- 1Pharmacy Department, Clinical Trial Institution, The People’s Hospital of Zhongjiang, Deyang, China
- 2West China School of Medicine, Sichuan University, Chengdu, China
- 3Molecular Urooncology, Department of Urology, Klinikum Rechts der Isar, Technical University of Munich, München, Germany
- 4Department of Oncology, 363 Hospital, Chengdu, China
In recent years, researchers have highly observed that neurological disorders (NSDs) with the aging of the population are a global health burden whose prevalence is increasing every year. Previous evidence suggested that the occurrence of neurological disorders is correlated with predisposing factors such as inflammation, aging, and injury. Particularly, the neuronal cells are susceptible to oxidative stress, leading to lesions caused by high oxygen-consuming properties. Oxidative stress (OS) is a state of peroxidation, which occurs as a result of the disruption of the balance between oxidizing and antioxidizing substances. The oxidative intermediates such as free radicals, hydrogen peroxide (H2O2), and superoxide anion (O2-) produced by OS promote disease progression. Curcumin, a natural diketone derived from turmeric, is a natural antioxidant with a wide range of neuroprotective, anti-inflammatory, anti-tumor, anti-aging, and antioxidant effects. Fortunately, curcumin is recognized for its potent antioxidant properties and is considered a promising candidate for the prevention and treatment of neurological diseases. Consequently, this review elucidates the mechanisms by which curcumin mitigates oxidative stress and emphasizes the potential in treating nervous system disorders, including depression, Alzheimer’s disease, Parkinson’s disease, epilepsy, subarachnoid hemorrhage, and glioblastoma. We aim to provide a new therapeutic option for the management of neurological diseases.
Introduction
Neurological disorders are diseases characterized by neurological damage and functional impairments resulting from various etiologies. These disorders can affect individuals at any age and are particularly prevalent among the elderly. As the global population ages, the number of individuals suffering from neurological disorders is expected to rise, placing significant strain on both human health and economic resources (Birbeck et al., 2015). Clinical manifestations of neurological disorders primarily include neurodegenerative diseases, epilepsy, arachnoid hemorrhage, and glioma. The etiology of these disorders is complex, involving factors such as genetic inheritance, oxidative stress, infections, trauma, cerebrovascular lesions, and metabolic abnormalities (Valori et al., 2019; Nimgampalle et al., 2023; Tang et al., 2023). Currently, most neurological disorders, especially neurodegenerative diseases like Alzheimer’s and Parkinson’s are considered irreversible (Abdolmaleky and Zhou, 2023). For instance, the different forms of amyloid β-protein (Ab) linked with the decreased overproduction, aberrant aggregation, or elimination are key events in the pathogenesis of Alzheimer’s disease (AD) (Ferrari and Sorbi, 2021; Fang et al., 2019), and oxidative stress may contribute to the developmental process (Monteiro et al., 2023; Ullah et al., 2024). Meanwhile, the occurrence of depression involves complex molecular mechanisms related to dysfunction of the monoaminergic nervous system, abnormality of the hypothalamic pituitary adrenal axis, imbalance of calcium homeostasis and calcium signaling pathways, mitochondrial dysfunction, autophagy, cell apoptosis, and oxidative stress (Bansal and Kuhad, 2016; Villa et al., 2018; Bhatt et al., 2020). Moreover, the clinical therapy of neurological disorders tends to be largely symptomatic due to the intricate etiology, suffering from the disadvantages of side effects, drug resistance, and insensitivity (Nimgampalle et al., 2023). Antidepressants commonly used in clinic, such as tricyclic antidepressants, monoamine oxidase inhibitors, selective 5-HT reuptake inhibitors, and 5-HT-norepinephrine reuptake inhibitors, have side effects as dry mouth, blurred vision, inability to drive, sexual dysfunction, headache, gastrointestinal symptoms, anxiety, and agitation (Barkin and Fawcett, 2000; Feighner, 1999; Fava, 2000). Unfortunately, the most existing therapy modalities for PD only target symptoms. Dopamine supplements temporarily control motor dysfunction caused by degeneration of the dopaminergic nigrostriatal system. Meanwhile, the drugs in PD including levodopa, dopamine agonists, COMT inhibitors, MAO-B inhibitors, anticholinergics, and amantadine still have unavoidable side effects as motor complications, hallucinations, insomnia, gastrointestinal disturbances, cognitive deficits, dry mouth, and constipation (Khanam and Siddique, 2018; Kwon et al., 2022; Faulkner, 2014). Consequently, research into the pathogenesis of various neurological disorders and more effective pharmacological interventions has become a major focus within the field of neurology.
Redox reactions constitute the primary chemical basis for energy conversion and metabolism in humans. Unfortunately, oxidative stress resulting from an imbalance between oxidative and antioxidant effects could lead to a variety of negative outcomes (Jones, 2024). Free radicals and reactive oxygen species (ROS), including superoxide anions (O2−), hydrogen peroxide (H2O2), and hydroxyl radicals (·OH) are the principal contributors to oxidative stress in the body. These species are correlated with electron transfer and the generation of various active intermediates through chemical reactions. Mitochondria, as crucial organelles for ATP production, are significant producers of reactive oxygen species. Fortunately, various reductases such as superoxide dismutase (SOD), catalase (CAT), glutathione peroxidase (GSH-Px), along with reducing compounds-glutathione, vitamin C, and vitamin E, help mitigate the harmful effects of oxidative stress in vivo (Sies, 2021). The nervous system is particularly vulnerable to oxidative stress due to the high oxygen consumption and susceptibility to depletion (Jones, 2015; Tonnies and Trushina, 2017; Maiese, 2023). In recent years, the impact of oxidative stress on various neurological disorders has been the focus of extensive research. Notably, oxidative stress in the context of neurodegenerative diseases has been shown to induce neuronal cell death and abnormal protein aggregation (Birla et al., 2020), which are key predisposing factors. However, the development of drugs targeting oxidative stress remains a challenge at this stage.
Curcumin (CU), a diketone compound derived from turmeric, has been widely utilized globally as a natural pigment, which has demonstrated various pharmacological effects, including anti-inflammatory, immunomodulatory, antioxidant, and anti-tumor properties (Kocaadam and Sanlier, 2017; Zia et al., 2021). Notably, the diketone structure of CU, characterized by its excellent reducing properties, serves as a primary basis for its antioxidant action (Nelson et al., 2017). Recent studies indicate that CU inhibits neuronal damage caused by oxidative stress and effectively mitigates disease progression by eliminating free radicals and ROS while enhancing reductase activity (Samarghandian et al., 2017; Sarawi et al., 2021). Furthermore, the beneficial effects of CU on oxidative stress have been corroborated by clinical trials. This study reviews the pharmacological mechanisms through which CU acts as an antioxidant, particularly in alleviating the detrimental effects of oxidative stress on neurodegenerative diseases, which searched for relevant studies on ‘curcumin, oxidative stress, nervous system diseases, depression, Alzheimer’s disease, Parkinson’s disease, epilepsy, subarachnoid hemorrhage, and glioma’ by PubMed, Web of Science, and CNKI databases. Additionally, the findings from clinical trials involving CU in neurodegenerative diseases are discussed to provide a foundation for future research on the antioxidant effects of CU in this context.
Physicochemical properties and metabolism
Curcumin, with the molecular formula C21H20O6, exhibits a polyphenolic chemical structure. The lipid-water partition coefficient of CU is LogP: 3.2, indicating extremely low solubility in water, while it is soluble in organic solvents such as DMSO, methanol, and acetone (Payton et al., 2007). Notably, CU possesses enolization properties due to its β-dicarbonyl structural unit, which contributes to its instability and irregular metabolism. However, the 1,3-diketone structure is also a crucial motif for protecting nerve cells from oxidative stress (LoPachin et al., 2011). In acidic or neutral solvents, CU primarily exists in its ketone form, while in alkaline solvents, acidic structures predominate, enhancing stability (Priyadarsini, 2014). Interestingly, the electron cloud conjugation effect of CU increases with solvent basicity, leading to pronounced deprotonation, which enhances solubility but also increases susceptibility to degradation (Ghosh et al., 2023; Pan et al., 2014). Consequently, due to these properties such as solubility and stability in various solvents, CU exhibits low bioavailability in the human body (Figure 1), necessitating a more complex mode of administration. A peak serum concentration of 0.22 μg/mL was observed 1 h after oral administration at a dose of 1.0 g/kg in a mouse model, while a peak concentration of 2.25 μg/mL was recorded 0.25 h after intraperitoneal administration at the same dose (Pan et al., 1999). Additionally, the area under the curve (AUC) for CU in rats was found to be 3.6 ± 0.6 min/μg/mL following oral administration of 500 mg/kg, and only 7.2 ± 1.2 min/μg/mL after intravenous administration of 10 mg/kg, further indicating poor absorption characteristics (Yang et al., 2007). Studies utilizing radioactive elements to investigate the distribution of CU have identified its presence in the liver, heart, kidneys, brain, lungs, and muscles of mice administered intraperitoneally (Perkins et al., 2002). Notably, Pan et al. (1999) demonstrated that CU can penetrate the brain, albeit at a concentration of only 0.4 ± 0.01 μg/g. These findings further substantiate the notion that CU is capable of crossing the blood-brain barrier, which is critical in the context of various neurological disorders, thereby exerting pharmacological effects. The liver serves as the primary organ for CU metabolism, which occurs through two principal pathways: O-substitution and reduction. The phenolic hydroxyl group of CU interacts with glucuronic acid and sulfate, resulting in the formation of CU glucosinolate and CU sulfate, respectively. The human phenol sulfotransferase isozymes SULT1A1 and SULT1A3 play essential roles in the sulfate substitution of CU (Ireson et al., 2002). Concurrently, the UDP enzyme family, a group of glucuronosyltransferases, facilitates the glucuronosyl substitution of CU in the human liver and gastrointestinal tract (Hoehle et al., 2007). The NADPH enzyme is responsible for reducing CU to dihydrocurcumin, which can further convert to tetrahydrocurcumin within the reduction pathway (Wang et al., 2021). Interestingly, these reduction products can undergo subsequent reduction reactions, yielding hexahydrocurcumin, octahydrocurcumin, and various ferulic acid analogs (Ireson et al., 2001). The elimination of CU is closely associated with the route of administration, as the majority of orally administered CU is excreted in feces. A clinical trial investigating long-term oral administration revealed that CU was present in the feces of subjects, while it was not detected in urine (Sharma et al., 2001). Furthermore, the studies revealed the presence of CU glucosinolates and sulfates in the urine of rats; however, the parent compound was not detected in the urine (Ravindranath and Chandrasekhara, 1980). A clinical study also demonstrated that CU was virtually undetectable in urine, with only the glucuronide conjugate observed (Irving et al., 2013). Notably, rats administered CU via intraperitoneal or intravenous routes exhibited biliary excretion (Holder et al., 1978; Lee et al., 2012). These physicochemical properties and the pharmacokinetic profile of CU in vivo indicate low bioavailability. Consequently, researchers have developed new formulations aimed at improving the half-life and bioavailability of CU.
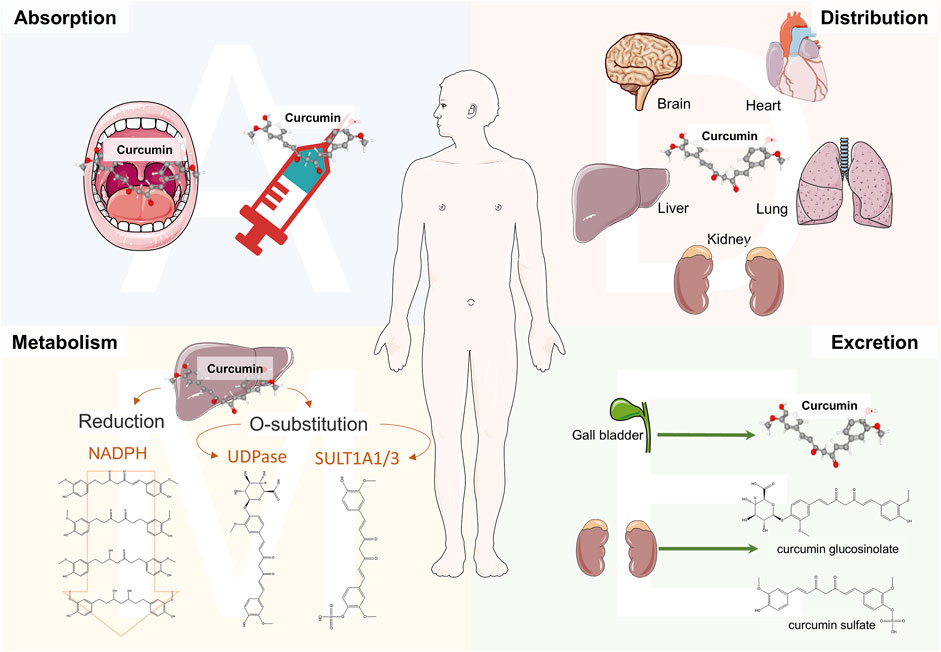
Figure 1. Schematic diagram of the pharmacokinetics of curcumin in vivo. The administration methods of curcumin (CU) include oral and the injection of the vein and abdominal cavity. CU entering the body is mainly distributed in the liver, kidneys, brain, and lungs. Particularly, CU could cross the blood-brain barrier in the brain, which is the basis for treating neurological disorders. The liver is the main metabolizing organ for curcumin and can generate a range of metabolites such as CU glucosinolates, CU sulfates, and hydrogenated curcuminoids by O-substitution and reduction pathways. Renal excretion is the metabolic pathway for CU glucosinolate and CU sulfate. Meanwhile, CU can be excreted through bile.
