- Department of Oncology, Shengjing Hospital of China Medical University, Shenyang, China
Breast cancer is the most commonly diagnosed cancer worldwide. Metal metabolism is pivotal for regulating cell fate and drug sensitivity in breast cancer. Iron and copper are essential metal ions critical for maintaining cellular function. The accumulation of iron and copper ions triggers distinct cell death pathways, known as ferroptosis and cuproptosis, respectively. Ferroptosis is characterized by iron-dependent lipid peroxidation, while cuproptosis involves copper-induced oxidative stress. They are increasingly recognized as promising targets for the development of anticancer drugs. Recently, compelling evidence demonstrated that the interplay between ferroptosis and cuproptosis plays a crucial role in regulating breast cancer progression. This review elucidates the converging pathways of ferroptosis and cuproptosis in breast cancer. Moreover, we examined the value of genes associated with ferroptosis and cuproptosis in the clinical diagnosis and treatment of breast cancer, mainly outlining the potential for a co-targeting approach. Lastly, we delve into the current challenges and limitations of this strategy. In general, this review offers an overview of the interaction between ferroptosis and cuproptosis in breast cancer, offering valuable perspectives for further research and clinical treatment.
1 Introduction
Breast cancer is the most common cancer among women worldwide (Kciuk et al., 2024). The incidence of breast cancer is increasing each year, and there will be more than 3 million new breast cancer cases annually by 2040 (Arnold et al., 2022). Despite advances in treatments such as surgery, chemotherapy, radiotherapy, targeted therapy, and endocrine therapy (Nagini, 2017), patients still face the risk of recurrence and mortality, with 685,000 deaths recorded in 2020 (Courtney et al., 2022). Consequently, it is urgent to identify novel biomarkers to predict the biological malignancy of tumors and support the development of targeted therapies.
Metal ions are crucial nutrients for living organisms (Jomova et al., 2022), serving as cofactors in nucleic acid and protein functions and playing critical roles in respiration, metabolism, and biosynthesis (Chang, 2015; Witkowska et al., 2021). Maintaining metal homeostasis chiefly depends on precisely regulating metal uptake, distribution, and excretion (Aron et al., 2015; Yang et al., 2024b). Iron, an essential trace transition metal, is indispensable for the functioning of living organisms (Liang and Ferrara, 2020). It plays pivotal roles in oxygen transportation, cell respiration, energy generation, and DNA synthesis (Abbasi et al., 2021). In breast cancer biology, iron has emerged as a critical element, that significantly influences tumor initiation, progression, and response to treatment through mechanisms such as estrogen redox cycling and oxidative stress (Ali et al., 2024; Scimeca and Bonanno, 2018; Torti and Torti, 2013). Maintaining appropriate iron levels is crucial (Anderson and Frazer, 2017) because excessive iron accumulation could be toxic (Bogdan et al., 2016; Ge et al., 2024; Kohgo et al., 2008; Liang and Ferrara, 2020). This toxicity could lead to ferroptosis, a type of cell death driven by iron-dependent lipid peroxidation (Angeli et al., 2014; Rishi et al., 2021; Zarjou et al., 2013). Copper, an essential trace element, plays a critical role in various metabolic processes and is implicated in breast cancer progression due to its involvement in cellular signaling pathways (Ge et al., 2022; Ramchandani et al., 2021; Yang L. et al., 2023). Similar to iron, the regulation of copper levels within human cells is rigorously controlled, as deviations from the optimal concentration impair biological processes and trigger cell death. Perturbations in copper homeostasis could lead to cuproptosis (Li L. et al., 2024), which explicitly affects mitochondrial lipoylated proteins (Tang et al., 2022; Wang et al., 2023b).
Ferroptosis and cuproptosis have garnered significant attention because of their unique features and distinctive regulatory mechanisms (Chen T et al., 2024). Recently, emerging evidence has suggested an intriguing convergence between ferroptosis and cuproptosis, revealing shared regulatory mechanisms (Liu and Chen, 2024; Wang J et al., 2024). In light of this, we aimed to present and explore the intersecting pathways of ferroptosis and cuproptosis within the context of breast cancer. By elucidating these convergences, we endeavor to offer insights that could augment treatment strategies for breast cancer. Concurrently, our review endeavors to assess the genes associated with ferroptosis and cuproptosis in breast cancer, probing their potential as prognostic markers and indicators of treatment response. Additionally, laying the foundation for futuristic opportunities to induce or co-target ferroptosis and cuproptosis holds promise for further enhancing treatment modalities in breast cancer management.
2 Converging pathways of ferroptosis and cuproptosis in breast cancer
2.1 Dysregulation of metal homeostasis
Metal ions are essential nutrients for living organisms, play critical roles as cofactors in nucleic acid and protein functions, and are indispensable for fundamental biological processes (Chang, 2015). Iron and copper share analogous characteristics, serving as vital nutrients and participating in pivotal biological functions. Their roles are indispensable for sustaining health and are relevant for understanding and managing various diseases (Lippard, 1999; Tsang et al., 2021). Within the framework of breast cancer, iron has emerged as a key player in its onset, progression, and relapse. Its impact spans diverse mechanisms, encompassing oxidative stress induction, DNA damage, estrogen signaling modulation, angiogenesis stimulation, and disruption of intracellular iron metabolism (Chang et al., 2019; Huang, 2008; Islam et al., 2022; Torti and Torti, 2013). Correspondingly, copper demonstrates intricate associations with myriad signaling pathways, thereby exerting a substantial influence on the malignant behavior of breast cancer (Chen L. et al., 2022).
Therefore, maintaining optimal iron and copper levels is crucial for biological functions such as oxygen transport, DNA synthesis, and antioxidant defenses (Bleackley and Macgillivray, 2011; Hirota, 2019; MacDonald, 2000; Prasad, 2014). Acknowledging the dual nature of iron and copper in biological systems is pivotal. While these metals are essential for life at appropriate concentrations, excesses and deficiencies can be detrimental. Excessive iron accumulation, for instance, leads to the formation of a labile iron pool, inducing cellular toxicity and contributing to cellular damage (Schneider and Bhatia, 2013). Similarly, inadequate or excessive copper could be detrimental to organismal growth. An overload of copper can heighten cellular toxicity and oxidative stress, impairing cell proliferation and function (Wang et al., 2023e). This imbalance has been linked to various conditions, including cancer, hematological disorders, brain injury, and other chronic ailments frequently encountered in clinical practice (Schneider and Bhatia, 2013). Researchers have ingeniously leveraged the connection between imbalanced metal levels and cancer progression, effectively transforming the modulation of metal levels into a treatment strategy for combating tumors. This approach has led to significant tumor suppression outcomes (Hunsaker and Franz, 2019).
Metal homeostasis modulation involves three dimensions: the removal of surplus intracellular metal ions, their redistribution across tumor cells, and their accumulation at toxic levels within cancerous cells. New strategies for cancer treatment target metal homeostasis, utilizing nanomolecule-based chelators, ionophores, metal complexes, and metal-based nanomaterials. These approaches regulate the tumor microenvironment, inhibit cell proliferation, and induce cell death (Steinbrueck et al., 2020; Weekley and He, 2017).
Iron and copper are central to diverse cellular death pathways, activating various mechanisms such as ferroptosis, cuproptosis, apoptosis, autophagy, necroptosis, and pyroptosis, each with unique pathways. Recent research highlights iron and copper as inducers of ferroptosis, while cuproptosis is a newly identified cell death mode specifically induced by copper (Hirschhorn and Stockwell, 2019; Tsvetkov et al., 2022). Therefore, manipulating cellular iron and copper homeostasis and targeting metabolic pathways could be potential strategies for treating breast cancer by inducing ferroptosis and cuproptosis. Exploring the convergence of iron and copper homeostasis may provide promising therapeutic avenues for breast cancer treatment.
2.1.1 Dysregulation of iron homeostasis
Iron, an essential micronutrient for biological processes (Forbes, 2009), serves critical functions across various metabolic pathways, such as facilitating oxygen transportation, supporting energy metabolism, aiding nucleotide synthesis, and participating in electron transport. Iron homeostasis, which is critical for fundamental physiological processes, is regulated and maintained by iron metabolism (Hentze et al., 2010; Prá et al., 2012). Maintaining iron levels within a balanced range is crucial, as excessive amounts can lead to toxicity (Hentze et al., 2010). Imbalances in the molecular processes governing iron absorption, utilization, storage, and elimination contribute to disease development (Muñoz et al., 2011a; Muñoz et al., 2011b; Theil, 2000). Excessive iron accumulation, coupled with its propensity to generate reactive oxygen species (ROS), suggests its potential involvement in various chronic illnesses, including diabetes, neurological disorders, cardiomyopathy, and several human cancers, such as lung cancer, colorectal cancer, and breast cancer (Jiang et al., 2004; Menshawey et al., 2020; Sempos et al., 1994; Stevens et al., 1988; Ward and Cloonan, 2019). Iron-induced oxidative stress may damage DNA, proteins, and organelles by producing harmful radicals and hydrogen peroxide via Haber–Weiss and Fenton-type reactions (Eaton and Qian, 2002; Nelson, 1992; Toyokuni, 1996). Furthermore, the role of iron in cell proliferation implies its importance in the expansion of malignant cells and may confer a selective advantage for tumor growth (Marques et al., 2014). In breast cancer cases, dysregulation of iron-binding proteins, such as transferrin, which facilitates the delivery of ferric ions into cells, and iron-transporting proteins, like ferroportin, which is responsible for exporting iron out of cells, is frequently observed. One observed phenomenon involves the downregulation of ferroportin levels by hepcidin through posttranscriptional modifications. This results in a greater ratio of hepcidin to ferroportin, ultimately leading to increased ferritin expression and subsequent iron overload (Pinnix et al., 2010; Zhang et al., 2014). Moreover, alterations in transferrin levels have also been noted (Chang et al., 2019; Rufaida Mustafa Ahmed and Nazik Elmalaika Obaid Seid Ahmed, 2017). Iron overload significantly increases the vulnerability of postmenopausal women to breast cancer development by stimulating and fostering oxidative stress (Huang, 2008; Jian et al., 2011). Moreover, recent research has indicated that elevated iron levels within the inflammatory microenvironment of breast tissue may contribute to the progression and metastasis of breast cancer (Cheng et al., 2020). Increased estrogen levels in breast tissue disturb intracellular iron metabolism, leading to excess iron. This excess iron can trigger the generation of superoxide anions and convert ferritin-bound Fe3+ to Fe2+, inducing estrogen-induced oxidative stress on nucleic acids and subsequent breast carcinogenesis (Islam et al., 2022). Therefore, directing interventions toward iron metabolic pathways and modulating cellular iron homeostasis could present a promising avenue for breast cancer treatment, by utilizing strategies such as iron chelation and addressing iron overload.
