- 1Department of Stomatology, Chengdu Fifth People’s Hospital/The Second Clinical Medical College, Chengdu University of TCM, Chengdu, Sichuan, China
- 2Department of Orthodontics, State Key Laboratory of Oral Diseases, West China School of Stomatology, Sichuan University, Chengdu, Sichuan, China
Neprilysin (NEP), a zinc-dependent membrane-bound metallopeptidase, regulates various bioactive peptides, particularly in kidneys, vascular endothelium, and the central nervous system. NEP’s involvement in metabolizing natriuretic peptides, insulin, and enkephalins makes it a promising target for treating cardiovascular and Alzheimer’s diseases. Several NEP inhibitors, such as sacubitril and omapatrilat, have been approved for clinical use, which inhibit NEP activity to prolong the bioactivity of beneficial peptides, thereby exerting therapeutic effects. However, despite the broad clinical application prospects of NEP inhibitors, they still have specific adverse reactions and side effects, such as hypotension, renal impairment, and a potentially increased risk of Alzheimer’s disease. This manuscript comprehensively reviews the progress on single-target and dual-target NEP inhibitors. Dual-target inhibitors often combine with other therapeutic targets, such as angiotensin receptors, to enhance therapeutic effects and reduce adverse reactions. The article also emphasizes these inhibitors' design strategies, structure-activity relationships (SAR), safety, and clinical performance.
1 Introduction
Neprilysin (EC 3.4.24.11, neutral endopeptidase, NEP), is widely found in tissues such as the central nervous system, kidneys, and vascular endothelium (Turner et al., 2001). NEP can degrade a diverse range of peptide substrates such as insulin, enkephalins, substance P, endothelin-1 (ET-1), and amyloid-β (Kerr and Kenny, 1974a; Skidgel et al., 1984; Howell et al., 1995). It cleaves these substrates at multiple sites, favoring the amino side of hydrophobic residues (Southwood et al., 1975). The extensive presence of NEP, coupled with its capacity to interact with various substrates, highlights its critical involvement in the physiological processes of the cardiovascular, renal, gastrointestinal, and neurological systems.
Beyond its broad distribution, NEP is crucial for processing and breaking down vasoactive peptides involved in diuresis and natriuresis. Essential peptides include natriuretic peptides (NP), angiotensin I (Ang I), angiotensin II (Ang II), adrenomedullin (ADM), bradykinin (BK), neurokinin A, neuropeptide Y, substance P, and ET-1 (Dorofeyeva, 1975; Stephenson and Kenny, 1987; Abassi et al., 1992; Jiang et al., 2004; Mangiafico et al., 2013).
Neprilysin (NEP) plays a crucial role in the regulation of cardiovascular and inflammatory processes by degrading various bioactive peptides, including angiotensin-(1-7) [Ang-(1-7)]. Ang-(1-7), which is converted from Ang II by angiotensin-converting enzyme 2 (ACE2), has vasodilatory and anti-inflammatory effects, opposing the vasoconstrictive and pro-inflammatory actions of Ang II. Inhibition of NEP can increase the levels of Ang-(1-7), thereby enhancing its protective effects on the cardiovascular system, such as lowering blood pressure, reducing cardiac remodeling, and suppressing inflammatory responses (Mukhomedzyanov et al., 2024). This mechanism is particularly important for the treatment of diseases like heart failure and hypertension (Medina and Arnold, 2019). Therefore, NEP inhibitors, by enhancing the activity of the ACE2/Ang-(1-7) axis, help to mitigate the impact of cardiovascular diseases and inflammatory events (Derkachev et al., 2024). NEP’s activity extends beyond the cardiovascular system, influencing neurological processes, pain, inflammation, mitosis, angiogenesis, and digestion (Jaffe et al., 2015; Riddell and Vader, 2017). Therefore, NEP has appeared as a potentially beneficial target for treating many diseases.
Neprilysin inhibitors (NEPI) can influence the metabolism of multiple peptides; previous studies have confirmed that inhibiting NEP can elevate the concentration of these peptides, improving the therapeutic effects for patients with chronic heart failure (Bayes-Genis et al., 2016). Moreover, NEPI is correlated with Alzheimer’s disease, cardiovascular diseases, arthritis, and other conditions. For example, inhibiting NEP activity can boost glucose-stimulated insulin secretion in isolated islet tissues from C57BL/6 mice (Esser et al., 2018) and promote skeletal growth through the CNP/NPR-B pathway (Hakata et al., 2024). In high-fat-fed NEP-deficient mice, improvements in insulin sensitivity, β-cell function, glucose tolerance, and β-cell proliferation have been observed (Willard et al., 2017; Parilla et al., 2018). Furthermore, Alzheimer’s disease (AD) is a heterogeneous neurodegenerative disorder marked by intracellular tau protein accumulation and extracellular β-amyloid (Aβ) deposition. Research indicates that NEP is one of the essential metalloproteases involved in the clearance of Aβ (Grimm et al., 2014), and its presynaptic neuronal localization aids in Aβ clearance. Thus, NEPIs may accelerate AD neuropathology by inhibiting the Aβ clearance pathway.
In recent years, efforts have been devoted to developing highly efficient and specific NEPIs, with some achieving promising results. Dual-target NEPIs, such as omapatrilat and fasidotril, have beneficial effects in treating cardiovascular diseases (C Marie et al., 1995; Sharma et al., 2020). However, the adverse reactions of NEPI also warrant attention. Some animal studies suggest potential negative impacts on cognitive function from NEPI, along with mild side effects such as vomiting, drowsiness, dizziness, and edema (Moreau et al., 2005). This paper reviews the progress in NEPI research, detailing the design strategies, structure-activity relationships (SAR), and bioactivity of single-target and dual-target NEPIs.
2 The structural and biological function of NEP and NEPI
2.1 The structure of NEP
NEP, a type II membrane-bound enzyme, comprises 749 amino acid residues and relies on zinc for its catalytic activity as an endopeptidase (Kerr and Kenny, 1974b; Skidgel et al., 1984; Howell et al., 1995). Its extracellular domain, part of the peptidase M13 family, features two α-helical structures forming a spherical, water-filled cleft that houses the catalytic site (Campbell, 2017). This domain resembles the bacterial protease thermolysin and contains a zinc atom essential for its catalytic activity, coordinated by histidine (His) and glutamate (Glu) residues. NEP also includes a transmembrane domain and a short intracellular domain. Located on the plasma membrane, NEP has three domains: a short intracellular domain (27 residues), a transmembrane domain (23 residues), and a larger extracellular catalytic domain (699 residues) (Moss et al., 2020). The extracellular region features a central hollow space that houses a key zinc-binding sequence, HEXXH, wherein a pair of histidine molecules anchor the zinc atom. At the same time, a glutamate residue is involved in the catalytic process (Moss et al., 2018).
The extracellular region of NEP is ellipsoidal, with major and minor axes measuring 86 and 60 nm. It has a predominantly α-helical secondary structure, divided into two large subdomains connected by a smaller linker region comprising four α-helical segments (Figure 1A). Subdomain one contains the N-terminal residues, while subdomain two contains the C-terminal residues, creating a central cavity. Four N-linked glycosylation sites are at positions N144, N284, and N324 in subdomain two and N627 in subdomain 1, with a single N-acetylglucosamine residue modeled at each site (Oefner et al., 2000a).
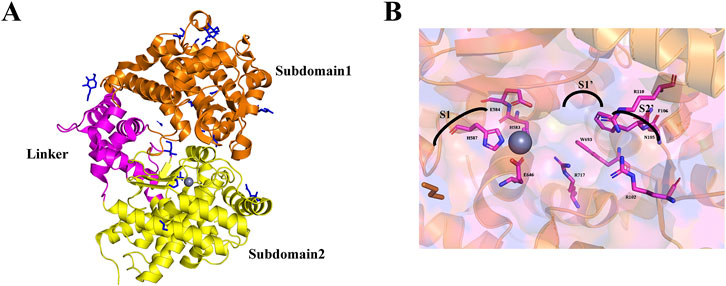
Figure 1. (A) The 3D ribbon diagram of the extracellular domain of NEP. The extracellular domain is further split into two subdomains colored orange for subdomain 1, yellow for subdomain 2, and pink for the linker region. (B) NEP active site binding pocket labeled with subsites and residues.
The catalytic site within subdomain 1’s cavity surface centers around a zinc ion and the conserved HEXXH motif. The zinc ion is coordinated by residues H583 (2.02 Å), H587 (2.10 Å), and E646 (1.93 Å), with E584 contributing to the catalytic mechanism and completing the coordination sphere. This forms a binding pocket with sub-sites S1, S1', and S2'. Inhibitors targeting NEP reveal the characteristics of these sub-sites (Figure 1B). The S1 site has minimal impact on binding affinity and exhibits relaxed specificity (Oefner et al., 2000b). The S1' sub-site forms a hydrophobic pocket for large hydrophobic and aromatic side chains (Tiraboschi et al., 1999), explaining NEP’s preference for cleaving at hydrophobic residues. The S2' sub-site can accommodate bulky side chains and shows relaxed specificity (Dion et al., 1997).
2.2 The biological functions of NEP
NEP belongs to the M13 peptidase family on chromosome 3q25.2 (Polna and Aleksandrowicz, 1975; Schiering et al., 2016). NEP has 749 amino acids and three main domains: a short amino-terminal cytoplasmic domain, a single transmembrane helical domain, and a carboxy-terminal extracellular domain containing a zinc-binding active site. These domains enable the enzyme’s catalytic activity when substrates attach to the extracellular domain (Emoto and Yanagisawa, 1995). NEP is an enzyme specializing in cleaving peptides with a molecular weight that generally does not exceed 3,000 Da. It does not act on larger proteins with a molecular weight well above this threshold. The limited access to the catalytic cleft determines the enzyme’s size-specific activity, which only allows substrates of a specific size to enter (Figure 2) (Oefner et al., 2000a). NEP catalyzes the hydrolysis of peptide bonds at the amino side of hydrophobic residues, with a particular affinity for phenylalanine or leucine at the P1 position. It cleaves the substrate at the carboxyl terminus, releasing dipeptides or tripeptides (Campbell, 2017). NEP catalyzes the hydrolysis of hydrophobic amino acids located at the N-terminus of peptides, showing a particular affinity for phenylalanine and leucine.
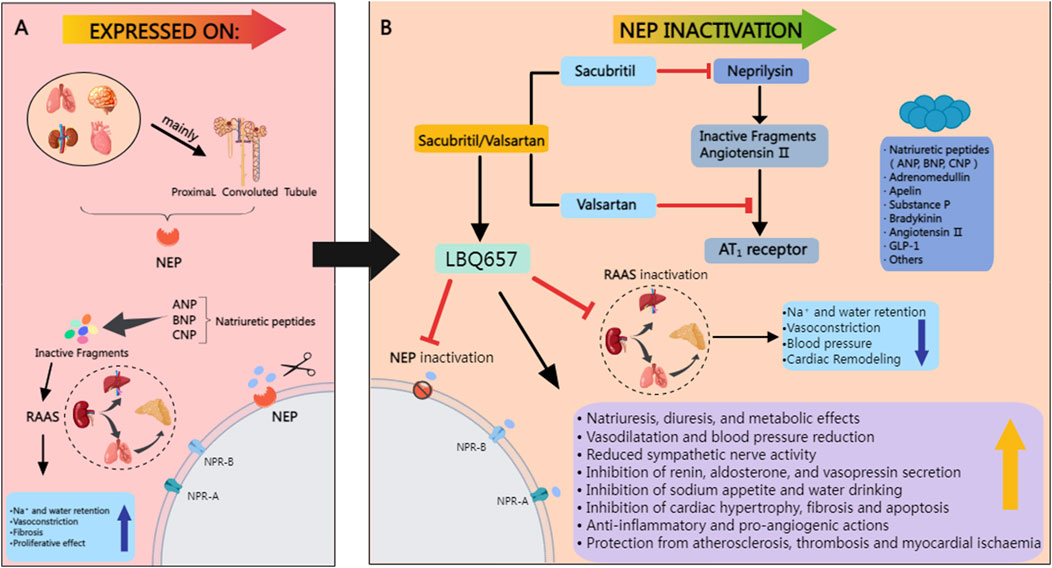
Figure 2. (A) NEP is abundantly present in various tissues, including the lungs, blood vessels, heart, brain, and kidneys, with the highest levels in the proximal tubules of the kidneys. It breaks down natriuretic peptides like ANP, BNP, and CNP, which inactivates the RAAS and promotes fluid retention. While beneficial for blood pressure regulation, this process raises the risk of cardiac metabolic problems due to its vasoconstrictive and antiproliferative effects. (B) It lowers arterial blood pressure by enhancing sodium excretion, promoting diuresis, and inducing blood vessel dilation, while also strengthening the anti-proliferative effects on heart remodeling, thus amplifying the hemodynamic effects of natriuretic peptides (NP). Red lines denote inhibitory actions. MedPeer. (2024). MedPeer Scientific Illustration System [Computer software]. Retrieved from http://www.medpeer.cn.
In addition to its broad distribution, neprilysin is instrumental in the metabolism and processing of various vasoactive peptides that are pivotal for diuresis and natriuresis. Key among these peptides are NP, Ang I, ADM, BK, neurokinin A, neuropeptide Y, substance P, and ET-1, all of which are subject to the enzyme’s catalytic action (Dorofeyeva, 1975; Stephenson and Kenny, 1987; Abassi et al., 1992; Jiang et al., 2004; Mangiafico et al., 2013). Neprilysin’s enzymatic function extends beyond the cardiovascular system; it also antagonizes a range of molecules integral to neurological functions, pain perception, inflammatory responses, cell division, blood vessel formation, and the digestive process (Jaffe et al., 2015; Riddell and Vader, 2017).