Depression
Depression is a prevalent mental disorder characterized by a persistent low mood and associated mental symptoms, including self-harm and suicidal ideation (Martino et al., 2022; Boumparis et al., 2016; McCarron et al., 2021). Currently, researchers have identified several factors that may contribute to the etiology of depression, including biochemical, neuroendocrine, neuroimmunological, genetic, and psychosocial influences (Menard et al., 2016; Penner-Goeke and Binder, 2019; Idunkova et al., 2023). However, the specific pathogenesis of the disorder remains unclear. Oxidative stress has long been recognized as a significant factor influencing depression. The phosphorylation of ATP within cells generates reactive species, including ROS, reactive nitrogen species, and free radicals (Brookes et al., 2004; Strzyz, 2022; Bando et al., 2021; Leite-Aguiar et al., 2024). While ROS plays a crucial role in the growth and development of nerve cells under physiological conditions, an excess of ROS can disrupt the balance of antioxidants, leading to oxidative stress, which adversely affects normal physiological functions. Glutathione (GSH) is an important antioxidant that scavenges ROS and free radicals, thereby preventing cellular peroxidation (Liu et al., 2022). The oxidized form of GSH can be regenerated into its reduced form by the action of reductases and NADPH (Zeeshan et al., 2016). Additionally, superoxide dismutase (SOD) catalyzes the conversion of superoxide radicals into hydrogen peroxide (H2O2), which is subsequently degraded by catalase. Recent studies have increasingly focused on the roles of GSH and SOD in the context of depression (Gibson et al., 2012; Camkurt et al., 2016). The brain, being the most oxygen-demanding organ, is particularly vulnerable to oxidative stress due to its high content of nerve cells and the abundance of neurofunctional substances such as lipids and proteins that are prone to oxidative damage (Hulbert et al., 2007). The peroxidation of these substances results in oxidation products that contribute to physiological processes, including decreased cellular function and cell death. Research indicates that levels of malondialdehyde (MDA) in the brains of patients with depression are higher than those in healthy individuals; notably, MDA is produced through lipid oxidation in nerve cells (Maes et al., 2011; Almulla et al., 2023). Nitric oxide (NO), a product of L-proline oxidation, is also found at elevated levels in depressed patients (Salim, 2017; Piper et al., 2023). Further studies have demonstrated that the inhibition of NO production can mimic the effects of antidepressants (Ghasemi, 2019). Additionally, the inhibitory effect of peroxides on telomerase represents a significant cause of cellular DNA damage. The 8-hydroxy-2′-deoxyguanosine (8-OHdG) is a modification product resulting from oxidative DNA damage induced by ROS. Research has shown that individuals with depression, particularly those experiencing recurrent episodes, exhibit significantly elevated levels of 8-OHdG compared to normal levels (Omari Shekaftik and Nasirzadeh, 2021; Syafrita et al., 2020). This finding suggests a strong positive correlation between oxidative stress and DNA damage in depressed patients.
Curcumin, a natural antioxidant, influences various oxidative reactions within the body. Previous studies have indicated that depression is often associated with decreased levels of antioxidants. The current study demonstrated that the depressive behavior of rats infected with Toxoplasma gondii improved following the administration of CU (Moradi et al., 2023). Additionally, this research revealed an increased expression of GSH and SOD enzymes in the hippocampus of the rat brain, along with a reduction in MDA levels, thereby confirming that CU effectively mitigates the impact of oxidative stress on depression. Nrf2, a key endogenous antioxidant transcription factor, is activated by the dissociation of the Keap1 protein, subsequently entering the nucleus to regulate the expression of antioxidant genes in conjunction with ARE elements (Vriend and Reiter, 2015). Furthermore, studies have identified Nrf2 as a promising antioxidant target for depression treatment (Dang et al., 2022). Continuous administration of CU at a dosage of 100 mg/kg/day for 28 days in CUMS model rats significantly alleviated depressive behaviors, including those observed in the SPT, forced swimming, and novelty inhibition tests (Liao et al., 2020). Further mechanistic studies have demonstrated that CU intake in rats leads to an increase in oxidative stress markers, including 8-OHdG, Nox2, and 4-HNE. Notably, prolonged CU intake reversed the inhibition of Nrf2 protein and activated the expression of the antioxidant enzymes NQO-1 and HO-1 via the Nrf2-AEB pathway in a depressed model of animals. A lipopolysaccharide-induced model of moderate depression (MDD) in rats further illustrated that CU enhances antioxidant activity by elevating Nrf2 expression (Fan et al., 2021). Additionally, this study confirmed that CU reduced the rise in miR-146a-5p levels and reversed the decrease in the expression of the p-ERK signaling pathway in glial cells, thereby protecting synaptic neurons in the CA1 region of the hippocampus. To address the low activity associated with curcumin’s low bioavailability, curcumin was formulated into nanocapsules, which proved more effective in enhancing antioxidant substances, such as the SOD enzyme, in Aβ25-35 protein-induced model mice (Fidelis et al., 2019). Furthermore, curcumin-zinc oxide nanoparticles significantly increased GSH concentrations in the cerebral cortex, hippocampus, and striatum compared to curcumin alone, and more effectively reduced MDA levels (Fahmy et al., 2024).
Multiple clinical trials have demonstrated the positive effects of CU on depression (Table 1). Notably, CU exhibits significant efficacy in major depressive disorder (MDD), whether administered alone or in combination with other antidepressants or monomers (Lopresti and Drummond, 2017; Sanmukhani et al., 2014). Combined CU extract plus saffron (15 mg bid) in a randomized, double-blind, placebo-controlled studyfor 12 weeks was associated with significantly greater improvements in depressive symptoms compared with placebo (p = 0.031), which the Spielberger State-Trait Anxiety Inventory state (STAI-S) scores (p < 0.001) and STAI-trait (STAI-T) scores (p = 0.001) decreased significantly. A 6-week clinical trial using fluoxetine as a positive control found that oral administration of CU at a dose of 1,000 mg/day produced effects comparable to those of 20 mg/day fluoxetine, with response rates on the 17-item Hamilton Depression Rating Scale (HAM-D17) exceeding 60% for both treatments, significantly outperforming placebo (12.5%–51.8%) (Sanmukhani et al., 2014). Interestingly, when CU was combined with fluoxetine, the HAM-D17 score of the subjects reached 77.8%, although this result was not statistically different from the effects of CU or fluoxetine alone. Additionally, CU has been shown to positively impact depression associated with other medical conditions. For instance, a study indicated that patients with diabetes and peripheral neuropathy who ingested 80 mg/day of Nano-curcumin for 8 weeks exhibited improved depression and anxiety scores compared to those receiving a placebo (Asadi et al., 2020). Furthermore, a dosage of 1 g/day of CU led to improvements in depression and behaviors associated with coke oven exposure in obese patients, as evidenced by a significant decrease in their Beck Anxiety Inventory (BAI) scores (Esmaily et al., 2015). Notably, CU also alleviates depression and anxiety-related symptoms in healthy menopausal women, with observed decreases in MDA levels and improvements in antioxidant capacity (Farshbaf-Khalili et al., 2022). This study further supports the notion that CU alleviates depression in humans by inhibiting oxidative stress.
Alzheimer’s disease
Alzheimer’s disease (AD) is a prevalent neurodegenerative disorder that leads to significant cognitive impairment, particularly in the elderly (Scheltens et al., 2021). The etiology of AD remains poorly understood (Rostagno, 2022). Generally, the primary pathological features of AD include the accumulation of Aβ protein, hyperphosphorylation of tau protein, and the formation of neurofibrillary tangles (Ferrari and Sorbi, 2021). The brain is an organ that is highly susceptible to oxygen consumption in humans, utilizing approximately 20% of the body’s oxygen (Ballance et al., 2019). Notably, neurons in the brain contain a substantial amount of lipids, nucleic acids, and proteins, all of which are particularly vulnerable to oxidation by reactive compounds in the body, leading to functional disorders in cells. Due to these characteristics, neurons are more susceptible to oxidative stress, which may induce a range of nervous system diseases, especially in the context of AD (Pratico, 2008) (Figure 2). Research has shown that oxidative stress can lead to an increase in phosphorylated tau protein through the P38/JNK pathway (Su et al., 2010). Additionally, oxidative stress promotes the cross-linking of tau protein with dityrosine (DIY), resulting in the formation of copolymers (Maina et al., 2021). These findings underscore the connection between oxidative stress and tau protein in the pathogenesis of AD. Previous studies have also reported that oxidative stress facilitates the deposition of amyloid beta (Aβ) (Andorn and Kalaria, 2000; Pratico et al., 2002). The Aβ peptide generates reactive oxygen species in the presence of transition metals such as copper and iron. Furthermore, similar to the tau protein, Aβ can form stable dityrosine cross-linked dimers with DIY under oxidative stress conditions, which exacerbates the detrimental effects of oxidative stress on neuronal cells (Smith et al., 2007). Most clinical trials have demonstrated that levels of oxidative coenzyme Q (an early marker of oxidative stress), 8-hydroxy-20-deoxyguanosine (a marker of DNA oxidative damage), and malondialdehyde (MDA, a lipid oxidation product) in the brains and blood of AD patients are significantly elevated compared to normal individuals (Isobe et al., 2010; Park et al., 2021; Nie et al., 2024). Mitochondria, which are key organelles involved in energy metabolism, facilitate the transfer of electrons through redox reactions to generate ATP. However, various mitochondrial dysfunctions can lead to abnormal electron transfer and reduced reductase activity, resulting in the production of harmful substances such as oxygen free radicals (Song et al., 2021; Bai et al., 2022). Notably, abnormal expression of oxidoreductase systems has also been documented in related studies (Nie et al., 2024). These foundational experiments and clinical investigations indicate that oxidative stress may influence the progression of AD through mechanisms such as mitochondrial dysfunction, alterations in the expression of catalytic enzyme systems, and DNA damage, thereby reinforcing the hypothesis of oxidative stress as a potential mechanism underlying AD.
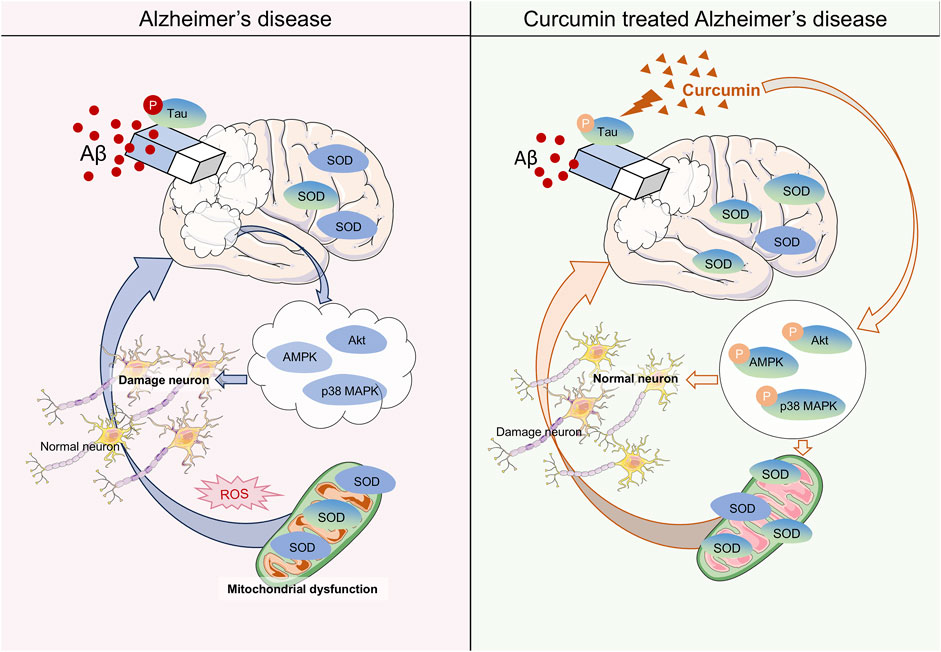
Figure 2. Mechanisms of Curcumin in the Treatment of Alzheimer’s Disease (AD). The mechanism of AD by affecting oxidative stress with Curcumin (CU). Curcumin reduces the AB and the hyperphosphorylation of tau proteins in the brain. In neuronal cells, curcumin increases the activity of the SOD enzyme to reduce mitochondrial dysfunction caused by oxidative stress. Meanwhile, CU improved the phosphorylation process of the AKT/p38 MAPK pathway to reverse neuronal apoptosis caused by oxidative stress.
Numerous studies have reported on the antioxidant properties of CU in the context of Alzheimer’s disease (AD). CU significantly improved cognitive impairment and memory deficits in AD model rats, with superoxide dismutase (SOD) levels in the brains of curcumin-administered rats being significantly higher than those in the AD model, in a dose-dependent manner. This research also validated the synergistic effect of curcumin with coenzyme Q10 in AD, further demonstrating curcumin’s antagonistic effect on oxidative stress (Kumar et al., 2023). Previous studies have shown that CU can reduce Aβ-induced oxidase activity and DNA damage. Furthermore, whether used for prevention, in combination, or as a treatment, CU can mitigate mitochondrial damage and cell apoptosis caused by Aβ-mediated oxidative stress, with this effect being most pronounced when CU is applied prophylactically. Interestingly, this influence in AD is closely related to curcumin’s ability to reverse the dephosphorylation of the AKT/p38 MAPK pathway induced by Aβ, thereby affecting downstream apoptotic proteins (Fan et al., 2017). Another study illustrated that CU restores Aβ-induced mitochondrial dysfunction and synaptotoxicity, further demonstrating that prophylactic application of CU represents the optimal stage for pharmacological intervention (Reddy et al., 2016). Meanwhile, research has shown that CU can reduce Aβ levels in Parkinson’s disease (PD) models and restore the number of damaged neurons, which is linked to increased superoxide dismutase (SOD) enzyme activity, reduced intracellular inflammation, and enhanced AMPK phosphorylation (Shao et al., 2023). However, the interplay of these three mechanisms in the context of PD remains unreported. SIRT1, a NAD+-dependent histone deacetylase, is known for up-regulating antioxidant and anti-inflammatory proteins (Liu et al., 2021). Bisdemethoxycurcumin (BDMC), a CU analog that retains the 1,3 diketone moiety, has been shown to protect neurons from oxidative stress damage by activating SIRT1 expression, suggesting its potential as a therapeutic compound for AD (Xu et al., 2020). Notably, a clinical trial involving 12 weeks of oral CU administration observed a reduction in insulin resistance in type 2 diabetes, which was associated with its effects on Aβ protein accumulation and tau protein hyperphosphorylation (Thota et al., 2020) (Table 2). This finding indirectly supports the therapeutic role of CU in AD. However, clinical trials assessing curcumin’s efficacy in AD have indicated that it does not appear to have a significant positive effect. In a randomized double-blind trial of oral CU over 24 weeks, no significant differences were observed in biomarkers or related psychiatric scores compared to the placebo (Ringman et al., 2012). Additionally, another clinical study reported similar findings (Baum et al., 2008). Importantly, both studies noted issues of low bioavailability, limited sample sizes, and short durations of CU administration. Therefore, further clinical trials are warranted to verify the effectiveness of CU in treating AD.
