and safety. Therefore, using it as a carrier material to construct an oral delivery system for API not only enhances the stability of API but also promotes its absorption and utilization in the body.
9.2 Nanosuspensions
Nanosuspensions are stable dispersion systems formed by dispersing drug microparticles or nanoparticles in a liquid medium, significantly improving the solubility, dissolution rate, and bioavailability of poorly soluble drugs (Chavhan, 2024). API nanosuspensions, prepared by Yu (2015) using a micro-precipitation-ultrasonic method, exhibited a drug loading of 44.4% ± 0.06% and achieved a release rate of 80% within 72 h in 20% EtOH/PBS. More importantly, API nanosuspensions increased the relative bioavailability of API by enhancing the Cmax, AUC(0-t), and MRT(0-t) parameters (Yu, 2015). Subsequently, Lv (2016) further demonstrated that API nanosuspensions improved the distribution of API in mice, increasing liver uptake and favoring drug targeting to liver tissue. Moreover, compared to API, the nanosuspensions significantly enhanced the toxicity to HepG2 cells, increased the apoptosis rate of HepG2 cells, strengthened G2/M phase arrest, and improved the inhibition rate of liver tumors in nude mice (Lv, 2016). In summary, API nanosuspensions significantly enhance the solubility and bioavailability of the drug, thereby improving its pharmacological activity, and showcasing broad application prospects and clinical translation value.
9.3 Others
Phospholipid phytosomes are complexes formed by combining active plant ingredients with phospholipids, widely used in drug delivery and nutritional supplementation (Gupta et al., 2022). Phospholipid phytosomes significantly improve the solubility, bioavailability, and stability of plant extracts by leveraging the hydrophilic and lipophilic properties of phospholipids (Gnananath et al., 2017). Studies have shown that API-phospholipid phytosomes (APLC) significantly improved the water solubility, dissolution, oral bioavailability, and in vivo antioxidant activity of API compared to pure API (Telange et al., 2017). This suggests that phospholipid phytosomes are a potential strategy to improve the delivery of API and other similar poorly water-soluble plant components. Additionally, magnesium complexes as drug carriers can also enhance drug stability and bioavailability. Research has shown that API-magnesium complexes can reduce oxidative stress and inflammatory responses in H2O2-treated HSCs, suggesting their potential as a promising hepatoprotective agent (Pan et al., 2020).
In summary, these advanced drug delivery systems effectively overcome the limitations of API in terms of bioavailability, water solubility, and drug interactions, significantly enhancing its efficacy and safety in clinical applications. Future research should continue to focus on optimizing these technologies, exploring new carrier materials and delivery strategies to further enhance the clinical application potential of API. The compositions and achieved improvements of the new dosage form of API are presented in Table 8.
10 Discussion and future perspective
As a naturally occurring flavonoid, API has garnered extensive academic interest in recent years due to its exceptional hepatoprotective properties. A substantial body of research evidence suggests that API holds significant potential in the prevention and treatment of various liver diseases, including liver injury caused by multiple factors, NAFLD/NASH, liver fibrosis, and HCC. The hepatoprotective effects of API are mediated through multiple molecular mechanisms, including the inhibition of inflammation, alleviation of hepatic oxidative stress, improvement of insulin resistance, promotion of fatty acid oxidation, reversal of macrophage polarization, regulation of bile acid metabolism, and the inhibition of HCC cell proliferation, differentiation, and induction of apoptosis. Importantly, signaling pathways such as Nrf2/HO-1, NF-κB, PI3K/Akt/mTOR, XO/NLRP3, Wnt/β-catenin, FXR, TGF-β1/Smad3, AMPK/SREBP, PPARα/γ, MAPKs, Caspases, and JAK2-STAT3 are considered critical targets through which API exerts its hepatoprotective effects. These findings provide robust scientific evidence for API as a potential therapeutic agent for liver diseases and lay a solid foundation for further exploration of its clinical application prospects. The molecular pathways of hepatoprotective effect of API are shown in Figure 5.
In terms of toxicity, existing experiments indicate that API exhibits low toxicity in both in vitro and in vivo studies. Even at relatively high doses, it does not induce significant adverse effects, demonstrating good safety. However, the pharmacokinetic properties of API remain challenging. Studies have shown that API has poor water solubility and low oral bioavailability, which may affect its absorption efficiency and ultimately its therapeutic efficacy in vivo. Furthermore, API’s slow metabolism and excretion may lead to accumulation in the body, potentially increasing toxicity risks or drug interactions. Although some studies have aimed at improving the pharmacokinetic properties of API, in-depth research on its absorption, distribution, metabolism, and excretion mechanisms remains limited, necessitating further studies to optimize its clinical application potential. To overcome these challenges, researchers are actively developing various drug delivery systems, such as nanoparticles, nanosuspensions, solid dispersions, and phospholipid phytosomes, to enhance the solubility and bioavailability of API, thereby increasing its therapeutic efficacy. Future studies should continue to explore and optimize these delivery systems to improve API’s drug characteristics and expand its clinical application prospects.
In light of these challenges, to effectively translate API into a clinically effective treatment for liver diseases, we propose the following recommendations:
(1) Further elucidation of mechanisms of action: Although the multiple biological activities of API have been confirmed, its specific mechanisms of action in liver diseases remain unclear. Future research should focus on elucidating its molecular mechanisms in oxidative stress, inflammatory responses, fibrosis progression, and the gut-liver axis to support its clinical application. Moreover, the integration of cutting-edge technologies such as single-cell sequencing, genomics, and transcriptomics in future studies could aid in revealing the comprehensive mechanisms of API, providing new perspectives for its application in the treatment of liver diseases.
(2) Toxicity evaluation and safety assessment: While API has demonstrated low toxicity in existing experiments, its long-term safety requires further evaluation. Future studies should systematically assess the safety of API in large-scale clinical trials and long-term toxicity studies, particularly at high doses and with prolonged use, to observe its potential impacts on the liver and other organs. In-depth toxicological research will ensure the safety of API in clinical applications.
(3) Drug delivery and bioavailability enhancement: The low bioavailability of API poses a significant obstacle to its clinical application. Future research should focus on developing various drug delivery systems, such as nanoparticles, nanosuspensions, and solid dispersions, to improve the solubility, bioavailability, and targeting capability of API. Additionally, exploring multifunctional carrier systems with combined functionalities, such as targeted delivery, controlled release, and imaging monitoring, could further enhance the therapeutic efficacy and clinical application prospects of API.
(4) Structural modification and prodrug design: Structural modifications of API or the design of prodrugs could enhance its pharmacological activity, stability, and absorption efficiency. For example, the addition of hydrophilic groups could improve its water solubility, thereby enhancing absorption efficiency; the introduction of specific chemical groups could enhance its anti-inflammatory or anti-fibrotic activity. Prodrug design could leverage enzymatic reactions within the body to release active API at the target site, thereby improving its efficacy.
(5) Clinical translation research and large-scale trials: Although current research has demonstrated the potential of API in hepatoprotection, large-scale clinical trials are lacking. Future studies should conduct large-scale, multicenter, randomized controlled clinical trials to validate the efficacy and safety of API in various liver diseases, such as NAFLD, liver fibrosis, and liver cancer. These clinical trials should cover diverse patient populations and include long-term follow-up to evaluate efficacy and safety, laying the groundwork for the clinical promotion of API.
(6) Exploration of combination therapy strategies: Future research should investigate combination therapy strategies involving API and other drugs, such as antiviral agents, antifibrotic drugs, or antioxidants, to evaluate their synergistic effects and potential drug interactions. Combination therapy may enhance overall efficacy, reduce the dosage and side effects of single drugs, and achieve better therapeutic outcomes.
In conclusion, this study comprehensively reviews the source, physicochemical properties, hepatoprotective effects, molecular mechanisms, toxicity, pharmacokinetics, and current development status of new formulations of API, while deeply exploring the challenges and opportunities in current research. Through this systematic summary, this study lays a solid theoretical foundation for further understanding the hepatoprotective effects and clinical application value of API, and it is expected to provide guidance for future research and application.
Author contributions
CW: Conceptualization, Methodology, Software, Writing–original draft. XF: Data curation, Investigation, Writing–original draft. WL: Writing–review and editing. LC: Writing–review and editing. XW: Writing–review and editing. YL: Writing–review and editing. RT: Writing–review and editing. TJ: Writing–review and editing. LZ: Writing–review and editing. GL: Writing–review and editing.
Funding
The author(s) declare that financial support was received for the research, authorship, and/or publication of this article. This work was supported by Sichuan Province Science and Technology Support Program (No: 2023YFSY0040), Sichuan Province Medical Research Project Program (No: S20024).
Conflict of interest
The authors declare that the research was conducted in the absence of any commercial or financial relationships that could be construed as a potential conflict of interest.
Generative AI statement
The author(s) declare that no Generative AI was used in the creation of this manuscript.
Publisher’s note
All claims expressed in this article are solely those of the authors and do not necessarily represent those of their affiliated organizations, or those of the publisher, the editors and the reviewers. Any product that may be evaluated in this article, or claim that may be made by its manufacturer, is not guaranteed or endorsed by the publisher.