The excess cellular iron reacts with hydrogen peroxide (H2O2) in the Fenton reaction, producing harmful hydroxyl radicals. These radicals damage lipids, proteins, and DNA, potentially leading to ferroptosis. Ferroptosis, dependent on iron, involves phospholipid peroxidation resulting from disrupted cellular iron homeostasis and redox balance (Dixon et al., 2012; Jiang X et al., 2021). Recently, researchers have delved deeply into the mechanisms of ferroptosis, concentrating on three main areas: iron metabolism and ROS production, lipid metabolism, and the system Xc-GSH-GPX4 pathway (Hassannia et al., 2019; Jiang X et al., 2021). Elevated iron accumulation is necessary to initiate ferroptosis, and alterations in genes and proteins involved in iron metabolism can modulate ferroptosis sensitivity by modifying cellular iron levels (Jiang X et al., 2021). Transferrin (TF) binds to Fe3+ with high affinity in the bloodstream. Once bound to TF, Fe3+ is transported into cells via transferrin receptor 1 (TFR1). Inside endosomes, STEAP3 reduces Fe3+ to Fe2+, which is then transported into the labile iron pool by divalent metal transporter 1 (DMT1). Once in the labile iron pool, Fe2+ contributes to the production of ROS and facilitates lipoxygenase activation. Excess iron is stored in ferritin, which undergoes autophagy-mediated degradation, a process defined as ferritinophagy, releasing labile Fe2+ and promoting lipid peroxidation (Fang et al., 2023; Jiang X et al., 2021; Paul et al., 2017; Zheng and Conrad, 2020). Furthermore, ROS production is augmented by mitochondrial metabolism (Jiang X et al., 2021; Stockwell, 2022). Importantly, cellular iron import or export is critical for regulating ferroptosis. Transferrin and its receptor (TFR1) enable iron transport into cells, thus initiating ferroptosis (Figure 1) (Wang et al., 2023b). The modulation of ferroptosis involves regulating the expression of TFR1, illustrating its capacity to either promote or inhibit the process. Ferritin heavy chain 1 (FTH1) modulates iron storage by interacting with NCOA4, which initiates iron autophagy. This process releases iron, triggering mitochondrial lipid peroxidation and subsequent ferroptosis (Fang et al., 2021). Research indicates that knocking out NCOA4 diminishes iron autophagy, making cells more resistant to ferroptosis (Fang et al., 2021). Moreover, ferroportin (FPN), an essential iron exporter for maintaining iron balance (Yang et al., 2020), has been identified as a potential target for preventing ferroptosis through the regulation of FPN protein degradation (Traeger et al., 2022; Yang et al., 2020). Hence, modulating cellular sensitivity to ferroptosis through regulating iron metabolism has emerged as an effective strategy for treating ferroptosis-related diseases (Jiang X et al., 2021). Clinically, iron chelators are employed to eliminate excess iron from the body, representing a viable treatment option for managing diseases associated with ferroptosis (Feng W. et al., 2022). Polyunsaturated fatty acid phospholipids (PUFA-PLs) act as key substrates for lipid peroxidation (LPO) (Feng W. et al., 2022), and the biosynthetic pathway of PUFA-PLs is crucial in regulating the initiation of ferroptosis (Feng W. et al., 2022; Li and Li, 2020). Lipid metabolism, one of the three core metabolic pathways, is critical in the development of cardiovascular disease, obesity, cancer, and other conditions (Bacci et al., 2021). As a result, enzymes regulating lipid synthesis, breakdown, and β-oxidation have become prominent therapeutic targets (Zechner et al., 2012). Abnormalities in lipid metabolism, particularly in fatty acid metabolism, are now widely acknowledged as key drivers in the pathogenesis of ferroptosis (Li and Li, 2020; Liang et al., 2022). Monounsaturated fatty acids (MUFAs) and polyunsaturated fatty acids (PUFAs) exert opposing effects on ferroptosis, likely due to their differences in chemical structure and oxidative stability, which influence their susceptibility to lipid peroxidation and thereby modulate ferroptosis (Li and Li, 2020; Magtanong et al., 2019). The balance between MUFA-PLs and PUFA-PLs, derived from monounsaturated and polyunsaturated fatty acids, respectively, is critical in modulating cellular sensitivity to ferroptosis (Li and Li, 2020; Magtanong et al., 2019). In this process, acetyl-CoA is first converted to malonyl-CoA via acetyl-CoA carboxylase (ACC), leading to the production of PUFAs. Long-chain acyl-CoA synthetase 4 (ACSL4) and lysophosphatidylcholine acyltransferase 3 (LPCAT3) (Stockwell, 2022) then facilitate the incorporation of PUFAs into phospholipids (PUFA-PLs) (Stockwell, 2022). Under the action of iron-dependent lipoxygenases and reactive oxygen species (ROS), PUFA-PLs are oxidized into PUFA-PL-OOH, triggering the onset of ferroptosis (Li and Li, 2020; Magtanong et al., 2019). Furthermore, the enzymatic activities of ACSL3 and ACSL4 play a critical role in modulating cellular sensitivity to ferroptosis (Figure 1) (Li and Li, 2020; Magtanong et al., 2019). Interestingly, ACSL3 activation has been found to protect cells from ferroptosis, with studies highlighting its role in gastric and breast cancer cells (Ma et al., 2022; Xie et al., 2022).
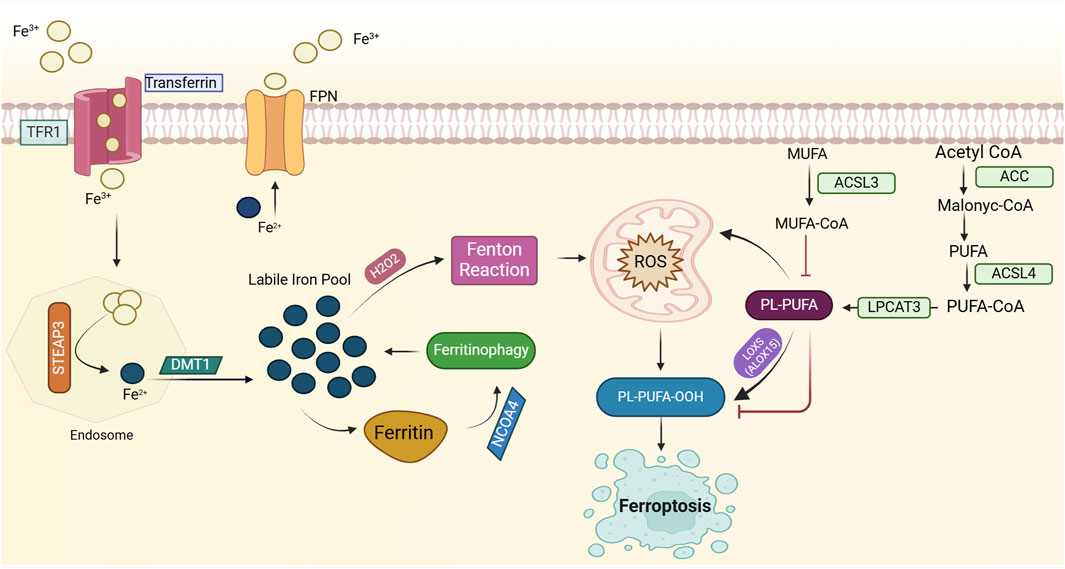
Figure 1. Mechanisms of ferroptosis induction. Ferroptosis is an iron-dependent form of cell death characterized by lipid peroxidation. Elevated intracellular iron, imported via transferrin (TF) and transferrin receptor 1 (TFR1), is reduced to its ferrous form (Fe2⁺) by STEAP3, and subsequently transported to the labile iron pool by DMT1. This labile iron contributes to the generation of reactive oxygen species (ROS), particularly hydroxyl radicals (⋅OH), through the Fenton reaction, where Fe2⁺ catalyzes the conversion of hydrogen peroxide (H₂O₂) into highly reactive ROS. ROS, in turn, initiate oxidative damage, including the peroxidation of polyunsaturated fatty acid phospholipids (PUFA-PLs), which serve as key substrates for ferroptosis. Enzymes such as acetyl-CoA carboxylase (ACC), long-chain acyl-CoA synthetase 4 (ACSL4), and lysophosphatidylcholine acyltransferase 3 (LPCAT3) facilitate the incorporation of PUFAs into phospholipids, thereby promoting lipid peroxidation. Ferroptosis is further exacerbated by ferritinophagy, a process that releases iron from ferritin stores, thereby increasing ROS production through NCOA4-mediated ferritin degradation. Lipid peroxidation is driven by iron-dependent lipoxygenases, producing toxic phospholipid hydroperoxides (PUFA-PL-OOH). Cellular sensitivity to ferroptosis is regulated by the dynamic balance of iron import/export (e.g., TFR1, ferroportin (FPN)), iron storage (e.g., ferritin), and lipid metabolism. Please see the text for specific details. Fe3+, ferric ion; Fe2+, ferrous ion; TFR1, transferrin receptor 1; FPN, ferroportin; STEAP3, six transmembrane epithelial antigen of prostate 3; H2O2, hydrogen peroxide; ROS, reactive oxygen species; PL-PUFA-OOH, phospholipid polyunsaturated fatty acid hydroperoxide, NCOA4, Nuclear Receptor Coactivator 4; ROS, Reactive Oxygen Species; MUFA, Monounsaturated Fatty Acids; ACSL3, Acyl-CoA Synthetase Long-Chain Family Member 3; MUFA-CoA, Monounsaturated Fatty Acid Coenzyme A; PL-PUFA, Phospholipid Polyunsaturated Fatty Acids; LOXS, Lipoxygenases; PL-PUFA-OOH, Phospholipid Polyunsaturated Fatty Acid Hydroperoxide; LPCAT3, Lysophosphatidylcholine Acyltransferase 3; PUFA, Polyunsaturated Fatty Acids; ACSL4, Acyl-CoA Synthetase Long-Chain Family Member 4; ACC, Acetyl-CoA Carboxylase.
2.1.2 Dysregulation of copper homeostasis
Copper, a crucial trace transition metal, is essential for enzymes and proteins in diverse organisms and influences biological processes like mitochondrial function, respiration, antioxidant defense, and cell proliferation. It exists primarily in two oxidation states, Cu+ and Cu2+, impacting its bioactivity (Ying et al., 2023). Cellular copper homeostasis is tightly regulated by copper-dependent proteins such as copper transporter 1 (CTR1) for uptake, copper chaperones for transport, and copper-transporting P-type ATPases (copper ATPases) for export, ensuring that optimal intracellular copper levels are crucial for overall health. Disruptions in copper homeostasis have been observed in various cancers like colorectal, lung, and breast cancer (Chen L. et al., 2022; Denoyer et al., 2015; Jiang Z et al., 2023). Copper holds significant importance in tumorigenesis and cancer progression, as it can bind and activate key molecules within multiple signaling pathways present in cancer, including breast cancer cells. Within these pathways, the Wnt signaling pathway is vital for maintaining breast cancer stem cells (BCSCs) stemness. Recent studies have suggested that disulfiram/copper (DSF/Cu) complexes can hinder cancer cell proliferation and metastasis by reducing the expression of β-catenin and C-myc, which are critical components of the Wnt pathway (Shivnani et al., 2023; Wang et al., 2020). In addition, the Notch pathway is essential for multiple biological processes in breast cancer cells, encompassing cell differentiation, apoptosis, and cell cycle progression (Farnie and Clarke, 2007). In essence, copper influences breast cancer metastasis through the promotion of Notch ligand shedding and the activation of key signaling pathways, such as the RAS-RAF-MEK-ERK and receptor tyrosine kinase (RTK)-related pathways, ultimately facilitating cancer cell migration and proliferation (Grasso et al., 2021). Furthermore, several studies have shown elevated copper levels in tumor tissues and serum across different cancer types, including breast, gastric, and lung cancers. In breast cancer patients, high serum copper levels are correlated with advanced tumor stage and disease progression (Guo et al., 2021; Heuberger et al., 2022; Jin et al., 2022; Pavithra et al., 2015). These findings underscore the significant regulatory role of copper in breast cancer signaling pathways, emphasizing its importance in treatment strategies. Thus, targeting alterations in copper homeostasis holds promise as a strategy to combat breast cancer.
Excessive copper can induce the death of breast cancer cells, primarily through a newly identified regulatory cell death mechanism known as cuproptosis (Figure 2) (Tsvetkov et al., 2022; Wang et al., 2023e). Copper uptake into cells is predominantly mediated by copper transporter 1 (CTR1), also known as solute carrier family 31 member 1 (SLC31A1) (Kuo et al., 2001). Cellular copper homeostasis is tightly regulated by adaptive mechanisms that modulate CTR1 expression based on intracellular copper levels. In response to copper depletion, CTR1 expression is upregulated to enhance copper uptake, whereas excess intracellular copper triggers the downregulation of CTR1 to prevent toxicity (Kuo et al., 2012). After entering the intracellular environment, copper is directed to specific subcellular compartments where it is either utilized for essential biochemical processes or sequestered to avoid toxicity. This precise trafficking is mediated by a network of copper chaperone proteins, ensuring its proper distribution and regulation within the cell. Cytochrome c oxidase copper chaperone (COX17) is essential for the delivery of copper to the mitochondrial cytochrome c oxidase complex, a process crucial for sustaining copper homeostasis within the electron transport chain. This copper-dependent mechanism is vital for the proper function of oxidative phosphorylation, enabling efficient ATP production (Palumaa et al., 2004). An additional key mediator in copper distribution is the copper chaperone for superoxide dismutase (CCS), which facilitates the transfer of copper to superoxide dismutase 1 (SOD1). SOD1, a critical antioxidant enzyme, plays a pivotal role in mitigating oxidative stress by catalyzing the dismutation of superoxide radicals, thereby protecting cells from ROS (Wright, 2020). Additionally, the antioxidant 1 copper chaperone (ATOX1) facilitates the transfer of copper to the ATPase copper-transporting α and β (ATP7A and ATP7B or ATP7/B), which are transmembrane proteins responsible for copper efflux. It ensures the regulated export of excess intracellular copper, maintaining copper homeostasis and preventing toxic accumulation (Hatori and Lutsenko, 2016; Schmidt et al., 2018). ATP7A/B primarily reside in the trans-Golgi network (TGN), where they facilitate copper transport from the cytosol into the TGN lumen, ensuring copper homeostasis and delivering it to enzymes in the secretory pathway. Elevated intracellular copper levels induce the translocation of these proteins from the TGN to vesicular compartments, which fuse with the plasma membrane to export copper. Once copper levels return to normal, ATP7A and ATP7B relocate back to the TGN (Hasan et al., 2012). As a result of their critical role in copper transport, mutations in ATP7A and ATP7B disrupt copper homeostasis, leading to Menkes disease and Wilson disease, respectively (de Bie et al., 2007). Proteins regulating copper metabolism interact intricately to maintain intracellular copper homeostasis. However, disruptions in copper homeostasis can result in excessive copper accumulation within cells, potentially leading to cuproptotic cell death. Cancer cells dependent on mitochondrial respiration exhibit increased vulnerability to cuproptosis, with mitochondrial electron transport chain inhibitors suppressing this form of cell death, highlighting the link between cuproptosis and mitochondrial metabolism (Tsvetkov et al., 2022). CRISPR screens have identified key regulators of cuproptosis, with ferredoxin 1 (FDX1) emerging as a central player. FDX1, a target of the copper ionophore elesclomol, reduces Cu2⁺ to its more toxic form, Cu⁺, and regulates protein lipoylation through interaction with lipoic acid synthetase (LIAS), which catalyzes the final step of lipoic acid biosynthesis (Figure 2) (Tsvetkov et al., 2022; Tsvetkov et al., 2019). Protein lipoylation, a posttranslational modification, is essential for the function of key enzymes in the mitochondrial tricarboxylic acid (TCA) cycle, including dihydrolipoamide S-acetyltransferase (DLAT), a subunit of the pyruvate dehydrogenase complex (Rowland et al., 2018). Genetic depletion of LIAS and DLAT, like FDX1 deletion, confers resistance to cuproptosis (Tsvetkov et al., 2022). Notably, despite their crucial role in cuproptosis, levels of FDX1, LIAS, and protein lipoylation decline during the process, potentially indicating a cellular attempt to counteract excessive copper toxicity by dampening protein lipoylation—an ultimately futile strategy. These findings emphasize the pivotal role of protein lipoylation in cuproptosis. The exact mechanisms by which protein lipoylation triggers cuproptosis remain unclear. However, direct binding of copper to lipoylated DLAT has been observed, resulting in protein oligomerization during cuproptosis (Tsvetkov et al., 2022). This suggests that the resultant protein aggregates may induce a toxic gain-of-function effect, ultimately driving cuproptotic cell death (Figure 2). These findings highlight a promising area for future investigation into the complex and distinctive process of cuproptosis.