Parkinson’s disease
Parkinson’s disease (PD), similar to AD, is a prevalent neurodegenerative condition that profoundly affects individuals aged over 60, particularly those over 80 (Cacabelos, 2017; Hou et al., 2019; Alzheimer’s Association Report, 2020). The pathological features of PD include abnormal dopamine function in the substantia nigra of the brain and the presence of Lewy bodies formed by the aggregation of α-synuclein (α-syn) (Atik et al., 2016; Fayyad et al., 2019; Braak et al., 2003; Koeglsperger et al., 2023). The etiology of PD is generally believed to be closely related to environmental and genetic factors, as well as dysfunctions within the nervous system (Samii et al., 2004). Aging is considered one of the most significant contributors to the onset of PD (Collier et al., 2017). In recent years, oxidative stress has emerged as a predisposing factor for various neurological diseases (Imbriani et al., 2022). Dopamine neurons (DAns) in the substantia nigra pars compacta (SNPc) serve as key sites for the synthesis and storage of dopamine (DA) in the body, regulating human cognition and behavioral activity through the nigrostriatal pathway, midbrain limbic cortex system, nodular funnel system, and hypothalamic spinal tract. Notably, DA, as a neurotransmitter containing catecholamines, is not only easily oxidized but also generates free radicals and toxic metabolites, including ROS, dopaquinone (DAQ), and 3,4-dihydroxyphenylacetaldehyde (DOPAL) (Vaarmann et al., 2010; Nagatsu et al., 2022; Jinsmaa et al., 2020). Dopamine (DA) enhances sensitivity to ROS in their presence, exemplifying a typical positive feedback regulation. The adduct formed by the binding of dopamine quinone (DAQ) to sulfhydryl groups in cysteine residues exacerbates protein misfolding and functional loss (Kishida et al., 2021; Boo, 2022). Concurrently, 3,4-dihydroxyphenylacetaldehyde (DOPAL) can induce protein cross-linking and aggregation, further contributing to neurodegeneration (Jinsmaa et al., 2020). Mitochondria function as the cellular “energy suppliers,” producing ATP through oxidative phosphorylation. Under normal circumstances, O2 is reduced to H2O within the mitochondria. However, the electrons involved in mitochondrial shuttling can be easily transferred to the mitochondrial matrix, resulting in the conversion of O2 to superoxide (O2−), a critical source of mitochondrial oxidative stress (Boo, 2022; Drose and Brandt, 2008). The superoxide dismutase (SOD) enzyme in mitochondria converts O2− into hydrogen peroxide (H2O2), which is then further processed by glutathione peroxidase (GPx) and peroxidase (PRx) to generate water (H2O), thereby facilitating the clearance of ROS (Ruszkiewicz and Albrecht, 2015; Averill-Bates, 2023). Unfortunately, mitochondrial dysfunction impedes this redox clearance mechanism, potentially involving mitochondrial electrons and calcium ions (Ca2+) in the formation of ROS, ultimately leading to neuronal dysfunction and apoptosis (Schmidt, 2012; Olson et al., 2005). These conditions may be linked to mitochondrial gene mutations, such as those in the Parkin, PINK1, and DJ-1 genes, which are associated with the development of PD, as well as exposure to toxic substances that can damage mitochondria, including heavy metals and 1-methyl-4-phenyl-1,2,5,6-tetrahydropyridine (MPTP) (Dionisio et al., 2021; Hao et al., 2010; van der Merwe et al., 2015).
Due to its notable antioxidant properties, CU plays a significant positive role in PD (Figure 3). In a rotenone-induced PD model, both CU alone and in combination with levodopa or rasagiline improved motor disorders and behavioral impairments in the model mice by reducing oxidative stress and DNA damage (El-Shamarka et al., 2023). Moreover, CU improved the histopathological changes induced by rotenone, and CU showed additive effects on L-dopa or rasagiline with neuroprotective. Additionally, CU upregulated the expression of Nrf2 in the p62-Keap1-Nrf2 pathway, which is an antioxidant and autophagic transcription factor, thus reversing mitochondrial and oxidative stress damage in the rotenone-induced PD model (Rathore et al., 2023). Furthermore, this research demonstrated that CU could degrade the levels of α-syn by enhancing the autophagic function of nerve cells, suggesting that CU may mitigate oxidative stress-induced PD through multiple pathways. Oxidative stress is closely associated with inflammatory responses. NF-κB serves as a crucial nuclear transcription factor in vivo, and its activated form can translocate to the nucleus to regulate the expression of various genes related to inflammation, apoptosis, and immunity. Studies have indicated that oxidative stress can influence the expression of NF-κB and exacerbate neuronal inflammation in PD (Sivandzade et al., 2019). CU has been shown to reverse the inflammatory response induced by MPP+ in brain astrocytes by inhibiting the expression of pro-inflammatory effectors such as TLR4 and NF-κB, while simultaneously increasing GSH levels to reduce ROS. This suggests that CU may exert therapeutic effects on PD through its anti-inflammatory and antioxidant properties in neuronal cells (Yu et al., 2016). A clinical trial investigating CU supplementation for therapeutic purposes revealed that patients receiving CU experienced a decrease in scores on the COMPASS-31 autonomic and the non-motor symptom (NMSS) questionnaires, as well as a reduction in phosphorylated α-synuclein (p-syn) levels in cutaneous nerves (Donadio et al., 2022). Notably, CU levels were monitored in the blood and cerebrospinal fluid of treated patients, supporting its potential therapeutic effects on central nervous system disorders. Furthermore, the Wnt/β-catenin signaling pathway has been confirmed to be associated with the development of AD (Wang et al., 2022; Ghasemi et al., 2023). Additionally, another study indicated that CU activates the Wnt/β-catenin signaling pathway by inhibiting the expression of GSK-3β, which may be a key protein through which CU influences this pathway in PD (Zhang et al., 2011).
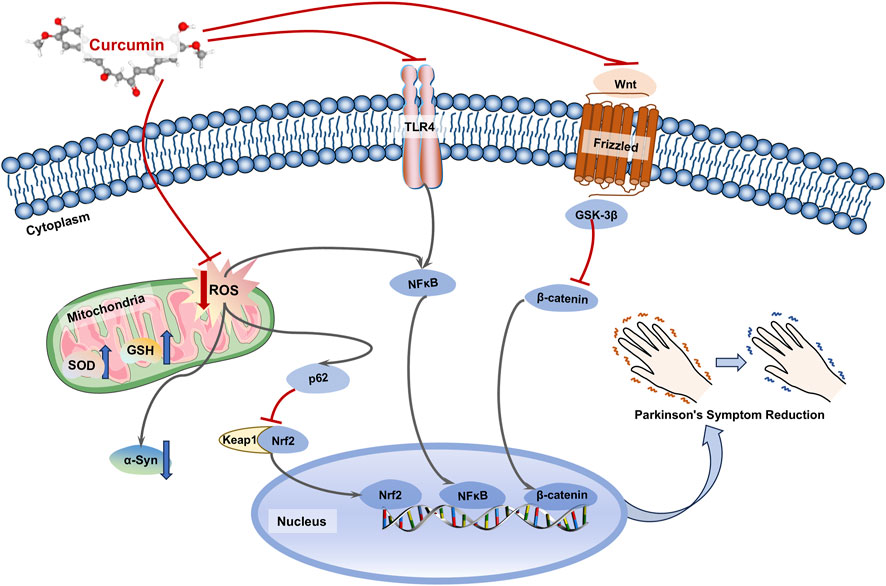
Figure 3. Mechanisms of curcumin in the treatment of Parkinson’s disease (PD). Curcumin (CU) could inhibit mitochondrial damage by oxidative stress in neuronal cells, and promote degradation of α-syn by autophagy through agonism of the Nrf2 transcription factor. Meanwhile, CU could inhibit inflammatory responses and intracellular antioxidant levels by affecting TLP4/NF-κB and Wnt/β-catenin pathways.
Epilepsy
Epilepsy, the most prevalent disorder of the central nervous system (CNS), affects over 50 million individuals globally, posing a significant threat to human health (Thijs et al., 2019). This condition is characterized by recurrent unprovoked seizures, which are generally classified as either generalized or partial. Contemporary research indicates that potential causes of epilepsy include stroke, traumatic brain injury, and neurological infections. Pathologically, epilepsy is characterized by abnormal discharges of nerve cells, neuronal loss, and glial activation (Geronzi et al., 2018). Approximately 26 antiepileptic drugs (AEDs) have been developed that provide effective control of the condition. However, nearly one-third of patients remain resistant to available AEDs and continue to suffer from the disorder (Tang et al., 2017). Furthermore, the narrow therapeutic range of AEDs can result in adverse effects, including affective disorders, cognitive impairment, hepatotoxicity, and recurrent seizures (Baum and Kwan, 2012). Therefore, there is a pressing need for the development of new AEDs that exhibit fewer side effects, enhanced efficacy, and improved safety profiles. Research has demonstrated that oxidative stress plays a role in the pathological processes underlying seizures, exacerbating abnormal neuronal firing and leading to excitotoxicity and oxidative neuronal damage (Tome et al., 2010). Additionally, the brain contains high levels of oxidizable lipids and metals, while possessing limited antioxidant mechanisms, which contributes to its heightened vulnerability to oxidative stress (Bellissimo et al., 2001; Freitas, 2009). Consequently, antioxidants may represent a promising approach to protect neurons from oxidative stress and mitigate epileptogenesis.
Natural antioxidants derived from plants have garnered increasing attention in recent years. CU has been demonstrated to play a beneficial role in epilepsy due to its antioxidant properties, which can alleviate the severity of seizures and reduce oxidative stress in the brain (Jiang et al., 2015; Huang et al., 2020). A randomized, double-blind controlled clinical trial revealed that patients receiving long-term treatment with nanocellular CU experienced a significant reduction in seizure frequency (Erfani et al., 2022). The ability of CU to reduce free radicals facilitates the inhibition of lipoxygenase (LOX), cyclooxygenase (COX), oxidized purinergic alcohols, and nitric oxide synthase (NOS) activities, thereby diminishing the expression of free radicals in various pathophysiological conditions. CU mitigates oxidative stress by forming dimers of dihydrofuran structure with free radicals (Oelkrug et al., 2014). Pentetrazole (PTZ) ignition is widely acknowledged as an animal model for studying epileptogenesis and evaluating antiepileptic drugs, as it lowers the threshold for epileptic seizures by repeatedly stimulating the brain chemically or electrically, ultimately resulting in seizures (Morimoto et al., 2004). Research has shown that pretreatment with CU alleviates seizures, oxidative stress, and cognitive deficits in PTZ-induced ignition in rats (Alam et al., 2023). These findings indicate that CU (300 mg/kg) significantly increased the latency of myoclonic jerks, clonic seizures, and generalized tonic-clonic seizures, improved seizure scores, and reduced the frequency of myoclonic jerks. Furthermore, CU pretreatment reversed the increase in brain malondialdehyde (MDA) levels and the decrease in GSH levels induced by PTZ in a dose-dependent manner (Mehla et al., 2010). Significant reductions in ROS generation, lipid peroxidation, and protein carbonyls were observed in PTZ animals supplemented with CU (Kaur et al., 2014). Similarly, another study demonstrated that CU inhibited the progression of PTZ ignition in mice in a dose-dependent manner by improving the levels of MDA and glutathione (Agarwal et al., 2011). The study reported that CU reduced ROS levels in SH-SY5Y cells (Drion et al., 2018), with the antioxidant effect potentially mediated by the induction of heme oxygenase (Hmox-1), an enzyme that responds to prevent free radical damage and cell death (Gozzelino et al., 2010). CU significantly ameliorated cognitive dysfunction and oxidative damage to the hippocampus and striatum in a persistent epileptic state (SE) model induced by lithium-prusside (Li-Pc). This study also found that pretreatment with CU significantly and dose-dependently attenuated the destruction of antioxidant enzymes in the hippocampus and striatum induced by Li-Pc (Ahmad, 2013). Interestingly, CU has been shown to reduce the expression of TNF-α, IL-10, and TLR4 proteins, as well as MDA levels. This may contribute to its protective effect against febrile convulsions in young mice through its anti-inflammatory and antioxidant properties, along with the downregulation of TLR4 (Atabaki et al., 2020). CU has been shown to ameliorate elevated nitric oxide levels, reduce glutathione levels, and restore catalase activity induced by pilocarpine, while also normalizing Na+, K+-ATPase activity in the hippocampus to control levels (Ezz et al., 2011). This indicates that CU is effective in reducing oxidative stress, and excitability, and inhibiting seizure induction in individuals with epilepsy. Furthermore, dietary intake of CU has been found to inhibit the onset and progression of FeCl3-induced seizures, likely due to curcumin’s significant activation of Na+, K+-ATPase activity and its ability to inhibit lipid and cellular protein damage (Jyoti et al., 2009). Notably, when combined with classical antiepileptic drugs such as valproic acid, phenytoin, phenobarbital, or carbamazepine, CU has been shown to reduce the required doses of these medications without compromising their antiepileptic effects (Reeta et al., 2011). Reeta KH et al. induced epileptic seizures in male rats by electric shock, and injected therapeutic and sub therapeutic doses of phenytoin, phenobarbital, and carbamazepine (AED) before the seizure, or administered CU together with sub-therapeutic doses of AED (Reeta et al., 2011). The results showed that therapeutic doses of AED could completely prevent epileptic seizures. However, sub-therapeutic doses of AED did not completely prevent epileptic seizures. The simultaneous of CU enhanced the protective percentage of AED in sub-therapeutic dose against epileptic seizures and prevented learning and memory impairments caused by seizures, while no such improvement was observed in the group using AED at lower therapeutic doses alone. In addition, the combined of CU would not cause the significant changes in AED serum levels. These findings suggest that CU, as an adjunct to antiepileptic drugs, holds considerable potential in the management of epilepsy, particularly in enhancing efficacy while minimizing dosage and side effects.
Subarachnoid hemorrhage
Subarachnoid hemorrhage (SAH) is a serious neurological condition characterized by high morbidity and mortality rates, making it the third most common subtype of stroke (Feigin et al., 2009; Sercombe et al., 2002). Approximately 85% of SAH cases result from ruptured intracranial aneurysms (van Gijn et al., 2007). Although the clinical manifestations in SAH patients can vary, the most prevalent symptom is the sudden onset of a thunderclap headache, which is notably painful, unexpected, and severe from the onset of the attack (Perry et al., 2013). Additional conditions may include signs of meningeal irritation, transient or prolonged coma, and focal neurological deficits such as cranial nerve palsy and hemiparesis (Claassen and Park, 2022). Despite significant advances in the understanding of SAH, the prognosis for affected patients remains poor and unsatisfactory. The mechanisms contributing to this unfavorable prognosis are complex and multifactorial, encompassing early brain injury (Pluta, 2005), cerebral vasospasm (Pennings et al., 2004), oxidative stress (Gaetani et al., 1998), inflammation (Fassbender et al., 2001), and diffuse cortical depolarization (Dreier et al., 2009). Research indicates that oxidative stress and lipid peroxidation in cerebrospinal fluid and serum increase in humans 3 days post-SAH (Kamezaki et al., 2002). This phenomenon may result from the oxidation of free extracellular hemoglobin to methemoglobin (MetHb) and its subsequent degradation to heme. Concurrently, free heme catalyzes the production of ROS, leading to oxidative stress that mediates proteolysis, lipid peroxidation, and DNA damage, ultimately resulting in cellular damage and death (Wagner et al., 2003). The inflammatory response induced by oxidative stress may play a crucial role in the pathogenesis of cerebral vasospasm (CVS) following subarachnoid hemorrhage (SAH) (Dumont et al., 2003). In patients with SAH, glutamate levels in cerebrospinal fluid (CSF) were significantly elevated, accompanied by a marked reduction in the expression of glutamate transporter protein-1 (EAAT-2, also known as GLT-1) (Wu et al., 2011). The neurotoxic effects of excessive glutamate include the overactivation of both ionotropic and metabotropic glutamate receptors, which leads to a substantial influx of Ca2+ into cells, resulting in apoptosis and necrosis (Lai et al., 2014). Furthermore, oxidative stress contributes to neuronal damage and mediates neuroinflammation, blood-brain barrier (BBB) disruption, and the production of spasminogen following SAH (Ayer and Zhang, 2008). Therefore, therapeutic strategies aimed at mitigating oxidative stress may prove beneficial for patients suffering from SAH.