References
Ahlmann, M., and Hempel, G. (2016). The effect of cyclophosphamide on the immune system: implications for clinical cancer therapy. Cancer Chemother. Pharmacol. 78 (4), 661–671. doi:10.1007/s00280-016-3152-1
Al-Amarat, W., Abukhalil, M. H., Alruhaimi, R. S., Alqhtani, H. A., Aldawood, N., Alfwuaires, M. A., et al. (2022). Upregulation of Nrf2/HO-1 signaling and attenuation of oxidative stress, inflammation, and cell death mediate the protective effect of apigenin against cyclophosphamide hepatotoxicity. Metabolites 12 (7), 648. doi:10.3390/metabo12070648
Ali, A. A., Mansour, A. B., and Attia, S. A. (2021). The potential protective role of apigenin against oxidative damage induced by nickel oxide nanoparticles in liver and kidney of male Wistar rat, Rattus norvegicus. Environ. Sci. Pollut. Res. Int. 28 (22), 27577–27592. doi:10.1007/s11356-021-12632-3
Ali, F., Rahul, , Naz, F., Jyoti, S., and Siddique, Y. H. (2014). Protective effect of apigenin against N-nitrosodiethylamine (NDEA)-induced hepatotoxicity in albino rats. Mutat. Res. Genet. Toxicol. Environ. Mutagen 767, 13–20. doi:10.1016/j.mrgentox.2014.04.006
Allameh, A., Niayesh-Mehr, R., Aliarab, A., Sebastiani, G., and Pantopoulos, K. (2023). Oxidative stress in liver pathophysiology and disease. Antioxidants (Basel) 12 (9), 1653. doi:10.3390/antiox12091653
Alshehri, S. M., Shakeel, F., Ibrahim, M. A., Elzayat, E. M., Altamimi, M., Mohsin, K., et al. (2019). Dissolution and bioavailability improvement of bioactive apigenin using solid dispersions prepared by different techniques. Saudi Pharm. J. 27 (2), 264–273. doi:10.1016/j.jsps.2018.11.008
Anwanwan, D., Singh, S. K., Singh, S., Saikam, V., and Singh, R. (2020). Challenges in liver cancer and possible treatment approaches. Biochim. Biophys. Acta Rev. Cancer 1873 (1), 188314. doi:10.1016/j.bbcan.2019.188314
Aydın, M. M., and Akçalı, K. C. (2018). Liver fibrosis. Turk J. Gastroenterol. 29 (1), 14–21. doi:10.5152/tjg.2018.17330
Bardallo, R. G., Panisello-Roselló, A., Sanchez-Nuno, S., Alva, N., Roselló-Catafau, J., and Carbonell, T. (2022). Nrf2 and oxidative stress in liver ischemia/reperfusion injury. Febs J. 289 (18), 5463–5479. doi:10.1111/febs.16336
Bathish, B., Robertson, H., Dillon, J. F., Dinkova-Kostova, A. T., and Hayes, J. D. (2022). Nonalcoholic steatohepatitis and mechanisms by which it is ameliorated by activation of the CNC-bZIP transcription factor Nrf2. Free Radic. Biol. Med. 188, 221–261. doi:10.1016/j.freeradbiomed.2022.06.226
Batool, Z., Xu, D., Zhang, X., Li, X., Li, Y., Chen, Z., et al. (2021). A review on furan: formation, analysis, occurrence, carcinogenicity, genotoxicity and reduction methods. Crit. Rev. Food Sci. Nutr. 61 (3), 395–406. doi:10.1080/10408398.2020.1734532
Berhe, M. G., and Gebreslassie, Y. T. (2023). Biomedical applications of biosynthesized nickel oxide nanoparticles. Int. J. Nanomedicine 18, 4229–4251. doi:10.2147/ijn.S410668
Berköz, M., Ünal, S., Karayakar, F., Yunusoğlu, O., Özkan-Yılmaz, F., Özlüer-Hunt, A., et al. (2021). Prophylactic effect of myricetin and apigenin against lipopolysaccharide-induced acute liver injury. Mol. Biol. Rep. 48 (9), 6363–6373. doi:10.1007/s11033-021-06637-x
Bhattacharya, S., Mondal, L., Mukherjee, B., Dutta, L., Ehsan, I., Debnath, M. C., et al. (2018). Apigenin loaded nanoparticle delayed development of hepatocellular carcinoma in rats. Nanomedicine 14 (6), 1905–1917. doi:10.1016/j.nano.2018.05.011
Cahyani, D. M., Miatmoko, A., Hariawan, B. S., Purwantari, K. E., and Sari, R. (2021). N-nitrosodiethylamine induces inflammation of liver in mice. J. Basic Clin. Physiol. Pharmacol. 32 (4), 505–510. doi:10.1515/jbcpp-2020-0475
Chavhan, R. (2024). Nanosuspensions: enhancing drug bioavailability through nanonization. Ann. Pharm. Fr. doi:10.1016/j.pharma.2024.06.003
Chen, B., Ning, K., Sun, M. L., and Zhang, X. A. (2023). Regulation and therapy, the role of JAK2/STAT3 signaling pathway in OA: a systematic review. Cell Commun. Signal 21 (1), 67. doi:10.1186/s12964-023-01094-4
Chen, H., Tan, H., Wan, J., Zeng, Y., Wang, J., Wang, H., et al. (2023). PPAR-γ signaling in nonalcoholic fatty liver disease: pathogenesis and therapeutic targets. Pharmacol. Ther. 245, 108391. doi:10.1016/j.pharmthera.2023.108391
Chen M., M., Zhong, W., and Xu, W. (2023). Alcohol and the mechanisms of liver disease. J. Gastroenterol. Hepatol. 38 (8), 1233–1240. doi:10.1111/jgh.16282
Chen, T., Wang, G. X., Pan, X. D., Wang, J., and Li, T. M. (2015). Effects of apigenin on proliferationof human hepatocellular carcinoma Hepg2 cells and glucose uptake. Chin. J. Exp. Tradit. Med. Formulae 21 (23), 106–109. doi:10.13422/j.cnki.syfjx.2015230106
Chen, Z., Tian, R., She, Z., Cai, J., and Li, H. (2020). Role of oxidative stress in the pathogenesis of nonalcoholic fatty liver disease. Free Radic. Biol. Med. 152, 116–141. doi:10.1016/j.freeradbiomed.2020.02.025
Chiang, L. C., Ng, L. T., Lin, I. C., Kuo, P. L., and Lin, C. C. (2006). Anti-proliferative effect of apigenin and its apoptotic induction in human Hep G2 cells. Cancer Lett. 237 (2), 207–214. doi:10.1016/j.canlet.2005.06.002
Cho, J. G., Choi, J. S., Lee, J. H., Cho, M. G., Kim, H. S., Noh, H. D., et al. (2019). MED28 over-expression shortens the cell cycle and induces genomic instability. Int. J. Mol. Sci. 20 (7), 1746. doi:10.3390/ijms20071746
Chou, J. C., Liu, C. C., and Lee, M. F. (2024). Apigenin suppresses MED28-mediated cell growth in human liver cancer cells. J. Agric. Food Chem. doi:10.1021/acs.jafc.3c09276
Clark, A. G., and Vignjevic, D. M. (2015). Modes of cancer cell invasion and the role of the microenvironment. Curr. Opin. Cell Biol. 36, 13–22. doi:10.1016/j.ceb.2015.06.004
Crozier, A., Lean, M., Mcdonald, M. S., and Black, C. (1997). Quantitative analysis of the flavonoid content of commercial tomatoes. Journal of Agricultural and Food Chemistry 45:590–595. doi:10.1021/jf960339y
Cui, Y. H., Chen, A., Xu, C., Cao, J. G., Xiang, H. L., and Zhang, J. S. (2018). Inhibition of spheroid formation and STAT3 phosphorylation through up-regulating expression of SHP-1 by apigenin in human liver cancer MHCC97H cells. J. Hunan Norm. Univ. Med. Sci. 15 (05), 1–4.