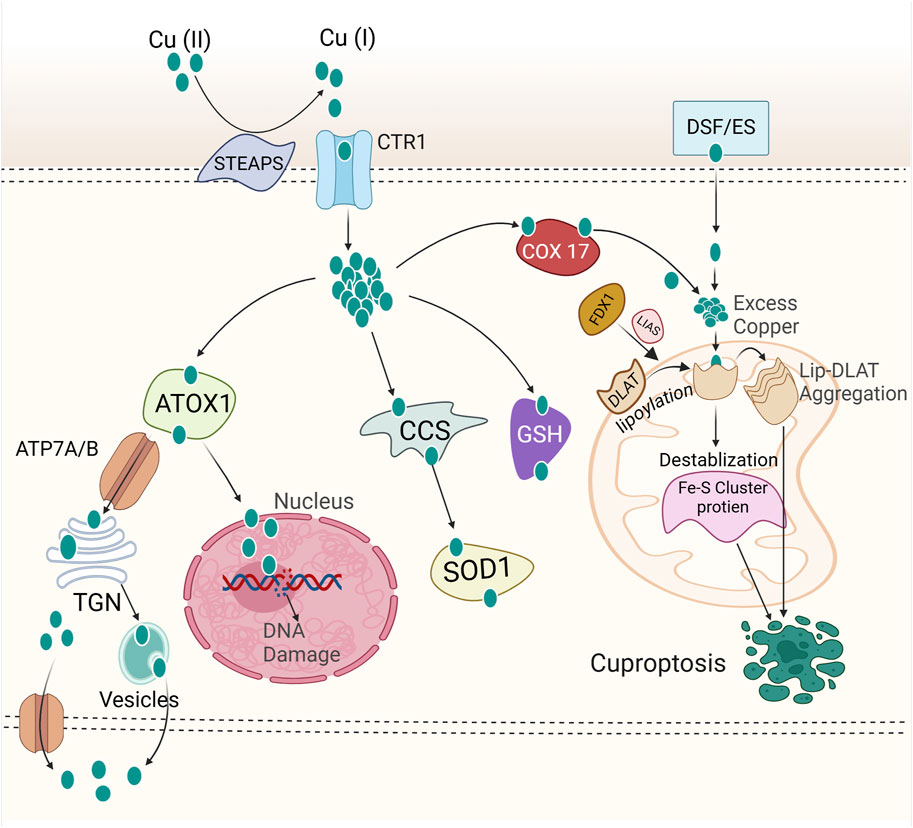
Figure 2. Mechanism of cuproptosis. Copper ions are reduced to their monovalent form (Cu⁺) by STEAP family proteins. Extracellular copper is imported into cells via copper ionophores (e.g., elesclomol) or through copper transporters such as CTR1 and DMT1. Once copper enters the cell, it binds to various copper chaperone proteins, such as ATOX1, CCS, and SOD1, which facilitate its transport to specific subcellular compartments, including the mitochondria, trans-Golgi network (TGN), and nucleus. The nucleus is implicated in cuproptosis through copper’s role in regulating gene expression and DNA repair. Disrupted copper homeostasis induces oxidative stress and DNA damage responses, promoting cellular stress that could lead to cuproptosis. ATP7A and ATP7B or ATP7A/B mediate copper efflux, maintaining intracellular copper homeostasis. Inside the cell, FDX1 reduces Cu2⁺ to Cu⁺, which interacts with lipoic acid synthetase (LIAS) to facilitate the lipoylation of key metabolic enzymes, including DLAT. It is hypothesized that Cu⁺ directly binds to lipoylated proteins, promoting their oligomerization. This oligomerization results in a toxic gain-of-function, ultimately triggering cell death via cuproptosis. Please see the text for specific details. FDX1, Ferredoxin 1; DLAT, Dihydrolipoamide S-acetyltransferase; CTR1, Copper Transporter 1; COX17, Cytochrome c Oxidase Copper Chaperone 17; DSF/ES, Disulfiram/Elesclomol; STEAPS, Six Transmembrane Epithelial Antigen of Prostate; ATOX1, Antioxidant 1 Copper Chaperone; SOD1, Superoxide Dismutase 1; CCS, Copper Chaperone for Superoxide Dismutase; GSH, Glutathione; TGN, Trans-Golgi network; ATP7A/B, ATPase copper transporting α and ATPase copper transporting β.
2.1.3 Convergence between iron and copper homeostasis
Copper complexed with disulfiram has attracted increased amounts of attention due to its anticancer effects (Zha et al., 2014). A recent study demonstrated that DSF/Cu activates ferroptosis in TNBC cells. Treatment with DSF/Cu led to increased lipid peroxidation, upregulation of HMOX1, and decreased levels of GPX4 and GSH, ultimately inducing cancer cell death through ferroptosis (Chu et al., 2023). DSF/Cu has emerged as a promising agent for inducing ferroptosis in TNBC cells, offering potential avenues for anticancer therapy (Ren et al., 2021).
The copper homeostasis gene, prion protein (PRNP), was notably downregulated in breast cancer cells, correlating with a better prognosis (Lin et al., 2023). PRNPs are involved in cancer-related signaling pathways, particularly those governing inflammatory responses and oxidative phosphorylation. PRNP overexpression markedly enhanced gefitinib sensitivity in BRCA cells. Overexpression of PRNP led to elevated ROS production following gefitinib treatment, while the ferroptosis-selective inhibitor ferrostatin-1 mitigated this increase in ROS levels in BRCA cells. PRNP expression was positively correlated with macrophages, Th1 cells, neutrophils, and B cells, while negatively correlated with NK CD56 bright cells and Th17 cells in BRCA. Single-cell analysis showed that PRNP was highly expressed in M1 phenotype macrophages, essential tumor-suppressing cells in the tumor stroma. These observations suggest that PRNP is potentially involved in ROS-mediated ferroptosis, highlighting its candidacy as a novel therapeutic target for chemotherapy and immunotherapy in breast cancer. Additionally, PRNP is correlated with a better prognosis and regulates ferroptosis following gefitinib treatment in breast cancer cells (Lin et al., 2023).
The convergence of iron and copper homeostasis is a promising avenue for inducing ferroptosis and cuproptosis in cancer cells. DSF/Cu and PRNP modulation offer potential therapeutic strategies for cancer treatment (Chu et al., 2023; Lin et al., 2023). Future research could focus on elucidating the precise mechanisms underlying the interplay between copper and iron homeostasis in regulating ferroptosis and cuproptosis in cancer cells. Additionally, exploring novel therapeutic agents/complexes that target both ferroptosis and cuproptosis could pave the way for advancements in breast cancer treatment.
2.2 Mitochondrial metabolism
Mitochondria, crucial for cellular energy production, play vital roles in various physiological and pathological processes (Brand et al., 2013). Mitochondria play a crucial role in the development of numerous human diseases and the regulation of multiple cell death pathways (Javadov et al., 2020). Research has revealed intimate connections between mitochondrial metabolism and ferroptosis, and cuproptosis (Wang J. et al., 2024), highlighting the need to characterize mitochondria for insights into underlying mechanisms, regulatory pathways, and disease ramifications. Mitochondria depend on iron, copper, and calcium for optimal ATP generation. The presence of these metal ions modulates the mitochondrial electron transport chain and other functions, influencing both ferroptosis and cuproptosis (Figure 3). This highlights a potential for anticancer therapy by targeting mitochondrial metabolism to address cuproptosis and ferroptosis simultaneously (Prasad Panda and Kesharwani, 2023). Moreover, the mitochondrial TCA cycle has emerged as a pivotal convergence point for ferroptosis and cuproptosis pathways, further underscoring its importance in governing cellular fate regulation (Liu and Chen, 2024).
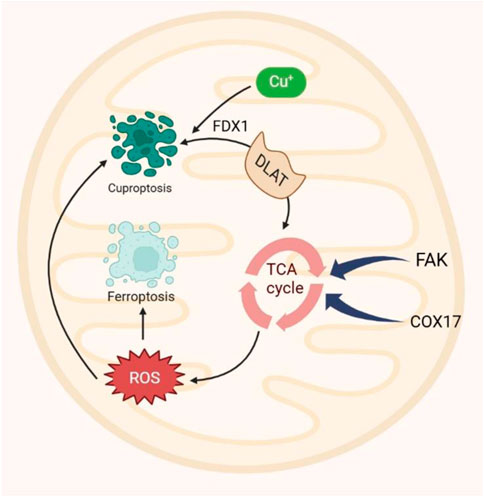
Figure 3. Mitochondria play a central role in ferroptosis by serving as major sources of intracellular ROS. Similarly, cuproptosis occurs in mitochondria, with the TCA cycle acting as a shared pathway for both processes. Dihydrolipoamide S-acetyltransferase (DLAT), a key TCA cycle component, is lipoylated by FDX1, and its interaction with copper triggers cuproptosis. This underscores the mitochondrial TCA cycle as a convergence point for ferroptosis and cuproptosis. Additionally, COX7A1 and FAK accelerate the TCA cycle, promoting the production of metabolites that drive ferroptosis and cuproptosis. ROS, reactive oxygen species; COX7A1, cytochrome c oxidase 7A1; FAK; focal adhesion kinase. FDX1, ferredoxin 1.
2.2.1 Mitochondrial metabolism and ferroptosis in breast cancer
Mitochondria have elevated iron concentrations (Lill and Freibert, 2020). Ferroptosis results in prominent morphological changes and dysfunction in mitochondria (Doll et al., 2017). The interaction between mitochondria and ferroptosis is well-documented, as they jointly regulate iron metabolism and lipid peroxidation (Wang J et al., 2024). While various therapeutic modalities, including endocrine therapy, immunotherapy, radiation, targeted therapy, and chemotherapy, are available for breast cancer treatment (Dong et al., 2024), interventions specifically targeting mitochondria to induce ferroptosis remain underexplored. The recently identified iron-sulfur proteins CISD1 and CISD2 facilitate the proliferation of breast cancer cells (Sohn et al., 2013). Silencing CISD1 and CISD2 or targeting breast cancer mitochondria with the mitogen derivative MAD-28 can destabilize iron-sulfur proteins, elevate mitochondrial iron accumulation, and hinder breast cancer cell growth (Bai et al., 2015). MAD-28 is highly selective, causing iron accumulation in the mitochondria of breast cancer cells only while leaving normal breast cells unaffected. This selectivity suggests that MAD-28 could be a promising agent for targeting ferroptosis and developing anticancer therapies (Dong et al., 2024). In addition, lipid metabolism, a crucial mitochondrial pathway in ferroptosis, has numerous applications in breast cancer diagnosis and treatment, with a focus on its regulation. Stearoyl-CoA desaturase-1 (SCD1) is upregulated in recurrent human breast cancer samples, indicating a poor prognosis for cancer patients. Thus, the expression of SCD1 can serve as a biomarker for breast cancer recurrence (Luis et al., 2021). Furthermore, Herceptin, also known as trastuzumab, a medication targeting HER-2 in breast cancer treatment, was found to elevate mitochondrial ROS levels in rat cardiomyocytes while reducing GPX4 expression, thereby leading to ferroptosis. The subsequent application of the ferroptosis inhibitor ferrostatin-1 successfully reversed the increase in ROS levels. This observation indicates the potential utility of ferroptosis inhibitors in mitigating cardiotoxicity associated with HER-2-positive breast cancer therapy (Sun L. et al., 2022). Furthermore, manganese superoxide dismutase 2 (SOD2), a key enzyme within the ETC, has emerged as a potential biomarker for breast cancer progression and a target for ferroptosis induction (Sui et al., 2022). Nanomaterial-based drugs, like sorafenib (Louandre et al., 2013) and simvastatin (Yao X. et al., 2021), hold considerable potential for inducing efficient ferroptosis in breast cancer cells without systemic toxicity (Li K. et al., 2022; Sang M et al., 2019; Yao X. et al., 2021).