CU has demonstrated multiple significant neuroprotective effects, particularly in conditions such as ischemic stroke, traumatic brain injury, and intracranial hemorrhage (Dohare et al., 2008; Cole et al., 2007; Thiyagarajan and Sharma, 2004). Notably, several studies indicate that CU may provide a protective effect against cerebral ischemia and cardiovascular spasms following subarachnoid hemorrhage (SAH) through its antioxidative properties (Ghoneim et al., 2002; Wang et al., 2005). In a study involving SAH model rats, oral administration of CU (10 mg/kg) over the course of 1 week resulted in a significant reduction in glutamate and GLT-1 expression, with malondialdehyde (MDA) levels in the hippocampus and cortical regions decreasing by 18% and 29%, respectively. Concurrently, the activities of antioxidant enzymes, including superoxide dismutase (SOD), catalase, glutathione reductase, and lactate dehydrogenase (LDH), were found to increase following CU treatment (Zhang et al., 2016). In another experiment utilizing a double hemorrhage model, SAH rats received an intraperitoneal injection of 20 mg/kg CU for 6 days, leading to improved mortality rates, reduced basal artery wall thickness, decreased neuronal degeneration, and enhanced system scores. These improvements may be associated with the observed reductions in glutamate and MDA levels, alongside increases in SOD and catalase activities within the hippocampus and cortex post-treatment (Kuo et al., 2011). These findings further support the efficacy of multiple CU treatments in counteracting glutamate neurotoxicity and oxidative stress, thereby improving mortality rates. Additionally, another study confirmed that CU significantly inhibited the overexpression of monocyte chemoattractant protein-1 (MCP-1) and tumor necrosis factor-alpha (TNF-α), reduced lipid peroxidation, and restored MDA levels, which collectively ameliorated neurological deficits, alleviated cerebral vasospasm, and significantly decreased mortality in SAH (Cai et al., 2017). Oxyhemoglobin (OxyHb), the primary component of blood, has been reported as a major contributor to cerebral vasospasm and neurological dysfunction in SAH (Luo et al., 2011). The mechanism may involve OxyHb clearing nitric oxide (NO), which leads to vascular spasm (Schwartz et al., 2000). This process activates the Rho/Rho kinase pathway and protein kinase C, resulting in cerebral vasoconstriction (Wickman et al., 2003). Additionally, OxyHb can be oxidized to methemoglobin (MetHb), leading to the production of ROS (Ayer and Zhang, 2008). In a study, cortical neurons were exposed to 10 μM OxyHb for 24 h in the presence of CU. The results indicated that both low and high doses of CU significantly reduced levels of ROS, MDA, tumor necrosis factor-alpha (TNF-α), interleukin-1 beta (IL-1β), interleukin-6 (IL-6), and the Bax/Bcl-2 ratio, while enhancing the activity of SOD and glutathione peroxidase (GSH-Px), thereby increasing cell viability. This suggests that CU attenuates OxyHb-induced oxidative stress and inflammation in neurons, ultimately suppressing apoptosis (Li et al., 2016). Cerebral vasospasm is a major cause of death and disability following subarachnoid hemorrhage (SAH), and it is promoted by oxidative stress (McGirt et al., 2002). A separate study found that CU (150 or 300 mg/kg) prevented cerebral vasospasm and limited secondary cerebral infarction after SAH in mice (Wakade et al., 2009). This effect may be attributed to the significant attenuation of inflammatory gene expression and lipid peroxidation in the cerebral cortex and middle artery.
Glioblastoma
Brain tumors are prevalent malignant neoplasms of the central nervous system, categorized into gliomas (including astrocytomas, ependymomas, and oligodendrogliomas) and non-gliomas (such as meningiomas and medulloblastomas) (Finch et al., 2021). These tumors are classified into four grades based on histological features. Grade I tumors exhibit moderate proliferative capacity and are typically amenable to surgical intervention. Grade II tumors demonstrate a significant propensity for infiltration and recurrence following treatment. Grade III tumors are characterized by larger atypical nuclear fission. In contrast, Grade IV tumors display vascular proliferation and necrosis, rendering them the most aggressive among brain tumors (Komori et al., 2018; Huttner, 2012). Notably, glioblastoma (GBM, classified as WHO Grade IV) comprises approximately 75%–80% of all brain malignancies and is recognized as the most common and invasive primary malignant brain tumor in adults (Agrawal et al., 2023). Numerous studies have indicated that alterations in redox balance are significant factors in the pathogenesis of GBM (Jain, 2018; Rezaei et al., 2022; Korfi et al., 2021). Elevated intracellular levels of oxidants, such as MDA and ROS, can disrupt the equilibrium between oxidants and antioxidants within cells, leading to increased oxidative stress (Janssen et al., 2000). Research has demonstrated that excessive ROS can ultimately result in both single-stranded and double-stranded DNA damage, impairing cell proliferation and intercellular adhesion, which may contribute to genomic instability and cell death (Sehitogullari et al., 2014). Elevated levels of ROS in cancer cells promote cell proliferation and tumor invasion. Currently, a variety of clinical options are available for the treatment of glioblastoma multiforme (GBM), including surgical resection, radiotherapy, and chemotherapy. The standard treatment regimen for patients with GBM involves maximal surgical resection followed by 6 weeks of radiotherapy and adjuvant chemotherapy with temozolomide (TMZ) (Stupp et al., 2009). Significant progress has been made in both diagnostics and therapeutics. Unfortunately, GBM remains largely resistant to treatment and is associated with a poor prognosis, with an average survival of 15 months after diagnosis and less than 10% of patients surviving 5 years post-diagnosis (Ostrom et al., 2015). Phytochemicals exhibit a wide variety of biological activities, target multiple pathways, and possess relatively low toxicity. Consequently, the incorporation of phytochemicals into current GBM therapy research has garnered widespread attention.
CU is a potential therapeutic agent for GBM that regulates various cellular processes, including GBM cell proliferation, apoptosis, cell cycle stagnation, autophagy, and the migratory capabilities of tumor cells (Shahcheraghi et al., 2019). Previous studies have demonstrated that CU can inhibit ROS-induced tumor formation and protect normal tissues from ROS-mediated DNA damage (Wilken et al., 2011; Daverey and Agrawal, 2016). CU has been shown to inhibit the proliferation and migration of glioblastoma cell lines, including U138MG, U87, U373, C6, U251, U87GB, and T98G, in a dose-dependent and time-dependent manner, while also inducing cell apoptosis, as further confirmed in vivo (Wilken et al., 2011; Zanotto-Filho et al., 2012). Glioblastoma stem cells (GSCs) are recognized as a primary contributor to tumor formation, drug resistance, and recurrence; CU has been found to inhibit the viability of GSCs (Gersey et al., 2017). Notably, subtoxic levels of 2.5 μM CU significantly reduce the proliferation, sphere formation ability, and colony formation potential of GSCs. This effect may be attributed to curcumin’s ability to induce ROS, promote activation of the MAPK pathway, and downregulate STAT3 activity and IAP family members (Shehzad and Lee, 2013). Furthermore, as a photosensitive compound, CU possesses a broad absorption peak (300–500 nm) that overlaps with blue light emission. It has also been shown to exhibit photodynamic effects by inducing ROS-mediated apoptosis in the presence of blue light (Leite et al., 2014). Various tumor cells exhibited significant sensitivity to the inhibitory effects of CU photodynamic therapy (PDT) activated by blue LED light (Xie et al., 2022). The photodynamic activation of CU (10 µM) in the presence of blue light resulted in a decrease in matrix metalloproteinases 2 (MMP2) and 9 (MMP9), as well as NF-κB and Nrf2. This cascade led to the activation of ROS-dependent apoptotic pathways in T98G cells, ultimately inducing oxidative stress and cell death (Alkahtani et al., 2023). These findings demonstrate that blue light application enhances the therapeutic efficacy of CU in glioblastoma treatment. Temozolomide (TMZ), a DNA alkylating agent, is commonly used to GBM in clinic. However, therapeutic effect of TMZ is still limited due to the frequent resistance in GBM. Research has found that CU may enhance the therapeutic response of U87MG glioblastoma with TMZ by enhancing cell apoptosis. Moreover, the combination of CU and TMZ has a synergistic effect on the production of reactive oxygen species (ROS) (Yin et al., 2014). However, CU is considered a poor candidate drug due to its low bioavailability, rapid clearance, and metabolism. Most research has focused on developing curcumin analogs and derivatives through chemical synthesis and structural modification to improve therapeutic efficacy, bioavailability, and selectivity. FLDP-5 and FLDP-8, curcumin analogs featuring a piperidone structure, can induce LN-18 cell death in a concentration-dependent manner (IC50: FLDP-5 2.5 µM; FLDP-8 4 μM; CU 31 µM) and inhibit cell migration and invasion (Razali et al., 2022). The involvement of oxidative stress in cell death induced by these analogs was confirmed by a significant increase in intracellular O2− and H2O2 levels, as well as DNA damage observed after 2 and 6 h of exposure. When U87 MG cells were exposed to demethoxycurcumin (DMC) at inhibitory concentrations (0–50 μg/mL), a corresponding increase in ROS production and apoptosis was noted (Kumar et al., 2018). The proposed mechanism suggests that DMC inhibits mitochondrial manganese superoxide dismutase (MnSOD), leading to the production of O2−, which in turn regulates the Akt/NF-κB signaling pathway associated with cell apoptosis. Notably, the therapeutic potential of CU for GBM has been enhanced through modifications in its dosage form (Gallien et al., 2021; Ghoreyshi et al., 2023). For example, a biodegradable PCL and Mpeg-PCL polymer can encapsulate CU, improving its solubility and absorption both in vitro and in vivo (Marslin et al., 2017). Although CU is typically regarded as an antioxidant, it can also promote ROS production in cancer cells, thereby inhibiting cell growth. This indicates that CU functions as a modulator of oxidative stress.
Conclusion
In recent decades, numerous in vivo and in vitro studies have demonstrated that curcumin, a natural antioxidant, holds significant potential in the prevention, treatment, and adjunctive therapy of neurological diseases. This natural compound has been shown to positively impact conditions such as depression, Alzheimer’s disease, Parkinson’s disease, epilepsy, subarachnoid hemorrhage, glioblastoma, and other neurological disorders through the antioxidant stress mechanism. Evidence indicates that curcumin can effectively alleviate the symptoms of these neurological diseases and moderately delay their progression by combating oxidative stress. Furthermore, CU is regarded as a safe compound with no serious side effects, which favorable lipophilicity enhances the ability to penetrate the blood-brain barrier, thereby exerting pharmacological effects. However, this property also presents challenges associated with poor oral absorption and bioavailability. Preclinical and clinical studies have revealed that CU is unstable under physiological conditions and exhibits suboptimal pharmacokinetic properties, which impede the therapeutic application in clinical settings. To address the issues of poor bioavailability and overall efficacy, researchers have sought to develop novel analogs of CU through structural modifications. A variety of advanced nanoformulations with CU have been developed to enhance bioavailability and targeting (Ullah et al., 2024). However, the therapeutic utility of these nanocarriers remains largely in the preclinical exploration phase. Despite the diverse therapeutic applications of CU, clinical research in neurological diseases is still insufficient and requires further high-quality studies to firmly establish its clinical efficacy. We believe that future research should focus on an in-depth exploration of the mechanisms and signaling pathways associated with CU in neurological diseases. Importantly, optimizing the administration methods and discovering new drug delivery systems are essential for improving bioavailability. Furthermore, clinical trials of CU should be widely promoted to validate the efficacy and safety, thereby providing a more practical reference for the application in neurological diseases.
Author contributions
YW: Visualization, Writing–original draft, Writing–review and editing. HL: Conceptualization, Visualization, Writing–review and editing, Writing–original draft. YuL: Funding acquisition, Software, Writing–review and editing. YZ: Investigation, Resources, Software, Writing–review and editing. TQ: Investigation, Resources, Software, Writing–review and editing. YaL: Investigation, Validation, Writing–review and editing. EC: Investigation, Validation, Writing–review and editing. YiB: Investigation, Validation, Writing–review and editing. YuB: Visualization, Writing–original draft, Writing–review and editing. YH: Conceptualization, Project administration, Writing–original draft, Writing–review and editing.
Funding
The author(s) declare that financial support was received for the research, authorship, and/or publication of this article. This study was supported by the Science and Technology Fund of Deyang (Nos 2022SZ043, 2023SZZ115).
Conflict of interest
The authors declare that the research was conducted in the absence of any commercial or financial relationships that could be construed as a potential conflict of interest.
Publisher’s note
All claims expressed in this article are solely those of the authors and do not necessarily represent those of their affiliated organizations, or those of the publisher, the editors and the reviewers. Any product that may be evaluated in this article, or claim that may be made by its manufacturer, is not guaranteed or endorsed by the publisher.