Dai, S., Wang, C., Zhao, X., Ma, C., Fu, K., Liu, Y., et al. (2023). Cucurbitacin B: a review of its pharmacology, toxicity, and pharmacokinetics. Pharmacol. Res. 187, 106587. doi:10.1016/j.phrs.2022.106587
Das, S., Das, J., Samadder, A., Paul, A., and Khuda-Bukhsh, A. R. (2013). Efficacy of PLGA-loaded apigenin nanoparticles in Benzo[a]pyrene and ultraviolet-B induced skin cancer of mice: mitochondria mediated apoptotic signalling cascades. Food Chem. Toxicol. 62, 670–680. doi:10.1016/j.fct.2013.09.037
de Carvalho Ribeiro, M., and Szabo, G. (2022). Role of the inflammasome in liver disease. Annu. Rev. Pathol. 17, 345–365. doi:10.1146/annurev-pathmechdis-032521-102529
de Conti, A., Beland, F. A., and Pogribny, I. P. (2017). The role of epigenomic alterations in furan-induced hepatobiliary pathologies. Food Chem. Toxicol. 109 (Pt 1), 677–682. doi:10.1016/j.fct.2017.07.049
Devarbhavi, H., Asrani, S. K., Arab, J. P., Nartey, Y. A., Pose, E., and Kamath, P. S. (2023). Global burden of liver disease: 2023 update. J. Hepatol. 79 (2), 516–537. doi:10.1016/j.jhep.2023.03.017
Dhara, M., Al Hoque, A., Sen, R., Dutta, D., Mukherjee, B., Paul, B., et al. (2023). Phosphorothioated amino-AS1411 aptamer functionalized stealth nanoliposome accelerates bio-therapeutic threshold of apigenin in neoplastic rat liver: a mechanistic approach. J. Nanobiotechnology 21 (1), 28. doi:10.1186/s12951-022-01764-4
Ding, S. M., Zhang, Z. H., Song, J., Cheng, X. D., Jiang, J., and Jia, X. B. (2014). Enhanced bioavailability of apigenin via preparation of a carbon nanopowder solid dispersion. Int. J. Nanomedicine 9, 2327–2333. doi:10.2147/ijn.S60938
Duan, S., Li, X., Han, J., Yang, Y., Luo, R., Cai, Y., et al. (2024). Transcriptotype-driven discovery of apigenin as a therapy against cholestatic liver fibrosis: through inhibition of PANoptosis and following type-I interferon responses. Antioxidants (Basel) 13 (3), 256. doi:10.3390/antiox13030256
Durán, N., Durán, M., de Jesus, M. B., Seabra, A. B., Fávaro, W. J., and Nakazato, G. (2016). Silver nanoparticles: a new view on mechanistic aspects on antimicrobial activity. Nanomedicine 12 (3), 789–799. doi:10.1016/j.nano.2015.11.016
Elmowafy, M., Shalaby, K., Elkomy, M. H., Alsaidan, O. A., Gomaa, H. A. M., Abdelgawad, M. A., et al. (2023). Polymeric nanoparticles for delivery of natural bioactive agents: recent advances and challenges. Polym. (Basel) 15 (5), 1123. doi:10.3390/polym15051123
Elzayat, E. M., Shakeel, F., Alshehri, S., Ibrahim, M. A., Altamimi, M. A., Kazi, M., et al. (2019). UHPLC assisted simultaneous separation of apigenin and prednisolone and its application in the pharmacokinetics of apigenin. J. Chromatogr. B Anal. Technol. Biomed. Life Sci. 1117, 58–65. doi:10.1016/j.jchromb.2019.04.006
Emadi, A., Jones, R. J., and Brodsky, R. A. (2009). Cyclophosphamide and cancer: golden anniversary. Nat. Rev. Clin. Oncol. 6 (11), 638–647. doi:10.1038/nrclinonc.2009.146
Engeland, K. (2022). Cell cycle regulation: p53-p21-RB signaling. Cell Death Differ. 29 (5), 946–960. doi:10.1038/s41418-022-00988-z
Escudier, B., Worden, F., and Kudo, M. (2019). Sorafenib: key lessons from over 10 years of experience. Expert Rev. Anticancer Ther. 19 (2), 177–189. doi:10.1080/14737140.2019.1559058
Fang, C., Pan, J., Qu, N., Lei, Y., Han, J., Zhang, J., et al. (2022). The AMPK pathway in fatty liver disease. Front. Physiol. 13, 970292. doi:10.3389/fphys.2022.970292
Farghali, H., Kgalalelo Kemelo, M., Wojnarová, L., and Kutinová Canová, N. (2016). In vitro and in vivo experimental hepatotoxic models in liver research: applications to the assessment of potential hepatoprotective drugs. Physiol. Res. 65 (Suppl. 4), S417-S425–s425. doi:10.33549/physiolres.933506
Fehaid, A., Al-Ghamdi, M. S., Alzahrani, K. J., Theyab, A., Al-Amer, O. M., Al-Shehri, S. S., et al. (2023). Apigenin protects from hepatorenal damage caused by lead acetate in rats. J. Biochem. Mol. Toxicol. 37 (3), e23275. doi:10.1002/jbt.23275
Feng, X., Weng, D., Zhou, F., Owen, Y. D., Qin, H., Zhao, J., et al. (2016). Activation of PPARγ by a natural flavonoid modulator, apigenin ameliorates obesity-related inflammation via regulation of macrophage polarization. EBioMedicine 9, 61–76. doi:10.1016/j.ebiom.2016.06.017
Feng, X., Yu, W., Li, X., Zhou, F., Zhang, W., Shen, Q., et al. (2017). Apigenin, a modulator of PPARγ, attenuates HFD-induced NAFLD by regulating hepatocyte lipid metabolism and oxidative stress via Nrf2 activation. Biochem. Pharmacol. 136, 136–149. doi:10.1016/j.bcp.2017.04.014
Fu, J., and Wu, H. (2023). Structural mechanisms of NLRP3 inflammasome assembly and activation. Annu. Rev. Immunol. 41, 301–316. doi:10.1146/annurev-immunol-081022-021207
Ganguly, S., Dewanjee, S., Sen, R., Chattopadhyay, D., Ganguly, S., Gaonkar, R., et al. (2021). Apigenin-loaded galactose tailored PLGA nanoparticles: a possible strategy for liver targeting to treat hepatocellular carcinoma. Colloids Surf. B Biointerfaces 204, 111778. doi:10.1016/j.colsurfb.2021.111778
Gao, A. M., Ke, Z. P., Wang, J. N., Yang, J. Y., Chen, S. Y., and Chen, H. (2013). Apigenin sensitizes doxorubicin-resistant hepatocellular carcinoma BEL-7402/ADM cells to doxorubicin via inhibiting PI3K/Akt/Nrf2 pathway. Carcinogenesis 34 (8), 1806–1814. doi:10.1093/carcin/bgt108
Gao, A. M., Zhang, X. Y., Hu, J. N., and Ke, Z. P. (2018). Apigenin sensitizes hepatocellular carcinoma cells to doxorubic through regulating miR-520b/ATG7 axis. Chem. Biol. Interact. 280, 45–50. doi:10.1016/j.cbi.2017.11.020
Gauthier, A., and Ho, M. (2013). Role of sorafenib in the treatment of advanced hepatocellular carcinoma: an update. Hepatol. Res. 43 (2), 147–154. doi:10.1111/j.1872-034X.2012.01113.x
Ghalehbandi, S., Yuzugulen, J., Pranjol, M. Z. I., and Pourgholami, M. H. (2023). The role of VEGF in cancer-induced angiogenesis and research progress of drugs targeting VEGF. Eur. J. Pharmacol. 949, 175586. doi:10.1016/j.ejphar.2023.175586
Ghosh, S. (2019). Cisplatin: the first metal based anticancer drug. Bioorg Chem. 88, 102925. doi:10.1016/j.bioorg.2019.102925
Ginès, P., Castera, L., Lammert, F., Graupera, I., Serra-Burriel, M., Allen, A. M., et al. (2022). Population screening for liver fibrosis: toward early diagnosis and intervention for chronic liver diseases. Hepatology 75 (1), 219–228. doi:10.1002/hep.32163
Gnananath, K., Sri Nataraj, K., and Ganga Rao, B. (2017). Phospholipid complex technique for superior bioavailability of phytoconstituents. Adv. Pharm. Bull. 7 (1), 35–42. doi:10.15171/apb.2017.005
Goudarzi, M., Kalantar, M., Sadeghi, E., Karamallah, M. H., and Kalantar, H. (2021). Protective effects of apigenin on altered lipid peroxidation, inflammation, and antioxidant factors in methotrexate-induced hepatotoxicity. Naunyn Schmiedeb. Arch. Pharmacol. 394 (3), 523–531. doi:10.1007/s00210-020-01991-2
Gradolatto, A., Basly, J. P., Berges, R., Teyssier, C., Chagnon, M. C., Siess, M. H., et al. (2005). Pharmacokinetics and metabolism of apigenin in female and male rats after a single oral administration. Drug Metab. Dispos. 33 (1), 49–54. doi:10.1124/dmd.104.000893
Gupta, M. K., Sansare, V., Shrivastava, B., Jadhav, S., and Gurav, P. (2022). Comprehensive review on use of phospholipid based vesicles for phytoactive delivery. J. Liposome Res. 32 (3), 211–223. doi:10.1080/08982104.2021.1968430
Hafezi, S., and Rahmani, M. (2021). Targeting BCL-2 in cancer: advances, challenges, and perspectives. Cancers (Basel) 13 (6), 1292. doi:10.3390/cancers13061292