In conclusion, mitochondria serve as central regulators of ferroptosis in breast cancer, and targeting mitochondrial metabolism represents a promising avenue for inducing ferroptosis in specific breast cancer subtypes. However, further research is warranted to elucidate the precise underlying mechanisms and optimize therapeutic strategies targeting mitochondrial pathways for effective breast cancer treatment.
2.2.2 Mitochondrial metabolism and cuproptosis in breast cancer
Mitochondria are pivotal copper-dependent organelles responsible for energy production through the indispensable cuproenzyme cytochrome c oxidase (CCO) (Carr and Winge, 2003). Disturbances in intracellular copper homeostasis, including loss-of-function mutations in genes crucial for copper regulation, invariably result in lethal genetic disorders such as Wilson disease and Menkes disease (Członkowska et al., 2018; Tümer and Møller, 2010). The mitochondrial TCA cycle is pivotal in the process of cuproptosis, where protein lipoylation is confined to four specific proteins (DBT, GCSH, DLST, and DLAT) involved in this cycle. This process is primarily mediated by the mitochondrial carrier family (MCF) (Chen L. et al., 2022; Tang et al., 2022).
Cuproptosis initiates with the accumulation of copper in the cytoplasm and organelles. This prompts the clustering of mitochondrial lipoylated modules and the destabilization of Fe-S cluster proteins essential for mitochondrial function (Chen L. et al., 2022; Tang et al., 2022). Cuproptosis is intricately connected to mitochondrial respiration, and the status of mitochondrial respiratory function impacts its susceptibility (Tang et al., 2022; Yuan et al., 2022). Recently, significant attention has been directed toward understanding the role of mitochondria and cuproptosis in breast cancer (Gururaja Rao, 2017; Liu X. et al., 2023; Porporato et al., 2018). Breast cancer cells undergo metabolic reprogramming, shifting from glycolysis to increased mitochondrial oxidative phosphorylation (OXPHOS) and metabolism, supporting rapid proliferation and metastasis (Li and Li, 2021). Mitochondrial dynamics exhibit flexibility, transitioning between different forms to adapt to microenvironmental changes and therapeutic stress, aiding cancer cell survival (Avagliano et al., 2019). Breast cancer stem cells (CSCs) and circulating tumor cells (CTCs) rely heavily on mitochondrial metabolism and OXPHOS for tumor initiation, metastasis, and treatment resistance (Wedam et al., 2023). Key oncogenes and tumor suppressors, such as Myc, TP53, PIK3CA, and Bcl-2 family proteins, regulate mitochondrial metabolism, contributing to tumor progression (Wedam et al., 2023). Mitochondrial dysfunction and alterations in metabolic pathways, such as those involving lipids, amino acids, and the TCA cycle, contribute to drug resistance, suggesting that targeting these pathways could enhance chemotherapy efficacy (Li and Li, 2021; Wedam et al., 2023). Importantly, targeting mitochondrial metabolism through the induction of cuproptosis represents a significant research avenue for cancer therapy, including breast cancer treatment. Breast cancer cells that rely on mitochondrial respiration are particularly susceptible to cuproptosis induction (Ai L. et al., 2024; Xie et al., 2023). Targeting mitochondria to induce cuproptosis in breast cancer cells leverages the role of copper in triggering cell death (Wang J. et al., 2024).
2.3 GSH metabolism
GSH plays a pivotal role in both ferroptosis and cuproptosis, serving distinct functions in each pathway (Badgley et al., 2020; Tsvetkov et al., 2022). In ferroptosis, it acts as an antioxidant, inhibiting lipid peroxidation (LPO), while in cuproptosis, it functions as a copper chaperone, binding copper to mitigate the aggregation of lipoylated proteins (Badgley et al., 2020; Tsvetkov et al., 2022). Interestingly, GSH has been shown to have inhibitory effects on both ferroptosis and cuproptosis, suggesting a converging point (Liu and Chen, 2024).
Depending on the cellular context, p53, a tumor suppressor, can inhibit or promote ferroptosis. The p53/p21 pathway activation enhances GSH synthesis, inhibiting phospholipid peroxidation and preventing ferroptosis (Tang D. et al., 2020). It is noteworthy that when lipid peroxidation damage is mild and repairable, p53 acts to prevent ferroptosis by promoting cellular repair mechanisms. However, when the damage is extensive or irreparable, p53 triggers ferroptosis to eliminate the damaged cells (Xu R. et al., 2023). The canonical p53 pathway involved in ferroptosis regulation includes modulation of iron metabolism. The p53 enhances the entry of Fe2⁺ into cells by upregulating the expression of TFR1 through lncRNA PVT1, and it also stimulates the production of reactive iron species by regulating SLC25A28 (Zhang Z. et al., 2020) and FDXR (Zhang et al., 2017), thereby facilitating ferroptosis (Xu R. et al., 2023). Moreover, buthionine sulfoximine, a GSH synthesis inhibitor, induces both ferroptosis and cuproptosis, making it a promising therapeutic candidate (Figure 4) (Jiang et al., 2015; Li Y. et al., 2020; Luo et al., 2021; Rappa et al., 2003; Tang D. et al., 2021; Tsvetkov et al., 2022). However, further research and clinical trials are needed to explore its potential.
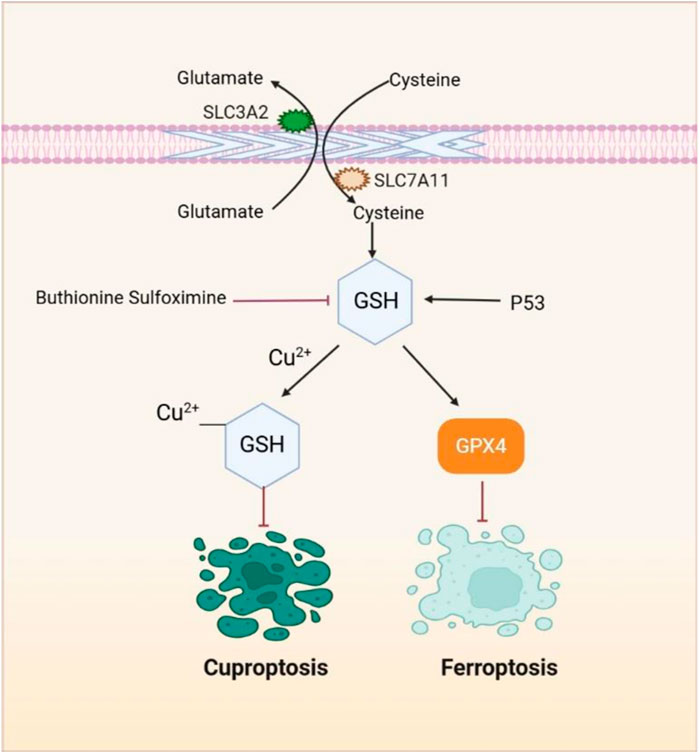
Figure 4. GSH, a pivotal element, plays a crucial role in both the ferroptosis and cuproptosis pathways. SLC7A11 facilitates the transport of cystine into cells, which is then used for the synthesis of GSH. As a potent reducing agent, GSH serves as a cofactor for GPX4, enabling GPX4 to degrade lipid peroxides and suppress lipid peroxidation and ferroptosis. Additionally, GSH functions as a copper chaperone, binding to copper and reducing its intracellular accumulation, thus preventing cuproptosis. Compounds such as buthionine sulfoximine induce both ferroptosis and cuproptosis by inhibiting GSH synthesis.
2.3.1 GSH metabolism and ferroptosis
In breast cancer, elevated GSH levels are frequently observed, especially in TNBC, potentially attributed to estrogen-mediated downregulation of transferrin receptor expression (Ge A. et al., 2024). Such heightened GSH levels contribute to resistance against ferroptosis and other cell death modalities, fostering cancer progression and therapeutic resistance (Liu Y. et al., 2022). Notably, GSH-rich environments in breast cancer cells counteract the cytotoxic effects of anticancer agents like cisplatin and paclitaxel by neutralizing ROS, thereby conferring treatment resistance. However, interventions targeting GSH metabolism, such as benzothiazole-mediated GSH inhibition, have demonstrated promising efficacy in sensitizing breast cancer cells to conventional treatments (Ge A. et al., 2024). Therefore, manipulating GSH levels holds therapeutic promise in breast cancer management. Strategies aimed at GSH depletion, achieved through inhibition of the cystine/glutamate antiporter or GPX4 inactivation, induce ferroptosis and enhance treatment efficacy (Ge A. et al., 2024; Xu C. et al., 2023). Reducing GSH levels has been shown to alleviate radiation resistance, particularly through hypoxia-inducible factor-1 (HIF-1) mediated metabolic reprogramming. Clinical implications include the development of therapeutic approaches targeting GSH metabolism, combining therapies integrating ferroptosis inducers with conventional treatments, and identifying GSH levels and ferroptosis-related gene expression as predictive biomarkers (Xu C. et al., 2023; Yang F. et al., 2023; Zhou T. J. et al., 2024). This comprehensive understanding of the interplay between GSH metabolism and ferroptosis offers novel avenues for refining breast cancer therapy and improving patient outcomes.
2.3.2 GSH metabolism and cuproptosis
Ferroptosis inducers like sorafenib and erastin downregulate GSH synthesis, decreasing intracellular GSH levels. This refers to increased cuproptosis, compromising copper-chelating capacity and promoting labile copper accumulation. Restoring GSH levels reversed the sensitizing effect of ferroptosis inducers on copper-induced cytotoxicity and cuproptosis. Combining GSH synthesis inhibitors such as buthionine sulfoximine with copper ionophores could be a potential therapeutic approach for inducing cuproptosis in breast cancer cells. However, specific studies are needed to explore their efficacy and safety (Mao et al., 2024; Wang W. et al., 2023; Wang Y. et al., 2024).
2.4 Autophagy
Autophagy is pivotal for maintaining cellular homeostasis under physiological and pathological conditions by facilitating the sequestration and degradation of various cellular components to meet metabolic demands and ensure organelle renewal (Levine and Kroemer, 2019; Mizushima and Komatsu, 2011). Perturbations in copper homeostasis are widely acknowledged to regulate not only ferroptosis and cuproptosis pathways but also the activation of autophagy (Li Y. et al., 2021; Xue et al., 2023a; Yang et al., 2021). Excess copper in cells activates transcription factors, upregulates ATG5 expression, and modulates the AMPK-mTOR pathway, thereby inducing autophagy (Xue et al., 2023a; Yang et al., 2021) and establishing potential cross-talk between ferroptosis and cuproptosis (Figure 5).