The blockade of two clearance mechanisms of NEP by ARNIs is a pivotal aspect of their therapeutic action. NEP, also known as neprilysin, is a membrane-bound metallopeptidase that plays a critical role in the degradation of various bioactive peptides, including atrial natriuretic peptide (ANP), brain natriuretic peptide (BNP), and substance P (Turner et al., 2001). The dual inhibition of NEP by ARNIs results in the blockade of two primary clearance pathways: the degradation of natriuretic peptides and the RAAS components (McMurray et al., 2014).
The first mechanism involves inhibiting natriuretic peptide degradation, leading to increased levels of these vasodilatory and antihypertrophic peptides, thereby enhancing their cardioprotective effects (Stuss, 2015). The second mechanism pertains to the blockade of the RAAS, where NEPI reduces the breakdown of angiotensin I and II, contributing to the attenuation of vasoconstriction and aldosterone release (Andersen et al., 1998).
2.3 The role of NEPI in diseases
NEPI affects the metabolism of various peptides, including amyloid peptides, corticotropin-releasing factor, luteinizing hormone-releasing hormone, oxytocin, and neurotensin (Bayes-Genis et al., 2016; Campbell, 2017). NEP plays a significant role in a variety of diseases affecting the natriuretic peptide system, the renin-angiotensin-aldosterone system (RAAS), and the kallikrein-kinin system (Domenig et al., 2016; Ponikowski et al., 2016). Previous studies have confirmed that inhibiting NEP can elevate these peptides, Improving chronic heart failure treatment outcomes (Campbell, 2017). NEPIs exert their effects by reducing the degradation of natriuretic peptides, thereby enhancing their bioactivity, which in turn promotes natriuresis, diuresis, vasodilation, inhibits cardiac hypertrophy and myocardial fibrosis, and delays the progression of heart failure (Pavo et al., 2020). Additionally, They may improve insulin and glucagon-like peptide-1 (GLP-1) levels by inhibiting the expression of dipeptidyl peptidase-4 (DPP4) (Pavo et al., 2020). Thus, by inhibiting NEP, the levels and functions of various bioactive peptides can be modulated, demonstrating potential biological functions and therapeutic potential in the treatment of a multitude of diseases.
2.3.1 Diabetes and its complications
Studies have revealed that inhibiting NEP activity enhances insulin secretion stimulated by glucose in islet tissues extracted from C57BL/6 mice (Esser et al., 2018). Furthermore, in vitro experiments showed that NEP-deficient mouse islet tissues are protected from insulin secretion dysfunction (Zraika et al., 2013). In vivo studies have shown that NEP-deficient mice fed a high-fat diet demonstrate enhanced insulin sensitivity, improved β-cell function, better glucose tolerance, and increased β-cell proliferation (Willard et al., 2017; Parilla et al., 2018). Study reveals that under acute physiological conditions, selectively inhibiting intestinal neprilysin boosts insulin secretion in response to oral glucose, and this impact is mediated through the GLP-1 receptor, thereby establishing a novel role for intestinal neprilysin in the regulation of beta-cell function (Esser et al., 2023). NEPIs are also effective in the management of type 2 diabetes mellitus (T2DM) by increasing the circulating level of GLP-1, which is degraded by NEP (Esser and Zraika, 2019). However, the influence of other enzymes, such as DPP-4 inhibitors, on NEP substrates can diminish the efficacy of NEPI or adversely affect insulin sensitivity and β-cell function (Esser et al., 2022). As a result, the levels of NEP substrates may rise, suggesting that a combined NEPI treatment approach for type 2 diabetes could be more efficacious (AlAnazi et al., 2023).
Diabetic nephropathy (DN) is recognized as a prevalent cause of end-stage renal disease, driven significantly by chronic hyperglycemia activating the renin-angiotensin system (RAS). Therefore, blocking RAS can help delay the progression of DN (Gallagher and Suckling, 2016). The persistent hyperactivation of the RAS is implicated in the progression of diabetic nephropathy. Concurrently, the natriuretic peptide system (NPS) is a counterbalancing negative feedback mechanism (Goru et al., 2017; Malek and Gaikwad, 2017). NEPI can enhance the bioavailability of natriuretic peptides, which may contribute to mitigating chronic kidney disease (CKD) progression (Lai et al., 2023). Clinically, urinary NEP levels are increased in diabetic patients, and NEPI can delay DN progression. Furthermore, research indicates that treatment with angiotensin receptor-neprilysin inhibitors (ARNIs) can lead to improvements in renal function, reduce glomerular and tubulointerstitial fibrosis, and weaken inflammation, pro-fibrotic and apoptotic signaling, ultimately delaying DN onset (Davis et al., 2022).
Diabetic retinopathy (DR) stands as the leading cause of vision loss among individuals with diabetes. DR is recognized as a progressive neurovascular condition affecting the retina, with its severity and progression closely linked to the duration of diabetes. Medications such as angiotensin receptor blockers (ARBs) and ARNIs have demonstrated the ability to significantly attenuate NEP activity (Dahrouj et al., 2013). Inhibiting retinal NEP activity and increasing retinal natriuretic peptide levels can improve DR treatment outcomes, and ARNIs can improve neurovascular lesions in the retinas of diabetic rats (Prasad et al., 2016).
Recent advancements in the field of diabetic complications have shed light on the potential therapeutic roles of neprilysin inhibitors, both as monotherapy and in combination with other agents, in the management of diabetic nephropathy (DKD) and diabetic cardiomyopathy (DCM). In a study, the combination of SGLT2 inhibitors with neprilysin inhibitors demonstrated promising results in improving glycemic control and reducing inflammation and oxidative stress, which are key factors in the progression of DKD (Tarun et al., 2024). Furthermore, the synergistic effects of these drugs on lowering glomerular pressure and proteinuria offer a novel approach to DKD management.
Residing on the cell surface of the corneal epithelium, where it remains undisturbed, NEP plays a pivotal role in controlling the chromatic interactions between corneal nerve fibers and the epithelial cells themselves through the modulation of peptides involved in these communications. Studies have shown that NEPI combined with topical ascorbic acid, citric acid, antibiotics, or steroids helps improve epithelial replanting on the corneal surface (Genova et al., 2018). Although a previous study has documented the presence of NEP in the human corneal epithelium, the exact physiological functions of this enzyme in this context have yet to be fully elucidated (Hasby and Saad, 2013).
2.3.2 Chronic kidney disease
In hypertensive animal models, long-term administration of omapatrilat has been observed to effectively halt the advancement of renal complications, including glomerulosclerosis, tubulointerstitial fibrosis, and overall kidney injury (Cao et al., 2001). In a 5/6 nephrectomy model, treatment with AVE 7688 has demonstrated significant efficacy in Mitigating proteinuria and ameliorating the severity of glomerulosclerosis and tubulointerstitial fibrosis (Benigni et al., 2004). AVE 7688 increased renal nitric oxide compounds, reduced endothelin-1 synthesis, decreased renal vascular contraction, and raised tubular ANP release (Benigni et al., 2004). In another 5/6 nephrectomy model, omapatrilat lowered systolic blood pressure and glomerular capillary pressure, reduced proteinuria, and mitigated glomerulosclerosis (Taal et al., 2001). Therefore, combined NEPI can improve CKD treatment outcomes.
2.3.3 Colitis
Ulcerative Colitis is an idiopathic chronic inflammatory condition that targets the colon’s mucosal lining, leading to relapsing and remitting symptoms. The absence or inhibition of NEP can exacerbate Clostridium difficile toxin A-induced intestinal inflammation (Sargın et al., 2017). Additionally, recombinant NEP can prevent inflammation in NEP knockout mice. A significant deficiency in NEP activity could contribute to a pro-inflammatory environment within the colonic mucosa (Ter Beek et al., 2007). The inflammatory state is linked to NEP and substance P (SP) loss. The pro-inflammatory action of low SP levels may be related to bioactive fragments. In UC, the metabolism of peptides that act as pathogenic agents is likely impaired due to a substantial decrease in the activity of the principal enzyme that catalyzes their hydrolysis.
Additional investigative efforts are warranted to elucidate the underlying reasons for the observed decline in peptide levels. Vu et al.'s research confirms Vasoactive Intestinal Peptide (VIP) as an anti-inflammatory agent, showing reduced DSS-induced Colitis in both VIP knockout mice and wild-type mice treated with VIP antagonists (Vu et al., 2014), NEP Deficiency might dampen VIP anti-inflammatory-action-by-affecting production of its active VPAC1-binding fragments (Pavlovic et al., 2011). In the colonic tissues of UC patients, the expression of SP and VIP significantly decreases, and NEP expression is markedly reduced.
2.3.4 Alzheimer’s disease
Alzheimer’s disease manifests as a neurodegenerative condition with hallmark pathologies: the intracellular presence of phosphorylated tau and the extracellular presence of β-amyloid plaques (Nalivaeva et al., 2020; Yiannopoulou and Papageorgiou, 2020). NEP is associated with the degradation and clearance of Aβ. NEP, a crucial metalloprotease, plays a significant role in the degradation and clearance of Aβ peptides (Grimm et al., 2014), and its presynaptic neuronal localization aids in Aβ removal (Iwata et al., 2004). In late-onset AD patients, there is a selective reduction in NEP mRNA expression and protein levels in brain areas. The decline in NEP levels and activity with age may contribute to late-onset Alzheimer’s disease in both humans and rodents (Iijima-Ando et al., 2008). Preclinical research suggests that the administration of NEPI may trigger Alzheimer ‘s-like pathologies in animal models (Liu et al., 2010). In mice (Poirier et al., 2006), NEPI exacerbates AD progression. A deficiency in NEP leads to impaired degradation of exogenous Aβ and disruptions in the metabolic regulation of endogenous Aβ levels. In the brains of NEP-deficient mice, Aβ levels are highest in the hippocampus, followed by the cortex. The thalamus/striatum, and lowest in the cerebellum, mirroring the pattern of vulnerability to Aβ deposition observed in the brains of AD patients (Liu et al., 2009; Liu et al., 2010; Pennant et al., 2014). Observations indicate (Marr and Spencer, 2010) that even a slight reduction in NEP activity can lead to AD development by facilitating the buildup of Aβ peptides. Studies (Ali et al., 2024) have suggested that NEPI might offer protective effects against the development of AD by increasing the levels of GLP-1, neuropeptide Y (NPY), and substance P. However, NEPI might contribute to AD development by increasing levels of BK and NPs (Bavishi et al., 2015). The contradictory findings on NEPIs' impact on AD progression are yet to be conclusively established in clinical settings.
2.3.5 Heart failure (HF)
HF represents the end stage of various cardiac diseases. Inhibiting the excessive activation of the neuroendocrine system is a crucial measure in the treatment of HF. However, traditional drug treatments based on angiotensin-converting enzyme inhibitors (ACEI) and angiotensin II receptor blockers (ARB) have been found to exhibit an “upper limit effect,” where the effectiveness of the drugs diminishes or is even lacking in some long-term users, leading to reduced clinical outcomes (Arundel et al., 2020). NEP plays a significant role in the pathogenesis and development of HF by affecting the natriuretic peptide system, the RAAS, and the kallikrein-kinin system (Domenig et al., 2016; Ponikowski et al., 2016). In 2006, the first ARNI—sacubitril/valsartan was introduced. Extensive clinical trials have confirmed that ARNI offers significant benefits in the treatment of heart failure with reduced ejection fraction (HFrEF), new-onset HF, and acute decompensated HF, as well as in improving prognosis (Seferovic et al., 2019). Furthermore, the apelin/label system plays a crucial role in the cardiovascular system including the regulation of vascular tension, blood pressure, and fluid homeostasis (Kuba et al., 2019). Recent studies have indicated that neprilysin can cleave and inactivate apelin peptides, suggesting that neprilysin may indirectly modulate the function of the apelin/label system by affecting the stability and activity of apelin peptides (Chapman et al., 2021).
3 NEP inhibitors
3.1 Single-target NEPIs
Candoxatril (Compound 1, UK79300, Figure 3A), developed by Pfizer, is a prodrug of Candoxatrilat (compound 2, UK73967, Figure 3A) when taken orally. Candoxatril is a novel orally active neutral endopeptidase inhibitor involving the peptide hormone atrial natriuretic factor (ANF) metabolic inactivation (O’Connell et al., 1992). During development, the introduction of chirality was most effectively achieved using a BINAP-based catalyst for asymmetric hydrogenation (Ager and De Vries, 2012). Candoxatril, a hormone composed of 28 amino acids, contributes to the maintenance of sodium and water balance within the body (O’Connell et al., 1992), and is well-tolerated in humans (Yandle et al., 1986; Ignatovich, 1975; Mukoyama et al., 1991; Cheung et al., 1994). Exogenous administration of atrial natriuretic peptide (ANP) results in natriuresis (Epstein et al., 1998; Gunning et al., 1992), vasodilation (Fujita et al., 1987; Edwards et al., 1988) and reduced plasma concentrations of renin and aldosterone (Steele et al., 1997), thereby alleviating symptoms of heart failure.
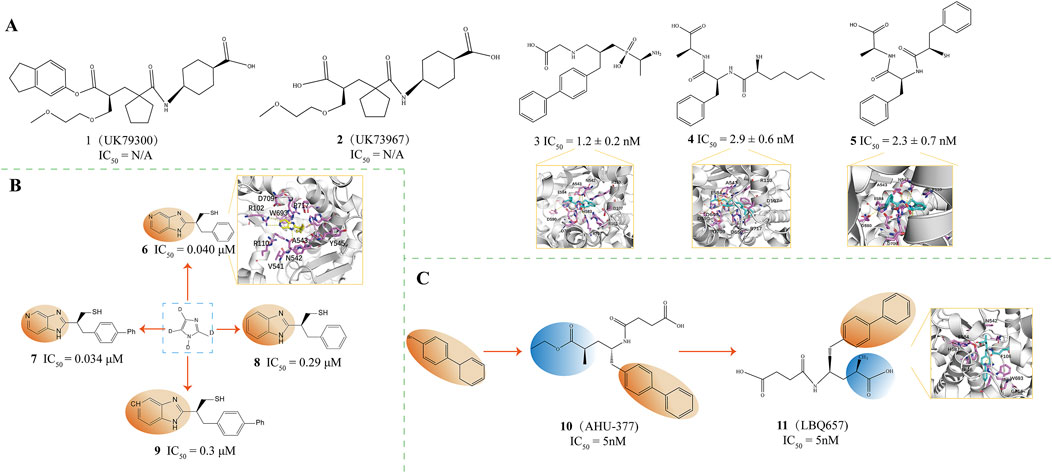
Figure 3. (A) NEP inhibitors. X-ray structure of NEP in complex with compound 3(PDB ID: 1R1H), compound 4(PDB ID: 1R1I), compound 5(PDB ID: 1R1J) (O’Connell et al., 1992; Oefner et al., 2004). (B) NEP inhibitors. X-ray structure of NEP in complex with compound 6(PDB ID: 1Y8J) (Sahli et al., 2005). (C) Structural Optimization of Compounds 10,11. Crystal structure of compound 11(PDB ID: 5JMY) (Flick et al., 2017; He et al., 2023).