Han, D., Yao, Y., Chen, L., Miao, Z., and Xu, S. (2022). Apigenin ameliorates di(2-ethylhexyl) phthalate-induced ferroptosis: The activation of glutathione peroxidase 4 and suppression of iron intake. Food Chem. Toxicol. 164, 113089. doi:10.1016/j.fct.2022.113089
Hicks, D. F., Goossens, N., Blas-García, A., Tsuchida, T., Wooden, B., Wallace, M. C., et al. (2017). Transcriptome-based repurposing of apigenin as a potential anti-fibrotic agent targeting hepatic stellate cells. Sci. Rep. 7, 42563. doi:10.1038/srep42563
Higashi, T., Friedman, S. L., and Hoshida, Y. (2017). Hepatic stellate cells as key target in liver fibrosis. Adv. Drug Deliv. Rev. 121, 27–42. doi:10.1016/j.addr.2017.05.007
Hoek-van den Hil, E. F., van Schothorst, E. M., van der Stelt, I., Swarts, H. J., van Vliet, M., Amolo, T., et al. (2015). Direct comparison of metabolic health effects of the flavonoids quercetin, hesperetin, epicatechin, apigenin and anthocyanins in high-fat-diet-fed mice. Genes Nutr. 10 (4), 469. doi:10.1007/s12263-015-0469-z
Hsu, M. C., Chen, C. H., Wang, M. C., Chen, W. H., Hu, P. A., Guo, B. C., et al. (2024). Apigenin targets fetuin-A to ameliorate obesity-induced insulin resistance. Int. J. Biol. Sci. 20 (5), 1563–1577. doi:10.7150/ijbs.91695
Hsu, M. C., Guo, B. C., Chen, C. H., Hu, P. A., and Lee, T. S. (2021). Apigenin ameliorates hepatic lipid accumulation by activating the autophagy-mitochondria pathway. J. Food Drug Anal. 29 (2), 240–254. doi:10.38212/2224-6614.3269
Hu, H., Chen, Y., Tan, S., Wu, S., Huang, Y., Fu, S., et al. (2022). The research progress of antiangiogenic therapy, immune therapy and tumor microenvironment. Front. Immunol. 13, 802846. doi:10.3389/fimmu.2022.802846
Hu, J., Li, A., Guo, Y., Ma, T., and Feng, S. (2023). The relationship between tumor metabolism and 5-fluorouracil resistance. Biochem. Pharmacol. 218, 115902. doi:10.1016/j.bcp.2023.115902
Hu, X. Y., Liang, J. Y., Guo, X. J., Liu, L., and Guo, Y. B. (2015). 5-Fluorouracil combined with apigenin enhances anticancer activity through mitochondrial membrane potential (ΔΨm)-mediated apoptosis in hepatocellular carcinoma. Clin. Exp. Pharmacol. Physiol. 42 (2), 146–153. doi:10.1111/1440-1681.12333
Huang, B., Lang, X., and Li, X. (2022). The role of IL-6/JAK2/STAT3 signaling pathway in cancers. Front. Oncol. 12, 1023177. doi:10.3389/fonc.2022.1023177
Huang, C., Wei, Y. X., Shen, M. C., Tu, Y. H., Wang, C. C., and Huang, H. C. (2016). Chrysin, abundant in Morinda citrifolia fruit water-EtOAc extracts, combined with apigenin synergistically induced apoptosis and inhibited migration in human breast and liver cancer cells. J. Agric. Food Chem. 64 (21), 4235–4245. doi:10.1021/acs.jafc.6b00766
Huang, Y., Xu, W., and Zhou, R. (2021). NLRP3 inflammasome activation and cell death. Cell Mol. Immunol. 18 (9), 2114–2127. doi:10.1038/s41423-021-00740-6
Huang, Y., Zhao, X., Zu, Y., Wang, L., Deng, Y., Wu, M., et al. (2019a). Enhanced solubility and bioavailability of apigenin via preparation of solid dispersions of mesoporous silica nanoparticles. Iran. J. Pharm. Res. 18 (1), 168–182.
Huang, Y., Zhao, X., Zu, Y., Wang, L., Wang, H., Wu, M., et al. (2019b). Enhanced solubility and bioavailability of apigenin via preparation of solid dispersions of mesoporous silica nanoparticles. Iran. J. Pharm. Res. (IJPR) 18 (1), 168–182.
Ishitsuka, Y., Kondo, Y., and Kadowaki, D. (2020). Toxicological property of acetaminophen: the dark side of a safe antipyretic/analgesic drug? Biol. Pharm. Bull. 43 (2), 195–206. doi:10.1248/bpb.b19-00722
Ismail, T., Lee, H. K., Kim, C., Kwon, T., Park, T. J., and Lee, H. S. (2018). KDM1A microenvironment, its oncogenic potential, and therapeutic significance. Epigenetics Chromatin 11 (1), 33. doi:10.1186/s13072-018-0203-3
Jaeschke, H., and Ramachandran, A. (2024). Acetaminophen hepatotoxicity: paradigm for understanding mechanisms of drug-induced liver injury. Annu. Rev. Pathol. 19, 453–478. doi:10.1146/annurev-pathmechdis-051122-094016
Janmeda, P., Jain, D., Chaudhary, P., Meena, M., and Singh, D. (2024). A systematic review on multipotent carcinogenic agent, N-nitrosodiethylamine (NDEA), its major risk assessment, and precautions. J. Appl. Toxicol. 44 (8), 1108–1128. doi:10.1002/jat.4574
Ji, J., Yu, Q., Dai, W., Wu, L., Feng, J., Zheng, Y., et al. (2021). Apigenin alleviates liver fibrosis by inhibiting hepatic stellate cell activation and autophagy via TGF-β1/smad3 and p38/pparα pathways. PPAR Res. 2021, 1–15. doi:10.1155/2021/6651839
Jiang, S. F., Xu, C., Chen, A., Cui, Y. H., Li, X., and Cao, J. G. (2017). Effects of apigenin on self-renewal and expression of CK2α of liver cancer stem-like cells derived from SMMC-7721 cell line. J. Hunan Norm. Univ. Med. Sci. 14 (05), 1–3.
Jiménez-Castro, M. B., Cornide-Petronio, M. E., Gracia-Sancho, J., and Peralta, C. (2019). Inflammasome-mediated inflammation in liver ischemia-reperfusion injury. Cells 8 (10), 1131. doi:10.3390/cells8101131
Johnson, A. P., Sabu, C., Nivitha, K. P., Sankar, R., Ameena Shirin, V. K., Henna, T. K., et al. (2022). Bioinspired and biomimetic micro- and nanostructures in biomedicine. J. Control Release 343, 724–754. doi:10.1016/j.jconrel.2022.02.013
Jung, U. J., Cho, Y. Y., and Choi, M. S. (2016). Apigenin ameliorates dyslipidemia, hepatic steatosis and insulin resistance by modulating metabolic and transcriptional profiles in the liver of high-fat diet-induced obese mice. Nutrients 8 (5), 305. doi:10.3390/nu8050305
Kalluri, R., and McAndrews, K. M. (2023). The role of extracellular vesicles in cancer. Cell 186 (8), 1610–1626. doi:10.1016/j.cell.2023.03.010
Kciuk, M., Gielecińska, A., Mujwar, S., Kołat, D., Kałuzińska-Kołat, Ż., Celik, I., et al. (2023). Doxorubicin-an agent with multiple mechanisms of anticancer activity. Cells 12 (4), 659. doi:10.3390/cells12040659
Keating, G. M. (2017). Sorafenib: a review in hepatocellular carcinoma. Target Oncol. 12 (2), 243–253. doi:10.1007/s11523-017-0484-7
Khan, H. A., Ahmad, M. Z., Khan, J. A., and Arshad, M. I. (2017). Crosstalk of liver immune cells and cell death mechanisms in different murine models of liver injury and its clinical relevance. Hepatobiliary Pancreat. Dis. Int. 16 (3), 245–256. doi:10.1016/s1499-3872(17)60014-6
Khan, R. S., Bril, F., Cusi, K., and Newsome, P. N. (2019). Modulation of insulin resistance in nonalcoholic fatty liver disease. Hepatology 70 (2), 711–724. doi:10.1002/hep.30429
Khan, T. H., and Sultana, S. (2006). Apigenin induces apoptosis in Hep G2 cells: possible role of TNF-alpha and IFN-gamma. Toxicology 217 (2-3), 206–212. doi:10.1016/j.tox.2005.09.019
Kim, B. R., Jeon, Y. K., and Nam, M. J. (2011). A mechanism of apigenin-induced apoptosis is potentially related to anti-angiogenesis and anti-migration in human hepatocellular carcinoma cells. Food Chem. Toxicol. 49 (7), 1626–1632. doi:10.1016/j.fct.2011.04.015
Kim, S. J., Cho, H. I., Kim, S. J., Park, J. H., Kim, J. S., Kim, Y. H., et al. (2014). Protective effect of linarin against D-galactosamine and lipopolysaccharide-induced fulminant hepatic failure. Eur. J. Pharmacol. 738, 66–73. doi:10.1016/j.ejphar.2014.05.024
Koziolek, M., Alcaro, S., Augustijns, P., Basit, A. W., Grimm, M., Hens, B., et al. (2019). The mechanisms of pharmacokinetic food-drug interactions - a perspective from the UNGAP group. Eur. J. Pharm. Sci. 134, 31–59. doi:10.1016/j.ejps.2019.04.003
Kubes, P., and Jenne, C. (2018). Immune responses in the liver. Annu. Rev. Immunol. 36, 247–277. doi:10.1146/annurev-immunol-051116-052415
Lackner, C., and Tiniakos, D. (2019). Fibrosis and alcohol-related liver disease. J. Hepatol. 70 (2), 294–304. doi:10.1016/j.jhep.2018.12.003