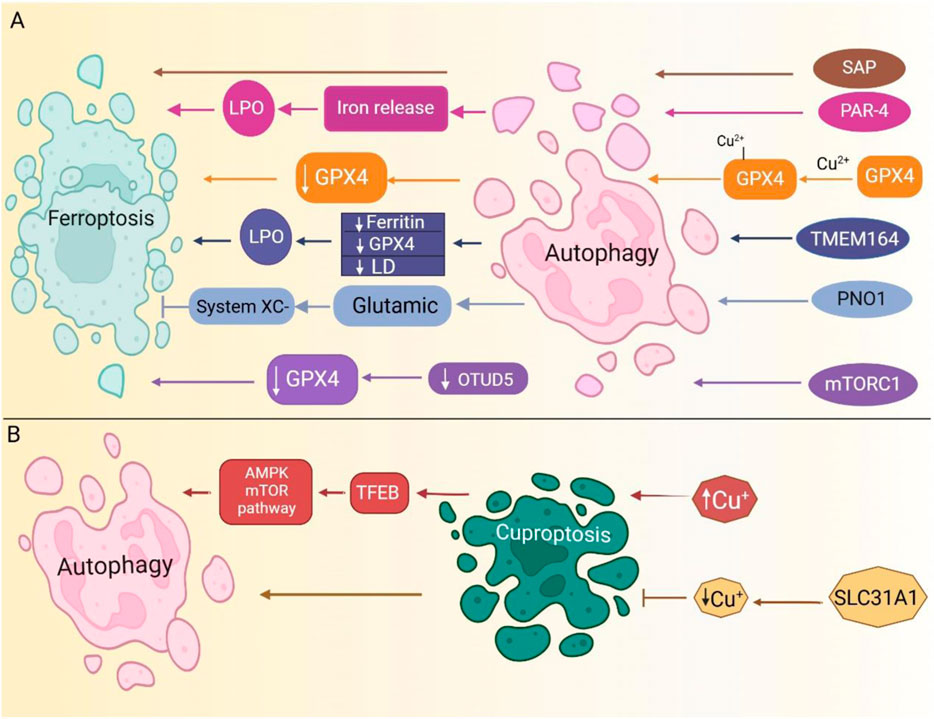
Figure 5. Autophagy serves as a key regulator of both ferroptosis and cuproptosis. (A). Several signaling pathways induce autophagy. PAR-4 upregulation triggers ferritinophagy, resulting in free iron release, increased lipid peroxidation (LPO), and ferroptosis induction. Additionally, mTORC1-mediated autophagy downregulates OTUD5, leading to GPX4 reduction and subsequent ferroptosis. SAP upregulation further enhances autophagy, driving ferroptosis. (B). Modulating copper uptake regulates autophagy pathways. For further details, please refer to the main text. (Routes are color-coded to indicate distinct pathways). SAP, severe acute pancreatitis; LPO, lipid peroxidation; PAR-4, Prostate apoptosis response-4; OTUD5, OTU deubiquitinase 5; mTORC1, mammalian target of rapamycin complex 1; LD, lipid droplets.
2.4.1 Autophagy and ferroptosis
The interaction between autophagy and ferroptosis in breast cancer is multifaceted, with autophagy exhibiting both promoting and protective roles in ferroptosis induction. Autophagy facilitates ferroptosis by promoting iron accumulation and lipid peroxidation, which are crucial events in ferroptosis initiation. Specific autophagic processes such as ferritinophagy and lipophagy release iron and lipids, promoting ferroptosis (Gao et al., 2016; Li J. et al., 2021; Liu et al., 2020). Conversely, inhibition of autophagy-related genes or cargo receptors impedes ferritin degradation, thus preventing ferroptosis onset. Moreover, ferroptosis inducers like erastin and sulfasalazine can induce autophagy in breast cancer cells. This induction of autophagy, however, seems to diminish the efficacy of ferroptosis inducers in killing breast cancer cells. Inhibiting autophagy in such conditions sensitizes breast cancer cells to ferroptosis inducers (Chipurupalli et al., 2023). The interplay among ferroptosis, autophagy, and iron metabolism underscores their interconnectedness in breast cancer pathophysiology.
Both ferroptosis and autophagy are driven by iron-dependent ROS generation, and alterations in intracellular iron levels and ferritin expression contribute to their respective cell death mechanisms. Heme oxygenase-1, which increases the cellular free iron pool, can further enhance ferroptosis in breast cancer cells (Chipurupalli et al., 2023; Lee et al., 2023; Ma et al., 2017; Xu C. et al., 2023). Moreover, in pancreatic cancer, transmembrane protein 164 (TMEM164) has been shown to activate autophagy, leading to the degradation of ferritin, GPX4, and lipid droplets. This process increases iron accumulation and lipid peroxidation, thereby promoting ferroptosis in cancer cells (Liu J. et al., 2023). Autophagy, therefore, plays a pivotal role in facilitating ferroptosis in cancer therapy. Interestingly, copper not only induces proteotoxic stress but also drives ferroptosis by directly binding to GPX4, resulting in the formation of GPX4 aggregates. These aggregates undergo autophagic degradation, further inducing ferroptosis (Xue et al., 2023b). Furthermore, in glioblastoma (GBM), inhibiting autophagy increases the sensitivity of GBM stem cells to temozolomide treatment by inducing ferroptosis (Buccarelli et al., 2018). Conversely, in hepatocellular carcinoma, autophagy driven by PNO1, a known ferroptosis inhibitor, enhances glutamate synthesis, activating system Xc− and ultimately preventing ferroptosis (Hu X. et al., 2022). In non-small cell lung cancer, curcumin induces ferroptosis through the activation of autophagy (Tang X. et al., 2021). These studies suggest that autophagy can either promote or suppress ferroptosis depending on the cancer type, which may be influenced by factors such as tumor heterogeneity or varying cellular environments. Further research is needed to clarify these interactions. Therapeutically, simultaneously targeting both ferroptosis and autophagy pathways has emerged as a promising strategy to overcome treatment resistance in breast cancer. Combination therapies incorporating ferroptosis inducers and autophagy modulators can potentially improve treatment effectiveness.
2.4.2 Autophagy and cuproptosis
Disturbance of copper homeostasis modulates ferroptosis and cuproptosis and initiates autophagy pathways (Li Y. et al., 2021; Xue et al., 2023a; Yang et al., 2021). The intracellular accumulation of copper activates transcription factor EB (TFEB), upregulates ATG5, sequestosome 1 (SQSTM1), and microtubule-associated protein 1 light chain 3 (MAP1LC3) expression, and modulates the AMPK-mTOR pathway, thereby stimulating autophagy (Xue et al., 2023a; Yang et al., 2021). Inhibition of the copper transporter SLC31A1 in pancreatic cancer cells results in decreased intracellular copper concentrations and enhances autophagy (Yu et al., 2019). However, the interplay between cuproptosis and autophagy in breast cancer remains ambiguous, yet it is postulated that disruptions in copper homeostasis may promote autophagy. Further investigation into this crosstalk is warranted to elucidate the underlying mechanisms and implications for cancer pathogenesis and treatment strategies.
3 The prognostic significance of genes associated with ferroptosis and cuproptosis in breast cancer
Identifying prognostic biomarkers/models to predict cancer progression is critical for two reasons. First, these biomarkers/models have practical clinical applications in patient treatment. Second, studying these biomarkers will provide new insights into disease mechanisms and the molecular processes driving pathological behavior (Passaro et al., 2024). Prognostic models integrating ferroptosis and cuproptosis genes show great potential for improving breast cancer prognosis and treatment (Li J. et al., 2023a). Breast cancer demonstrates extensive heterogeneity at both inter- and intra-tumoral levels, driven by distinct genomic, epigenomic, transcriptomic, and proteomic variations that contribute to differential therapeutic responses and disease progression. Ferroptosis is particularly relevant in TNBC due to its increased sensitivity to ferroptosis induction, though quantification across subtypes remains challenging. Similarly, cuproptosis may impact tumor cells with elevated copper levels. This heterogeneity complicates treatment, underscoring the need for biomarker-based studies to optimize targeted therapeutic approaches. The gene signatures effectively predict patient outcomes and overall survival. Additionally, they correlate with the tumor immune microenvironment and response to immunotherapy, guiding personalized treatment strategies like combining immunotherapy with ferroptosis/cuproptosis inducers for better outcomes (Li et al., 2023b; Li J. et al., 2022; Xu L. et al., 2023). Table 1 provides an overview of the impact of ferroptosis and cuproptosis genes on breast cancer prognosis.
3.1 Ferroptosis-associated genes and the prognosis of breast cancer
Ferroptosis has emerged as a promising therapeutic avenue for treating breast cancer (Li Z. et al., 2020). Notably, research indicates that ferroptosis-related marker genes have the potential to serve as novel biomarkers for predicting the prognosis of breast cancer patients (Yang et al., 2021; Zhou L. et al., 2023). In particular, ferroptosis pathway status was significantly associated with clinical outcomes and intra-tumoral heterogeneity in breast cancer patients, as NDUFA13 expression was identified as a positive biomarker for activating the ferroptosis pathway in breast cancer patients (Li Y. et al., 2022). Thus, an in-depth exploration of the involvement of ferroptosis-related genes underscores their significance in determining breast cancer prognosis, overall survival, and guiding treatment approaches. Building on this foundation, a recent study introduced a novel prognostic model for breast cancer, that integrates four ferroptosis-related genes (CISD1, ALOX15, CARS1, and SLC7A11) (Wang L. et al., 2022). Furthermore, the model independently and accurately predicted overall survival in breast cancer patients. Significantly, a nomogram was created to provide precise prognostic predictions for breast cancer individuals (Wang L. et al., 2022). In a related study, researchers constructed an eight-gene model associated with ferroptosis to predict breast cancer patient’s prognosis. This model effectively categorized patients into high- or low-risk groups. The inclusion of these 8 genes (ALOX15, CHAC1, CISD1, CS, SLC7A11, EMC2, G6PD, and ACSF2) is highly valuable for prognostic prediction in breast cancer patients (Li H. et al., 2021).
Moreover, another novel prognostic model, composed of nine ferroptosis-related genes has demonstrated significant accuracy in predicting survival outcomes in breast cancer patients. These genes included PROM2, ANO6, FLT3, G6PD, IFNG, NGB, PIK3CA, SLC1A4, and TP63. Among them, five genes (ANO6, FLT3, G6PD, IFNG, and PIK3CA) were identified as drivers contributing to ferroptosis progression. Conversely, two genes (PROM2 and TP63) exhibit suppressor functions, while the remaining two genes (NGB and SLC1A4) serve as markers within the context of ferroptosis (Lu et al., 2022). Additionally, a model comprising nine ferroptosis-related genes (ALOX15, CISD1, CS, GCLC, GPX4, SLC7A11, EMC2, G6PD, and ACSF2) was employed in this study. It is believed that it holds promise as an innovative biomarker for predicting the prognosis of breast cancer patients (Wang D. et al., 2021). Expanding this line of inquiry, a survival prediction model was developed using eleven prognostic-related genes (TP63, IFNG, MT3, ANO6, FLT3, PTGS2, SLC1A4, JUN, SLC7A5, CHAC1, and TF) derived from differentially expressed genes (DEGs). The model exhibited strong predictive capacity in breast cancer patients (Li Q. et al., 2023).
In addition, a study developed a gene signature focusing on genetic diversity, consisting of protective genes (ALB, ANGPTL7, NGB, and IL6) and risk-related genes (BLOC1S5-TXNDC5). This diagnostic signature accurately predicts breast cancer risk levels. Researchers have validated its reliability and applicability through independent cohort analysis, PCR-based mRNA detection in clinical tissue samples, and Western blot analysis in BRCA cell lines (Wang N. et al., 2022). Another study established a ferroptosis activation-risk-related score model (FeAS) that included 13 genes, which were verified using machine learning and single-cell RNA sequencing data. The model showed promising prognostic capacity and could guide clinical treatment to prevent drug resistance, influencing breast cancer patient outcomes (Liu S. et al., 2023). A novel prediction signature comprising six genes (CARS1, CHAC1, FANCD2, AIFM2, G6PD, and HMOX1) was developed utilizing the least absolute shrinkage and selection operator (LASSO) Cox regression methodology. The expression levels of these six genes were subsequently validated through real-time quantitative polymerase chain reaction and immunohistochemistry assays using samples from the Human Protein Atlas. Notably, patients categorized into the high-risk group based on this signature demonstrated a greater propensity for relapse or metastasis. Furthermore, the risk score derived from this signature emerged as an independent prognostic factor for disease-free survival (Zhu J. et al., 2022). A study identified nine ferroptosis-related genes with prognostic value in breast cancer: BCL2, SUSD3, SERPINA3, AGBL2, SEC14L2, ELOVL2, FGD3, CASC1, and TPRG1. The prognostic model based on these genes showed that patients with high ferroptosis scores had significantly better overall survival (OS) than those with low ferroptosis scores. Additionally, the models demonstrated high reliability in predicting one-, three-, and five-year survival rates through time-dependent ROC curve analysis (Yin and Tang, 2022).