Candoxatrilat is a neutral endopeptidase inhibitor that reduces ANP degradation in mild heart failure patients (Wilkins et al., 1992). Candoxatrilat and SC 46542 (an antagonist of ANP clearance receptors) inhibit two major mechanisms for clearing ANP - the enzyme E−24.11 and C-ANP receptors respectively. When administered alone at “physiological” plasma ANP levels, candoxatrilat does not significantly affect sodium excretion (Wilkins et al., 1992). The simultaneous blockade of two clearance mechanisms, namely, the enzyme E-24.11 and the C-ANP receptors, has yielded a more pronounced elevation of plasma ANP levels and a more robust natriuretic effect than inhibiting a single pathway (Polna and Aleksandrowicz, 1975; Rajcáni et al., 1975). Likewise, the clearance systems become more overwhelmed when plasma ANP levels are increased—whether through peptide infusion or under pathological states like heart failure. The capacity for one clearance mechanism to make up for the suppression of another is restricted, thereby highlighting the natriuretic capacity of candoxatrilat (Wilkins et al., 1992). Comparisons of the renal effects of candoxatrilat with low-dose and high-dose ANP infusions indicate different mechanisms for increasing sodium excretion, suggesting that the natriuretic response to ANP results from actions on both glomerular and tubular levels (Cogan, 1990). Researchers observed that the addition of candoxatrilat or SC 46542 to ANP at a dosage of 100 ng·kg-1·min-1, as well as the combined administration of candoxatrilat and SC 46542, led to an increase in urinary sodium and cyclic GMP excretion to levels similar to or higher than those achieved with ANP at 300 ng kg-1 min-1, however, these compounds were unable to replicate all the effects of high-dose ANP infusion (Chien et al., 1987; Trippodo and Barbee, 1987). These data suggest that candoxatrilat selectively amplifies the renal impact of ANP in conscious, normotensive rats, surpassing the effects achieved with medicinal dosages of ANP (Wilkins et al., 1992).
Oefner and colleagues investigated the interactions of stable inhibitors with NEP Through X-ray crystallography to understand enzyme inhibition patterns better. Among these, compound 3 (IC50 = 1.2 ± 0.2 nM, PDB ID = 1R1H, Figure 3A) was found to bind NEP with a zinc ion in an almost tetrahedral coordination geometry. The single O atom of the phosphonate group forms a tetrahedral bond with the zinc ion, maintaining a bond length of 1.94 Å (Oefner et al., 2004). Among these, compound 3 was found to bind NEP with a zinc ion in an almost tetrahedral coordination geometry. The single O atom of the phosphonate group forms a tetrahedral bond with the zinc ion, maintaining a bond length of 1.94 Å(Pauptit et al., 1988). The free amino terminus of this inhibitor contributes to the stabilization of the complex by forming a hydrogen bond with the carbonyl oxygen atom of the Ala543 backbone (Oefner et al., 2004). Introducing the free amino group into compound 3 yielded compound 4 (IC50 = 2.9 ± 0.6 nM, PDB ID = 1R1I, Figure 3A) and compound 5 (IC50 = 2.3 ± 0.7 nM, PDB ID = 1R1J, Figure 3A) (Chen et al., 2000). The rearrangement structures of NEP binary complexes with compounds 4 and 5 indicate that both interact with the Zn atom through bidentate coordination (Oefner et al., 2004). Compounds 8 and 9 display identical C-terminal extensions, which engage in similar molecular engagements with the enzyme, underscoring the supportive role of the S1 subsite in stabilization (Oefner et al., 2004). Overall, for all three compounds, the L-alanine residue at the carboxyl terminus is uniformly recognized by the amino acids within the S2' subsite, particularly reaching out to interact with the side chains of Arg102, Asp107, and Arg110 (Oefner et al., 2004).
Sahli and colleagues have developed a class of non-peptidic inhibitors that target the zinc-dependent metalloprotease neprilysin, using aromatic heterocycles as the core structure. These inhibitors demonstrate nanomolar inhibitory potency, with IC50 values in the nanomolar activity range (0.034 ± 0.30 mm) (Sahli et al., 2005). They investigated a pair of distinct synthetic pathways, ultimately identifying a superior approach distinguished by incorporating a thiol group via a Mitsunobu reaction as the culminating step in a complex sequence of reactions, which coordinates with the ZnII ion of the metalloprotease. This approach led to significantly improved binding affinity of the novel inhibitors for neprilysin (Sahli et al., 2005). Notably, inhibitors that have an imidazo [4,5-c]pyridine core, such as compound 6 (IC50 = 0.040 μM, PDB ID: 1Y8J, Figure 3B) and compound 7 (IC50 = 0.034 μM, Figure 3B), as well as those with a benzimidazole core, such as compounds 8 (IC50 = 0.29 μM, Figure 3B) and 9 (IC50 = 0.3 μM, Figure 3B), have shown promising activity (Sahli et al., 2005). Compound 6 demonstrated a high affinity for neprilysin, characterized by forming hydrogen bonds with amino acid residues Asn542 and Arg717 and beneficial π-π stacking interactions with the imidazole ring of His711 (Sahli et al., 2005).
Sacubitril (Compound 10, AHU-377, IC50 = 5 nM, Figure 3C) is a medication developed by Novartis, which functions as a combination of a neprilysin inhibitor and an ARB (Flick et al., 2017; He et al., 2023; Li et al., 2023; Smits et al., 2024). Sacubitril targets neprilysin, an enzyme degrading the plasma ANF. Sacubitril maintains endogenous ANF levels by inhibiting neprilysin, protecting the cardiac neuroendocrine system, which benefits heart failure patients. In animal models, sacubitril has increased sodium excretion in urine, indicating its diuretic effect (He et al., 2023).
LBQ657 (Compound 11, IC50 = 5 nM, PDB ID = 5JMY, Figure 3C) is an active neutral endopeptidase inhibitor. It is an active metabolite of the prodrug AHU-377 formed in vivo through esterase-mediated demethylation (Ksander et al., 1995). The LBQ657 molecule contains two chiral centers, the precise configurations enabling the most favorable interactions with the active site of neprilysin (Schiering et al., 2016). The carbonyl and amine Groups within the amide backbone of LBQ657 engage in hydrogen bonding with the asparagine side chains at position 542 and arginine at position 717 (Schiering et al., 2016). LBQ657 features an amide-NH linkage to the chiral carbon atom, representing a structural deviation from prior inhibitors that usually connect through the amide’s carbonyl oxygen. Despite this difference, the nitrogen and oxygen atoms in LBQ657 retain similar spatial orientations and interaction patterns within the complex as those observed in earlier compounds (Oefner et al., 2000b; Oefner et al., 2004; Sahli et al., 2005). The succinate in P2' has been optimized to place the carboxyl group in an ideal position to interact with Arg 102 and Arg 110, which is unique to LBQ657 (Ksander et al., 1995). By inhibiting NEP, LBQ657 increases the levels of certain peptide hormones (such as ANP and BNP) with vasodilatory and natriuretic effects in the body. These peptides activate guanylate cyclase through their receptors, increasing intracellular cyclic GMP levels, thereby exerting their physiological effects, including vasodilation, promoting urine excretion, and suppressing the RAAS (He et al., 2023).
3.2 Dual-target inhibitors
3.2.1 NEP and ACE dual-target inhibitors
Fasidotril (Compound 12, Figure 4A) is a diester prodrug developed by Bioproject. Its active metabolite, Fasidotrilat (Compound 13, Figure 4A), effectively inhibits angiotensin I-converting enzyme (ACE IC50 = 9.8 nM) and neutral endopeptidase (NEP IC50 = 5.1 nM) (C Marie et al., 1995). In heart failure models, long-term treatment with fasidotril improves myocardial hypertrophy and increases survival rates without lowering blood pressure (C Marie et al., 1995). Studies have shown that in clinical studies, fasidotril strongly affects renin-dependent and volume-dependent hypertension models and can sustainably reduce blood pressure (C Marie et al., 1995). Fasidotril inhibits NEP, which reduces the degradation of ANP and BNP, enhancing their natriuretic and vasodilatory actions, thereby helping to alleviate the symptoms of heart failure (Pathadka et al., 2021). Additionally, by blocking ACE, fasidotril decreases the production of Ang II, mitigating its vasoconstrictive and pro-inflammatory actions, and offering therapeutic benefits for hypertension and myocardial infarction (Tsutsui et al., 2021). This makes fasidotril a promising candidate for treating myocardial infarction and congestive heart failure.
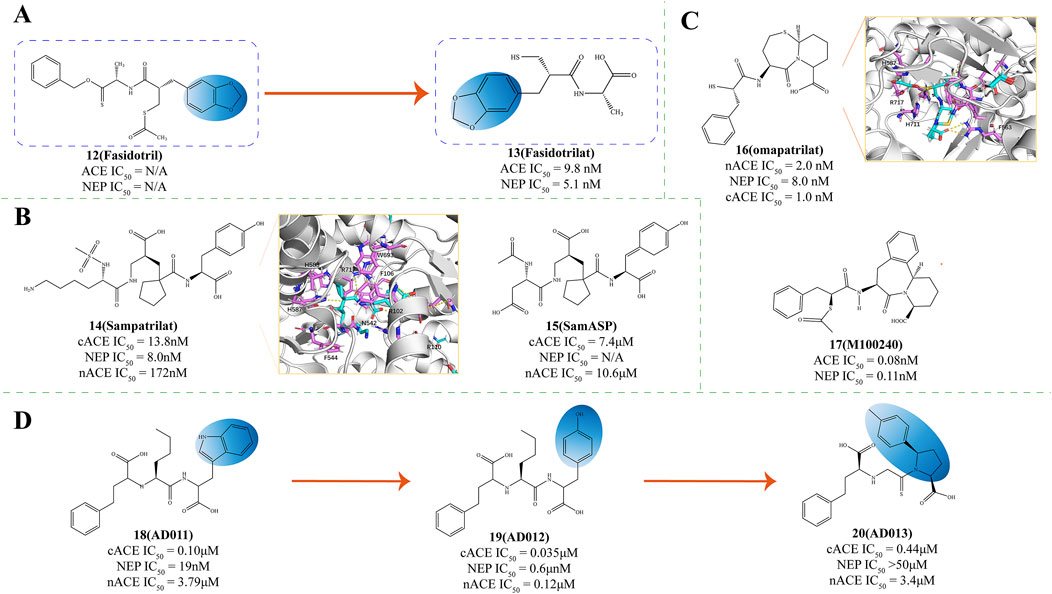
Figure 4. (A) Structural Optimization of Compounds 12,13 (C Marie et al., 1995). (B) Structures of NEP and ACE dual inhibitor (Wallis et al., 1998; Venn et al., 1998b; Venn et al., 1998a; Sharma et al., 2020). (C) Structures of NEP and ACE dual inhibitor. X-ray structure of NEP in complex with compound 16 (PDB ID: 6SUK) and compound 17 M100240 (Cozier et al., 2018; Rousso et al., 1999). (D) The structural optimization of ACE/NEP dual inhibitor (Oefner et al., 2000b; Arendse et al., 2022; Oefner et al., 2004; Sharma et al., 2020).
Sampatrilat (Compound 14, UK 81252, Figure 4B) functions as a dual-action inhibitor targeting both ACE and NEP and it holds promise for therapeutic use in managing conditions such as hypertension and congestive heart failure (Wallis et al., 1998; Venn et al., 1998b; Venn et al., 1998a). Given its dual action, sampatrilat may offer more excellent benefits in treating chronic heart failure than traditional ACE inhibitors (Norton et al., 1999).
SamASP (Compound 15, Figure 4B) interacts with NEP akin to sampatrilat, securing its position in the S2' and S1' subsites, the region surrounding the zinc ion, and extending its reach into the Non-prime subsites (Sharma et al., 2020). In the non-prime region, the secondary amide and aspartic acid-like side chains on the C5 position of SamASP offer a fitting interaction that aligns well with the enzyme’s binding requirements, with the carboxyl group of the tartaric ester located in the densest electron density regions. The secondary amide’s strategic placement enables its C1 and C2 atoms to engage in hydrophobic interactions with the Val-710 residue (Sharma et al., 2020).
Omapatrilat (Compound 16, Vanlev, NEP IC50 = 8.0 nM, PDB ID: 6SUK, Figure 4C) is an investigative pharmaceutical that functions as an inhibitor for both NEP and ACE (Sharma et al., 2020). In clinical studies, omapatrilat has been extensively investigated as a dual ACEI/NEPI (Cozier et al., 2018; Robl et al., 1997), and has proven significant efficacy in lowering blood pressure among individuals with hypertension (Azizi et al., 2000; Kostis et al., 2004). However, compared to placebo, omapatrilat is associated with a notably higher occurrence of side effects such as coughing, skin flushing, short-term facial redness, gastrointestinal disturbances, and potentially life-threatening adverse reactions such as angioedema (Arendse et al., 2019). These adverse safety profiles have hindered the advancement of this promising vasopeptidase inhibitor.