Lakka, N., Pai, B., Mani, M. S., and Dsouza, H. S. (2023). Potential diagnostic biomarkers for lead-induced hepatotoxicity and the role of synthetic chelators and bioactive compounds. Toxicol. Res. (Camb) 12 (2), 178–188. doi:10.1093/toxres/tfad014
Lanthier, N., Lebrun, V., Molendi-Coste, O., van Rooijen, N., and Leclercq, I. A. (2022). Liver fetuin-A at initiation of insulin resistance. Metabolites 12 (11), 1023. doi:10.3390/metabo12111023
Lee, T. K., Guan, X. Y., and Ma, S. (2022). Cancer stem cells in hepatocellular carcinoma - from origin to clinical implications. Nat. Rev. Gastroenterol. Hepatol. 19 (1), 26–44. doi:10.1038/s41575-021-00508-3
Li, G., Chi, C. W., Shao, X. F., and Fang, C. H. (2017). Application of molecular imaging technology in evaluating the inhibiting effect of apigenin in vivo on subcutaneous hepatocellular carcinoma. Biochem. Biophys. Res. Commun. 487 (1), 122–127. doi:10.1016/j.bbrc.2017.04.029
Li, K., Li, M., Luo, Z., Mao, Y., Yu, Y., He, Y., et al. (2020). Overcoming the hypoxia-induced drug resistance in liver tumor by the concurrent use of apigenin and paclitaxel. Biochem. Biophys. Res. Commun. 526 (2), 321–327. doi:10.1016/j.bbrc.2020.03.010
Liu, Y., Zhao, F., Wang, Q., Zhao, Q., Hou, G., and Meng, Q. (2023). Current perspectives on paclitaxel: focus on its production, delivery and combination therapy. Mini Rev. Med. Chem. 23 (18), 1780–1796. doi:10.2174/1389557523666230210145150
Liu, Y. C., Yeh, C. T., and Lin, K. H. (2020). Cancer stem cell functions in hepatocellular carcinoma and comprehensive therapeutic strategies. Cells 9 (6), 1331. doi:10.3390/cells9061331
Lu, J., Meng, Z., Cheng, B., Liu, M., Tao, S., and Guan, S. (2019). Apigenin reduces the excessive accumulation of lipids induced by palmitic acid via the AMPK signaling pathway in HepG2 cells. Exp. Ther. Med. 18 (4), 2965–2971. doi:10.3892/etm.2019.7905
Lu, Z., Liu, L., Zhao, S., Zhao, J., Li, S., and Li, M. (2023). Apigenin attenuates atherosclerosis and non-alcoholic fatty liver disease through inhibition of NLRP3 inflammasome in mice. Sci. Rep. 13 (1), 7996. doi:10.1038/s41598-023-34654-2
Lv, C. (2016). Biodistribution of apigenin nanosuspension and its pharmacodynamics studies on anti-liver cancer in vivo and vitro.
Lv, Y., Gao, X., Luo, Y., Fan, W., Shen, T., Ding, C., et al. (2019). Apigenin ameliorates HFD-induced NAFLD through regulation of the XO/NLRP3 pathways. J. Nutr. Biochem. 71, 110–121. doi:10.1016/j.jnutbio.2019.05.015
Mackowiak, B., Fu, Y., Maccioni, L., and Gao, B. (2024). Alcohol-associated liver disease. J. Clin. Invest 134 (3). doi:10.1172/jci176345
Madunić, J., Madunić, I. V., Gajski, G., Popić, J., and Garaj-Vrhovac, V. (2018). Apigenin: a dietary flavonoid with diverse anticancer properties. Cancer Lett. 413, 11–22. doi:10.1016/j.canlet.2017.10.041
Maes, M., Vinken, M., and Jaeschke, H. (2016). Experimental models of hepatotoxicity related to acute liver failure. Toxicol. Appl. Pharmacol. 290, 86–97. doi:10.1016/j.taap.2015.11.016
Mahmoud, M., Mahmoud, D., Brotherton, T., Awali, M., and Qureshi, K. (2023). S1486Impact of obesity on liver fibrosis and steatosis in patients diagnosed with methotrexate-associated liver injury: a retrospective study. Am. J. Gastroenterology 118 (10S), S1128. doi:10.14309/01.ajg.0000955584.31466.98
Mani, R., and Natesan, V. (2018). Chrysin: sources, beneficial pharmacological activities, and molecular mechanism of action. Phytochemistry 145, 187–196. doi:10.1016/j.phytochem.2017.09.016
Marquardt, J. U., Andersen, J. B., and Thorgeirsson, S. S. (2015). Functional and genetic deconstruction of the cellular origin in liver cancer. Nat. Rev. Cancer 15 (11), 653–667. doi:10.1038/nrc4017
Martínez-Razo, L. D., Martínez-Ibarra, A., Vázquez-Martínez, E. R., and Cerbón, M. (2021). The impact of Di-(2-ethylhexyl) Phthalate and Mono(2-ethylhexyl) Phthalate in placental development, function, and pathophysiology. Environ. Int. 146, 106228. doi:10.1016/j.envint.2020.106228
McSweeney, K. R., Gadanec, L. K., Qaradakhi, T., Ali, B. A., Zulli, A., and Apostolopoulos, V. (2021). Mechanisms of cisplatin-induced acute kidney injury: pathological mechanisms, pharmacological interventions, and genetic mitigations. Cancers (Basel) 13 (7), 1572. doi:10.3390/cancers13071572
Melaibari, M., Alkreathy, H. M., Esmat, A., Rajeh, N. A., Shaik, R. A., Alghamdi, A. A., et al. (2023). Anti-fibrotic efficacy of apigenin in a mice model of carbon tetrachloride-induced hepatic fibrosis by modulation of oxidative stress, inflammation, and fibrogenesis: a preclinical study. Biomedicines 11 (5), 1342. doi:10.3390/biomedicines11051342
Méndez-Sánchez, N., Coronel-Castillo, C. E., and Ordoñez-Vázquez, A. L. (2023). Current therapies for cholestatic diseases. Biomedicines 11 (6), 1713. doi:10.3390/biomedicines11061713
Meng, Z., Gao, M., Wang, C., Guan, S., Zhang, D., and Lu, J. (2023). Apigenin alleviated high-fat-diet-induced hepatic pyroptosis by mitophagy-ROS-CTSB-NLRP3 pathway in mice and AML12 cells. J. Agric. Food Chem. 71 (18), 7032–7045. doi:10.1021/acs.jafc.2c07581
Meng, Z., Zhu, B., Gao, M., Wang, G., Zhou, H., Lu, J., et al. (2022). Apigenin alleviated PA-induced pyroptosis by activating autophagy in hepatocytes. Food Funct. 13 (10), 5559–5570. doi:10.1039/d1fo03771d
Moore, M. J. (1991). Clinical pharmacokinetics of cyclophosphamide. Clin. Pharmacokinet. 20 (3), 194–208. doi:10.2165/00003088-199120030-00002
Morse, M. A., Sun, W., Kim, R., He, A. R., Abada, P. B., Mynderse, M., et al. (2019). The role of angiogenesis in hepatocellular carcinoma. Clin. Cancer Res. 25 (3), 912–920. doi:10.1158/1078-0432.Ccr-18-1254
Neshat, S. Y., Quiroz, V. M., Wang, Y., Tamayo, S., and Doloff, J. C. (2021). Liver disease: induction, progression, immunological mechanisms, and therapeutic interventions. Int. J. Mol. Sci. 22 (13), 6777. doi:10.3390/ijms22136777
Pan, F. F., Zheng, Y. B., Shi, C. J., Zhang, F. W., Zhang, J. F., and Fu, W. M. (2021). H19-Wnt/β-catenin regulatory axis mediates the suppressive effects of apigenin on tumor growth in hepatocellular carcinoma. Eur. J. Pharmacol. 893, 173810. doi:10.1016/j.ejphar.2020.173810
Pan, X., Shao, Y., Wang, F., Cai, Z., Liu, S., Xi, J., et al. (2020). Protective effect of apigenin magnesium complex on H(2)O(2)-induced oxidative stress and inflammatory responses in rat hepatic stellate cells. Pharm. Biol. 58 (1), 553–560. doi:10.1080/13880209.2020.1772840
Papa, A., and Pandolfi, P. P. (2019). The PTEN⁻PI3K Axis in cancer. Biomolecules 9 (4), 153. doi:10.3390/biom9040153
Papachristou, F., Anninou, N., Koukoulis, G., Paraskakis, S., Sertaridou, E., Tsalikidis, C., et al. (2021). Differential effects of cisplatin combined with the flavonoid apigenin on HepG2, Hep3B, and Huh7 liver cancer cell lines. Mutat. Res. Genet. Toxicol. Environ. Mutagen 866, 503352. doi:10.1016/j.mrgentox.2021.503352
Parola, M., and Pinzani, M. (2019). Liver fibrosis: pathophysiology, pathogenetic targets and clinical issues. Mol. Asp. Med. 65, 37–55. doi:10.1016/j.mam.2018.09.002
Patel, K. D., Singh, R. K., and Kim, H. W. (2019). Carbon-based nanomaterials as an emerging platform for theranostics. Mater. Horizons 6, 434–469. doi:10.1039/c8mh00966j
Pfalzgraff, A., and Weindl, G. (2019). Intracellular lipopolysaccharide sensing as a potential therapeutic target for sepsis. Trends Pharmacol. Sci. 40 (3), 187–197. doi:10.1016/j.tips.2019.01.001
Qiao, M., Yang, J., Zhu, Y., Zhao, Y., and Hu, J. (2020). Transcriptomics and proteomics analysis of system-level mechanisms in the liver of apigenin-treated fibrotic rats. Life Sci. 248, 117475. doi:10.1016/j.lfs.2020.117475