References
Abdolmaleky, H. M., and Zhou, J. R. (2023). Underlying mechanisms of brain aging and neurodegenerative diseases as potential targets for preventive or therapeutic strategies using phytochemicals. Nutrients 15 (15), 3456. doi:10.3390/nu15153456
Agarwal, N. B., Jain, S., Agarwal, N. K., Mediratta, P. K., and Sharma, K. K. (2011). Modulation of pentylenetetrazole-induced kindling and oxidative stress by curcumin in mice. Phytomedicine 18 (8-9), 756–759. doi:10.1016/j.phymed.2010.11.007
Agrawal, K., Asthana, S., and Kumar, D. (2023). Role of oxidative stress in metabolic reprogramming of brain cancer. Cancers (Basel) 15 (20), 4920. doi:10.3390/cancers15204920
Ahmad, M. (2013). Protective effects of curcumin against lithium-pilocarpine induced status epilepticus, cognitive dysfunction and oxidative stress in young rats. Saudi J. Biol. Sci. 20 (2), 155–162. doi:10.1016/j.sjbs.2013.01.002
Alam, M. N., Singh, L., Khan, N. A., Asiri, Y. I., Hassan, M. Z., Afzal, O., et al. (2023). Ameliorative effect of ethanolic extract of moringa oleifera leaves in combination with curcumin against PTZ-induced kindled epilepsy in rats: in vivo and in silico. Pharm. (Basel) 16 (9), 1223. doi:10.3390/ph16091223
Alkahtani, S., Al-Johani N, S., Alarifi, S., and Afzal, M. (2023). Cytotoxicity mechanisms of blue-light-activated curcumin in T98G cell line: inducing apoptosis through ROS-dependent downregulation of MMP pathways. Int. J. Mol. Sci. 24 (4), 3842. doi:10.3390/ijms24043842
Almulla, A. F., Thipakorn, Y., Algon, A. A. A., Tunvirachaisakul, C., Al-Hakeim, H. K., and Maes, M. (2023). Reverse cholesterol transport and lipid peroxidation biomarkers in major depression and bipolar disorder: a systematic review and meta-analysis. Brain Behav. Immun. 113, 374–388. doi:10.1016/j.bbi.2023.08.007
Alzheimer’s Association Report (2020). Alzheimer's disease facts and figures. Alzheimers Dement. doi:10.1002/alz.12068
Andorn, A. C., and Kalaria, R. N. (2000). Factors affecting pro- and anti-oxidant properties of fragments of the b-protein precursor (bPP): implication for Alzheimer's disease. J. Alzheimers Dis. 2 (2), 69–78. doi:10.3233/jad-2000-2201
Asadi, S., Gholami, M. S., Siassi, F., Qorbani, M., and Sotoudeh, G. (2020). Beneficial effects of nano-curcumin supplement on depression and anxiety in diabetic patients with peripheral neuropathy: a randomized, double-blind, placebo-controlled clinical trial. Phytother. Res. 34 (4), 896–903. doi:10.1002/ptr.6571
Atabaki, R., Roohbakhsh, A., Moghimi, A., and Mehri, S. (2020). Protective effects of maternal administration of curcumin and hesperidin in the rat offspring following repeated febrile seizure: role of inflammation and TLR4. Int. Immunopharmacol. 86, 106720. doi:10.1016/j.intimp.2020.106720
Atik, A., Stewart, T., and Zhang, J. (2016). Alpha-synuclein as a biomarker for Parkinson's disease. Brain Pathol. 26 (3), 410–418. doi:10.1111/bpa.12370
Averill-Bates, D. A. (2023). The antioxidant glutathione. Vitam. Horm. 121, 109–141. doi:10.1016/bs.vh.2022.09.002
Ayer, R. E., and Zhang, J. H. (2008). Oxidative stress in subarachnoid haemorrhage: significance in acute brain injury and vasospasm. Acta Neurochir. Suppl. 104, 33–41. doi:10.1007/978-3-211-75718-5_7
Bai, R., Guo, J., Ye, X. Y., Xie, Y., and Xie, T. (2022). Oxidative stress: the core pathogenesis and mechanism of Alzheimer's disease. Ageing Res. Rev. 77, 101619. doi:10.1016/j.arr.2022.101619
Ballance, W. C., Qin, E. C., Chung, H. J., Gillette, M. U., and Kong, H. (2019). Reactive oxygen species-responsive drug delivery systems for the treatment of neurodegenerative diseases. Biomaterials 217, 119292. doi:10.1016/j.biomaterials.2019.119292
Bando, K., Kuroishi, T., Tada, H., Oizumi, T., Tanaka, Y., Takahashi, T., et al. (2021). Nitrogen-containing bisphosphonates and lipopolysaccharide mutually augment inflammation via adenosine triphosphate (ATP)-mediated and interleukin 1β (IL-1β)-mediated production of neutrophil extracellular traps (NETs). J. Bone Min. Res. 36 (9), 1866–1878. doi:10.1002/jbmr.4384
Bansal, Y., and Kuhad, A. (2016). Mitochondrial dysfunction in depression. Curr. Neuropharmacol. 14 (6), 610–618. doi:10.2174/1570159x14666160229114755
Barkin, R. L., and Fawcett, J. (2000). The management challenges of chronic pain: the role of antidepressants. Am. J. Ther. 7 (1), 31–47. doi:10.1097/00045391-200007010-00006
Baum, L., and Kwan, P. (2012). Antiepileptic drug delivery. Adv. Drug Deliv. Rev. 64 (10), 885–886. doi:10.1016/j.addr.2012.04.007
Baum, L., Lam, C. W., Cheung, S. K., Kwok, T., Lui, V., Tsoh, J., et al. (2008). Six-month randomized, placebo-controlled, double-blind, pilot clinical trial of curcumin in patients with Alzheimer disease. J. Clin. Psychopharmacol. 28 (1), 110–113. doi:10.1097/jcp.0b013e318160862c
Bellissimo, M. I., Amado, D., Abdalla, D. S., Ferreira, E. C., and Cavalheiro, E. A. (2001). Naffah-Mazzacoratti MG: superoxide dismutase, glutathione peroxidase activities and the hydroperoxide concentration are modified in the hippocampus of epileptic rats. Epilepsy Res. 46 (2), 121–128. doi:10.1016/s0920-1211(01)00269-8
Bhatt, S., Nagappa, A. N., and Patil, C. R. (2020). Role of oxidative stress in depression. Drug Discov. Today 25 (7), 1270–1276. doi:10.1016/j.drudis.2020.05.001
Birbeck, G. L., Meyer, A. C., and Ogunniyi, A. (2015). Nervous system disorders across the life course in resource-limited settings. Nature 527 (7578), S167–S171. doi:10.1038/nature16031
Birla, H., Minocha, T., Kumar, G., Misra, A., and Singh, S. K. (2020). Role of oxidative stress and metal toxicity in the progression of Alzheimer's disease. Curr. Neuropharmacol. 18 (7), 552–562. doi:10.2174/1570159X18666200122122512
Boo, Y. C. (2022). Metabolic basis and clinical evidence for skin lightening effects of thiol compounds. Antioxidants (Basel) 11 (3), 503. doi:10.3390/antiox11030503
Boumparis, N., Karyotaki, E., Kleiboer, A., Hofmann, S. G., and Cuijpers, P. (2016). The effect of psychotherapeutic interventions on positive and negative affect in depression: a systematic review and meta-analysis. J. Affect Disord. 202, 153–162. doi:10.1016/j.jad.2016.05.019
Braak, H., Del Tredici, K., Rub, U., de Vos, R. A., Jansen Steur, E. N., and Braak, E. (2003). Staging of brain pathology related to sporadic Parkinson's disease. Neurobiol. Aging 24 (2), 197–211. doi:10.1016/s0197-4580(02)00065-9
Brookes, P. S., Yoon, Y., Robotham, J. L., Anders, M. W., and Sheu, S. S. (2004). Calcium, ATP, and ROS: a mitochondrial love-hate triangle. Am. J. Physiol. Cell Physiol. 287 (4), C817–C833. doi:10.1152/ajpcell.00139.2004
Cacabelos, R. (2017). Parkinson's disease: from pathogenesis to pharmacogenomics. Int. J. Mol. Sci. 18 (3), 551. doi:10.3390/ijms18030551
Cai, J., Xu, D., Bai, X., Pan, R., Wang, B., Sun, S., et al. (2017). Curcumin mitigates cerebral vasospasm and early brain injury following subarachnoid hemorrhage via inhibiting cerebral inflammation. Brain Behav. 7 (9), e00790. doi:10.1002/brb3.790
Camkurt, M. A., Findikli, E., Izci, F., Kurutas, E. B., and Tuman, T. C. (2016). Evaluation of malondialdehyde, superoxide dismutase and catalase activity and their diagnostic value in drug naive, first episode, non-smoker major depression patients and healthy controls. Psychiatry Res. 238, 81–85. doi:10.1016/j.psychres.2016.01.075
Claassen, J., and Park, S. (2022). Spontaneous subarachnoid haemorrhage. Lancet 400 (10355), 846–862. doi:10.1016/S0140-6736(22)00938-2
Cole, G. M., Teter, B., and Frautschy, S. A. (2007). Neuroprotective effects of curcumin. Adv. Exp. Med. Biol. 595, 197–212. doi:10.1007/978-0-387-46401-5_8
Collier, T. J., Kanaan, N. M., and Kordower, J. H. (2017). Aging and Parkinson's disease: different sides of the same coin? Mov. Disord. 32 (7), 983–990. doi:10.1002/mds.27037
Dang, R., Wang, M., Li, X., Wang, H., Liu, L., Wu, Q., et al. (2022). Edaravone ameliorates depressive and anxiety-like behaviors via Sirt1/Nrf2/HO-1/Gpx4 pathway. J. Neuroinflammation 19 (1), 41. doi:10.1186/s12974-022-02400-6
Daverey, A., and Agrawal, S. K. (2016). Curcumin alleviates oxidative stress and mitochondrial dysfunction in astrocytes. Neuroscience 333, 92–103. doi:10.1016/j.neuroscience.2016.07.012
Dionisio, P. A., Amaral, J. D., and Rodrigues, C. M. P. (2021). Oxidative stress and regulated cell death in Parkinson's disease. Ageing Res. Rev. 67, 101263. doi:10.1016/j.arr.2021.101263
Dohare, P., Garg, P., Jain, V., Nath, C., and Ray, M. (2008). Dose dependence and therapeutic window for the neuroprotective effects of curcumin in thromboembolic model of rat. Behav. Brain Res. 193 (2), 289–297. doi:10.1016/j.bbr.2008.06.012
Donadio, V., Incensi, A., Rizzo, G., Fileccia, E., Ventruto, F., Riva, A., et al. (2022). The effect of curcumin on idiopathic Parkinson disease: a clinical and skin biopsy study. J. Neuropathol. Exp. Neurol. 81 (7), 545–552. doi:10.1093/jnen/nlac034
Dreier, J. P., Major, S., Manning, A., Woitzik, J., Drenckhahn, C., Steinbrink, J., et al. (2009). Cortical spreading ischaemia is a novel process involved in ischaemic damage in patients with aneurysmal subarachnoid haemorrhage. Brain 132 (Pt 7), 1866–1881. doi:10.1093/brain/awp102
Drion, C. M., van Scheppingen, J., Arena, A., Geijtenbeek, K. W., Kooijman, L., van Vliet, E. A., et al. (2018). Effects of rapamycin and curcumin on inflammation and oxidative stress in vitro and in vivo - in search of potential anti-epileptogenic strategies for temporal lobe epilepsy. J. Neuroinflammation 15 (1), 212. doi:10.1186/s12974-018-1247-9
Drose, S., and Brandt, U. (2008). The mechanism of mitochondrial superoxide production by the cytochrome bc1 complex. J. Biol. Chem. 283 (31), 21649–21654. doi:10.1074/jbc.M803236200
Dumont, A. S., Dumont, R. J., Chow, M. M., Lin, C. L., Calisaneller, T., Ley, K. F., et al. (2003). Cerebral vasospasm after subarachnoid hemorrhage: putative role of inflammation. Neurosurgery 53 (1), 123–133. discussion 133-125. doi:10.1227/01.neu.0000068863.37133.9e
El-Shamarka, M. E., Abdel-Salam, O. M., Shafee, N., and Zeidan, H. M. (2023). Curcumin modulation of L-dopa and rasagiline-induced neuroprotection in rotenone model of Parkinson's disease. Iran. J. Basic Med. Sci. 26 (2), 139–147. doi:10.22038/IJBMS.2022.61687.13650
Erfani, M., Ashrafzadeh, F., Rahimi, H. R., Ebrahimi, S. A., Kalali, K., Beiraghi Toosi, M., et al. (2022). Effect of curcumin on pediatric intractable epilepsy. Iran. J. Child. Neurol. 16 (3), 35–45. doi:10.22037/ijcn.v15i4.28648
Esmaily, H., Sahebkar, A., Iranshahi, M., Ganjali, S., Mohammadi, A., Ferns, G., et al. (2015). An investigation of the effects of curcumin on anxiety and depression in obese individuals: a randomized controlled trial. Chin. J. Integr. Med. 21 (5), 332–338. doi:10.1007/s11655-015-2160-z
Ezz, H. S., Khadrawy, Y. A., and Noor, N. A. (2011). The neuroprotective effect of curcumin and Nigella sativa oil against oxidative stress in the pilocarpine model of epilepsy: a comparison with valproate. Neurochem. Res. 36 (11), 2195–2204. doi:10.1007/s11064-011-0544-9
Fahmy, H. M., Aboalasaad, F. A., Mohamed, A. S., Elhusseiny, F. A., Khadrawy, Y. A., and Elmekawy, A. (2024). Evaluation of the therapeutic effect of curcumin-conjugated zinc oxide nanoparticles on reserpine-induced depression in wistar rats. Biol. Trace Elem. Res. 202 (6), 2630–2644. doi:10.1007/s12011-023-03849-z
Fan, C., Li, Y., Lan, T., Wang, W., Mao, X., and Yu, S. Y. (2021). Prophylactic treatment of curcumin in a rat model of depression by attenuating hippocampal synaptic loss. Food Funct. 12 (22), 11202–11213. doi:10.1039/d1fo02676c
Fan, C. D., Li, Y., Fu, X. T., Wu, Q. J., Hou, Y. J., Yang, M. F., et al. (2017). Reversal of beta-amyloid-induced neurotoxicity in PC12 cells by curcumin, the important role of ROS-mediated signaling and ERK pathway. Cell Mol. Neurobiol. 37 (2), 211–222. doi:10.1007/s10571-016-0362-3
Fang, E. F., Hou, Y., Palikaras, K., Adriaanse, B. A., Kerr, J. S., Yang, B., et al. (2019). Mitophagy inhibits amyloid-β and tau pathology and reverses cognitive deficits in models of Alzheimer's disease. Nat. Neurosci. 22 (3), 401–412. doi:10.1038/s41593-018-0332-9
Farshbaf-Khalili, A., Ostadrahimi, A., Mirghafourvand, M., Ataei-Almanghadim, K., Dousti, S., and Iranshahi, A. M. (2022). Clinical efficacy of curcumin and vitamin E on inflammatory-oxidative stress biomarkers and primary symptoms of menopause in healthy postmenopausal women: a triple-blind randomized controlled trial. J. Nutr. Metab. 2022, 6339715. doi:10.1155/2022/6339715
Fassbender, K., Hodapp, B., Rossol, S., Bertsch, T., Schmeck, J., Schutt, S., et al. (2001). Inflammatory cytokines in subarachnoid haemorrhage: association with abnormal blood flow velocities in basal cerebral arteries. J. Neurol. Neurosurg. Psychiatry 70 (4), 534–537. doi:10.1136/jnnp.70.4.534
Faulkner, M. A. (2014). Safety overview of FDA-approved medications for the treatment of the motor symptoms of Parkinson's disease. Expert Opin. Drug Saf. 13 (8), 1055–1069. doi:10.1517/14740338.2014.931369
Fayyad, M., Salim, S., Majbour, N., Erskine, D., Stoops, E., Mollenhauer, B., et al. (2019). Parkinson's disease biomarkers based on α-synuclein. J. Neurochem. 150 (5), 626–636. doi:10.1111/jnc.14809
Feighner, J. P. (1999). Mechanism of action of antidepressant medications. J. Clin. Psychiatry 60 (Suppl. 4), 4–13. ; discussion 12-13.