Omapatrilat was crafted to mimic a tripeptide, allowing it to engage with the S1, S1', and S2' subsites of the target metalloproteases, a design intended to enhance its binding specificity and efficacy (Sharma et al., 2020). The primary binding occurs in the NEP complex structure at the S1' and S2' subsites. At the same time, a segment of the bicyclic group protrudes into the S3' region, facilitating a more extensive interaction with the enzyme’s binding pockets (Sharma et al., 2020). The thiol group of omapatrilat forms a coordination complex with the zinc ion and two water molecules, facilitating interactions with His711 and the backbone of Ala543 (Sharma et al., 2020). The phenyl moiety of omapatrilat delves into the S1' pocket, creating many hydrophobic contacts with Phe106, Phe563, Val580, and Trp693. Moreover, its Cα-analogous atom also participates in hydrophobic interactions with His583, thereby enhancing the compound’s binding to the NEP enzyme (Sharma et al., 2020). The P1′ carbonyl of omapatrilat has hydrophobic interactions with His-711 at C11 and bidentate interactions with Arg-717 at O4 (Sharma et al., 2020).
M100240 (Compound 17, Figure 4C), a sulfur ester of MDL 100173, is a dual inhibitor targeting both angiotensin I-converting enzyme (ACE IC50 = 0.08 nmol/L) and neutral endopeptidase (NEP IC50 = 0.11 nmol/L) (Rousso et al., 1999). M100240 has been demonstrated in clinical research to decrease ACE activity and the concentration of angiotensin II. Simultaneously, it raises plasma renin levels and potentiates the beneficial actions of atrial natriuretic peptides (Rousso et al., 1999). This could offer distinctive therapeutic advantages in conditions marked by heightened vascular volume or sodium surplus and increased venous pressure (Schiering et al., 2016). Studies further suggest that M100240 exhibits safety and good tolerability in individuals, whether in a fed or fasting state (Schiering et al., 2016).
Lauren B. and the research team created three dipeptides with a carboxyl-3-phenyl propyl group at the terminal end and N-terminal nitrogen inspired by the structure of LisW—an ACE inhibitor. This synthesis was conducted to investigate the structural prerequisites for achieving dual inhibition of both ACE and NEP (Arendse et al., 2022). Removal of the P1′ amine from LisW to create AD 011 (Compound 18, Figure 4D) improved NEPI potency but showed poorer NEP affinity compared to ACE affinity (Oefner et al., 2000b; Arendse et al., 2022). Substituting the P2′ tryptophan residue in AD 011 with tyrosine to produce AD 012 (Compound 19, Figure 4D) further enhanced binding to NEP (Oefner et al., 2004; Arendse et al., 2022). In contrast to the earlier reported 2-mercapto-3-phenylpropionyl derivatives that possessed identical P1′ and P2′ groups, compound AD 013 (Compound 20, Figure 4D) exhibited a diminished binding affinity for NEP and the catalytic domain of cACE (Sharma et al., 2020). For inhibitors lacking suitable P1′ side chains for NEP S1′ subsite binding, those with a 2-mercapto-3-phenylpropionyl N-terminal may adopt a more selective binding orientation in NEP (Arendse et al., 2022).
3.2.2 NEP and ECE dual target inhibitors
Daglutril (Compound 21, SLV-306, Figure 5A) represents an innovative dual-action inhibitor targeting both NEP and ECE (Seed et al., 2012). The fundamental principle of this drug is to inhibit both neprilysin and endothelin-converting enzymes (Seed et al., 2012). The dual-action inhibitor’s benefit is bypassing the compensatory actions that occur with single enzyme inhibition, potentially offering better efficacy by blocking both enzymes simultaneously (Ferro et al., 1998). Following oral intake, Daglutril is metabolized into its active form (Dickstein et al., 2004), which then inhibits the systemic transformation of Big-ET1 and boosts circulating levels of ANP (Seed et al., 2012). In a compact, randomized, crossover, double-masked, placebo-controlled study, Daglutril was found to lower blood pressure in individuals with type 2 diabetic nephropathy throughout the 8-day treatment period, yet it did not demonstrate a reduction in albuminuria (Parvanova et al., 2013).
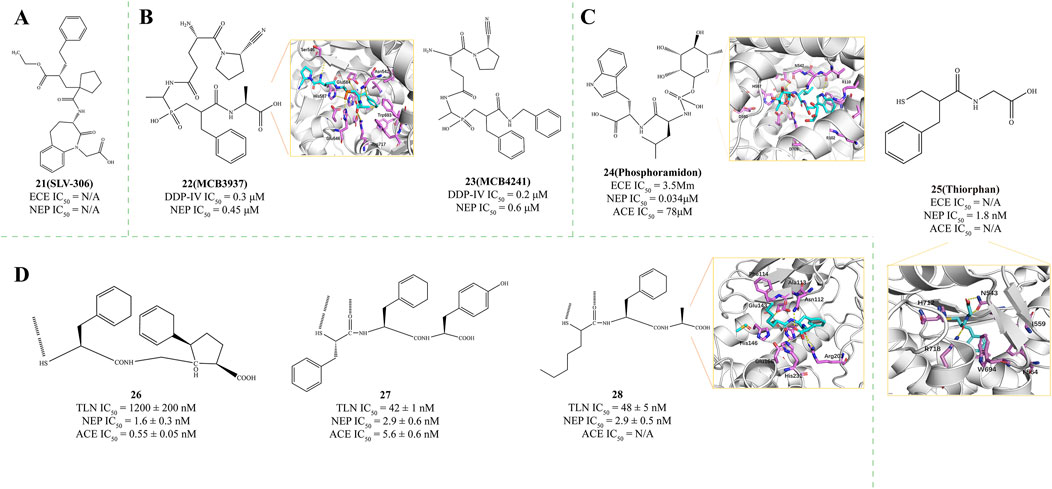
Figure 5. (A) Structures of NEP and ECE dual inhibitor (Seed et al., 2012). (B) Structures of NEP and DDP-IV dual inhibitor. X-ray structure of NEP in complex with compound 23 (PDB ID: 2QPJ) (Oefner et al., 2004). (C) Structures of NEP, ECE, and ACE Multi-Target Inhibitor. X-ray structure of NEP in complex with compound 24 (PDB ID: 1DMT) (Oefner et al., 2000b; Roques et al., 1980; Lambert et al., 1993; Labiuk et al., 2019). (D) Structures of NEP, TLN, and ACE Multi-Target Inhibitor. X-ray structure of NEP in complex with compound 28 (PDB ID: 1QF1) (Gaucher et al., 1999).
3.2.3 NEP and DPP-IV dual target inhibitors
Dipeptidyl peptidase IV (DPP-IV) is a versatile type II transmembrane serine protease that functions as a glycoprotein. It comprises 766 amino acids: 6 amino acids are located within the cytoplasm, 22 amino acids comprise the portion that spans the plasma membrane, and the remaining 738 amino acids constitute the extracellular domain (Misumi et al., 1992). Oefner et al. proposed that DPP-IV inhibitors could be connected to NEPIs through their terminal unreacted substituents, residues, or C-terminal portions (Oefner et al., 2004). Following this design strategy, they synthesized NEP/DPP-IV dual inhibitors MCB 3937 (Compound 22, DPP-IV IC50 = 0.3 μM, NEP IC50 = 0.45 μM, PDB ID: 2QPJ, Figure 5B) and MCB 4241 (Compound 23, DPP-IV IC50 = 0.2 μM, NEP IC50 = 0.6 μM, Figure 5B) (Oefner et al., 2004). The benzyl group of the Inhibitors engages with the S1′ subsite of the enzyme, enhancing the binding affinity for NEP. This interaction is mainly facilitated by the aromatic or bulky hydrophobic residues present in the subsite (Oefner et al., 2004). The concept of dual NEP/DPP-IV inhibition can, in theory, be extended to a range of established enzyme inhibitor classes to potentially enhance their inhibitory efficacy.
3.2.4 Angiotensin receptor neprilysin inhibitors
ARNIs are a novel class of cardiovascular drugs that have emerged as a significant advancement in the treatment of heart failure and hypertension. ARNIs exert their effects by inhibiting the angiotensin receptor and neprilysin, a neutral endopeptidase that degrades various vasoactive peptides, including natriuretic peptides (Yamamoto and Rakugi, 2021).
The first-in-class ARNI, sacubitril/valsartan, has demonstrated superior cardioprotective effects compared to traditional renin-angiotensin system inhibitors (RAS-Is) in large clinical trials such as the PARADIGM-HF trial (Tsukamoto et al., 2023). This drug combines the neprilysin inhibition of sacubitril with the angiotensin receptor blockade of valsartan, enhancing the levels of beneficial peptides like natriuretic peptides while blocking the effects of angiotensin II (Kario and Williams, 2022).
ARNIs are effective in reducing cardiovascular mortality and heart failure hospitalizations by 20% in ambulatory patients with heart failure and reduced ejection fraction, as reported in the PARADIGM-HF trial (Mann et al., 2022). They have also been suggested to provide renoprotective effects beyond those of RAS-Is in patients with heart failure (Tsukamoto et al., 2023).
Furthermore, ARNIs have been studied for their efficacy in blood pressure management. Accumulating evidence suggests that sacubitril/valsartan is superior to conventional RAS Blockers in lowering blood pressure in patients with hypertension (Bozkurt et al., 2023). This class of drugs is effective in improving renal outcomes and has a lower risk of renal dysfunction and a higher estimated glomerular filtration rate (eGFR) compared to RAS-Is, without an increased risk of hyperkalemia (Chopra et al., 2023).
3.3 Multi-target inhibitors
3.3.1 NEP, ECE, and ACE multi-target inhibitors
Phosphoramidon (Compound 24, Figure 5C) is a microbial metabolite and a specific metalloprotease thermolysin inhibitor. Phosphoramidon inhibits neprilysin (NEP, IC50 = 0.034 μM, PDB ID: 1DMT), as well as an endothelin-converting enzyme (ECE, IC50 = 3.5 μM) and angiotensin-converting enzyme (ACE, IC50 = 78 μM) (Oefner et al., 2000b). The rhamnose group at the P1 position of the inhibitor is mainly in contact with the solvent, allowing the majority of the phosphoramidon surface to engage with the enzyme, thereby providing a secondary stabilization effect on the S1 subsite (Gaucher et al., 1999; Ksander et al., 1997b). The NEP’s1 subsite creates a substantial hydrophobic pocket that selectively binds with aromatic or bulky hydrophobic moieties (Tiraboschi et al., 1999). The S2′ subsite, notably spacious, reaches into the solvent environment through the junctional helix A3, approaching the side chains of residues R102, D107, and R110. This observation verifies that the S2′ subsite of NEP exhibits lower specificity and can accommodate larger side chains. These polar residues are not involved in the binding process with the inhibitor (Lambert et al., 1993).
As a NEPI, Racecadotril can rapidly metabolize into the effective Thiorphan (compound 25, IC50 = 1.8 nM, PDB ID = 5V48, Patent: US20110319656, Figure 5C) in the body (Roques et al., 1980; Lambert et al., 1993; Labiuk et al., 2019; Schwartz, 2000; Eberlin et al., 2012). In contrast, its inhibitory effects on ACE and Endothelin-Converting Enzyme 1 (ECE1) are significantly weaker, with Ki values exceeding 0.1 and 10 μM, respectively. Thiorphan exerts its inhibitory effect on NEP by binding to the zinc ion at the enzyme’s active site via its thiol group, a critical interaction for its activity (Labiuk et al., 2019). This coordination interferes with the catalytic mechanism of NEP, thereby inhibiting its peptidase activity (Labiuk et al., 2019). Additionally, the phenyl ring structure of Thiorphan penetrates the central cavity of NEP and interacts hydrophobically with surrounding residues such as Phe107, Ile559, Phe564, Val581, and Trp694. These hydrophobic interactions are essential for Thiorphan’s stable binding in the NEP active site, contributing to its inhibitory potency (Labiuk et al., 2019). These features make Thiorphan a promising candidate drug, warranting further research and development to explore its potential in treating related diseases.
3.3.2 NEP, TLN, and ACE multi-target inhibitors
The three-dimensional model of thermolysin (TLN) has been widely used for identifying the active sites of NEP (Tiraboschi et al., 1999) and for inhibitor design (Ksander et al., 1997a). Previous research has identified R-Mercaptoacyl dipeptides such as compound Ala-Pro (Coric et al., 1996) and compound Gly-(5-Ph)Pro (Fournie-Zaluski et al., 1996), which bind to NEP through thiol-induced Zn2+ ion coordination and interactions with the enzyme’s1′ and S2′ subsites (Gaucher et al., 1999; Holmes and Matthews, 1981). Based on this discovery, they further validated the binding activity of three R-Mercaptoacyl dipeptides to NEP: [(2S)-2-sulfanyl-3-phenylpropanoyl]Gly-(5-Ph)Pro26 (TLN IC50 = 1200 ± 200 nM; NEP IC50 = 1.6 ± 0.3 nM; ACE IC50 = 0.55 ± 0.05 nM, Figure 5D), [(2S)-2-sulfanyl-3-phenylpropanoyl]Phe-Tyr27 (TLN IC50 = 42 ± 1 nM; NEP IC50 = 2.9 ± 0.6 nM; ACE IC50 = 5.6 ± 0.6 nM, Figure 5D), and [(2S, R)-2-sulfanylheptanoyl]-Phe-Ala28 (TLN IC50 = 48 ± 5 nM, PDB ID: 1QF1; NEP IC50 = 2.9 ± 0.5 nM, Figure 5D) (Gaucher et al., 1999). Compounds 27 and 28 exhibit higher affinity than compound 26. Comparing the inhibitory potency of these compounds and their various analogs against TLN, NEP, and ACE provides insights that confirm their binding modes with the latter two enzymes (Gaucher et al., 1999). A novel binding mode for thermolysin recognition, distinguished by the bidentate coordination of the zinc atom through thiol and oxygen groups, has been suggested. This concept opens up new avenues for designing inhibitors modeled after this interaction (Gaucher et al., 1999).