Continuing this trend, a prognostic prediction model consisting of 15 ferroptosis-related genes (IFNG, FH, MT1G, CISD1, GABARAPL1, SLC1A5, SLC2A12, SLC2A8, WIPI1, NRAS, BRD4, FADS2, DUOX1, HSF1, and TFAP2C) was established. The prognostic model exhibited high accuracy according to time-dependent ROC curve analysis, with AUCs of 0.948, 0.956, and 0.940 for the 1-, 3-, and 5-year intervals, respectively (Wu et al., 2022). The prognostic model offers a theoretical framework for precise prognosis prediction in clinical settings for triple-negative breast cancer (Wu et al., 2022). Significantly, another study identified MTHFD2 as a significant molecular biomarker for prognostic prediction and a novel therapeutic target in TNBC. Additionally, MTHFD2 was identified as a potential regulatory gene for ferroptosis in TNBC. In vitro experiments revealed that MTHFD2 knockdown inhibited proliferation, induced apoptosis, and suppressed migration and invasion in TNBC cells (Zhang H. et al., 2023). Furthermore, a novel predictive signature comprising three ferroptosis-related genes, namely TFR2, ZNP36, and RGS4, was developed to predict prognostic outcomes in TNBC cell lines (Yang et al., 2024a). Notably, the study documents the negative regulatory function of TFR2 in TNBC ferroptosis, with TFR2 downregulation leading to inhibited proliferation and ferroptosis induction in TNBC (Yang et al., 2024a). Lastly, a breakthrough documented for the first time that short-term treatment with endocrine agents can sensitize ER+ breast cancer cells to ferroptosis-inducing agents, suggesting a sensitization mechanism independent of genetic mutations. Building on this concept, researchers developed a 55-gene signature, referred to as the FERscore (Hu et al., 2024), specifically designed to predict the susceptibility of breast cancer to ferroptosis. Patients with breast cancer who had lower FERscores were associated with significantly improved survival outcomes. Data from both cell lines and primary tumor samples revealed that ER+ breast cancer typically exhibited lower FERscores compared to other subtypes. However, in endocrine-resistant ER+ tumor cells and residual tumors following endocrine therapy, the FERscore was markedly elevated. In breast cancer, higher FERscore levels were positively associated with traits such as mesenchymal phenotype, stemness, immune cell infiltration, and cancer-associated fibroblast (CAF) enrichment, while they were negatively associated with features like estrogen response and DNA repair capacity (Hu et al., 2024).
3.2 Cuproptosis-associated genes and prognosis of breast cancer
Cuproptosis can be regulated by specific genes known as cuproptosis-related regulators (CRRs), which include DLD, PDHB, ATP7B, ATP7A, DLAT, DLST, SLC31A1, DBT, FDX1, LIPT1, LIAS, GCSH, and PDHA1 (Tsvetkov et al., 2022). Expanding on this, CRRs could enhance our understanding of cuproptosis in diseases, including breast cancer. Growing evidence indicates that cell death pattern signatures significantly predict prognosis, the tumor immune microenvironment (TIME), and immunotherapy response in breast cancer patients (Li Z. et al., 2022). Recently, signatures related to ferroptosis (Zhu L. et al., 2021), pyroptosis (Xu L et al., 2022), and necroptosis have been identified. The role of cuproptosis in breast cancer remains underexplored, necessitating further investigation into genetic changes in CRGs to uncover therapeutic opportunities. In line with this, a novel breast cancer signature consisting of CRGs such as PGK1, SLC52A2, and RAD23B has shown potential for prognosis prediction, with RAD23B emerging as a promising target linked to disease progression and drug resistance (Song et al., 2022).
Building on these findings, ten genes responsible for copper-induced cell death through genome-wide CRISPR-Cas9 loss-of-function screens and individual gene knockout studies. Seven of these genes (FDX1, LIAS, LIPT1, DLD, DLAT, PDHA1, and PDHB) promote cuproptosis, while the other three (MTF1, GLS, and CDKN2A) inhibit cuproptosis. Patients with luminal A and basal subtypes were classified into cluster1 and cluster2, respectively. The basal subtype is significantly associated with the worst prognosis in patients with breast cancer, whereas the luminal A subtype is linked to the best clinical outcomes. Furthermore, the cluster expression patterns of cuproptosis-related genes (CRGs) differed. Cluster1 exhibited increased expression of most cuproptosis-promoting genes (LIPT1, LIAS, PDHB, FDX1, DLAT, and DLD), while cluster2 exhibited increased expression of cuproptosis-inhibiting genes (CDKN2A and GLS) and one cuproptosis-promoting gene (PDHA1). This suggests that cuproptosis may inhibit breast cancer progression by inducing tumor cell death (Li W. et al., 2022). Additional studies have developed a cuproptosis-related signature with six genes (MTF1, DKN2A, PDHA1, DLD, LIPT1, FDX1) for breast cancer, which accurately predicted the OS rate (Jiang B et al., 2022). Another study underscored the ability of the SLC7A5, STC2, MAPT, TFF1, CHAD, GREB1, SCUBE2, SUSD3, MMP7, CHI3L1, and FABP7 genes to predict the overall survival rate of breast cancer patients (Zheng et al., 2023). In parallel, a novel prognostic risk signature for breast cancer patients was constructed using 11 cuproptosis hub genes namely, PGK1, MRPL39, COPB2, HSPH1, NFKBIA, PRDX1, PCMT1, MPZL3, DLG3, DIP2B, LACTB2 (Shen L. et al., 2022).
Recently, high SLC31A1 expression in breast cancer patients has led to poor overall survival, distant metastasis-free survival, and relapse-free survival (RFS), suggesting that SLC31A1 may be an unfavorable prognostic biomarker (Li X. et al., 2022). Moreover, elevated SLC31A1 expression in breast cancer samples indicates poor prognosis, shorter overall survival, and a dysregulated immune response. Low levels predict sensitivity to CTLA4 inhibitors but inadequate response to paclitaxel (Li L. et al., 2022). Researchers developed a nomogram model utilizing cuproptosis-related genes (NLRP3, LIPT1, PDHA1, and DLST) and discovered that the signature derived from these genes effectively stratifies patient subtypes and correlates closely with the TME. Additionally, these genes were identified as independent prognostic indicators for breast cancer patients (Zhou et al., 2022). In addition, elevated PDHA1 expression correlated with poorer outcomes in breast cancer patients. Furthermore, immune infiltration analysis of CRGs revealed that PDHA1 expression is significantly associated with the infiltration levels of CD4+ memory T cells, M0 and M1 macrophages, and mast cells in breast cancer.
PDHA1 has been reported to be an independent prognostic biomarker and a potential target for breast cancer immunotherapy (Huang et al., 2022). A study identified a novel prognostic model comprising four CRGs (ATOX1, DLAT, SLC31A2, and SLC25A3) for HER-2-positive breast cancer patients. Among HER-2-positive breast cancer patients, DLAT was confirmed to be downregulated and correlated with improved survival. Elevated DLAT expression was associated with resistance to HER-2-targeted therapy and sensitivity to immunotherapy (Sha R. et al., 2024). In this case-control study, high expression of LIAS, LIPT1, and ATP7B, along with low CDKN2A expression, was linked to improved invasive disease-free survival (iDFS). In the cohort study, high expression of LIAS, FDX1, LIPT1, DLD, PDH1, and ATP7B, coupled with low CDKN2A expression, was associated with favorable RFS in patients with estrogen receptor-positive early breast cancer (ER+ EBC). The developed prognostic nomogram model exhibited predictive solid capability for the 7-year RFS of ER+ EBC patients (Fan Y. et al., 2023). In addition, researchers developed an 11-gene risk model for TNBC treatment, targeting 11 key CRGs (NFE2L2, NLRP3, ATP7A, ATP7B, SLC31A1, LIAS, LIPT1, GLS, DLAT, PDHB, DLST). The model predicts 5–15-year survival with an AUC of 0.836 (Shi et al., 2023). Another six-gene risk model, including PTPRN2, SCARB1, SLC37A2, YES1, LY6D, and NOTCH3, has been proven effective and reliable in predicting the prognosis of triple-negative breast cancer patients. The accuracy of the risk model in predicting TNBC prognosis was enhanced by establishing a nomogram that outperformed the TNM staging system (Zhu B. et al., 2022). The findings revealed that CRGs may impact tumor immunity in TNBC, clinical features, and prognosis, making them valuable tools for patient prognosis prediction (Sha et al., 2022; Zhu B. et al., 2022).
4 The landscape of ferroptosis/cuproptosis-related lncRNA in the prognosis of breast cancer
Although approximately 75% of the genome is transcribed into RNA, only 3% is translated into mRNA, which encodes proteins. Most of the transcriptome consists of noncoding RNAs (ncRNAs) lacking protein-coding potential. These ncRNAs may be classified based on their length, structure, and origin, with the four major types relevant to breast cancer being microRNAs (miRNAs), circular RNAs (circRNAs), long non-coding RNAs (lncRNAs), and tRNA-derived small RNAs (tsRNAs). Each ncRNA type plays a distinct role in gene regulation and cancer biology.
Small non-coding RNAs (sncRNAs), including miRNAs, circRNAs, and tsRNAs, are generally shorter than 200 nucleotides. Despite their small size and comprising less than 1% of the human transcriptome, sncRNAs are critical regulators of gene expression and various cellular processes (Klimenko, 2017; Smal et al., 2024). MiRNAs function primarily by binding to target mRNAs, leading to their degradation or translational repression. CircRNAs, on the other hand, could act as miRNA sponges, modulating gene expression indirectly by sequestering miRNAs. TsRNAs, derived from tRNAs, have emerging roles in controlling translation and responding to cellular stress. The biogenesis of sncRNAs involves highly regulated processing pathways, such as the enzymatic cleavage of precursor miRNAs by Drosha and Dicer (Pagès et al., 2018; Russell et al., 2024).
In contrast, long non-coding RNAs (lncRNAs), which are over 200 nucleotides in length, are involved in a wider array of regulatory functions. These include chromatin remodeling, transcriptional regulation, and serving as molecular scaffolds (Li D. et al., 2022; Mattick et al., 2023). LncRNAs associated with ferroptosis and cuproptosis in breast cancer have shown promise as biomarkers for early detection, prognostic modeling, and therapeutic targeting. While miRNAs, circRNAs, and tsRNAs are also crucial in cancer biology, this manuscript primarily focuses on lncRNAs due to their significant involvement in regulating ferroptosis and cuproptosis pathways in breast cancer. Table 2 provides a detailed overview of how lncRNAs related to these processes influence breast cancer prognosis.
To provide a comprehensive understanding, we briefly discuss the broader sncRNA landscape, recognizing the significant roles of miRNAs, circRNAs, and tsRNAs in cancer progression. However, the central focus remains on lncRNAs, given their pivotal role in the regulation of ferroptosis and cuproptosis in breast cancer.
4.1 Ferroptosis associated lncRNA in the prognosis of breast cancer
Recent research has identified eleven long non-coding RNAs (lncRNAs) associated with ferroptosis as potential prognostic indicators in breast cancer patients. Specifically, Kaplan-Meier analysis indicated that high-risk lncRNA signatures are linked to poorer outcomes. The AUC for these lncRNA signatures was 0.682, confirming their predictive accuracy for breast cancer prognosis. Among these, the lncRNAs identified as independent prognostic markers include KLHDC7B-DT, AC012213.3, LIPE-AS1, SIDT1-AS1, AC009171.2, AC137630.3, HSD11B1-AS1, LINC02446, TFAP2A-AS1, AC079298.3, YTHDF3-AS1 (Jia et al., 2021). Another study pinpointed seven ferroptosis- and immune-related differentially expressed lncRNAs (FI-DELs) namely AL035661.1, ADAMTS9-AS1, AC078883.1, FTX, AC007686.3, CBR3-AS1, TMEM105, that are significantly associated with overall survival in patients with breast infiltrating ductal and lobular carcinoma. The model had an AUC exceeding 0.6 across the training, validation, and cohorts. Furthermore, the predictive model exhibited high sensitivity (87.84%) and specificity (97.06%), underscoring its potential clinical utility (Wei et al., 2022). Moreover, a prognostic signature comprising eight ferroptosis-related lncRNAs (AL133467.1, LINC01235, AC072039.2, USP30-AS1, AC108474.1, MAPT-AS1, TDRKH-AS1, LIPE-AS1) was developed using multivariate Cox regression analysis. The predictive accuracy of this signature was validated via receiver operating characteristic (ROC) curve analysis. The area under the time-dependent ROC curve (AUC) in the training cohort was 0.853 at 1 year, 0.802 at 2 years, and 0.740 at 5 years. In the validation cohort, the AUC values were 0.791 at 1 year, 0.778 at 2 years, and 0.722 at 5 years (Zhang K. et al., 2021).