Feigin, V. L., Lawes, C. M., Bennett, D. A., Barker-Collo, S. L., and Parag, V. (2009). Worldwide stroke incidence and early case fatality reported in 56 population-based studies: a systematic review. Lancet Neurol. 8 (4), 355–369. doi:10.1016/S1474-4422(09)70025-0
Ferrari, C., and Sorbi, S. (2021). The complexity of Alzheimer's disease: an evolving puzzle. Physiol. Rev. 101 (3), 1047–1081. doi:10.1152/physrev.00015.2020
Fidelis, E. M., Savall, A. S. P., da Luz Abreu, E., Carvalho, F., Teixeira, F. E. G., Haas, S. E., et al. (2019). Curcumin-loaded nanocapsules reverses the depressant-like behavior and oxidative stress induced by β-amyloid in mice. Neuroscience 423, 122–130. doi:10.1016/j.neuroscience.2019.09.032
Finch, A., Solomou, G., Wykes, V., Pohl, U., Bardella, C., and Watts, C. (2021). Advances in research of adult gliomas. Int. J. Mol. Sci. 22 (2), 924. doi:10.3390/ijms22020924
Freitas, R. M. (2009). Investigation of oxidative stress involvement in hippocampus in epilepsy model induced by pilocarpine. Neurosci. Lett. 462 (3), 225–229. doi:10.1016/j.neulet.2009.07.037
Gaetani, P., Pasqualin, A., Rodriguez y Baena, R., Borasio, E., and Marzatico, F. (1998). Oxidative stress in the human brain after subarachnoid hemorrhage. J. Neurosurg. 89 (5), 748–754. doi:10.3171/jns.1998.89.5.0748
Gallien, J., Srinageshwar, B., Gallo, K., Holtgrefe, G., Koneru, S., Otero, P. S., et al. (2021). Curcumin loaded dendrimers specifically reduce viability of glioblastoma cell lines. Molecules 26 (19), 6050. doi:10.3390/molecules26196050
Geronzi, U., Lotti, F., and Grosso, S. (2018). Oxidative stress in epilepsy. Expert Rev. Neurother. 18 (5), 427–434. doi:10.1080/14737175.2018.1465410
Gersey, Z. C., Rodriguez, G. A., Barbarite, E., Sanchez, A., Walters, W. M., Ohaeto, K. C., et al. (2017). Curcumin decreases malignant characteristics of glioblastoma stem cells via induction of reactive oxygen species. BMC Cancer 17 (1), 99. doi:10.1186/s12885-017-3058-2
Ghasemi, A., Qaffaripour, Z., Tourani, M., Saleki, K., Rahmani-Kukia, N., Khatami, S. H., et al. (2023). The relationship between long non-coding RNAs and Wnt/β-catenin signaling pathway in the pathogenesis of Alzheimer's disease. Exp. Neurol. 366, 114434. doi:10.1016/j.expneurol.2023.114434
Ghasemi, M. (2019). Nitric oxide: antidepressant mechanisms and inflammation. Adv. Pharmacol. 86, 121–152. doi:10.1016/bs.apha.2019.04.004
Ghoneim, A. I., Abdel-Naim, A. B., Khalifa, A. E., and El-Denshary, E. S. (2002). Protective effects of curcumin against ischaemia/reperfusion insult in rat forebrain. Pharmacol. Res. 46 (3), 273–279. doi:10.1016/s1043-6618(02)00123-8
Ghoreyshi, N., Ghahremanloo, A., Javid, H., Homayouni Tabrizi, M., and Hashemy, S. I. (2023). Effect of folic acid-linked chitosan-coated PLGA-based curcumin nanoparticles on the redox system of glioblastoma cancer cells. Phytochem. Anal. 34 (8), 950–958. doi:10.1002/pca.3263
Ghosh, M., Parida, S., Khatoon, H., Bera, N., Mishra, S., and Sarkar, N. (2023). Excited state photophysics of curcumin and its modulation in alkaline non-aqueous medium. Chemphyschem 24 (16), e202300174. doi:10.1002/cphc.202300174
Gibson, S. A., Korade, Z., and Shelton, R. C. (2012). Oxidative stress and glutathione response in tissue cultures from persons with major depression. J. Psychiatr. Res. 46 (10), 1326–1332. doi:10.1016/j.jpsychires.2012.06.008
Gozzelino, R., Jeney, V., and Soares, M. P. (2010). Mechanisms of cell protection by heme oxygenase-1. Annu. Rev. Pharmacol. Toxicol. 50, 323–354. doi:10.1146/annurev.pharmtox.010909.105600
Hao, L. Y., Giasson, B. I., and Bonini, N. M. (2010). DJ-1 is critical for mitochondrial function and rescues PINK1 loss of function. Proc. Natl. Acad. Sci. U. S. A. 107 (21), 9747–9752. doi:10.1073/pnas.0911175107
Hoehle, S. I., Pfeiffer, E., and Metzler, M. (2007). Glucuronidation of curcuminoids by human microsomal and recombinant UDP-glucuronosyltransferases. Mol. Nutr. Food Res. 51 (8), 932–938. doi:10.1002/mnfr.200600283
Holder, G. M., Plummer, J. L., and Ryan, A. J. (1978). The metabolism and excretion of curcumin (1,7-bis-(4-hydroxy-3-methoxyphenyl)-1,6-heptadiene-3,5-dione) in the rat. Xenobiotica 8 (12), 761–768. doi:10.3109/00498257809069589
Hou, Y., Dan, X., Babbar, M., Wei, Y., Hasselbalch, S. G., Croteau, D. L., et al. (2019). Ageing as a risk factor for neurodegenerative disease. Nat. Rev. Neurol. 15 (10), 565–581. doi:10.1038/s41582-019-0244-7
Huang, R., Zhu, Y., Lin, L., Song, S., Cheng, L., and Zhu, R. (2020). Solid lipid nanoparticles enhanced the neuroprotective role of curcumin against epilepsy through activation of bcl-2 family and P38 MAPK pathways. ACS Chem. Neurosci. 11 (13), 1985–1995. doi:10.1021/acschemneuro.0c00242
Hulbert, A. J., Pamplona, R., Buffenstein, R., and Buttemer, W. A. (2007). Life and death: metabolic rate, membrane composition, and life span of animals. Physiol. Rev. 87 (4), 1175–1213. doi:10.1152/physrev.00047.2006
Huttner, A. (2012). Overview of primary brain tumors: pathologic classification, epidemiology, molecular biology, and prognostic markers. Hematol. Oncol. Clin. North Am. 26 (4), 715–732. doi:10.1016/j.hoc.2012.05.004
Idunkova, A., Lacinova, L., and Dubiel-Hoppanova, L. (2023). Stress, depression, and hippocampus: from biochemistry to electrophysiology. Gen. Physiol. Biophys. 42 (2), 107–122. doi:10.4149/gpb_2023001
Imbriani, P., Martella, G., Bonsi, P., and Pisani, A. (2022). Oxidative stress and synaptic dysfunction in rodent models of Parkinson's disease. Neurobiol. Dis. 173, 105851. doi:10.1016/j.nbd.2022.105851
Ireson, C., Orr, S., Jones, D. J., Verschoyle, R., Lim, C. K., Luo, J. L., et al. (2001). Characterization of metabolites of the chemopreventive agent curcumin in human and rat hepatocytes and in the rat in vivo, and evaluation of their ability to inhibit phorbol ester-induced prostaglandin E2 production. Cancer Res. 61 (3), 1058–1064.
Ireson, C. R., Jones, D. J., Orr, S., Coughtrie, M. W., Boocock, D. J., Williams, M. L., et al. (2002). Metabolism of the cancer chemopreventive agent curcumin in human and rat intestine. Cancer Epidemiol. Biomarkers Prev. 11 (1), 105–111.
Irving, G. R., Howells, L. M., Sale, S., Kralj-Hans, I., Atkin, W. S., Clark, S. K., et al. (2013). Prolonged biologically active colonic tissue levels of curcumin achieved after oral administration--a clinical pilot study including assessment of patient acceptability. Cancer Prev. Res. (Phila) 6 (2), 119–128. doi:10.1158/1940-6207.CAPR-12-0281
Isobe, C., Abe, T., and Terayama, Y. (2010). Levels of reduced and oxidized coenzyme Q-10 and 8-hydroxy-2'-deoxyguanosine in the CSF of patients with Alzheimer's disease demonstrate that mitochondrial oxidative damage and/or oxidative DNA damage contributes to the neurodegenerative process. J. Neurol. 257 (3), 399–404. doi:10.1007/s00415-009-5333-x
Jain, K. K. (2018). A critical overview of targeted therapies for glioblastoma. Front. Oncol. 8, 419. doi:10.3389/fonc.2018.00419
Janssen, A. M., Bosman, C. B., van Duijn, W., Oostendorp-van de Ruit, M. M., Kubben, F. J., Griffioen, G., et al. (2000). Superoxide dismutases in gastric and esophageal cancer and the prognostic impact in gastric cancer. Clin. Cancer Res. 6 (8), 3183–3192.
Jiang, Z., Guo, M., Shi, C., Wang, H., Yao, L., Liu, L., et al. (2015). Protection against cognitive impairment and modification of epileptogenesis with curcumin in a post-status epilepticus model of temporal lobe epilepsy. Neuroscience 310, 362–371. doi:10.1016/j.neuroscience.2015.09.058
Jinsmaa, Y., Isonaka, R., Sharabi, Y., and Goldstein, D. S. (2020). 3,4-Dihydroxyphenylacetaldehyde is more efficient than dopamine in oligomerizing and quinonizing α-synuclein. J. Pharmacol. Exp. Ther. 372 (2), 157–165. doi:10.1124/jpet.119.262246
Jones, D. P. (2024). Redox organization of living systems. Free Radic. Biol. Med. 217, 179–189. doi:10.1016/j.freeradbiomed.2024.03.008
Jyoti, A., Sethi, P., and Sharma, D. (2009). Curcumin protects against electrobehavioral progression of seizures in the iron-induced experimental model of epileptogenesis. Epilepsy Behav. 14 (2), 300–308. doi:10.1016/j.yebeh.2008.11.011
Kamezaki, T., Yanaka, K., Nagase, S., Fujita, K., Kato, N., and Nose, T. (2002). Increased levels of lipid peroxides as predictive of symptomatic vasospasm and poor outcome after aneurysmal subarachnoid hemorrhage. J. Neurosurg. 97 (6), 1302–1305. doi:10.3171/jns.2002.97.6.1302
Kaur, H., Bal, A., and Sandhir, R. (2014). Curcumin supplementation improves mitochondrial and behavioral deficits in experimental model of chronic epilepsy. Pharmacol. Biochem. Behav. 125, 55–64. doi:10.1016/j.pbb.2014.08.001
Khanam, S., and Siddique, Y. H. (2018). Dopamine: agonists and neurodegenerative disorders. Curr. Drug Targets 19 (14), 1599–1611. doi:10.2174/1389450118666171117124340
Kishida, R., Ito, S., Sugumaran, M., Arevalo, R. L., Nakanishi, H., and Kasai, H. (2021). Density functional theory-based calculation shed new light on the bizarre addition of cysteine thiol to dopaquinone. Int. J. Mol. Sci. 22 (3), 1373. doi:10.3390/ijms22031373
Kocaadam, B., and Sanlier, N. (2017). Curcumin, an active component of turmeric (Curcuma longa), and its effects on health. Crit. Rev. Food Sci. Nutr. 57 (13), 2889–2895. doi:10.1080/10408398.2015.1077195
Koeglsperger, T., Rumpf, S. L., Schliesser, P., Struebing, F. L., Brendel, M., Levin, J., et al. (2023). Neuropathology of incidental Lewy body and prodromal Parkinson's disease. Mol. Neurodegener. 18 (1), 32. doi:10.1186/s13024-023-00622-7
Komori, T., Muragaki, Y., and Chernov, M. F. (2018). Pathology and genetics of gliomas. Prog. Neurol. Surg. 31, 1–37. doi:10.1159/000466835
Korfi, F., Javid, H., Assaran Darban, R., and Hashemy, S. I. (2021). The effect of SP/NK1R on the expression and activity of catalase and superoxide dismutase in glioblastoma cancer cells. Biochem. Res. Int. 2021, 6620708. doi:10.1155/2021/6620708
Kumar, P., Singh, A., Kumar, A., Kumar, R., Pal, R., Sachan, A. K., et al. (2023). Effect of curcumin and coenzyme Q10 alone and in combination on learning and memory in an animal model of Alzheimer's disease. Biomedicines 11 (5), 1422. doi:10.3390/biomedicines11051422
Kumar, R., Lal, N., Nemaysh, V., and Luthra, P. M. (2018). Demethoxycurcumin mediated targeting of MnSOD leading to activation of apoptotic pathway and inhibition of Akt/NF-κB survival signalling in human glioma U87 MG cells. Toxicol. Appl. Pharmacol. 345, 75–93. doi:10.1016/j.taap.2018.02.020
Kuo, C. P., Lu, C. H., Wen, L. L., Cherng, C. H., Wong, C. S., Borel, C. O., et al. (2011). Neuroprotective effect of curcumin in an experimental rat model of subarachnoid hemorrhage. Anesthesiology 115 (6), 1229–1238. doi:10.1097/ALN.0b013e31823306f0
Kwon, D. K., Kwatra, M., Wang, J., and Ko, H. S. (2022). Levodopa-induced dyskinesia in Parkinson's disease: pathogenesis and emerging treatment strategies. Cells 11 (23), 3736. doi:10.3390/cells11233736
Lai, T. W., Zhang, S., and Wang, Y. T. (2014). Excitotoxicity and stroke: identifying novel targets for neuroprotection. Prog. Neurobiol. 115, 157–188. doi:10.1016/j.pneurobio.2013.11.006
Lee, J. H., Oh, J. H., and Lee, Y. J. (2012). Biliary excretion of curcumin is mediated by multidrug resistance-associated protein 2. Biol. Pharm. Bull. 35 (5), 777–780. doi:10.1248/bpb.35.777
Leite, D. P., Paolillo, F. R., Parmesano, T. N., Fontana, C. R., and Bagnato, V. S. (2014). Effects of photodynamic therapy with blue light and curcumin as mouth rinse for oral disinfection: a randomized controlled trial. Photomed. Laser Surg. 32 (11), 627–632. doi:10.1089/pho.2014.3805
Leite-Aguiar, R., Bello-Santos, V. G., Castro, N. G., Coutinho-Silva, R., and Savio, L. E. B. (2024). Techniques for evaluating the ATP-gated ion channel P2X7 receptor function in macrophages and microglial cells. J. Immunol. Methods 532, 113727. doi:10.1016/j.jim.2024.113727
Li, X., Zhao, L., Yue, L., Liu, H., Yang, X., Wang, X., et al. (2016). Evidence for the protective effects of curcumin against oxyhemoglobin-induced injury in rat cortical neurons. Brain Res. Bull. 120, 34–40. doi:10.1016/j.brainresbull.2015.11.006
Liao, D., Lv, C., Cao, L., Yao, D., Wu, Y., Long, M., et al. (2020). Curcumin attenuates chronic unpredictable mild stress-induced depressive-like behaviors via restoring changes in oxidative stress and the activation of Nrf2 signaling pathway in rats. Oxid. Med. Cell Longev. 2020, 9268083. doi:10.1155/2020/9268083
Liu, L., Xia, G., Li, P., Wang, Y., and Zhao, Q. (2021). Sirt-1 regulates physiological process and exerts protective effects against oxidative stress. Biomed. Res. Int. 2021, 5542545. doi:10.1155/2021/5542545
Liu, T., Sun, L., Zhang, Y., Wang, Y., and Zheng, J. (2022). Imbalanced GSH/ROS and sequential cell death. J. Biochem. Mol. Toxicol. 36 (1), e22942. doi:10.1002/jbt.22942
LoPachin, R. M., Gavin, T., Geohagen, B. C., Zhang, L., Casper, D., Lekhraj, R., et al. (2011). β-dicarbonyl enolates: a new class of neuroprotectants. J. Neurochem. 116 (1), 132–143. doi:10.1111/j.1471-4159.2010.07091.x