3.4 Clinical drugs
Entresto (LCZ696, sacubitril/valsartan) is an innovative pharmaceutical compound that integrates the functions of an ARB with the NEPI prodrug AHU 377, offering a dual-action approach for the treatment of heart failure and hypertension (Gu et al., 2010). LCZ696 is a novel ARNi that offers a dual therapeutic action. Upon oral ingestion, it swiftly initiates NEPI while simultaneously blocking angiotensin receptors, which is beneficial for managing heart failure and other cardiovascular conditions (Ksander et al., 1995). It is effective in treating hypertension and heart failure, showing antihypertensive effects in animal models and good tolerability in clinical trials with primarily mild adverse events (Ksander et al., 1995). In clinical studies, LCZ696 has demonstrated superior blood pressure reduction compared to placebo, AHU 377 alone, or valsartan alone in phase 1-2 hypertension trials (Ruilope et al., 2010). It has been demonstrated to elicit the anticipated alterations in plasma hormone levels, affirming the blockade of the AT1 receptor as evidenced by elevated plasma renin concentration, and the inhibition of neprilysin, as indicated by the increased concentrations of plasma ANP and cGMP (Ruilope et al., 2010).
The PARADIGM-HF clinical trial investigated the effects of sacubitril/valsartan, demonstrating that this medication significantly reduced the risk of cardiovascular death and heart failure hospitalization by 20% and the risk of all-cause mortality by 16% (Savarese et al., 2023). Regarding adverse reactions, the group treated with LCZ696 exhibited a reduced risk of experiencing cough, hyperkalemia, and renal dysfunction compared to the control group. Additionally, there were minimal treatment discontinuations due to symptomatic hypotension, and no significant differences were observed in the risk of angioedema between the groups (Savarese et al., 2023). Subgroup analyses highlighted LCZ696’s benefits in reducing sudden cardiac death, improving quality of life, enhancing metabolic profiles in type 2 diabetes patients, lowering uric acid levels, protecting renal function, and reducing heart failure rehospitalizations (Packer et al., 2018; Tomson et al., 2021; Jankowski et al., 2021; Ryu et al., 2021). Subgroup analyses highlighted LCZ696’s benefits in reducing sudden cardiac death, improving quality of life, enhancing metabolic profiles in type 2 diabetes patients, lowering uric acid levels, protecting renal function, and reducing heart failure rehospitalizations (Johansen et al., 2021). A study involving 3,518 HFrEF patients across 150 medical institutions recommended strengthening medication management and initiating LCZ696 therapy early and at adequate doses in eligible patients to improve clinical outcomes and prognosis (Johansen et al., 2021; Cai et al., 2013). Additionally, recent studies have shown that patients receiving a high dose of 400 mg of sacubitril/valsartan have a better prognosis compared to those on a low dose of less than 200 mg, with no significant differences in the incidence of hyperkalemia, serious renal events, and angioedema among the dosage groups (Doi et al., 2024). In another PARADIGM-HF trial, sacubitril/valsartan’s efficacy in reducing cardiovascular death or heart failure hospitalization was sustained or enhanced in patients with hypotension, highlighting its potential as a safe and effective treatment option in such cases (Matsumoto et al., 2024).
In a PARAGLIDE-HF trial’s secondary analysis, the win ratio (WR) was employed to evaluate the efficacy of sacubitril/valsartan compared to valsartan in heart failure patients with an ejection fraction >40% (Shoji et al., 2024). The primary hierarchical outcome, which included cardiovascular death, heart failure hospitalizations, urgent visits, and a 25% reduction in NT-proBNP, showed a significant treatment effect favoring sacubitril/valsartan (WR, 1.46; 95% CI, 1.08–1.97) in the subgroup with ejection fraction ≤60%. Sensitivity analyses, adjusting for NT-proBNP changes and incorporating renal outcomes, consistently supported the superiority of sacubitril/valsartan. These findings underscore the robustness of sacubitril/valsartan’s treatment benefit and suggest the value of pre-specifying WR sensitivity analyses in future studies for a comprehensive assessment of treatment effects. Another win ratio analysis of the PARAGON-HF study indicates that sacubitril-valsartan provides significant clinical benefits in patients with heart failure and preserved ejection fraction, with these benefits being independent of left ventricular ejection fraction and sex (Yoon et al., 2024).
4 Conclusion and perspectives
Neutral endopeptidase is a widely distributed membrane-bound zinc-dependent metallopeptidase that plays a critical role in various physiological processes, including diuresis, natriuresis, and the processing and degradation of vasoactive peptides. NEPIs are a class of drugs with significant clinical value, enhancing the physiological effects of bioactive peptides by inhibiting NEP activity. This makes them promising in treating Alzheimer’s disease, cardiovascular diseases, arthritis, and other conditions.
This review summarizes the research progress of NEPIs, focusing on single-target and dual-target inhibitors of NEP. Representative NEP single-target inhibitors such as Sacubitril and Thiorphan have demonstrated high efficacy and selectivity, marking them as promising candidates for further research. Current research on NEP dual-target inhibitors Mainly involves NEP with neprilysin and ACE and multi-target inhibitors, including ECE and DPP-IV. Despite their broad clinical potential, NEPIs' safety and side effects remain critical concerns in clinical application. NEPIs may cause hypotension, renal dysfunction, and electrolyte imbalances. Therefore, when using NEPIs, careful monitoring of physiological indicators and dosage adjustment according to individual patient conditions are essential. As research progresses, NEPIs' applications are expected to expand, and further investigation into their safety and side effects will help improve clinical outcomes.
The widespread distribution of NEP and its involvement in various crucial physiological processes make its inhibitors a vital research focus for treating multiple diseases. Although NEPIs offer many advantages, they also present challenges. For example, the binding affinity and stability of single-target inhibitors need further improvement, and there needs to be more comprehensive research regarding their efficacy and specificity. Future research may uncover new targets, leading to the design of more precise multi-target inhibitors. Additionally, personalized treatment plans and combination therapy strategies based on patients' genotypes and protein expression profiles could help optimize the use of NEPIs, enhance treatment efficacy, and reduce side effects.
In summary, NEP inhibitors hold significant potential for treating various diseases. Future research should explore their targets and combination therapies with other treatments to enhance their safety and broaden their application range, providing more effective and safer treatment options for patients.
Author contributions
XZ: Writing–original draft, Writing–review and editing. CH: Investigation, Writing–review and editing. ET: Investigation, Methodology, Resources, Validation, Writing–review and editing. YS: Resources, Writing–review and editing. WL: Writing–review and editing. JL: Writing–review and editing.
Funding
The author(s) declare that no financial support was received for the research, authorship, and/or publication of this article.
Conflict of interest
The authors declare that the research was conducted in the absence of any commercial or financial relationships that could be construed as a potential conflict of interest.
Generative AI statement
The author(s) declare that no Generative AI was used in the creation of this manuscript.
Publisher’s note
All claims expressed in this article are solely those of the authors and do not necessarily represent those of their affiliated organizations, or those of the publisher, the editors and the reviewers. Any product that may be evaluated in this article, or claim that may be made by its manufacturer, is not guaranteed or endorsed by the publisher.
References
Abassi, Z., Golomb, E., and Keiser, H. R. (1992). Neutral endopeptidase inhibition increases the urinary excretion and plasma levels of endothelin. Metabolism 41, 683–685. doi:10.1016/0026-0495(92)90303-R
Ager, D. J., and De Vries, J. G. (2012). “9.4 industrial applications of asymmetric reduction of C=C bonds,” in Comprehensive chirality (Elsevier), 73–82. doi:10.1016/B978-0-08-095167-6.00904-6
AlAnazi, F. H., Al-kuraishy, H. M., Al-Gareeb, A. I., Alexiou, A., Papadakis, M., Ogaly, H. A., et al. (2023). Effects of neprilysin and neprilysin inhibitors on glucose homeostasis: controversial points and a promising arena. J. Diabetes 15, 397–408. doi:10.1111/1753-0407.13389
Ali, N. H., Al-Kuraishy, H. M., Al-Gareeb, A. I., Alnaaim, S. A., Alexiou, A., and Papadakis, M. (2024). Neprilysin inhibitors and risk of Alzheimer’s disease: A future perspective. J. Cell. Mol. Med. 28, e17993. doi:10.1111/jcmm.17993
Andersen, P., Seljeflot, I., Herzog, A., Arnesen, H., Hjermann, I., and Holme, I. (1998). Effects of doxazosin and atenolol on atherothrombogenic risk profile in hypertensive middle-aged men. J. Cardiovasc Pharmacol. 31, 677–683. doi:10.1097/00005344-199805000-00005
Arendse, L. B., Cozier, G. E., Eyermann, C. J., Basarab, G. S., Schwager, S. L., Chibale, K., et al. (2022). Probing the requirements for dual angiotensin-converting enzyme C-domain selective/neprilysin inhibition. J. Med. Chem. 65, 3371–3387. doi:10.1021/acs.jmedchem.1c01924
Arendse, L. B., Danser, A. H. J., Poglitsch, M., Touyz, R. M., Burnett, J. C., Llorens-Cortes, C., et al. (2019). Novel therapeutic approaches targeting the renin-angiotensin system and associated peptides in hypertension and heart failure. Pharmacol. Rev. 71, 539–570. doi:10.1124/pr.118.017129
Arundel, C., Sheriff, H. M., Lam, P. H., Mohammed, S. F., Jones, L. G., Jurgens, C. Y., et al. (2020). Renin-angiotensin inhibition and outcomes in nursing home residents with heart failure. Am. J. Ther. 27, e235–e242. doi:10.1097/MJT.0000000000000836
Azizi, M., Massien, C., Michaud, A., and Corvol, P. (2000). In vitro and in vivo inhibition of the 2 active sites of ACE by omapatrilat, a vasopeptidase inhibitor. Hypertension 35, 1226–1231. doi:10.1161/01.HYP.35.6.1226
Bavishi, C., Messerli, F. H., Kadosh, B., Ruilope, L. M., and Kario, K. (2015). Role of neprilysin inhibitor combinations in hypertension: insights from hypertension and heart failure trials. Eur. Heart J. 36, 1967–1973. doi:10.1093/eurheartj/ehv142
Bayes-Genis, A., Barallat, J., and Richards, A. M. (2016). A test in context: neprilysin: function, inhibition, and biomarker. J. Am. Coll. Cardiol. 68, 639–653. doi:10.1016/j.jacc.2016.04.060
Benigni, A., Zoja, C., Zatelli, C., Corna, D., Longaretti, L., Rottoli, D., et al. (2004). Vasopeptidase inhibitor restores the balance of vasoactive hormones in progressive nephropathy. Kidney Int. 66, 1959–1965. doi:10.1111/j.1523-1755.2004.00982.x
Bozkurt, B., Nair, A. P., Misra, A., Scott, C. Z., Mahar, J. H., and Fedson, S. (2023). Neprilysin inhibitors in heart failure: the science, mechanism of action, clinical studies, and unanswered questions. JACC Basic Transl. Sci. 8, 88–105. doi:10.1016/j.jacbts.2022.05.010
Cai, G., Zheng, Y., Sun, X., and Chen, X.the Survey of Prevalence, Awareness, and Treatment Rat, and es in Chronic Kidney Disease Patients with Hypertension in China Collaborative Group (2013). Prevalence, awareness, treatment, and control of hypertension in elderly adults with chronic kidney disease: results from the survey of prevalence, awareness, and treatment rates in chronic kidney disease patients with hypertension in China. J Am. Geriatrics Soc. 61, 2160–2167. doi:10.1111/jgs.12551
Campbell, D. J. (2017). Long-term neprilysin inhibition — implications for ARNIs. Nat. Rev. Cardiol. 14, 171–186. doi:10.1038/nrcardio.2016.200
Cao, Z., Burrell, L. M., Tikkanen, I., Bonnet, F., Cooper, M. E., and Gilbert, R. E. (2001). Vasopeptidase inhibition attenuates the progression of renal injury in subtotal nephrectomized rats. Kidney Int. 60, 715–721. doi:10.1046/j.1523-1755.2001.060002715.x
Chapman, F. A., Nyimanu, D., Maguire, J. J., Davenport, A. P., Newby, D. E., and Dhaun, N. (2021). The therapeutic potential of apelin in kidney disease. Nat. Rev. Nephrol. 17, 840–853. doi:10.1038/s41581-021-00461-z
Chen, H., Noble, F., Mothé, A., Meudal, H., Coric, P., Danascimento, S., et al. (2000). Phosphinic derivatives as new dual enkephalin-degrading enzyme inhibitors: synthesis, biological properties, and antinociceptive activities. J. Med. Chem. 43, 1398–1408. doi:10.1021/jm990483l
Cheung, B. M. Y., Dickerson, J. E. C., Ashby, M. J., Brown, M. J., and Brown, J. (1994). Effects of physiological increments in human alpha-atrial natriuretic peptide and human brain natriuretic peptide in normal male subjects. Clin. Sci. 86, 723–730. doi:10.1042/cs0860723
Chien, Y. W., Frohlich, E. D., and Trippodo, N. C. (1987). Atrial natriuretic peptide increases resistance to venous return in rats. Am. J. Physiology-Heart Circulatory Physiology 252, H894–H899. doi:10.1152/ajpheart.1987.252.5.H894
Chopra, H. K., Wander, G. S., Ponde, C. K., Nanda, N. C., Khullar, D., Venugopal, K., et al. (2023). The power and promise of angiotensin receptor neprilysin inhibitor (ARNI) in heart failure management: national consensus statement. J. Assoc. Physicians India 71, 11–12. doi:10.5005/japi-11001-0209
Cogan, M. G. (1990). Renal effects of atrial natriuretic factor. Annu. Rev. Physiol. 52, 699–708. doi:10.1146/annurev.ph.52.030190.003411
Coric, P., Turcaud, S., Meudal, H., Roques, B. P., and Fournie-Zaluski, M.-C. (1996). Optimal recognition of neutral endopeptidase and angiotensin-converting enzyme active sites by mercaptoacyldipeptides as a means to design potent dual inhibitors. J. Med. Chem. 39, 1210–1219. doi:10.1021/jm950590p
Cozier, G. E., Arendse, L. B., Schwager, S. L., Sturrock, E. D., and Acharya, K. R. (2018). Molecular basis for multiple omapatrilat binding sites within the ACE C-domain: implications for drug design. J. Med. Chem. 61, 10141–10154. doi:10.1021/acs.jmedchem.8b01309
Dahrouj, M., Alsarraf, O., Liu, Y., Crosson, C. E., and Ablonczy, Z. (2013). C-type natriuretic peptide protects the retinal pigment epithelium against advanced glycation end product–induced barrier dysfunction. J. Pharmacol. Exp. Ther. 344, 96–102. doi:10.1124/jpet.112.199307
Davis, K. N., Hines, A. E., Schaefer, M. C., and Naseman, K. W. (2022). Protecting the kidneys: update on therapies to treat diabetic nephropathy. Clin. Diabetes 40, 305–311. doi:10.2337/cd21-0090
Derkachev, I. A., Popov, S. V., Maslov, L. N., Mukhomedzyanov, A. V., Naryzhnaya, N. V., Gorbunov, A. S., et al. (2024). Angiotensin 1–7 increases cardiac tolerance to ischemia/reperfusion and mitigates adverse remodeling of the heart—the signaling mechanism. Fundamemntal Clin. Pharma 38, 489–501. doi:10.1111/fcp.12983
Dickstein, K., De Voogd, H. J., Miric, M. P., Willenbrock, R., Mitrovic, V., Pacher, R., et al. (2004). Effect of single doses of SLV306, an inhibitor of both neutral endopeptidase and endothelin-converting enzyme, on pulmonary pressures in congestive heart failure. Am. J. Cardiol. 94, 237–239. doi:10.1016/j.amjcard.2004.03.074
Dion, N., Cohen, P., Crine, P., and Boileau, G. (1997). Characterisation of neprilysin (EC 3.4.24.11) S 2, subsite. FEBS Lett. 411, 140–144. doi:10.1016/S0014-5793(97)00681-9
Doi, S., Kida, K., Nasu, T., Ishii, S., Kagiyama, N., Fujimoto, W., et al. (2024). Uptitration of sacubitril/valsartan and outcomes in patients with heart failure - insight from the REVIEW-HF registry. Circ. J. doi:10.1253/circj.CJ-24-0636
Domenig, O., Manzel, A., Grobe, N., Königshausen, E., Kaltenecker, C. C., Kovarik, J. J., et al. (2016). Neprilysin is a mediator of alternative renin-angiotensin-system activation in the murine and human kidney. Sci. Rep. 6, 33678. doi:10.1038/srep33678
Dorofeyeva, L. V. (1975). Obtaining of measles virus haemagglutinin from strain L-16 grown in primary cell cultures. Acta Virol. 19, 497.