In addition to these findings, 12-FRLncRNA signature, consisting of LINC01871, LINC00393, AC121247.2, LINC02384, LIPE-AS1, HSD11B1-AS1, AC010655.2, LINC01419, PTPRD-AS1, AC099329.2, OTUD6B-AS1, and LINC02266, has been shown to predict the prognosis of breast cancer patients accurately. Research suggests this ferroptosis-related prognostic signature could be a novel biomarker for forecasting breast cancer outcomes (Xu Z. et al., 2021). Furthermore, another study identified four ferroptosis-related differentially expressed lncRNAs (FR-DELs), namely LINC01152, AC004585.1, MAPT-IT1, and AC026401.3—that are correlated with overall survival in patients with breast cancer. The AUC of the prognostic model using these four biomarkers exceeded 0.60 in all three groups. The predictive model demonstrated a sensitivity of 86.89% and a specificity of 86.73% using these biomarkers (Yao et al., 2022). Moreover, another ferroptosis-related lncRNA risk model demonstrated considerable clinical significance in predicting breast cancer prognosis and response to immunotherapy. The constructed signature could also be used to assess the immune landscape of breast cancer patients. Notably, low-risk patients exhibited enrichment of immune-related pathways and increased infiltration of various immune cell types. CYTOR, LMNTD2-AS1, LYPLAL1-AS1, USP30-AS1, RHPN1, LINC01655, AP005131.2, AC004988.1, and AC079289.3 were upregulated in a breast cancer cell line (SKBR3) compared to a normal human breast epithelial cell line (MCF10A). Conversely, HSD11B1-AS1 was downregulated in breast cancer cell lines (MCF7, SKBR3, and MDA-MB-231). Researchers have expanded upon this model to develop a hybrid nomogram capable of predicting 1-year, 3-year, and 5-year OS rates (Shen S. et al., 2022).
In addition, five lncRNAs (LINC01235, LINC02166, AL133467.1, TGFB2-AS1, and LINC02266) were found to be associated with ferroptosis, with moderate accuracy in predicting recurrence-free survival. These lncRNAs were identified as independent predictor factors, forming a nomogram for clinical RFS (Wang Y. et al., 2022). Finally, high levels of PTPRG-AS1 were detected in TNBC patients. Significantly, POU2F2 was identified as a transcriptional activator of PTPRG-AS1, which in turn regulated ferroptosis and cell proliferation in TNBC through the miR-376c-3p/SLC7A11 signaling pathway. Consequently, the POU2F2/PTPRG-AS1/miR-376c-3p/SLC7A11 axis holds potential as both a novel biomarker and therapeutic target for ferroptosis-mediated cancer therapy in TNBC (Li J. et al., 2024).
4.2 Cuproptosis associated lncRNAs in the prognosis of breast cancer
Long noncoding RNAs (lncRNAs) are closely associated with the accumulation of copper ions (Scheurer et al., 2022). Notably, lncRNAs related to cuproptosis have been identified as prognostic markers for sarcoma, gastric cancer, and renal cell carcinoma (Feng A. et al., 2022; Xu S. et al., 2022; Yang M. et al., 2022). Additionally, lncRNAs are pivotal in modulating the biological processes involved in breast cancer (Jiang Z. R. et al., 2022; Pardini and Dragomir, 2021). To further illustrate, researchers have constructed prognostic biomarker/signature models that can independently predict the prognosis of breast cancer patients and estimate OS and treatment outcomes. Specifically, researchers constructed a risk model consisting of 11 cuproptosis-related lncRNAs: GORAB-AS1, AC079922.2, AL589765.4, AC005696.4, CYTOR, ZNF197-AS1, AC002398.1, AL451085.3, YTHDF3-AS1, AC008771.1, and LINC02446. The AUC values for the receiver operating characteristic (ROC) curves at 1, 3, and 5 years were 0.849, 0.779, and 0.794, respectively. Moreover, high-risk patients exhibit high sensitivity to anti-CD276 immunotherapy and conventional chemotherapeutic drugs such as imatinib, lapatinib, and pazopanib (Jiang Z. R. et al., 2022). Another recent study explored the cuproptosis-related prognostic 2-lncRNAs (USP2- AS1, NIFK-AS1) signature (BCCuS) in breast cancer and validated it as an independent prognostic factor for breast cancer (Xu Q. T. et al., 2022). Interestingly, USP2-AS1 exhibited a positive correlation with four genes (DLAT, PDHA1, FDX1, and DLD) and a negative correlation with two genes (LIAS and PDHB). Conversely, NIFK-AS1 showed a positive correlation with three genes (LIAS, LIPT1, and PDHB) and a negative correlation with two genes (DLD and DLAT) (Xu Q. T. et al., 2022).
Ten cuproptosis-related lncRNAs have been identified as potential biomarkers for predicting the survival prognosis of breast cancer. These lncRNAs included AL118556.1, AL451123.1, MFF-DT, AL133243.2, ZKSCAN7-AS1, AC012676.3, AC009506.1, AC079766.1, MIR1915HG, and AC138028.2, which are significantly associated with OS. The study showed that the expressions of MFF-DT, AL133243.2, MIR1915HG, ZKSCAN7-AS1, and AC009506.1 were upregulated in breast cancer tissues, while AL118556.1, AL451123.1, and AC138028.2 were downregulated. Furthermore, increased expression levels of MFF-DT, AL133243.2, MIR1915HG, and ZKSCAN7-AS1, along with decreased expression levels of AL118556.1 and AC138028.2, were also observed in breast cancer cell lines. Thus, MFF-DT, AL133243.2, and MIR1915HG are anticipated to be promising prognostic markers for breast cancer (Pan et al., 2023).
In addition, nine cuproptosis-associated lncRNAs were identified, and a lncRNA–mRNA co-expression network was established. Among these, ARHGAP28-AS1, LINC01711, LRRC8C-DT, PCAT18, and SIAH2-AS1 were found to be protective lncRNAs for patients with breast cancer. In contrast, TDRKH-AS1, SAMMSON, WDFY3-AS2, and LINC00393 were identified as risk factors. This study provides a foundation for exploring predictive biomarkers in breast cancer patients and contributes to a better understanding of the biological mechanisms involving cuproptosis-related lncRNAs (Guo et al., 2022). Furthermore, researchers identified six cuproptosis-related lncRNAs that could regulate breast cancer cell proliferation and metastasis. High-risk patients have poorer survival rates and lower sensitivity to chemotherapy, endocrine therapy, and radiation therapy. Low-risk patients exhibited reduced expression of biomarkers associated with resistance to CDK4/6 inhibitors (CCNE1, E2F1, E2F2) and PARP inhibitors (BRCA1/BRCA2), suggesting an enhanced potential for response to PARP and CDK4/6 inhibitor therapies. The plate colony formation assay showed decreased colony formation in MCF-7 cells after silencing YTHDF3-AS1, LINC00839, and OTUD6B-AS1 and increased colony formation after silencing NIFK-AS1 and TOLLIP-AS1. Similar results were observed in the CCK-8 kit assay, indicating the importance of YTHDF3-AS1, LINC00839, and OTUD6B-AS1 in promoting breast cancer cell proliferation. Knocking down YTHDF3-AS1, LINC00839, and OTUD6B-AS1 decreased MCF-7 cell invasiveness while silencing NIFK-AS1, TP53TG1, and TOLLIP-AS1 increased invasiveness. Wound healing assays yielded consistent results, suggesting that carcinogenesis-related lncRNAs play a significant role in breast cancer metastasis (Wu X. et al., 2023).
Ten long noncoding RNAs (lncRNAs), termed CuImP-LncRNAs, were identified as being associated with both cuproptosis and immune responses. These included AL139241.1, MFF-DT, AL451123.1, AC009120.5, AL137847.1, HECW2-AS1, LINC01031, NIFK-AS1, AL592301.1, and U73166.1 (Li Y. et al., 2023). Furthermore, the findings provide a novel predictive model for breast cancer prognosis, aiding in the optimization of individualized therapy for patients. This model not only offers accuracy but also opens avenues for alternative treatment approaches. In addition, this study lays the groundwork for further research into cuproptosis-related ncRNAs in breast cancer, facilitating the development of new biomarkers and therapeutic targets for this disease. Moreover, a recent study investigated nine lncRNAs related to cuproptosis (Li F. et al., 2024). Specifically, the study analyzed immune function, tumor mutation burden, and tumor immune dysfunction and exclusion differences among patients with varying risk scores, resulting in the construction of a prognostic model for breast cancer prediction. The AUC values of this model at 1 year, 3 years, and 10 years were 0.783, 0.728, and 0.795, respectively. Notably, these values surpassed those of other models, indicating its superior predictive performance (Wang F. et al., 2020; Zhang D. et al., 2020).
5 Potential interplay and clinical association between F/CRGs
A recent study identified potential interconnections between cuproptosis and ferroptosis regulators (Shen Y. et al., 2022). To validate the regulation between cuproptosis and ferroptosis regulators, the expression of cuproptosis regulators was analyzed by knocking down several ferroptosis regulators. In the GSE120472 cohort, knockout of PTEN in primary mouse embryonic fibroblasts (MEFs) resulted in the upregulation of 3 cuproptosis regulators, including DBT, SLC31A1, and ATP7A. In the GSE184356 cohort, the downregulation of TFAM led to a significant change in PDHA1, PDHB, ATP7A, and ATP7B in human dermal fibroblasts. In the GSE145548 cohort, the downregulation of ATF2 in MCF7 breast cancer cells resulted in substantial changes in the expression of cuproptosis regulators (DLST, GCSH, PDHA1, LIPT1, and DLD) (Shen Y. et al., 2022). This study validated the correlation between cuproptosis and ferroptosis regulators by transfecting siRNAs and shRNA into A549 cells. The findings further revealed that SLC31A1 expression was upregulated following PTEN downregulation, ATP7A expression increased after TFAM downregulation, and LIPT1 expression was inhibited following ATF2 downregulation. Thus, the results indicated the cross-talk and biological regulation between cuproptosis and ferroptosis regulators in cancers, including breast cancer. Moreover, a study devised a novel approach to construct a signature model comprising eleven cuproptosis-related ferroptosis genes (Zhang and Zhang, 2023). This signature included G6PD, GPX4, PANX1, PIK3CA, CHAC1, SOCS1, CHMP6, ANO6, CS, SLC7A5, and EMC2, encompassing both ferroptosis driver genes (ANO6, CHAC1, CS, EMC2, G6PD, PANX1, and PIK3CA) and ferroptosis suppressor genes (CHMP6, GPX4, and SOCS1). The identified cuproptosis-related FRG signature shows potential as a novel prognostic biomarker for predicting overall survival in breast cancer patients (Zhang and Zhang, 2023).
Moreover, another recent study revealed that the correlation between immune cell infiltration and patient outcomes in breast cancer is associated with genes linked to cuproptosis and ferroptosis (Li Y. et al., 2023). An innovative approach was employed to establish a predictive model for breast cancer patients by integrating ferroptosis and cuproptosis genes. The selected genes included TRIM45, KNOP1, HOXC10, SGPP1, and ANKRD52. The study confirmed their varied expression patterns in tumor and non-tumor biological tissue samples, both at the cellular and tissue levels. Subsequently, the relationship between these genes and tumor staging, cellular infiltration, and clinical indicators was analyzed (Li et al., 2023a). The proposed scoring model demonstrates the potential for guiding clinical decisions and tailoring treatment approaches for breast cancer patients, offering insights into selecting antitumor drugs for breast cancer treatment. As reported, a high ACSL4/low GPX4 profile holds significant practical value in predicting pathological complete response to neoadjuvant chemotherapy in patients with breast cancer (Sha et al., 2021). Future research should focus on unraveling the complex molecular mechanisms underlying the interplay between cuproptosis and ferroptosis in breast cancer. Efforts should also prioritize exploring and refining the identified signature models by incorporating additional genetic and epigenetic factors. Such advancements aim to enhance the predictive accuracy of these models, particularly in forecasting treatment responses and facilitating the personalization of therapeutic strategies (Figure 6).