Qin, Y., Zhao, D., Zhou, H. G., Wang, X. H., Zhong, W. L., Chen, S., et al. (2016). Apigenin inhibits NF-κB and snail signaling, EMT and metastasis in human hepatocellular carcinoma. Oncotarget 7 (27), 41421–41431. doi:10.18632/oncotarget.9404
Qiu, Y. Y., Zhang, J., Zeng, F. Y., and Zhu, Y. Z. (2023). Roles of the peroxisome proliferator-activated receptors (PPARs) in the pathogenesis of nonalcoholic fatty liver disease (NAFLD). Pharmacol. Res. 192, 106786. doi:10.1016/j.phrs.2023.106786
Rašković, A., Gigov, S., Čapo, I., Paut Kusturica, M., Milijašević, B., Kojić-Damjanov, S., et al. (2017). Antioxidative and protective actions of apigenin in a paracetamol-induced hepatotoxicity rat model. Eur. J. Drug Metab. Pharmacokinet. 42 (5), 849–856. doi:10.1007/s13318-017-0407-0
Rathinam, V. A. K., Zhao, Y., and Shao, F. (2019). Innate immunity to intracellular LPS. Nat. Immunol. 20 (5), 527–533. doi:10.1038/s41590-019-0368-3
Rocha, C. R. R., Silva, M. M., Quinet, A., Cabral-Neto, J. B., and Menck, C. F. M. (2018). DNA repair pathways and cisplatin resistance: an intimate relationship. Clin. (Sao Paulo) 73 (Suppl. 1), e478s. doi:10.6061/clinics/2018/e478s
Roehlen, N., Crouchet, E., and Baumert, T. F. (2020). Liver fibrosis: mechanistic concepts and therapeutic perspectives. Cells 9 (4), 875. doi:10.3390/cells9040875
Sahindokuyucu-Kocasari, F., Akyol, Y., Ozmen, O., Erdemli-Kose, S. B., and Garli, S. (2021). Apigenin alleviates methotrexate-induced liver and kidney injury in mice. Hum. Exp. Toxicol. 40 (10), 1721–1731. doi:10.1177/09603271211009964
Salari, N., Faraji, F., Jafarpour, S., Faraji, F., Rasoulpoor, S., Dokaneheifard, S., et al. (2022). Anti-cancer activity of chrysin in cancer therapy: a systematic review. Indian J. Surg. Oncol. 13 (4), 681–690. doi:10.1007/s13193-022-01550-6
Salehi, B., Venditti, A., Sharifi-Rad, M., Kręgiel, D., Sharifi-Rad, J., Durazzo, A., et al. (2019). The therapeutic potential of apigenin. Int. J. Mol. Sci. 20 (6), 1305. doi:10.3390/ijms20061305
Sánchez-López, E., Gomes, D., Esteruelas, G., Bonilla, L., Lopez-Machado, A. L., Galindo, R., et al. (2020). Metal-based nanoparticles as antimicrobial agents: an overview. Nanomater. (Basel) 10 (2), 292. doi:10.3390/nano10020292
Saquib, Q., Siddiqui, M. A., Ahmad, J., Ansari, S. M., Faisal, M., Wahab, R., et al. (2018). Nickel oxide nanoparticles induced transcriptomic alterations in HEPG2 cells. Adv. Exp. Med. Biol. 1048, 163–174. doi:10.1007/978-3-319-72041-8_10
Scholten, D., Trebicka, J., Liedtke, C., and Weiskirchen, R. (2015). The carbon tetrachloride model in mice. Lab. Anim. 49 (1 Suppl. l), 4–11. doi:10.1177/0023677215571192
Sethy, C., and Kundu, C. N. (2021). 5-Fluorouracil (5-FU) resistance and the new strategy to enhance the sensitivity against cancer: implication of DNA repair inhibition. Biomed. Pharmacother. 137, 111285. doi:10.1016/j.biopha.2021.111285
Seydi, E., Rasekh, H. R., Salimi, A., Mohsenifar, Z., and Pourahmad, J. (2016). Selective toxicity of apigenin on cancerous hepatocytes by directly targeting their mitochondria. Anticancer Agents Med. Chem. 16 (12), 1576–1586. doi:10.2174/1871520616666160425110839
Sheibani, M., Azizi, Y., Shayan, M., Nezamoleslami, S., Eslami, F., Farjoo, M. H., et al. (2022). Doxorubicin-induced cardiotoxicity: an overview on pre-clinical therapeutic approaches. Cardiovasc Toxicol. 22 (4), 292–310. doi:10.1007/s12012-022-09721-1
Shetty, A., Cho, W., Alazawi, W., and Syn, W. K. (2017). Methotrexate hepatotoxicity and the impact of nonalcoholic fatty liver disease. Am. J. Med. Sci. 354 (2), 172–181. doi:10.1016/j.amjms.2017.03.014
Shi, T., Zhuang, R., Zhou, H., Wang, F., Shao, Y., and Cai, Z. (2015). Effect of apigenin on protein expressions of PPARs in liver tissues of rats with nonalcoholic steatohepatitis. Zhonghua Gan Zang Bing Za Zhi 23 (2), 124–129. doi:10.3760/cma.j.issn.1007-3418.2015.02.010
Singh, D., Khan, M. A., Mishra, D., Goel, A., Ansari, M. A., Akhtar, K., et al. (2024). Apigenin enhances sorafenib anti-tumour efficacy in hepatocellular carcinoma. Transl. Oncol. 43, 101920. doi:10.1016/j.tranon.2024.101920
Singh, P., and Lim, B. (2022). Targeting apoptosis in cancer. Curr. Oncol. Rep. 24 (3), 273–284. doi:10.1007/s11912-022-01199-y
Şirin, N., Elmas, L., Seçme, M., and Dodurga, Y. (2020). Investigation of possible effects of apigenin, sorafenib and combined applications on apoptosis and cell cycle in hepatocellular cancer cells. Gene 737, 144428. doi:10.1016/j.gene.2020.144428
Smith, B. K., Marcinko, K., Desjardins, E. M., Lally, J. S., Ford, R. J., and Steinberg, G. R. (2016). Treatment of nonalcoholic fatty liver disease: role of AMPK. Am. J. Physiol. Endocrinol. Metab. 311 (4), E730-E740–e740. doi:10.1152/ajpendo.00225.2016
Spiegel, M., and Sroka, Z. (2023). Quantum-mechanical characteristics of apigenin: antiradical, metal chelation and inhibitory properties in physiologically relevant media. Fitoterapia 164, 105352. doi:10.1016/j.fitote.2022.105352
Sun, T., Li, S., Li, X., Lei, Y., Wang, B., Liu, X., et al. (2024). Apigenin intervenes in liver fibrosis by regulating PKM2-HIF-1α mediated oxidative stress. Biochem. Biophys. Res. Commun. 721, 150130. doi:10.1016/j.bbrc.2024.150130
Szabo, G., and Petrasek, J. (2015). Inflammasome activation and function in liver disease. Nat. Rev. Gastroenterol. Hepatol. 12 (7), 387–400. doi:10.1038/nrgastro.2015.94
Tang, D., Chen, K., Huang, L., and Li, J. (2017). Pharmacokinetic properties and drug interactions of apigenin, a natural flavone. Expert Opin. Drug Metab. Toxicol. 13 (3), 323–330. doi:10.1080/17425255.2017.1251903
Telange, D. R., Patil, A. T., Pethe, A. M., Fegade, H., Anand, S., and Dave, V. S. (2017). Formulation and characterization of an apigenin-phospholipid phytosome (APLC) for improved solubility, in vivo bioavailability, and antioxidant potential. Eur. J. Pharm. Sci. 108, 36–49. doi:10.1016/j.ejps.2016.12.009
Teng, Z., Yuan, C., Zhang, F., Huan, M., Cao, W., Li, K., et al. (2012). Intestinal absorption and first-pass metabolism of polyphenol compounds in rat and their transport dynamics in Caco-2 cells. PLoS One 7 (1), e29647. doi:10.1371/journal.pone.0029647
Tsalkidou, E. G., Tsaroucha, A. K., Chatzaki, E., Lambropoulou, M., Papachristou, F., Trypsianis, G., et al. (2014). The effects of apigenin on the expression of Fas/FasL apoptotic pathway in warm liver ischemia-reperfusion injury in rats. Biomed. Res. Int. 2014, 157216. doi:10.1155/2014/157216
Tsaroucha, A. K., Tsiaousidou, A., Ouzounidis, N., Tsalkidou, E., Lambropoulou, M., Giakoustidis, D., et al. (2016). Intraperitoneal administration of apigenin in liver ischemia/reperfusion injury protective effects. Saudi J. Gastroenterol. 22 (6), 415–422. doi:10.4103/1319-3767.195556
Unsal, V., Cicek, M., and Sabancilar, İ. (2021). Toxicity of carbon tetrachloride, free radicals and role of antioxidants. Rev. Environ. Health 36 (2), 279–295. doi:10.1515/reveh-2020-0048
Üsküdar Cansu, D., Öztaş, E., Yilmaz, E., and Korkmaz, C. (2019). Cyclophosphamide-induced severe acute hepatitis in a rheumatic disease: case-based review. Rheumatol. Int. 39 (2), 377–385. doi:10.1007/s00296-018-4189-8
van den Anker, J., Reed, M. D., Allegaert, K., and Kearns, G. L. (2018). Developmental changes in pharmacokinetics and pharmacodynamics. J. Clin. Pharmacol. 58 (Suppl. 10), S10-S25–s25. doi:10.1002/jcph.1284
van der Zanden, S. Y., Qiao, X., and Neefjes, J. (2021). New insights into the activities and toxicities of the old anticancer drug doxorubicin. Febs J. 288 (21), 6095–6111. doi:10.1111/febs.15583