Eberlin, M., Mück, T., and Michel, M. C. (2012). A comprehensive review of the pharmacodynamics, pharmacokinetics, and clinical effects of the neutral endopeptidase inhibitor Racecadotril. Front. Pharmacol. 3, 93. doi:10.3389/fphar.2012.00093
Edwards, B. S., Zimmerman, R. S., Schwab, T. R., Heublein, D. M., and Burnett, J. C. (1988). Atrial stretch, not pressure, is the principal determinant controlling the acute release of atrial natriuretic factor. Circ. Res. 62, 191–195. doi:10.1161/01.RES.62.2.191
Emoto, N., and Yanagisawa, M. (1995). Endothelin-converting enzyme-2 is a membrane-bound, phosphoramidon-sensitive metalloprotease with acidic pH optimum. J. Biol. Chem. 270, 15262–15268. doi:10.1074/jbc.270.25.15262
Epstein, F. H., Levin, E. R., Gardner, D. G., and Samson, W. K. (1998). Natriuretic peptides. N. Engl. J. Med. 339, 321–328. doi:10.1056/NEJM199807303390507
Esser, N., Barrow, B. M., Choung, E., Shen, N. J., and Zraika, S. (2018). Neprilysin inhibition in mouse islets enhances insulin secretion in a GLP-1 receptor dependent manner. Islets 10, 175–180. doi:10.1080/19382014.2018.1502521
Esser, N., and Zraika, S. (2019). Neprilysin inhibition: a new therapeutic option for type 2 diabetes? Diabetologia 62, 1113–1122. doi:10.1007/s00125-019-4889-y
Esser, N., Mongovin, S. M., Parilla, J., Barrow, B. M., Mundinger, T. O., and Fountaine, B. S. (2022). Neprilysin inhibition improves intravenous but not oral glucose-mediated insulin secretion via GLP-1R signaling in mice with β-cell dysfunction Am. J. Physiol. Endocrinol. Metab. 322, E307–E318. doi:10.1152/ajpendo.00234.2021
Esser, N., Mundinger, T. O., Barrow, B. M., and Zraika, S. (2023). Acute inhibition of intestinal neprilysin enhances insulin secretion via GLP-1 receptor signaling in male mice Endocrinology 164, bqad055. doi:10.1210/endocr/bqad055
Ferro, C. J., Spratt, J. C., Haynes, W. G., and Webb, D. J. (1998). Inhibition of neutral endopeptidase causes vasoconstriction of human resistance vessels in vivo. Circulation 97, 2323–2330. doi:10.1161/01.CIR.97.23.2323
Flick, A. C., Ding, H. X., Leverett, C. A., Kyne, R. E., Liu, K. K.-C., Fink, S. J., et al. (2017). Synthetic approaches to the new drugs approved during 2015. J. Med. Chem. 60, 6480–6515. doi:10.1021/acs.jmedchem.7b00010
Fournie-Zaluski, M.-C., Coric, P., Thery, V., Gonzalez, W., Meudal, H., Turcaud, S., et al. (1996). Design of orally active dual inhibitors of neutral endopeptidase and angiotensin-converting enzyme with long duration of action. J. Med. Chem. 39, 2594–2608. doi:10.1021/jm950783c
Fujita, T., Ito, Y., Noda, H., Sato, Y., Ando, K., Kangawa, K., et al. (1987). Vasodilatory actions of alpha-human atrial natriuretic peptide and high Ca2+ effects in normal man. J. Clin. Invest. 80, 832–840. doi:10.1172/JCI113141
Gallagher, H., and Suckling, R. J. (2016). Diabetic nephropathy: where are we on the journey from pathophysiology to treatment? Diabetes Obes. Metab. 18, 641–647. doi:10.1111/dom.12630
Gaucher, J. F., Selkti, M., Tiraboschi, G., Prangé, T., Roques, B. P., Tomas, A., et al. (1999). Crystal structures of alpha-mercaptoacyldipeptides in the thermolysin active site: structural parameters for a Zn monodentation or bidentation in metalloendopeptidases. Biochemistry 38, 12569–12576. doi:10.1021/bi991043z
Genova, R. M., Meyer, K. J., Anderson, M. G., Harper, M. M., and Pieper, A. A. (2018). Neprilysin inhibition promotes corneal wound healing. Sci. Rep. 8, 14385. doi:10.1038/s41598-018-32773-9
Goru, S. K., Kadakol, A., Malek, V., Pandey, A., Sharma, N., and Gaikwad, A. B. (2017). Diminazene aceturate prevents nephropathy by increasing glomerular ACE2 and AT2 receptor expression in a rat model of type1 diabetes. Br. J Pharmacol. 174, 3118–3130. doi:10.1111/bph.13946
Grimm, M. O. W., Lehmann, J., Mett, J., Zimmer, V. C., Grösgen, S., Stahlmann, C. P., et al. (2014). Impact of Vitamin D on amyloid precursor protein processing and amyloid-β peptide degradation in Alzheimer's disease. Neurodegener. Dis. 13, 75–81. doi:10.1159/000355462
Gu, J., Noe, A., Chandra, P., Al-Fayoumi, S., Ligueros-Saylan, M., Sarangapani, R., et al. (2010). Pharmacokinetics and pharmacodynamics of LCZ696, a novel dual-acting angiotensin receptor—neprilysin inhibitor (ARNi). J. Clin. Pharma 50, 401–414. doi:10.1177/0091270009343932
Gunning, M. E., Brady, H. R., Otuechere, G., Brenner, B. M., and Zeidel, M. L. (1992). Atrial natriuretic peptide(31-67) inhibits Na+ transport in rabbit inner medullary collecting duct cells. Role of prostaglandin E2. J. Clin. Invest. 89, 1411–1417. doi:10.1172/JCI115730
Hakata, T., Ueda, Y., Yamashita, T., Yamauchi, I., Kosugi, D., and Sugawa, T. (2024). Neprilysin inhibition promotes skeletal growth via the CNP/NPR-B pathway. Endocrinology 165, bqae058. doi:10.1210/endocr/bqae058
Hasby, E. A., and Saad, H. A. (2013). Immunohistochemical expression of fas ligand (FasL) and neprilysin (neutral endopeptidase/CD10) in keratoconus. Int. Ophthalmol. 33, 125–131. doi:10.1007/s10792-012-9651-0
He, Y., Jin, Y., Xue, H., Liu, R., Zhang, M., Liao, R., et al. (2023). Pharmacokinetics and pharmacodynamics of sacubitril/valsartan in peritoneal dialysis patients. Nephrol. Dial. Transplant. 38, 1880–1889. doi:10.1093/ndt/gfad038
Holmes, M. A., and Matthews, B. W. (1981). Binding of hydroxamic acid inhibitors to crystalline thermolysin suggests a pentacoordinate zinc intermediate in catalysis. Biochemistry 20, 6912–6920. doi:10.1021/bi00527a026
Howell, S., Nalbantoglu, J., and Crine, P. (1995). Neutral endopeptidase can hydrolyze beta-amyloid(1-40) but shows no effect on beta-amyloid precursor protein metabolism. Peptides 16, 647–652. doi:10.1016/0196-9781(95)00021-b
Ignatovich, V. F. (1975). Enhancement of the antigenic activity and virulence of the vaccine strain E of Rickettsia prow azeki by passages in cell culture. Acta Virol. 19, 481–485.
Iijima-Ando, K., Hearn, S. A., Granger, L., Shenton, C., Gatt, A., Chiang, H.-C., et al. (2008). Overexpression of neprilysin reduces alzheimer amyloid-beta42 (Abeta42)-induced neuron loss and intraneuronal Abeta42 deposits but causes a reduction in cAMP-responsive element-binding protein-mediated transcription, age-dependent axon pathology, and premature death in Drosophila. J. Biol. Chem. 283, 19066–19076. doi:10.1074/jbc.M710509200
Iwata, N., Mizukami, H., Shirotani, K., Takaki, Y., Muramatsu, S., Lu, B., et al. (2004). Presynaptic localization of neprilysin contributes to efficient clearance of amyloid-beta peptide in mouse brain. J. Neurosci. 24, 991–998. doi:10.1523/JNEUROSCI.4792-03.2004
Jaffe, A. S., Apple, F. S., Mebazaa, A., and Vodovar, N. (2015). Unraveling N-terminal pro–B-type natriuretic peptide: another piece to a very complex puzzle in heart failure patients. Clin. Chem. 61, 1016–1018. doi:10.1373/clinchem.2015.243626
Jankowski, J., Floege, J., Fliser, D., Böhm, M., and Marx, N. (2021). Cardiovascular disease in chronic kidney disease: pathophysiological insights and therapeutic options. Circulation 143, 1157–1172. doi:10.1161/CIRCULATIONAHA.120.050686
Jiang, W., Jiang, H.-F., Pan, C.-S., Cai, D.-Y., Qi, Y.-F., Pang, Y.-Z., et al. (2004). Relationship between the contents of adrenomedullin and distributions of neutral endopeptidase in blood and tissues of spontaneously hypertensive rats. Hypertens. Res. 27, 109–117. doi:10.1291/hypres.27.109
Johansen, K. L., Chertow, G. M., Foley, R. N., Gilbertson, D. T., Herzog, C. A., Ishani, A., et al. (2021). US renal data system 2020 annual data report: epidemiology of kidney disease in the United States. Am. J. Kidney Dis. 77, A7–A8. doi:10.1053/j.ajkd.2021.01.002
Kario, K., and Williams, B. (2022). Angiotensin receptor-neprilysin inhibitors for hypertension-hemodynamic effects and relevance to hypertensive heart disease. Hypertens. Res. 45, 1097–1110. doi:10.1038/s41440-022-00923-2
Kerr, M. A., and Kenny, A. J. (1974a). The purification and specificity of a neutral endopeptidase from rabbit kidney brush border. Biochem. J. 137, 477–488. doi:10.1042/bj1370477
Kerr, M. A., and Kenny, A. J. (1974b). The purification and specificity of a neutral endopeptidase from rabbit kidney brush border. Biochem. J. 137, 477–488. doi:10.1042/bj1370477
Kostis, J., Packer, M., Black, H. R., Schmieder, R., Henry, D., and Levy, E. (2004). Omapatrilat and enalapril in patients with hypertension: the Omapatrilat Cardiovascular Treatment vs. Enalapril (OCTAVE) trial. Am. J. Hypertens. 17, 103–111. doi:10.1016/j.amjhyper.2003.09.014
Ksander, G. M., De Jesus, R., Yuan, A., Ghai, R. D., McMartin, C., and Bohacek, R. (1997a). Meta-substituted benzofused macrocyclic lactams as zinc metalloprotease inhibitors. J. Med. Chem. 40, 506–514. doi:10.1021/jm960583g
Ksander, G. M., De Jesus, R., Yuan, A., Ghai, R.D., Trapani, A., McMartin, C., et al. (1997b). Ortho-substituted benzofused macrocyclic lactams as zinc metalloprotease inhibitors. J. Med. Chem. 40 (4), 495–505. doi:10.1021/jm960582o
Ksander, G. M., Ghai, R. D., deJesus, R., Diefenbacher, C., Yuan, A., Berry, C., et al. (1995). Dicarboxylic acid dipeptide neutral endopeptidase inhibitors. J. Med. Chem. 38, 1689–1700. doi:10.1021/jm00010a014
Kuba, K., Sato, T., Imai, Y., and Yamaguchi, T. (2019). Apelin and Elabela/Toddler; double ligands for APJ/Apelin receptor in heart development, physiology, and pathology. Peptides 111, 62–70. doi:10.1016/j.peptides.2018.04.011
Labiuk, S. L., Sygusch, J., and Grochulski, P. (2019). Structures of soluble rabbit neprilysin complexed with phosphoramidon or thiophen. Acta Crystallogr. F. Struct. Biol. Commun. 75, 405–411. doi:10.1107/S2053230X19006046
Lai, W., Huang, R., Wang, B., Shi, M., Guo, F., Li, L., et al. (2023). Novel aspect of neprilysin in kidney fibrosis via ACSL4-mediated ferroptosis of tubular epithelial cells. MedComm 4, e330. doi:10.1002/mco2.330
Lambert, D. M., Mergen, F., Poupaert, J. H., and Dumont, P. (1993). Analgesic potency of S-acetylthiorphan after intravenous administration to mice. Eur. J. Pharmacol. 243, 129–134. doi:10.1016/0014-2999(93)90371-N
Li, Q., Fang, Y., Peng, D., Li, L., Deng, C., Yang, H., et al. (2023). Sacubitril/valsartan reduces susceptibility to atrial fibrillation by improving atrial remodeling in spontaneously hypertensive rats. Eur. J. Pharmacol. 952, 175754. doi:10.1016/j.ejphar.2023.175754
Liu, Y., Studzinski, C., Beckett, T., Guan, H., Hersh, M. A., Murphy, M. P., et al. (2009). Expression of neprilysin in skeletal muscle reduces amyloid burden in a transgenic mouse model of Alzheimer Disease. Mol. Ther. 17, 1381–1386. doi:10.1038/mt.2009.115
Liu, Y., Studzinski, C., Beckett, T., Murphy, M. P., Klein, R. L., and Hersh, L. B. (2010). Circulating neprilysin clears brain amyloid. Mol. Cell. Neurosci. 45, 101–107. doi:10.1016/j.mcn.2010.05.014
Malek, V., and Gaikwad, A. B. (2017). Neprilysin inhibitors: a new hope to halt the diabetic cardiovascular and renal complications? Biomed. & Pharmacother. 90, 752–759. doi:10.1016/j.biopha.2017.04.024
Mangiafico, S., Costello-Boerrigter, L. C., Andersen, I. A., Cataliotti, A., and Burnett, J. C. (2013). Neutral endopeptidase inhibition and the natriuretic peptide system: an evolving strategy in cardiovascular therapeutics. Eur. Heart J. 34, 886–893. doi:10.1093/eurheartj/ehs262
Mann, D. L., Givertz, M. M., Vader, J. M., Starling, R. C., Shah, P., McNulty, S. E., et al. (2022). Effect of treatment with sacubitril/valsartan in patients with advanced heart failure and reduced ejection fraction: a randomized clinical trial. JAMA Cardiol. 7, 17–25. doi:10.1001/jamacardio.2021.4567
Marie, C., Mossiat, C., Lecomte, J. M., Schwartz, J. C., and Bralet, J. (1995). Hemodynamic effects of acute and chronic treatment with aladotril, a mixed inhibitor of neutral endopeptidase and angiotensin I-converting enzyme, in conscious rats with myocardial infarction. J. Pharmacol. Exp. Ther. 275, 1324–1331.