Lopresti, A. L., and Drummond, P. D. (2017). Efficacy of curcumin, and a saffron/curcumin combination for the treatment of major depression: a randomised, double-blind, placebo-controlled study. J. Affect Disord. 207, 188–196. doi:10.1016/j.jad.2016.09.047
Luo, C., Yi, B., Chen, Z., Tang, W., Chen, Y., Hu, R., et al. (2011). PKGIα inhibits the proliferation of cerebral arterial smooth muscle cell induced by oxyhemoglobin after subarachnoid hemorrhage. Acta Neurochir. Suppl. 110 (Pt 1), 167–171. doi:10.1007/978-3-7091-0353-1_29
Maes, M., Galecki, P., Chang, Y. S., and Berk, M. (2011). A review on the oxidative and nitrosative stress (O&NS) pathways in major depression and their possible contribution to the (neuro)degenerative processes in that illness. Prog. Neuropsychopharmacol. Biol. Psychiatry 35 (3), 676–692. doi:10.1016/j.pnpbp.2010.05.004
Maiese, K. (2023). The impact of aging and oxidative stress in metabolic and nervous system disorders: programmed cell death and molecular signal transduction crosstalk. Front. Immunol. 14, 1273570. doi:10.3389/fimmu.2023.1273570
Maina, M. B., Al-Hilaly, Y. K., Burra, G., Rickard, J. E., Harrington, C. R., Wischik, C. M., et al. (2021). Oxidative stress conditions result in trapping of PHF-core tau (297-391) intermediates. Cells 10 (3), 703. doi:10.3390/cells10030703
Marslin, G., Carmelino Cardoso Sarmento, B. F., Franklin, G., Ribeiro Martins, J. A., Ribeiro Silva, C. J., Castro Gomes, A. F., et al. (2017). Curcumin encapsulated into methoxy poly(ethylene glycol) poly(ε-caprolactone) nanoparticles increases cellular uptake and neuroprotective effect in glioma cells. Planta Medica 83 (5), 434–444. doi:10.1055/s-0042-112030
Martino, D. J., Valerio, M. P., Lomastro, J., and Igoa, A. (2022). Clinical course in patients with melancholic and nonmelancholic bipolar depression. J. Nerv. Ment. Dis. 210 (11), 862–868. doi:10.1097/NMD.0000000000001553
McCarron, R. M., Shapiro, B., Rawles, J., and Luo, J. (2021). Depression. Ann. Intern Med. 174 (5), ITC65–ITC80. doi:10.7326/AITC202105180
McGirt, M. J., Parra, A., Sheng, H., Higuchi, Y., Oury, T. D., Laskowitz, D. T., et al. (2002). Attenuation of cerebral vasospasm after subarachnoid hemorrhage in mice overexpressing extracellular superoxide dismutase. Stroke 33 (9), 2317–2323. doi:10.1161/01.str.0000027207.67639.1e
Mehla, J., Reeta, K. H., Gupta, P., and Gupta, Y. K. (2010). Protective effect of curcumin against seizures and cognitive impairment in a pentylenetetrazole-kindled epileptic rat model. Life Sci. 87 (19-22), 596–603. doi:10.1016/j.lfs.2010.09.006
Menard, C., Hodes, G. E., and Russo, S. J. (2016). Pathogenesis of depression: insights from human and rodent studies. Neuroscience 321, 138–162. doi:10.1016/j.neuroscience.2015.05.053
Monteiro, A. R., Barbosa, D. J., Remiao, F., and Silva, R. (2023). Alzheimer's disease: insights and new prospects in disease pathophysiology, biomarkers and disease-modifying drugs. Biochem. Pharmacol. 211, 115522. doi:10.1016/j.bcp.2023.115522
Moradi, F., Dashti, N., Farahvash, A., Baghaei Naeini, F., and Zarebavani, M. (2023). Curcumin ameliorates chronic Toxoplasma gondii infection-induced affective disorders through modulation of proinflammatory cytokines and oxidative stress. Iran. J. Basic Med. Sci. 26 (4), 461–467. doi:10.22038/IJBMS.2023.68487.14937
Morimoto, K., Fahnestock, M., and Racine, R. J. (2004). Kindling and status epilepticus models of epilepsy: rewiring the brain. Prog. Neurobiol. 73 (1), 1–60. doi:10.1016/j.pneurobio.2004.03.009
Nagatsu, T., Nakashima, A., Watanabe, H., Ito, S., and Wakamatsu, K. (2022). Neuromelanin in Parkinson's disease: tyrosine hydroxylase and tyrosinase. Int. J. Mol. Sci. 23 (8), 4176. doi:10.3390/ijms23084176
Nelson, K. M., Dahlin, J. L., Bisson, J., Graham, J., Pauli, G. F., and Walters, M. A. (2017). The essential medicinal chemistry of curcumin. J. Med. Chem. 60 (5), 1620–1637. doi:10.1021/acs.jmedchem.6b00975
Nie, Y., Chu, C., Qin, Q., Shen, H., Wen, L., Tang, Y., et al. (2024). Lipid metabolism and oxidative stress in patients with Alzheimer's disease and amnestic mild cognitive impairment. Brain Pathol. 34 (1), e13202. doi:10.1111/bpa.13202
Nimgampalle, M., Chakravarthy, H., Sharma, S., Shree, S., Bhat, A. R., Pradeepkiran, J. A., et al. (2023). Neurotransmitter systems in the etiology of major neurological disorders: emerging insights and therapeutic implications. Ageing Res. Rev. 89, 101994. doi:10.1016/j.arr.2023.101994
Oelkrug, C., Lange, C. M., Wenzel, E., Fricke, S., Hartke, M., Simasi, J., et al. (2014). Analysis of the tumoricidal and anti-cachectic potential of curcumin. Anticancer Res. 34 (9), 4781–4788.
Olson, P. A., Tkatch, T., Hernandez-Lopez, S., Ulrich, S., Ilijic, E., Mugnaini, E., et al. (2005). G-protein-coupled receptor modulation of striatal CaV1.3 L-type Ca2+ channels is dependent on a Shank-binding domain. J. Neurosci. 25 (5), 1050–1062. doi:10.1523/JNEUROSCI.3327-04.2005
Omari Shekaftik, S., and Nasirzadeh, N. (2021). 8-Hydroxy-2'-deoxyguanosine (8-OHdG) as a biomarker of oxidative DNA damage induced by occupational exposure to nanomaterials: a systematic review. Nanotoxicology 15 (6), 850–864. doi:10.1080/17435390.2021.1936254
Ostrom, Q. T., Gittleman, H., Fulop, J., Liu, M., Blanda, R., Kromer, C., et al. (2015). CBTRUS statistical Report: primary brain and central nervous system tumors diagnosed in the United States in 2008-2012. Neuro Oncol. 17 (Suppl. 4), iv1–iv62. doi:10.1093/neuonc/nov189
Pan, K., Luo, Y., Gan, Y., Baek, S. J., and Zhong, Q. (2014). pH-driven encapsulation of curcumin in self-assembled casein nanoparticles for enhanced dispersibility and bioactivity. Soft Matter 10 (35), 6820–6830. doi:10.1039/c4sm00239c
Pan, M. H., Huang, T. M., and Lin, J. K. (1999). Biotransformation of curcumin through reduction and glucuronidation in mice. Drug Metab. Dispos. 27 (4), 486–494.
Park, M. W., Cha, H. W., Kim, J., Kim, J. H., Yang, H., Yoon, S., et al. (2021). NOX4 promotes ferroptosis of astrocytes by oxidative stress-induced lipid peroxidation via the impairment of mitochondrial metabolism in Alzheimer's diseases. Redox Biol. 41, 101947. doi:10.1016/j.redox.2021.101947
Payton, F., Sandusky, P., and Alworth, W. L. (2007). NMR study of the solution structure of curcumin. J. Nat. Prod. 70 (2), 143–146. doi:10.1021/np060263s
Penner-Goeke, S., and Binder, E. B. (2019). Epigenetics and depression. Clin. Neurosci. 21 (4), 397–405. doi:10.31887/DCNS.2019.21.4/ebinder
Pennings, F. A., Bouma, G. J., and Ince, C. (2004). Direct observation of the human cerebral microcirculation during aneurysm surgery reveals increased arteriolar contractility. Stroke 35 (6), 1284–1288. doi:10.1161/01.STR.0000126039.91400.cb
Perkins, S., Verschoyle, R. D., Hill, K., Parveen, I., Threadgill, M. D., Sharma, R. A., et al. (2002). Chemopreventive efficacy and pharmacokinetics of curcumin in the min/+ mouse, a model of familial adenomatous polyposis. Cancer Epidemiol. Biomarkers Prev. 11 (6), 535–540.
Perry, J. J., Stiell, I. G., Sivilotti, M. L., Bullard, M. J., Hohl, C. M., Sutherland, J., et al. (2013). Clinical decision rules to rule out subarachnoid hemorrhage for acute headache. JAMA 310 (12), 1248–1255. doi:10.1001/jama.2013.278018
Piper, J. A., Al, H. N., Jansen, M. I., Rodgers, K. J., Musumeci, G., Dhungana, A., et al. (2023). L-proline prevents endoplasmic reticulum stress in microglial cells exposed to L-azetidine-2-carboxylic acid. Molecules 28 (12), 4808. doi:10.3390/molecules28124808
Pluta, R. M. (2005). Delayed cerebral vasospasm and nitric oxide: review, new hypothesis, and proposed treatment. Pharmacol. Ther. 105 (1), 23–56. doi:10.1016/j.pharmthera.2004.10.002
Pratico, D. (2008). Oxidative stress hypothesis in Alzheimer's disease: a reappraisal. Trends Pharmacol. Sci. 29 (12), 609–615. doi:10.1016/j.tips.2008.09.001
Pratico, D., Uryu, K., Sung, S., Tang, S., Trojanowski, J. Q., and Lee, V. M. (2002). Aluminum modulates brain amyloidosis through oxidative stress in APP transgenic mice. FASEB J. 16 (9), 1138–1140. doi:10.1096/fj.02-0012fje
Priyadarsini, K. I. (2014). The chemistry of curcumin: from extraction to therapeutic agent. Molecules 19 (12), 20091–20112. doi:10.3390/molecules191220091
Rathore, A. S., Singh, S. S., Birla, H., Zahra, W., Keshri, P. K., Dilnashin, H., et al. (2023). Curcumin modulates p62-keap1-nrf2-mediated autophagy in rotenone-induced Parkinson's disease mouse models. ACS Chem. Neurosci. doi:10.1021/acschemneuro.2c00706
Ravindranath, V., and Chandrasekhara, N. (1980). Absorption and tissue distribution of curcumin in rats. Toxicology 16 (3), 259–265. doi:10.1016/0300-483x(80)90122-5
Razali, N. S. C., Lam, K. W., Rajab, N. F., Jamal, A. R. A., Kamaluddin, N. F., and Chan, K. M. (2022). Curcumin piperidone derivatives induce anti-proliferative and anti-migratory effects in LN-18 human glioblastoma cells. Sci. Rep. 12 (1), 13131. doi:10.1038/s41598-022-16274-4
Reddy, P. H., Manczak, M., Yin, X., Grady, M. C., Mitchell, A., Kandimalla, R., et al. (2016). Protective effects of a natural product, curcumin, against amyloid β induced mitochondrial and synaptic toxicities in Alzheimer's disease. J. Investig. Med. 64 (8), 1220–1234. doi:10.1136/jim-2016-000240
Reeta, K. H., Mehla, J., Pahuja, M., and Gupta, Y. K. (2011). Pharmacokinetic and pharmacodynamic interactions of valproate, phenytoin, phenobarbitone and carbamazepine with curcumin in experimental models of epilepsy in rats. Pharmacol. Biochem. Behav. 99 (3), 399–407. doi:10.1016/j.pbb.2011.05.011
Rezaei, S., Assaran Darban, R., Javid, H., and Hashemy, S. I. (2022). The therapeutic potential of aprepitant in glioblastoma cancer cells through redox modification. Biomed. Res. Int. 2022, 8540403. doi:10.1155/2022/8540403
Ringman, J. M., Frautschy, S. A., Teng, E., Begum, A. N., Bardens, J., Beigi, M., et al. (2012). Oral curcumin for Alzheimer's disease: tolerability and efficacy in a 24-week randomized, double blind, placebo-controlled study. Alzheimers Res. Ther. 4 (5), 43. doi:10.1186/alzrt146
Rostagno, A. A. (2022). Pathogenesis of Alzheimer's disease. Int. J. Mol. Sci. 24 (1), 107. doi:10.3390/ijms24010107
Ruszkiewicz, J., and Albrecht, J. (2015). Changes in the mitochondrial antioxidant systems in neurodegenerative diseases and acute brain disorders. Neurochem. Int. 88, 66–72. doi:10.1016/j.neuint.2014.12.012
Salim, S. (2017). Oxidative stress and the central nervous system. J. Pharmacol. Exp. Ther. 360 (1), 201–205. doi:10.1124/jpet.116.237503
Samarghandian, S., Azimi-Nezhad, M., Farkhondeh, T., and Samini, F. (2017). Anti-oxidative effects of curcumin on immobilization-induced oxidative stress in rat brain, liver and kidney. Biomed. Pharmacother. 87, 223–229. doi:10.1016/j.biopha.2016.12.105
Samii, A., Nutt, J. G., and Ransom, B. R. (2004). Parkinson's disease. Lancet 363 (9423), 1783–1793. doi:10.1016/S0140-6736(04)16305-8
Sanmukhani, J., Satodia, V., Trivedi, J., Patel, T., Tiwari, D., Panchal, B., et al. (2014). Efficacy and safety of curcumin in major depressive disorder: a randomized controlled trial. Phytother. Res. 28 (4), 579–585. doi:10.1002/ptr.5025
Sarawi, W. S., Alhusaini, A. M., Fadda, L. M., Alomar, H. A., Albaker, A. B., Aljrboa, A. S., et al. (2021). Curcumin and nano-curcumin mitigate copper neurotoxicity by modulating oxidative stress, inflammation, and akt/GSK-3β signaling. Molecules 26 (18), 5591. doi:10.3390/molecules26185591
Scheltens, P., De Strooper, B., Kivipelto, M., Holstege, H., Chetelat, G., Teunissen, C. E., et al. (2021). Alzheimer's disease. Lancet 397 (10284), 1577–1590. doi:10.1016/S0140-6736(20)32205-4
Schmidt, H. (2012). Three functional facets of calbindin D-28k. Front. Mol. Neurosci. 5, 25. doi:10.3389/fnmol.2012.00025
Schwartz, A. Y., Sehba, F. A., and Bederson, J. B. (2000). Decreased nitric oxide availability contributes to acute cerebral ischemia after subarachnoid hemorrhage. Neurosurgery 47 (1), 208–214. discussion 214-205. doi:10.1097/00006123-200007000-00042
Sehitogullari, A., Aslan, M., Sayir, F., Kahraman, A., and Demir, H. (2014). Serum paraoxonase-1 enzyme activities and oxidative stress levels in patients with esophageal squamous cell carcinoma. Redox Rep. 19 (5), 199–205. doi:10.1179/1351000214Y.0000000091
Sercombe, R., Dinh, Y. R., and Gomis, P. (2002). Cerebrovascular inflammation following subarachnoid hemorrhage. Jpn. J. Pharmacol. 88 (3), 227–249. doi:10.1254/jjp.88.227
Shahcheraghi, S. H., Zangui, M., Lotfi, M., Ghayour-Mobarhan, M., Ghorbani, A., Jaliani, H. Z., et al. (2019). Therapeutic potential of curcumin in the treatment of glioblastoma multiforme. Curr. Pharm. Des. 25 (3), 333–342. doi:10.2174/1381612825666190313123704
Shao, S., Ye, X., Su, W., and Wang, Y. (2023). Curcumin alleviates Alzheimer's disease by inhibiting inflammatory response, oxidative stress and activating the AMPK pathway. J. Chem. Neuroanat. 134, 102363. doi:10.1016/j.jchemneu.2023.102363
Sharma, R. A., McLelland, H. R., Hill, K. A., Ireson, C. R., Euden, S. A., Manson, M. M., et al. (2001). Pharmacodynamic and pharmacokinetic study of oral Curcuma extract in patients with colorectal cancer. Clin. Cancer Res. 7 (7), 1894–1900.