Vogel, A., Meyer, T., Sapisochin, G., Salem, R., and Saborowski, A. (2022). Hepatocellular carcinoma. Lancet 400 (10360), 1345–1362. doi:10.1016/s0140-6736(22)01200-4
Vrhovac Madunić, I., Madunić, J., Antunović, M., Paradžik, M., Garaj-Vrhovac, V., Breljak, D., et al. (2018). Apigenin, a dietary flavonoid, induces apoptosis, DNA damage, and oxidative stress in human breast cancer MCF-7 and MDA MB-231 cells. Naunyn Schmiedeb. Arch. Pharmacol. 391 (5), 537–550. doi:10.1007/s00210-018-1486-4
Walker, D. K. (2004). The use of pharmacokinetic and pharmacodynamic data in the assessment of drug safety in early drug development. Br. J. Clin. Pharmacol. 58 (6), 601–608. doi:10.1111/j.1365-2125.2004.02194.x
Wan, L., Guo, C., Yu, Q., Li, Y., Wang, X., Wang, X., et al. (2007). Quantitative determination of apigenin and its metabolism in rat plasma after intravenous bolus administration by HPLC coupled with tandem mass spectrometry. J. Chromatogr. B Anal. Technol. Biomed. Life Sci. 855 (2), 286–289. doi:10.1016/j.jchromb.2007.05.007
Wang, B. Y. (2018). The promotion effect of apigenin on the differentiation of SMMC-7721 cells induced by mouse embryonic hepatocytes.
Wang, C., Ma, C., Gong, L., Dai, S., and Li, Y. (2021a). Preventive and therapeutic role of betaine in liver disease: a review on molecular mechanisms. Eur. J. Pharmacol. 912, 174604. doi:10.1016/j.ejphar.2021.174604
Wang, C., Ma, C., Gong, L., Guo, Y., Fu, K., Zhang, Y., et al. (2021b). Macrophage polarization and its role in liver disease. Front. Immunol. 12, 803037. doi:10.3389/fimmu.2021.803037
Wang, C., Wu, R., Zhang, S., Gong, L., Fu, K., Yao, C., et al. (2023). A comprehensive review on pharmacological, toxicity, and pharmacokinetic properties of phillygenin: current landscape and future perspectives. Biomed. Pharmacother. 166, 115410. doi:10.1016/j.biopha.2023.115410
Wang, E., Chen, F., Hu, X., and Yuan, Y. (2014). Protective effects of apigenin against furan-induced toxicity in mice. Food Funct. 5 (8), 1804–1812. doi:10.1039/c4fo00038b
Wang, F., Liu, J. C., Zhou, R. J., Zhao, X., Liu, M., Ye, H., et al. (2017). Apigenin protects against alcohol-induced liver injury in mice by regulating hepatic CYP2E1-mediated oxidative stress and PPARα-mediated lipogenic gene expression. Chem. Biol. Interact. 275, 171–177. doi:10.1016/j.cbi.2017.08.006
Wang, F., Zhou, R. J., Zhao, X., Ye, H., and Xie, M. L. (2018). Apigenin inhibits ethanol-induced oxidative stress and LPS-induced inflammatory cytokine production in cultured rat hepatocytes. J. Appl. Biomed., S1214021X1730162X.
Wang, L. (2022). Studies on the extraction, purification and oral delivery system of apigenin from Adinandra nitida Merr. ex H. L. Li.
Wang, S. M. (2020). Transcriptome studies on microRNAs of apigenin in the prevention and treatment of hepatocellular carcinoma.
Weber, L. W., Boll, M., and Stampfl, A. (2003). Hepatotoxicity and mechanism of action of haloalkanes: carbon tetrachloride as a toxicological model. Crit. Rev. Toxicol. 33 (2), 105–136. doi:10.1080/713611034
Wei, Y., Yang, P., Cao, S., and Zhao, L. (2018). The combination of curcumin and 5-fluorouracil in cancer therapy. Arch. Pharm. Res. 41 (1), 1–13. doi:10.1007/s12272-017-0979-x
Wen, H. B., Cao, Y. C., Dong, X. Y., Xu, J. H., and Cao, J. G. (2007). Induction of differentiation of HepG2 cells by apigenin. J. Hunan Norm. Univ. Med. Sci. (03), 6–9.
Wu, M. Y., Yiang, G. T., Liao, W. T., Tsai, A. P., Cheng, Y. L., Cheng, P. W., et al. (2018). Current mechanistic concepts in ischemia and reperfusion injury. Cell Physiol. Biochem. 46 (4), 1650–1667. doi:10.1159/000489241
Wu, Y. H., Ai, X. H., Dai, W. X., Wu, X. P., Tang, S. Y., and Jiang, H. (2009). Induction of apoptosis through up-regulating PTEN in human hepatocellular carcinoma HepG2 cell line by apigenin. China J. Mod. Med. 19 (12), 1793–1796.
Xia, S., Pan, Y., Liang, Y., Xu, J., and Cai, X. (2020). The microenvironmental and metabolic aspects of sorafenib resistance in hepatocellular carcinoma. EBioMedicine 51, 102610. doi:10.1016/j.ebiom.2019.102610
Xu, D., Xu, M., Jeong, S., Qian, Y., Wu, H., Xia, Q., et al. (2018). The role of Nrf2 in liver disease: novel molecular mechanisms and therapeutic approaches. Front. Pharmacol. 9, 1428. doi:10.3389/fphar.2018.01428
Yan, M., Huo, Y., Yin, S., and Hu, H. (2018). Mechanisms of acetaminophen-induced liver injury and its implications for therapeutic interventions. Redox Biol. 17, 274–283. doi:10.1016/j.redox.2018.04.019
Yang, J., Pi, C., and Wang, G. (2018). Inhibition of PI3K/Akt/mTOR pathway by apigenin induces apoptosis and autophagy in hepatocellular carcinoma cells. Biomed. Pharmacother. 103, 699–707. doi:10.1016/j.biopha.2018.04.072
Yang, J., Wang, X. Y., Xue, J., Gu, Z. L., and Xie, M. L. (2013). Protective effect of apigenin on mouse acute liver injury induced by acetaminophen is associated with increment of hepatic glutathione reductase activity. Food Funct. 4 (6), 939–943. doi:10.1039/c3fo60071h
Yang, M., Jiang, Z. H., Li, C. G., Zhu, Y. J., Li, Z., Tang, Y. Z., et al. (2018). Apigenin prevents metabolic syndrome in high-fructose diet-fed mice by Keap1-Nrf2 pathway. Biomed. Pharmacother. 105, 1283–1290. doi:10.1016/j.biopha.2018.06.108
Yue, S., Xue, N., Li, H., Huang, B., Chen, Z., and Wang, X. (2020). Hepatoprotective effect of apigenin against liver injury via the non-canonical NF-κB pathway in vivo and in vitro. Inflammation 43 (5), 1634–1648. doi:10.1007/s10753-020-01238-5
Zaefarian, F., Abdollahi, M. R., Cowieson, A., and Ravindran, V. (2019). Avian liver: the forgotten organ. Anim. (Basel) 9 (2), 63. doi:10.3390/ani9020063
Zarei, M., Karimi, E., Oskoueian, E., Es-Haghi, A., and Yazdi, M. E. T. (2021). Comparative study on the biological effects of sodium citrate-based and apigenin-based synthesized silver nanoparticles. Nutr. Cancer 73 (8), 1511–1519. doi:10.1080/01635581.2020.1801780
Zeng, J., Fan, J., and Zhou, H. (2023). Bile acid-mediated signaling in cholestatic liver diseases. Cell Biosci. 13 (1), 77. doi:10.1186/s13578-023-01035-1
Zhai, Y., Guo, S., Liu, C., Yang, C., Dou, J., Li, L., et al. (2013). Preparation and in vitro evaluation of apigenin-loaded polymeric micelles. Colloids Surfaces A Physicochem. Eng. Aspects 429 (Complete), 24–30. doi:10.1016/j.colsurfa.2013.03.051
Zhang D., D., Zhang, Y., and Sun, B. (2022). The molecular mechanisms of liver fibrosis and its potential therapy in application. Int. J. Mol. Sci. 23 (20), 12572. doi:10.3390/ijms232012572
Zhang, J., Liang, X., Li, J., Yin, H., Liu, F., Hu, C., et al. (2020). Apigenin attenuates acetaminophen-induced hepatotoxicity by activating AMP-activated protein kinase/carnitine palmitoyltransferase I pathway. Front. Pharmacol. 11, 549057. doi:10.3389/fphar.2020.549057
Zhang, M., and Zhang, S. (2020). T cells in fibrosis and fibrotic diseases. Front. Immunol. 11, 1142. doi:10.3389/fimmu.2020.01142
Zhang, W., Zhuang, X., Wu, C., Jin, Y., Xing, J., Hou, M., et al. (2024). Apigenin inhibits tumor angiogenesis by hindering microvesicle biogenesis via ARHGEF1. Cancer Lett. 596, 216961. doi:10.1016/j.canlet.2024.216961
Zhang, X., Qi, W., Xu, Q., Li, X., Zhou, L., and Ye, L. (2022). Di(2-ethylhexyl) phthalate (DEHP) and thyroid: biological mechanisms of interference and possible clinical implications. Environ. Sci. Pollut. Res. Int. 29 (2), 1634–1644. doi:10.1007/s11356-021-17027-y
Zhang, X. M. (2020). Effect of apigenin on proliferation and lipid metabolism of hepatocellular carcinoma and its molecular mechanism.