Marr, R. A., and Spencer, B. J. (2010). Diabetes NEP-Like Endopeptidases and Alzheimers Disease. CAR 7, 223–229. doi:10.2174/156720510791050849
Matsumoto, S., Shen, L., Henderson, A. D., Böhm, M., Desai, A. S., Køber, L., et al. (2024). Asymptomatic vs symptomatic hypotension with sacubitril/valsartan in heart failure and reduced ejection fraction in PARADIGM-HF. J. Am. Coll. Cardiol. 84, 1685–1700. doi:10.1016/j.jacc.2024.08.012
McMurray, J. J. V., Packer, M., Desai, A. S., Gong, J., Lefkowitz, M. P., Rizkala, A. R., et al. (2014). Angiotensin-neprilysin inhibition versus enalapril in heart failure. N. Engl. J. Med. 371, 993–1004. doi:10.1056/NEJMoa1409077
Medina, D., and Arnold, A. C. (2019). Angiotensin-(1-7): translational avenues in cardiovascular control. Am. J. Hypertens. 32, 1133–1142. doi:10.1093/ajh/hpz146
Misumi, Y., Hayashi, Y., Arakawa, F., and Ikehara, Y. (1992). Molecular cloning and sequence analysis of human dipeptidyl peptidase IV, a serine proteinase on the cell surface. Biochimica Biophysica Acta (BBA) - Gene Struct. Expr. 1131, 333–336. doi:10.1016/0167-4781(92)90036-Y
Moreau, M. E., Garbacki, N., Molinaro, G., Brown, N. J., Marceau, F., and Adam, A. (2005). The kallikrein-kinin system: current and future pharmacological targets. J. Pharmacol. Sci. 99, 6–38. doi:10.1254/jphs.SRJ05001X
Moss, S., Subramanian, V., and Acharya, K. R. (2018). High resolution crystal structure of substrate-free human neprilysin. J. Struct. Biol. 204, 19–25. doi:10.1016/j.jsb.2018.06.004
Moss, S., Subramanian, V., and Acharya, K. R. (2020). Crystal structure of peptide-bound neprilysin reveals key binding interactions. FEBS Lett. 594, 327–336. doi:10.1002/1873-3468.13602
Mukhomedzyanov, A. V., Popov, S. V., Maslov, L. N., Naryzhnaya, N. V., Sirotina, M. A., Kurbatov, B. K., et al. (2024). Angiotensin 1-7 – a peptide that increases the resistance of the heart to ischemia and reperfusion: narrative review. SJCEM 39, 26–33. doi:10.29001/2073-8552-2024-39-3-26-33
Mukoyama, M., Nakao, K., Hosoda, K., Suga, S., Saito, Y., Ogawa, Y., et al. (1991). Brain natriuretic peptide as a novel cardiac hormone in humans. Evidence for an exquisite dual natriuretic peptide system, atrial natriuretic peptide and brain natriuretic peptide. J. Clin. Invest. 87, 1402–1412. doi:10.1172/JCI115146
Nalivaeva, N. N., Zhuravin, I. A., and Turner, A. J. (2020). Neprilysin expression and functions in development, ageing and disease. Mech. Ageing Dev. 192, 111363. doi:10.1016/j.mad.2020.111363
Norton, G., Woodiwiss, A. J., Hartford, C., Trifunovic, B., Middlemost, S., Lee, A., et al. (1999). Sustained antihypertensive actions of a dual angiotensin-converting enzyme neutral endopeptidase inhibitor, sampatrilat, in black hypertensive subjects. Am. J. Hypertens. 12, 563–571. doi:10.1016/S0895-7061(99)00009-6
O’Connell, J. E., Jardine, A. G., Davidson, G., and Connell, J. M. C. (1992). Candoxatril, an orally active neutral endopeptidase inhibitor, raises plasma atrial natriuretic factor and is natriuretic in essential hypertension. J. Hypertens. 10, 271–277. doi:10.1097/00004872-199203000-00011
Oefner, C., D’Arcy, A., Hennig, M., Winkler, F. K., and Dale, G. E. (2000a). Structure of human neutral endopeptidase (Neprilysin) complexed with phosphoramidon. J. Mol. Biol. 296, 341–349. doi:10.1006/jmbi.1999.3492
Oefner, C., D’Arcy, A., Hennig, M., Winkler, F. K., and Dale, G. E. (2000b). Structure of human neutral endopeptidase (Neprilysin) complexed with phosphoramidon. J. Mol. Biol. 296, 341–349. doi:10.1006/jmbi.1999.3492
Oefner, C., Roques, B. P., Fournie-Zaluski, M.-C., and Dale, G. E. (2004). Structural analysis of neprilysin with various specific and potent inhibitors. Acta Crystallogr. D. Biol. Crystallogr. 60, 392–396. doi:10.1107/S0907444903027410
Packer, M., Claggett, B., Lefkowitz, M. P., McMurray, J. J. V., Rouleau, J. L., Solomon, S. D., et al. (2018). Effect of neprilysin inhibition on renal function in patients with type 2 diabetes and chronic heart failure who are receiving target doses of inhibitors of the renin-angiotensin system: a secondary analysis of the PARADIGM-HF trial. Lancet Diabetes & Endocrinol. 6, 547–554. doi:10.1016/S2213-8587(18)30100-1
Parilla, J. H., Hull, R. L., and Zraika, S. (2018). Neprilysin deficiency is associated with expansion of islet β-cell mass in high fat-fed mice. J. Histochem Cytochem 66, 523–530. doi:10.1369/0022155418765164
Parvanova, A., Van Der Meer, I. M., Iliev, I., Perna, A., Gaspari, F., Trevisan, R., et al. (2013). Effect on blood pressure of combined inhibition of endothelin-converting enzyme and neutral endopeptidase with daglutril in patients with type 2 diabetes who have albuminuria: a randomised, crossover, double-blind, placebo-controlled trial. Lancet Diabetes & Endocrinol. 1, 19–27. doi:10.1016/S2213-8587(13)70029-9
Pathadka, S., Yan, V. K. C., Li, X., Tse, G., Wan, E. Y. F., Lau, H., et al. (2021). Hospitalization and mortality in patients with heart failure treated with sacubitril/valsartan vs. Enalapril: a real-world, population-based study. Front. Cardiovasc. Med. 7, 602363. doi:10.3389/fcvm.2020.602363
Pauptit, R. A., Karlsson, R., Picot, D., Jenkins, J. A., Niklaus-Reimer, A.-S., and Jansonius, J. N. (1988). Crystal structure of neutral protease from Bacillus cereus refined at 3.0A˚resolution and comparison with the homologous but more thermostable enzyme thermolysin. J. Mol. Biol. 199, 525–537. doi:10.1016/0022-2836(88)90623-7
Pavlovic, S., Liezmann, C., Blois, S. M., Joachim, R., Kruse, J., Romani, N., et al. (2011). Substance P is a key mediator of stress-induced protection from allergic sensitization via modified antigen presentation. J. Immunol. 186, 848–855. doi:10.4049/jimmunol.0903878
Pavo, N., Prausmüller, S., Bartko, P. E., Goliasch, G., and Hülsmann, M. (2020). Neprilysin as a biomarker: challenges and opportunities. Card. Fail Rev. 6, e23. doi:10.15420/cfr.2019.21
Pennant, W. A., An, S., Gwak, S.-J., Choi, S., Banh, D. T., Nguyen, A. B. L., et al. (2014). Local non-viral gene delivery of apoptin delays the onset of paresis in an experimental model of intramedullary spinal cord tumor. Spinal Cord 52, 3–8. doi:10.1038/sc.2013.106
Poirier, R., Wolfer, D. P., Welzl, H., Tracy, J., Galsworthy, M. J., Nitsch, R. M., et al. (2006). Neuronal neprilysin overexpression is associated with attenuation of Abeta-related spatial memory deficit. Neurobiol. Dis. 24, 475–483. doi:10.1016/j.nbd.2006.08.003
Polna, I., and Aleksandrowicz, J. (1975). Effect of adsorbents on IgM and IgG measles antibodies. Acta Virol. 19, 449–456.
Ponikowski, P., Voors, A. A., Anker, S. D., Bueno, H., Cleland, J. G. F., Coats, A. J. S., et al. (2016). 2016 ESC Guidelines for the diagnosis and treatment of acute and chronic heart failure: the Task Force for the diagnosis and treatment of acute and chronic heart failure of the European Society of Cardiology (ESC)Developed with the special contribution of the Heart Failure Association (HFA) of the ESC. Eur. Heart J. 37, 2129–2200. doi:10.1093/eurheartj/ehw128
Prasad, T., Roksnoer, L. C. W., Zhu, P., Verma, A., Li, Y., Batenburg, W. W., et al. (2016). Beneficial effects of combined at 1 receptor/neprilysin inhibition (ARNI) versus at 1 receptor blockade alone in the diabetic eye. Invest. Ophthalmol. Vis. Sci. 57, 6722–6730. doi:10.1167/iovs.16-20289
Rajcáni, J., Krobová, J., and Málková, D. (1975). Distribution of Lednice (Yaba 1) virus in the chick embryo. Acta Virol. 19, 467–472.