Shehzad, A., and Lee, Y. S. (2013). Molecular mechanisms of curcumin action: signal transduction. Biofactors 39 (1), 27–36. doi:10.1002/biof.1065
Sies, H. (2021). Oxidative eustress: on constant alert for redox homeostasis. Redox Biol. 41, 101867. doi:10.1016/j.redox.2021.101867
Sivandzade, F., Prasad, S., Bhalerao, A., and Cucullo, L. (2019). NRF2 and NF-қB interplay in cerebrovascular and neurodegenerative disorders: molecular mechanisms and possible therapeutic approaches. Redox Biol. 21, 101059. doi:10.1016/j.redox.2018.11.017
Smith, D. G., Cappai, R., and Barnham, K. J. (2007). The redox chemistry of the Alzheimer's disease amyloid beta peptide. Biochim. Biophys. Acta 1768 (8), 1976–1990. doi:10.1016/j.bbamem.2007.02.002
Song, T., Song, X., Zhu, C., Patrick, R., Skurla, M., Santangelo, I., et al. (2021). Mitochondrial dysfunction, oxidative stress, neuroinflammation, and metabolic alterations in the progression of Alzheimer's disease: a meta-analysis of in vivo magnetic resonance spectroscopy studies. Ageing Res. Rev. 72, 101503. doi:10.1016/j.arr.2021.101503
Strzyz, P. (2022). ATP and ROS signal cell extrusion. Nat. Rev. Mol. Cell Biol. 23 (6), 387. doi:10.1038/s41580-022-00487-6
Stupp, R., Hegi, M. E., Mason, W. P., van den Bent, M. J., Taphoorn, M. J., Janzer, R. C., et al. (2009). Effects of radiotherapy with concomitant and adjuvant temozolomide versus radiotherapy alone on survival in glioblastoma in a randomised phase III study: 5-year analysis of the EORTC-NCIC trial. Lancet Oncol. 10 (5), 459–466. doi:10.1016/S1470-2045(09)70025-7
Su, B., Wang, X., Lee, H. G., Tabaton, M., Perry, G., Smith, M. A., et al. (2010). Chronic oxidative stress causes increased tau phosphorylation in M17 neuroblastoma cells. Neurosci. Lett. 468 (3), 267–271. doi:10.1016/j.neulet.2009.11.010
Syafrita, Y., Amir, D., Susanti, R., and Fadhilah, I. (2020). Relationship of brain-derived neurotrophic factor, malondialdehyde, and 8-Hydroxy 2-Deoxyguanosine with post-ischemic stroke depression. Dement. Neuropsychol. 14 (1), 41–46. doi:10.1590/1980-57642020dn14-010007
Tang, F., Hartz, A. M. S., and Bauer, B. (2017). Drug-resistant epilepsy: multiple hypotheses, few answers. Front. Neurol. 8, 301. doi:10.3389/fneur.2017.00301
Tang, L., Liu, S., Li, S., Chen, Y., Xie, B., and Zhou, J. (2023). Induction mechanism of ferroptosis, necroptosis, and pyroptosis: a novel therapeutic target in nervous system diseases. Int. J. Mol. Sci. 24 (12), 10127. doi:10.3390/ijms241210127
Thijs, R. D., Surges, R., O'Brien, T. J., and Sander, J. W. (2019). Epilepsy in adults. Lancet 393 (10172), 689–701. doi:10.1016/S0140-6736(18)32596-0
Thiyagarajan, M., and Sharma, S. S. (2004). Neuroprotective effect of curcumin in middle cerebral artery occlusion induced focal cerebral ischemia in rats. Life Sci. 74 (8), 969–985. doi:10.1016/j.lfs.2003.06.042
Thota, R. N., Rosato, J. I., Dias, C. B., Burrows, T. L., Martins, R. N., and Garg, M. L. (2020). Dietary supplementation with curcumin reduce circulating levels of glycogen synthase kinase-3β and islet amyloid polypeptide in adults with high risk of type 2 diabetes and Alzheimer's disease. Nutrients 12 (4), 1032. doi:10.3390/nu12041032
Tome, A. R., Feng, D., and Freitas, R. M. (2010). The effects of alpha-tocopherol on hippocampal oxidative stress prior to in pilocarpine-induced seizures. Neurochem. Res. 35 (4), 580–587. doi:10.1007/s11064-009-0102-x
Tonnies, E., and Trushina, E. (2017). Oxidative stress, synaptic dysfunction, and Alzheimer's disease. J. Alzheimers Dis. 57 (4), 1105–1121. doi:10.3233/JAD-161088
Ullah, N., Hasnain, S. Z. U., Baloch, R., Amin, A., Nasibova, A., Selakovic, D., et al. (2024). Exploring essential oil-based bio-composites: molecular docking and in vitro analysis for oral bacterial biofilm inhibition. Front. Chem. 12, 1383620. doi:10.3389/fchem.2024.1383620
Vaarmann, A., Gandhi, S., and Abramov, A. Y. (2010). Dopamine induces Ca2+ signaling in astrocytes through reactive oxygen species generated by monoamine oxidase. J. Biol. Chem. 285 (32), 25018–25023. doi:10.1074/jbc.M110.111450
Valori, C. F., Guidotti, G., Brambilla, L., and Rossi, D. (2019). Astrocytes: emerging therapeutic targets in neurological disorders. Trends Mol. Med. 25 (9), 750–759. doi:10.1016/j.molmed.2019.04.010
van der Merwe, C., Jalali Sefid Dashti, Z., Christoffels, A., Loos, B., and Bardien, S. (2015). Evidence for a common biological pathway linking three Parkinson's disease-causing genes: parkin, PINK1 and DJ-1. Eur. J. Neurosci. 41 (9), 1113–1125. doi:10.1111/ejn.12872
van Gijn, J., Kerr, R. S., and Rinkel, G. J. (2007). Subarachnoid haemorrhage. Lancet 369 (9558), 306–318. doi:10.1016/S0140-6736(07)60153-6
Villa, R. F., Ferrari, F., and Moretti, A. (2018). Post-stroke depression: mechanisms and pharmacological treatment. Pharmacol. Ther. 184, 131–144. doi:10.1016/j.pharmthera.2017.11.005
Vriend, J., and Reiter, R. J. (2015). The Keap1-Nrf2-antioxidant response element pathway: a review of its regulation by melatonin and the proteasome. Mol. Cell Endocrinol. 401, 213–220. doi:10.1016/j.mce.2014.12.013
Wagner, K. R., Sharp, F. R., Ardizzone, T. D., Lu, A., and Clark, J. F. (2003). Heme and iron metabolism: role in cerebral hemorrhage. J. Cereb. Blood Flow. Metab. 23 (6), 629–652. doi:10.1097/01.WCB.0000073905.87928.6D
Wakade, C., King, M. D., Laird, M. D., and Alleyne, C. H. (2009). Dhandapani KM: curcumin attenuates vascular inflammation and cerebral vasospasm after subarachnoid hemorrhage in mice. Antioxid. Redox Signal 11 (1), 35–45. doi:10.1089/ars.2008.2056
Wang, P., Li, H., Lin, Z., Luo, H., and Luo, W. (2021). Comparing the effect of piperine and ilepcimide on the pharmacokinetics of curcumin in SD rats. Front. Pharmacol. 12, 725362. doi:10.3389/fphar.2021.725362
Wang, Q., Huang, X., Su, Y., Yin, G., Wang, S., Yu, B., et al. (2022). Activation of Wnt/β-catenin pathway mitigates blood-brain barrier dysfunction in Alzheimer's disease. Brain 145 (12), 4474–4488. doi:10.1093/brain/awac236
Wang, Q., Sun, A. Y., Simonyi, A., Jensen, M. D., Shelat, P. B., Rottinghaus, G. E., et al. (2005). Neuroprotective mechanisms of curcumin against cerebral ischemia-induced neuronal apoptosis and behavioral deficits. J. Neurosci. Res. 82 (1), 138–148. doi:10.1002/jnr.20610
Wickman, G., Lan, C., and Vollrath, B. (2003). Functional roles of the rho/rho kinase pathway and protein kinase C in the regulation of cerebrovascular constriction mediated by hemoglobin: relevance to subarachnoid hemorrhage and vasospasm. Circ. Res. 92 (7), 809–816. doi:10.1161/01.RES.0000066663.12256.B2
Wilken, R., Veena, M. S., Wang, M. B., and Srivatsan, E. S. (2011). Curcumin: a review of anti-cancer properties and therapeutic activity in head and neck squamous cell carcinoma. Mol. Cancer 10, 12. doi:10.1186/1476-4598-10-12
Wu, C. T., Wen, L. L., Wong, C. S., Tsai, S. Y., Chan, S. M., Yeh, C. C., et al. (2011). Temporal changes in glutamate, glutamate transporters, basilar arteries wall thickness, and neuronal variability in an experimental rat model of subarachnoid hemorrhage. Anesth. Analg. 112 (3), 666–673. doi:10.1213/ANE.0b013e318207c51f
Xie, L., Ji, X., Zhang, Q., and Wei, Y. (2022). Curcumin combined with photodynamic therapy, promising therapies for the treatment of cancer. Biomed. Pharmacother. 146, 112567. doi:10.1016/j.biopha.2021.112567
Xu, Y., Hu, R., He, D., Zhou, G., Wu, H., Xu, C., et al. (2020). Bisdemethoxycurcumin inhibits oxidative stress and antagonizes Alzheimer's disease by up-regulating SIRT1. Brain Behav. 10 (7), e01655. doi:10.1002/brb3.1655
Yang, K. Y., Lin, L. C., Tseng, T. Y., Wang, S. C., and Tsai, T. H. (2007). Oral bioavailability of curcumin in rat and the herbal analysis from Curcuma longa by LC-MS/MS. J. Chromatogr. B Anal. Technol. Biomed. Life Sci. 853 (1-2), 183–189. doi:10.1016/j.jchromb.2007.03.010
Yin, H., Zhou, Y., Wen, C., Zhou, C., Zhang, W., Hu, X., et al. (2014). Curcumin sensitizes glioblastoma to temozolomide by simultaneously generating ROS and disrupting AKT/mTOR signaling. Oncol. Rep. 32 (4), 1610–1616. doi:10.3892/or.2014.3342
Yu, S., Wang, X., He, X., Wang, Y., Gao, S., Ren, L., et al. (2016). Curcumin exerts anti-inflammatory and antioxidative properties in 1-methyl-4-phenylpyridinium ion (MPP(+))-stimulated mesencephalic astrocytes by interference with TLR4 and downstream signaling pathway. Cell Stress Chaperones 21 (4), 697–705. doi:10.1007/s12192-016-0695-3
Zanotto-Filho, A., Braganhol, E., Edelweiss, M. I., Behr, G. A., Zanin, R., Schroder, R., et al. (2012). The curry spice curcumin selectively inhibits cancer cells growth in vitro and in preclinical model of glioblastoma. J. Nutr. Biochem. 23 (6), 591–601. doi:10.1016/j.jnutbio.2011.02.015
Zeeshan, H. M., Lee, G. H., Kim, H. R., and Chae, H. J. (2016). Endoplasmic reticulum stress and associated ROS. Int. J. Mol. Sci. 17 (3), 327. doi:10.3390/ijms17030327
Zhang, L., Kong, X. J., Wang, Z. Q., Xu, F. S., and Zhu, Y.-T. (2016). A study on neuroprotective effects of curcumin on the diabetic rat brain. J. Nutr. Health & Aging 20 (8), 835–840. doi:10.1007/s12603-016-0723-0
Zhang, X., Yin, W. K., Shi, X. D., and Li, Y. (2011). Curcumin activates Wnt/β-catenin signaling pathway through inhibiting the activity of GSK-3β in APPswe transfected SY5Y cells. Eur. J. Pharm. Sci. 42 (5), 540–546. doi:10.1016/j.ejps.2011.02.009
Keywords: curcumin, neurological disorders, oxidative stress, antioxidant properties, molecular mechanism
Citation: Wei Y, Li H, Li Y, Zeng Y, Quan T, Leng Y, Chang E, Bai Y, Bian Y and Hou Y (2024) Advances of curcumin in nervous system diseases: the effect of regulating oxidative stress and clinical studies. Front. Pharmacol. 15:1496661. doi: 10.3389/fphar.2024.1496661
Received: 15 September 2024; Accepted: 24 October 2024;
Published: 01 November 2024.
Edited by:
Ming Xu, Shimonoseki City University, JapanReviewed by:
Aziz Eftekhari, Ege University, TürkiyeZiyi Wang, Johns Hopkins University, United States
Copyright © 2024 Wei, Li, Li, Zeng, Quan, Leng, Chang, Bai, Bian and Hou. This is an open-access article distributed under the terms of the Creative Commons Attribution License (CC BY). The use, distribution or reproduction in other forums is permitted, provided the original author(s) and the copyright owner(s) are credited and that the original publication in this journal is cited, in accordance with accepted academic practice. No use, distribution or reproduction is permitted which does not comply with these terms.
*Correspondence: Yi Hou, aG91eWk5NEAxNjMuY29t; Yuan Bian, Ymlhbnl1YW54Y0AxNjMuY29t
†These authors have contributed equally to this work and share first authorship