Zhang, Y., Zhu, R., Wang, J., Cui, Z., Wang, Y., and Zhao, Y. (2019). Upregulation of lncRNA H19 promotes nasopharyngeal carcinoma proliferation and metastasis in let-7 dependent manner. Artif. Cells Nanomed Biotechnol. 47 (1), 3854–3861. doi:10.1080/21691401.2019.1669618
Zhao, L., Zhang, J., Hu, C., Wang, T., Lu, J., Wu, C., et al. (2020). Apigenin prevents acetaminophen-induced liver injury by activating the SIRT1 pathway. Front. Pharmacol. 11, 514. doi:10.3389/fphar.2020.00514
Zheng, S., Cao, P., Yin, Z., Wang, X., Chen, Y., Yu, M., et al. (2021). Apigenin protects mice against 3,5-diethoxycarbonyl-1,4-dihydrocollidine-induced cholestasis. Food Funct. 12 (5), 2323–2334. doi:10.1039/d0fo02910f
Zhou, Q., Wang, X. J., Li, J., Wu, Y. R., Wang, W., Yu, Z. Y., et al. (2023). Self-assembly and interaction mechanisms of edible dock protein and flavonoids regulated by the phenolic hydroxyl position. Food Chem. 424, 136383. doi:10.1016/j.foodchem.2023.136383
Zhou, R. J., Ye, H., Wang, F., Wang, J. L., and Xie, M. L. (2017). Apigenin inhibits d-galactosamine/LPS-induced liver injury through upregulation of hepatic Nrf-2 and PPARγ expressions in mice. Biochem. Biophys. Res. Commun. 493 (1), 625–630. doi:10.1016/j.bbrc.2017.08.141
Zhou, R. J., Zhao, Y., Fan, K., and Xie, M. L. (2020). Protective effect of apigenin on d-galactosamine/LPS-induced hepatocellular injury by increment of Nrf-2 nucleus translocation. Naunyn Schmiedeb. Arch. Pharmacol. 393 (6), 929–936. doi:10.1007/s00210-019-01760-w
Zhou, W. (2021). Role of MOB2 in regulation of the migration and invasion of human hepatocellular carcinoma cells via Hippo signaling pathway and the mechanism of apigenin intervention.
Zhu, L., and Chen, L. (2019). Progress in research on paclitaxel and tumor immunotherapy. Cell Mol. Biol. Lett. 24, 40. doi:10.1186/s11658-019-0164-y
Zielińska, A., Carreiró, F., Oliveira, A. M., Neves, A., Pires, B., Venkatesh, D. N., et al. (2020). Polymeric nanoparticles: production, characterization, toxicology and ecotoxicology. Molecules 25 (16), 3731. doi:10.3390/molecules25163731
Glossary
API apigenin
APAP acetaminophen
NAFLD non-alcoholic fatty liver disease
ALD alcoholic liver disease
HCC hepatocellular carcinoma
Nrf2 nuclear factor erythroid 2-related factor 2
NF-κB nuclear factor kappa-B
PI3K phosphatidylinositide 3-kinase
Akt protein kinase B
mTOR mammalian target of rapamycin
NLRP3 NOD-like receptor family pyrin domain containing 3
FXR farnesoid X receptor
TGF-β1 transforming growth factor-β1
Smad3 mothers against decapentaplegic homolog 3
PPARα peroxisome proliferator-activated receptor alpha
APAP acetaminophen
NAPQI N-acetyl-p-benzoquinone imine
GR glutathione reductase
GSH glutathione
MTX methotrexate
ALT alanine aminotransferase
AST aspartate aminotransferase
CAT catalase
ALP alkaline phosphatase
GSH-Px glutathione peroxidase
SOD superoxide dismutase
TNF-α tumor necrosis factor α
IL-1β/6 interleukin-1β/6
MDA malondialdehyde
CYC cyclophosphamide;
ROS reactive oxygen species
LPO lipid peroxidation
MPO myeloperoxidase
Bax Bcl-2-associated X
Bcl-2 B-cell lymphoma-2
Fu Furan
CCl4 carbon tetrachloride
DEHP Di (2-ethylhexyl) phthalate
PbAc lead acetate
NDEA N-nitrosodiethylamine
LPS lipopolysaccharide
D-GalN D-Galactosamine
I/R Ischemia-reperfusion
NiONPs Nickel oxide nanoparticles
PA palmitic acid
NASH nonalcoholic steatohepatitis
OA oleic acid
XO xanthine oxidase
CTSB cathepsin B
AMPK AMP-activated protein kinase
FAS fatty acid synthase
JAK2 Janus kinase 2
STAT3 signal transducer and activator of transcription 3
TC total cholesterol
TG triglyceride
ECM extracellular matrix
HSCs hepatic stellate cells
BDL bile duct ligation
MMP2/8 matrix metalloproteinase 2/8
TIMP1 tissue inhibitors of metalloproteinase 1
iNOS inducible nitric oxide synthase
NO nitric oxide
ERK extracellular signal-regulated kinase
H2O2 hydrogen peroxide
TLR4 Toll-like receptor4
DDC 3,5-diethoxycarbonyl-1,4-dihydro-2,4,6-trimethylpyridine
MAPK mitogen-activated protein kinases
IFN-I type I interferon
miRNAs microRNA
SHP-1 Src homology 2 domain-containing protein tyrosine phosphatase 1
EVs extracellular vesicles
EMT epithelial-mesenchymal transition
SOR sorafenib
DOX doxorubicin
PTX paclitaxel
CDDP cisplatin
5-FU 5-Fluorouracil
CHR chrysin
HPLC-MS/MS high-performance liquid chromatography-tandem mass spectrometry
UPLC-MS/MS ultra-performance liquid chromatography-tandem mass spectrometry
DEN diethylnitrosamine
Keywords: apigenin, liver disease, hepatoprotection, toxicity, pharmacokinetics, new formulations
Citation: Wang C, Feng X, Li W, Chen L, Wang X, Lan Y, Tang R, Jiang T, Zheng L and Liu G (2024) Apigenin as an emerging hepatoprotective agent: current status and future perspectives. Front. Pharmacol. 15:1508060. doi: 10.3389/fphar.2024.1508060
Received: 08 October 2024; Accepted: 04 December 2024;
Published: 19 December 2024.
Edited by:
Shang-Gao Liao, Guizhou Medical University, ChinaReviewed by:
Xinyu Wang, Philadelphia College of Osteopathic Medicine (PCOM), United StatesPhiwayinkosi V. Dludla, South African Medical Research Council, South Africa
Tzong-Shyuan Lee, National Taiwan University, Taiwan
Copyright © 2024 Wang, Feng, Li, Chen, Wang, Lan, Tang, Jiang, Zheng and Liu. This is an open-access article distributed under the terms of the Creative Commons Attribution License (CC BY). The use, distribution or reproduction in other forums is permitted, provided the original author(s) and the copyright owner(s) are credited and that the original publication in this journal is cited, in accordance with accepted academic practice. No use, distribution or reproduction is permitted which does not comply with these terms.
*Correspondence: Ting Jiang, dGluZ2ppYW5ndGluYUAxNjMuY29t; Lingli Zheng, emhlbmdsaW5nbGlAY21jLmVkdS5jbg==; Gang Liu, Y3lmeWdjcEAxNjMuY29t
†These authors have contributed equally to this work