Riddell, E., and Vader, J. M. (2017). Potential expanded indications for neprilysin inhibitors. Curr. Heart Fail Rep. 14, 134–145. doi:10.1007/s11897-017-0327-y
Robl, J. A., Sun, C.-Q., Stevenson, J., Ryono, D. E., Simpkins, L. M., Cimarusti, M. P., et al. (1997). Dual metalloprotease inhibitors: mercaptoacetyl-based fused heterocyclic dipeptide mimetics as inhibitors of angiotensin-converting enzyme and neutral endopeptidase. J. Med. Chem. 40, 1570–1577. doi:10.1021/jm970041e
Roques, B. P., Fournié-Zaluski, M. C., Soroca, E., Lecomte, J. M., Malfroy, B., Llorens, C., et al. (1980). The enkephalinase inhibitor thiorphan shows antinociceptive activity in mice. Nature 288, 286–288. doi:10.1038/288286a0
Rousso, P., Buclin, T., Nussberger, J., Décosterd, L. A., La Roche, S. D., Brunner-Ferber, F., et al. (1999). Effects of a dual inhibitor of angiotensin converting enzyme and neutral endopeptidase, MDL 100 240, on endocrine and renal functions in healthy volunteers. J. Hypertens. 17, 427–437. doi:10.1097/00004872-199917030-00017
Ruilope, L. M., Dukat, A., Böhm, M., Lacourcière, Y., Gong, J., and Lefkowitz, M. P. (2010). Blood-pressure reduction with LCZ696, a novel dual-acting inhibitor of the angiotensin II receptor and neprilysin: a randomised, double-blind, placebo-controlled, active comparator study. Lancet 375, 1255–1266. doi:10.1016/S0140-6736(09)61966-8
Ryu, H., Kim, J., Kang, E., Hong, Y., Chae, D.-W., Choi, K. H., et al. (2021). Incidence of cardiovascular events and mortality in Korean patients with chronic kidney disease. Sci. Rep. 11, 1131. doi:10.1038/s41598-020-80877-y
Sahli, S., Frank, B., Schweizer, W. B., Diederich, F., Blum-Kaelin, D., Aebi, J. D., et al. (2005). Second-generation inhibitors for the metalloprotease neprilysin based on bicyclic heteroaromatic scaffolds: synthesis, biological activity, and X-ray crystal-structure analysis. Helvetica Chim. Acta 88, 731–750. doi:10.1002/hlca.200590051
Sargın, Z. G., Erin, N., Tazegul, G., Elpek, G. Ö., and Yıldırım, B. (2017). Profound loss of neprilysin accompanied by decreased levels of neuropeptides and increased CRP in ulcerative colitis. PLoS ONE 12, e0189526. doi:10.1371/journal.pone.0189526
Savarese, G., Kishi, T., Vardeny, O., Adamsson Eryd, S., Bodegård, J., Lund, L. H., et al. (2023). Heart Failure drug treatment-Inertia, Titration, and discontinuation: a multinational observational study (evolution HF). JACC Heart Fail. 11, 1–14. doi:10.1016/j.jchf.2022.08.009
Schiering, N., D’Arcy, A., Villard, F., Ramage, P., Logel, C., Cumin, F., et al. (2016). Structure of neprilysin in complex with the active metabolite of sacubitril. Sci. Rep. 6, 27909. doi:10.1038/srep27909
Schwartz, J.-C. (2000). Racecadotril: a new approach to the treatment of diarrhoea. Int. J. Antimicrob. Agents 14, 75–79. doi:10.1016/S0924-8579(99)00151-X
Seed, A., Kuc, R. E., Maguire, J. J., Hillier, C., Johnston, F., Essers, H., et al. (2012). The dual endothelin converting enzyme/neutral endopeptidase inhibitor SLV-306 (daglutril), inhibits systemic conversion of big endothelin-1 in humans. Life Sci. 91, 743–748. doi:10.1016/j.lfs.2012.03.022
Seferovic, P. M., Ponikowski, P., Anker, S. D., Bauersachs, J., Chioncel, O., Cleland, J. G. F., et al. (2019). Clinical practice update on heart failure 2019: pharmacotherapy, procedures, devices and patient management. An expert consensus meeting report of the Heart Failure Association of the European Society of Cardiology. Eur. J. Heart Fail 21, 1169–1186. doi:10.1002/ejhf.1531
Sharma, U., Cozier, G. E., Sturrock, E. D., and Acharya, K. R. (2020). Molecular basis for omapatrilat and sampatrilat binding to neprilysin—implications for dual inhibitor design with angiotensin-converting enzyme. J. Med. Chem. 63, 5488–5500. doi:10.1021/acs.jmedchem.0c00441
Shoji, S., Cyr, D. D., Hernandez, A. F., Velazquez, E. J., Ward, J. H., Williamson, K. M., et al. (2024). Win ratio analyses using a modified hierarchical composite outcome: insights from PARAGLIDE-HF. Am. Heart J. S0002-8703 (24), 00282–00285. doi:10.1016/j.ahj.2024.10.020
Skidgel, R. A., Engelbrecht, S., Johnson, A. R., and Erdös, E. G. (1984). Hydrolysis of substance P and neurotensin by converting enzyme and neutral endopeptidase. Peptides 5, 769–776. doi:10.1016/0196-9781(84)90020-2
Smits, M. M., Galsgaard, K. D., Jepsen, S. L., Albrechtsen, N. W., Hartmann, B., and Holst, J. J. (2024). In vivo inhibition of dipeptidyl peptidase 4 allows measurement of GLP-1 secretion in mice. Diabetes 73, 671–681. doi:10.2337/db23-0848
Southwood, T. R., Khalaf, S., and Sinden, R. E. (1975). The micro-organisms of tsetse flies. Acta Trop. 32, 259–266.
Steele, I. C., McDOWELL, G., Moore, A., Campbell, N. P. S., Shaw, C., Buchanan, K. D., et al. (1997). Responses of atrial natriuretic peptide and brain natriuretic peptide to exercise in patients with chronic heart failure and normal control subjects. Eur. J. Clin. Investig. 27, 270–276. doi:10.1046/j.1365-2362.1997.1070653.x
Stephenson, S. L., and Kenny, A. J. (1987). The hydrolysis of α -human atrial natriuretic peptide by pig kidney microvillar membranes is initiated by endopeptidase-24.11. Biochem. J. 243, 183–187. doi:10.1042/bj2430183
Stuss, D. T. (2015). From silos to systems: an integrated approach to neuroscience innovation. Nat. Rev. Drug Discov. 14, 295–296. doi:10.1038/nrd4615
Taal, M. W., Nenov, V. D., Wong, W., Satyal, S. R., Sakharova, O., Choi, J. H., et al. (2001). Vasopeptidase inhibition affords greater renoprotection than angiotensin-converting enzyme inhibition alone. J. Am. Soc. Nephrol. 12, 2051–2059. doi:10.1681/ASN.V12102051
Tarun, T., Ghanta, S. N., Ong, V., Kore, R., Menon, L., Kovesdy, C., et al. (2024). Updates on new therapies for patients with CKD. Kidney Int. Rep. 9, 16–28. doi:10.1016/j.ekir.2023.10.006
Ter Beek, W. P., Biemond, I., Muller, E. S. M., Van Den Berg, M., and Lamers, C. B. H. W. (2007). Substance P receptor expression in patients with inflammatory bowel disease. Determination by three different techniques, i.e., storage phosphor autoradiography, RT-PCR and immunohistochemistry. Neuropeptides 41, 301–306. doi:10.1016/j.npep.2007.05.002
Tiraboschi, G., Jullian, N., Thery, V., Antonczak, S., Fournie-Zaluski, M.-C., and Roques, B. P. (1999). A three-dimensional construction of the active site (region 507–749) of human neutral endopeptidase (EC.3.4.24.11). Protein Eng. Des. Sel. 12, 141–149. doi:10.1093/protein/12.2.141
Tomson, C. R. V., Cheung, A. K., Mann, J. F. E., Chang, T. I., Cushman, W. C., Furth, S. L., et al. (2021). Management of blood pressure in patients with chronic kidney disease not receiving dialysis: synopsis of the 2021 KDIGO clinical practice guideline. Ann. Intern Med. 174, 1270–1281. doi:10.7326/M21-0834
Trippodo, N. C., and Barbee, R. W. (1987). Atrial natriuretic factor decreases whole-body capillary absorption in rats. Am. J. Physiology-Regulatory, Integr. Comp. Physiology 252, R915–R920. doi:10.1152/ajpregu.1987.252.5.R915
Tsukamoto, S., Uehara, T., Azushima, K., Wakui, H., and Tamura, K. (2023). Updates for cardio-kidney protective effects by angiotensin receptor-neprilysin inhibitor: requirement for additional evidence of kidney protection. J. Am. Heart Assoc. 12, e029565. doi:10.1161/JAHA.122.029565
Tsutsui, H., Momomura, S., Saito, Y., Ito, H., Yamamoto, K., Sakata, Y., et al. (2021). Efficacy and safety of sacubitril/valsartan in Japanese patients with chronic heart failure and reduced ejection fraction ― results from the PARALLEL-HF study. Circ. J. 85, 584–594. doi:10.1253/circj.CJ-20-0854
Turner, A. J., Isaac, R. E., and Coates, D. (2001). The neprilysin (NEP) family of zinc metalloendopeptidases: genomics and function. Bioessays 23, 261–269. doi:10.1002/1521-1878(200103)23:3<261::AID-BIES1036>3.0.CO;2-K
Venn, R. F., Barnard, G., Kaye, B., Macrae, P. V., and Saunders, K. C. (1998a). Clinical analysis of sampatrilat, a combined renal endopeptidase and angiotensin-converting enzyme inhibitor II: assay in the plasma and urine of human volunteers by dissociation enhanced lanthanide fluorescence immunoassay (DELFIA). J. Pharm. Biomed. Analysis 16, 883–892. doi:10.1016/S0731-7085(97)00127-1
Venn, R. F., Kaye, B., Macrae, P. V., and Saunders, K. C. (1998b). Clinical analysis of sampatrilat, a combined renal endopeptidase and angiotensin-converting enzyme inhibitor I: assay in plasma of human volunteers by atmospheric-pressure ionisation mass-spectrometry following derivatisation with BF3-methanol. J. Pharm. Biomed. Analysis 16, 875–881. doi:10.1016/S0731-7085(97)00126-X
Vu, J. P., Million, M., Larauche, M., Luong, L., Norris, J., Waschek, J. A., et al. (2014). Inhibition of vasoactive intestinal polypeptide (VIP) induces resistance to dextran sodium sulfate (DSS)-Induced colitis in mice. J. Mol. Neurosci. 52, 37–47. doi:10.1007/s12031-013-0205-3
Wallis, E. J., Ramsay, L. E., and Hettiarachchi, J. (1998). Combined inhibition of neutral endopeptidase and angiotensin-converting enzyme by sampatrilat in essential hypertension. Clin. Pharmacol. Ther. 64, 439–449. doi:10.1016/S0009-9236(98)90075-3
Wilkins, M. R., Settle, S. L., Kirk, J. E., Taylor, S. A., Moore, K. P., and Unwin, R. J. (1992). Response to atrial natriuretic peptide, endopeptidase 24.11 inhibitor and C-ANP receptor ligand in the rat. Br. J Pharmacol. 107, 50–57. doi:10.1111/j.1476-5381.1992.tb14462.x
Willard, J. R., Barrow, B. M., and Zraika, S. (2017). Improved glycaemia in high-fat-fed neprilysin-deficient mice is associated with reduced DPP-4 activity and increased active GLP-1 levels. Diabetologia 60, 701–708. doi:10.1007/s00125-016-4172-4
Yamamoto, K., and Rakugi, H. (2021). Angiotensin receptor-neprilysin inhibitors: comprehensive review and implications in hypertension treatment. Hypertens. Res. 44, 1239–1250. doi:10.1038/s41440-021-00706-1
Yandle, T. G., Richards, A. M., Nicholls, M. G., Cuneo, R., Espiner, E. A., and Livesey, J. H. (1986). Metabolic clearance rate and plasma half life of alpha-human atrial natriuretic peptide in man. Life Sci. 38, 1827–1833. doi:10.1016/0024-3205(86)90137-2
Yiannopoulou, K. G., and Papageorgiou, S. G. (2020). Current and future treatments in alzheimer disease: an update. J. Cent. Nerv. Syst. Dis. 12, 1179573520907397. doi:10.1177/1179573520907397
Yoon, M., Kim, W., Kook, W., Park, J. J., and Greenberg, B. (2024). Analysis of the PARAGON-HF study results using win ratio. Circ. Heart Fail 17, e011860. doi:10.1161/CIRCHEARTFAILURE.124.011860
Zraika, S., Koh, D.-S., Barrow, B. M., Lu, B., Kahn, S. E., and Andrikopoulos, S. (2013). Neprilysin deficiency protects against fat-induced insulin secretory dysfunction by maintaining calcium influx. Diabetes 62, 1593–1601. doi:10.2337/db11-1593
Glossary
NEP neprilysin
NEPI NEP inhibitor
NP natriuretic peptides
Ang I angiotensin I
Ang II angiotensin II
Ang-(1-7) angiotensin-(1-7)
ACE2 angiotensin-converting enzyme 2
ADM adrenomedullin
BK bradykinin
NEPI Neprilysin inhibitors
AD Alzheimer’s disease
Aβ β-amyloid
SAR structure-activity relationships
ET-1 endothelin-1
GLP-1 glucagon-like peptide-1
DPP4 dipeptidyl peptidase-4
DN Diabetic nephropathy
RAS renin-angiotensin system
NPS natriuretic peptide system
CKD chronic kidney disease
ARNI angiotensin receptor-neprilysin inhibitor
DR Diabetic retinopathy
ARBs angiotensin receptor blockers
SP substance P
VIP Vasoactive Intestinal Peptide
NPY neuropeptide Y
HF Heart failure
ACEI ngiotensin-converting enzyme inhibitors
ARB angiotensin II receptor blockers
RAAS renin-angiotensin-aldosterone system
HFrEF Heart failure with a reduced ejection fraction
ANF atrial natriuretic factor
ANP atrial natriuretic peptide
BNP brain natriuretic peptide
Keywords: neprilysin, NEP inhibitor, structure-activity relationship, drug design, clinical
Citation: Zhang X, Hu C, Tian E, Shen Y, Liu W and Li J (2024) Comprehensive review on neprilysin (NEP) inhibitors: design, structure-activity relationships, and clinical applications. Front. Pharmacol. 15:1501407. doi: 10.3389/fphar.2024.1501407
Received: 27 September 2024; Accepted: 18 November 2024;
Published: 23 December 2024.
Edited by:
Ali H. Eid, Qatar University, QatarReviewed by:
Ibrahim Fadil Benter, Final International University, CyprusMark C. Chappell, Wake Forest University, United States
Vajir M. Malek, Beckman Research Institute, United States
Elena Kaschina, Charité University Medicine Berlin, Germany
Copyright © 2024 Zhang, Hu, Tian, Shen, Liu and Li. This is an open-access article distributed under the terms of the Creative Commons Attribution License (CC BY). The use, distribution or reproduction in other forums is permitted, provided the original author(s) and the copyright owner(s) are credited and that the original publication in this journal is cited, in accordance with accepted academic practice. No use, distribution or reproduction is permitted which does not comply with these terms.
*Correspondence: Wei Liu, bGl1d2VpXzIwMjEwNTI1QHFxLmNvbQ==; Juan Li, bGlqdWFuQHNjdS5lZHUuY24=