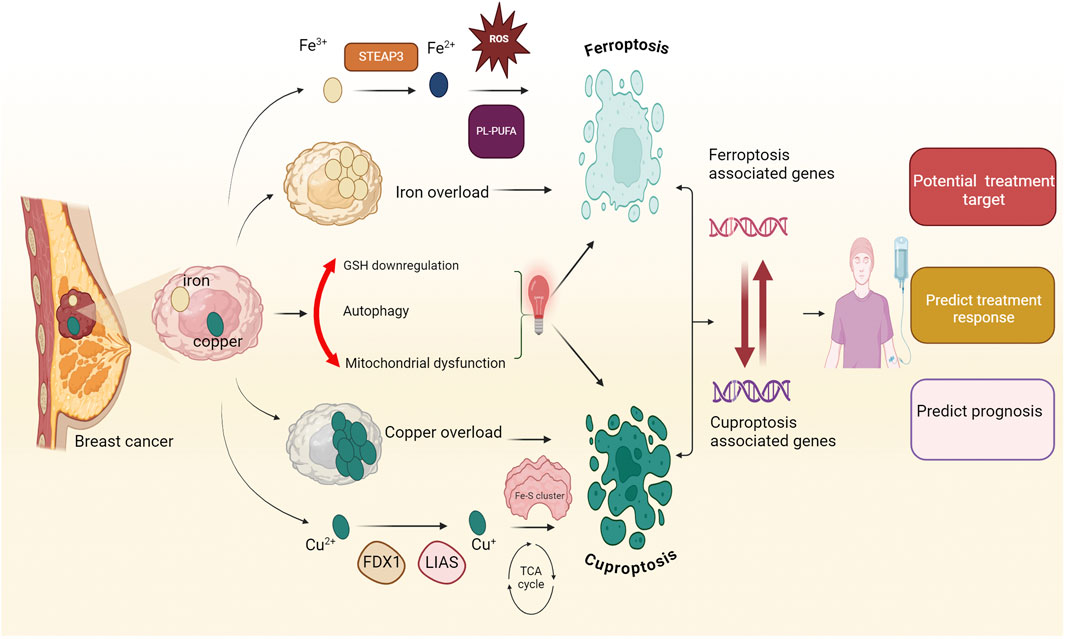
Figure 6. Association between ferroptosis and cuproptosis. Ferroptosis and cuproptosis are distinct forms of regulated cell death induced by iron and copper overload. Ferroptosis is characterized by the accumulation of iron-dependent lipid peroxides, leading to oxidative damage and cell death. In contrast, cuproptosis is marked by mitochondrial dysfunction and proteotoxic stress from excessive intracellular copper. The intersecting pathways of ferroptosis and cuproptosis in breast cancer highlight a complex relationship involving metal homeostasis, mitochondrial dysfunction, glutathione metabolism, and autophagy. The prognostic significance of ferroptosis and cuproptosis gene signatures in breast cancer is substantial, suggesting their potential utility in predicting survival rates, modulating treatment strategies, and addressing drug resistance challenges. The intricate interplay between ferroptosis and cuproptosis gene signatures has profound implications for both the prognosis and treatment of breast cancer. The red bidirectional arrow demonstrates an innovative linkage between ferroptosis and cuproptosis genes. The identification of this linkage suggests avenues for further investigation into the synergistic effects and potential therapeutic implications of targeting both ferroptosis and the cuproptosis pathway in breast cancer. Fe-S cluster, iron-Sulfur cluster; Fe3+, ferric ion; Fe2+, ferrous ion; Cu2+, cupric ion; Cu+, cuprous ion; STEAP3, six-Transmembrane Epithelial Antigen of the Prostate 3; ROS, reactive Oxygen Species; PL-PUFA, phospholipid Polyunsaturated Fatty Acid; TCA cycle, tricarboxylic Acid cycle.
5.1 Ferroptosis in the clinical settings of breast cancer
Ferroptosis has become a pivotal focus in cancer research due to its association with intracellular iron accumulation and disruptions in iron, lipid, and amino acid metabolism. Compounds such as erastin, RSL3, and ferrostatin-1 have been identified as key regulators of ferroptosis; however, the detailed mechanisms governing its role in cancer progression remain elusive, necessitating further investigation to unlock its therapeutic potential (Huang et al., 2024). Emerging evidence suggests that ferroptosis significantly influences breast cancer tumorigenesis, progression, invasion, and drug resistance (Ge et al., 2024). Inducing ferroptosis in these cells inhibits tumor growth, offering a promising strategy for developing new treatments and overcoming drug resistance.
5.1.1 Conventional therapies and ferroptosis in breast cancer
Over the past decade, research has linked ferroptosis to chemotherapy resistance in cancer, highlighting its potential as a therapeutic strategy. Key regulators of ferroptosis, including proteins and enzymes involved in iron metabolism, lipid peroxidation, and the system Xc–pathway, play critical roles in modulating chemotherapy resistance. In TNBC, anthracyclines often demonstrate limited efficacy due to chemotherapy resistance and notable side effects. Studies suggest that inducing ferroptosis may enhance the sensitivity of TNBC cells to these agents, providing a promising approach to improve therapeutic outcomes (Luo et al., 2022; Zhao J. et al., 2023). Recent advancements in research have identified several chemotherapeutic agents that can induce ferroptosis as a mechanism for their anti-cancer effects. Notable examples include cisplatin, temozolomide, orlistat, and sorafenib. These drugs exploit the ferroptotic pathway to enhance their efficacy against various cancer types, presenting a novel strategy for improving treatment outcomes (Mou et al., 2019; Yin et al., 2022). Anomanolide C has been shown to inhibit the progression and metastasis of TNBC by enhancing the ubiquitination of the GPX4 protein, leading to autophagy-dependent ferroptosis (Chen Y-M. et al., 2023). This research highlights the potential of targeting ferroptosis as a strategy to sensitize tumors to chemotherapy in future cancer therapies. Saponin formosanin C (FC), a potent ferroptosis inducer from Paris formosana, enhances ferritinophagy and chemosensitivity to cisplatin in TNBC cells (Chen H-C. et al., 2022), while gallium maltolate (GaM) demonstrates synergistic anti-tumor effects with cisplatin, leading to TNBC cell death by impeding cell cycle progression and proliferation (Chen H-C. et al., 2023). Propofol, traditionally an anesthetic, has been shown to inhibit TNBC cell proliferation and potentiate doxorubicin and paclitaxel’s effects through the p53-SLC7A11-GPX4 pathway (Sun C. et al., 2022; Xu et al., 2020). Additionally, SOCS1, a new target for TNBC treatment, regulates cisplatin resistance and tumor progression (Wang et al., 2023d). A recent study provides new evidence that doxorubicin (DOX) exerts its anti-cancer effects, in part, by inducing ferroptosis. A critical role for DnaJ heat shock protein family (Hsp40) member C12 (DNAJC12) in breast cancer chemotherapy resistance was identified, as DNAJC12 was shown to suppress both DOX-induced ferroptosis and apoptosis, contributing to treatment resistance. Moreover, the DNAJC12/HSP70/AKT signaling axis appears to mediate this resistance by inhibiting these cell death pathways. Additionally, the findings further support the potential of AKT inhibitors or HSP70 inhibitors in reversing this resistance mechanism. This may offer a new perspective on overcoming DOX resistance in estrogen receptor-positive (ER+) breast cancer or other molecular subtypes with high DNAJC12 expression. These results suggest that targeting this axis may enhance the efficacy of DOX by restoring sensitivity to ferroptosis and apoptosis (Hu et al., 2024). Leveraging ferroptosis to enhance the efficacy of adjuvant chemotherapies and counteract chemotherapy resistance offers a promising therapeutic approach for treating breast cancer.
Numerous studies indicate that radiotherapy can exert anticancer effects by inducing ferroptosis. Ionizing radiation (IR) initiates cell death through several mechanisms, primarily by generating ROS and upregulating ACSL4, which leads to lipid peroxidation and triggers ferroptosis (Lei G. et al., 2020). A nanomedicine, BZAMH (Zeng et al., 2023), based on a metal-organic framework and combining high-Z element radio-sensitization, induces both ferroptosis and apoptosis in TNBC by disrupting GSH production with L-buthionine-sulfoximine (BSO) and promoting the Fenton reaction with supplied ferrous ions (Zeng et al., 2023). Additionally, iron-saturated lactoferrin (Holo-Lf) enhances ROS generation, damages DNA, and alleviates hypoxia in TNBC cells, improving radiosensitivity through disrupted iron metabolism and promoting ferroptosis (Zhang Z et al., 2021).
The TME has an immunosuppressive effect and lipid metabolism, a key feature of the TME, is closely linked to ferroptosis through lipid peroxidation (Mao et al., 2021; Xia et al., 2021). This intricate crosstalk between ferroptosis and the TME plays a crucial role in determining immunotherapy efficacy or resistance (Tang D. et al., 2021; Wang et al., 2021). Numerous studies demonstrate that ferroptosis significantly influences the immune response by affecting the activity of immune cells (Kim et al., 2022; Liao et al., 2022). In the TME, different immune cell subtypes, such as T cells, B cells, granulocytes, and monocytes, may experience spontaneous ferroptosis, which in turn impacts the overall immune response (Xu S. et al., 2021). In recent years, immunotherapy has emerged as a promising approach for TNBC treatment, with studies indicating its potential to induce ferroptosis. In TNBC, the LAR subtype, which expresses the androgen receptor, demonstrates increased levels of oxidized phosphatidylethanolamine and alterations in glutathione metabolism, especially involving GPX4. Notably, the inhibition of GPX4 can trigger ferroptosis in this subtype (Yang F. et al., 2023). Research has shown that inhibiting GPX4 not only triggers ferroptosis in tumors but also boosts anti-tumor immunity. The combination of a GPX4 inhibitor with anti-PD1 therapy is more effective than monotherapy (Fan Y et al., 2023). Furthermore, protein arginine methyltransferase 5 (PRMT5) plays a crucial role in regulating ferroptosis, as it methylates and stabilizes Kelch-like ECH-associated protein 1 (KEAP1), a ubiquitinating enzyme that blocks the NRF2/HO-1 pathway, increasing TNBC resistance to both ferroptosis and immunotherapy (Wang et al., 2023f). This highlights PRMT5 as a potential therapeutic target, suggesting that combining immunotherapy with PRMT5 inhibitors could enhance treatment effectiveness. Additionally, a newly designed small-molecule photosensitizer, Ir(III) photosensitizer (IrFc1) (Ling et al., 2022), coupled with transferrin and ferrocene, can promote ferroptosis in TNBC cells through a self-amplifying mechanism, leading to lipid oxidation and immunogenic cell death upon irradiation. TNBC cells exhibit higher tumor-infiltrating lymphocytes (TILs) and PD-L1 levels compared to other breast cancer subtypes, and PD-L1 inhibitors like atezolizumab and pembrolizumab have demonstrated efficacy in slowing disease progression in advanced metastatic TNBC (Adams et al., 2020; Jiang et al., 2021; Zhang X. et al., 2022). However, a limited number of patients respond effectively, primarily due to drug resistance and the absence of reliable biomarkers for patient stratification. Moreover, research by Zhou et al. has identified TYRO3 as a contributor to anti-PD-L1 resistance through the inhibition of ferroptosis via the AKT/NRF2 axis, suggesting that targeting TYRO3 with receptor tyrosine kinase inhibitors to restore ferroptosis may help overcome resistance and improve survival in aggressive TNBC cases (Jiang et al., 2021). Shao and colleagues explored the heterogeneity of ferroptosis phenotypes in different TNBC subtypes. They found increased iron metabolism in the MES subtype and highlighted ferroptosis-related pathways in the LAR subtype, while the BLIS and IM subtypes showed no distinct ferroptosis characteristics. GPX4 production and ferroptosis inhibition primarily occur through androgen receptor (AR) signaling. Thus, combining GPX4 inhibitors with immune checkpoint inhibitors may offer a novel treatment strategy for tumors resembling the LAR subtype (Jiang L. et al., 2023).
5.1.2 Nanotherapeutics and ferroptosis in breast cancer
Utilizing nanoparticles characterized by their small size and low toxicity provides a solution, as these particles can effectively carry ferroptosis inducers (FINs). More importantly, nanoparticles can be engineered for targeted drug delivery to tumor cells, thereby minimizing the detrimental effects on healthy cells. Employing nanoparticle-loaded FINs for targeted transport could significantly enhance the efficacy of cancer treatments (Qi X. et al., 2022). Recent research has focused on inducing ferroptosis in breast cancer through various innovative drug delivery systems. Notable advancements include folate-labeled exosomes that enhance ferroptosis by depleting glutathione and increasing ROS in MDA-MB-231 cells. Other studies have developed nanocomposites and carrier-free nano drugs that combine ferroptosis inducers with autophagy promoters, demonstrating potent anticancer effects. These approaches highlight the potential of targeted therapies to improve breast cancer treatment outcomes (Li Z. et al., 2020).
A self-assembled nanosystem comprising drug-organic-inorganic components (DFTA) demonstrates effective inhibition of ER+ breast cancer progression. This system utilizes DOX as a chemotherapeutic agent, ferric chloride (FeCl₃) as a ferroptosis inducer, and tannic acid (TA) to activate superoxide dismutase (SOD). This combination triggers a cascade reaction that generates ROS and significantly depletes GSH levels. Furthermore, integrating photothermal therapy (PT) enhances ROS production efficiency, paving the way for a synergistic approach combining chemotherapy, PT, and ferroptosis in the treatment of ER+ breast cancer (Xiong et al., 2019). Yttrium oxide nanoparticles (Y2O3-NPs) selectively induce cytotoxicity in MDA-MB-231 cells by generating oxidative stress, elevating ROS levels, causing DNA damage, and triggering apoptosis and ferroptosis through upregulation of CASP3, CASP8, and HO-1, and downregulation of BCL2 (Emad et al., 2023). The BSOandOXA@MOF-LR formulation exhibits strong tumor-suppressive properties and significantly enhances survival rates in 4T1 tumor xenograft mice. This effect is attributed to the combined action of amplified ferroptosis and the elimination of GSH, which sensitizes cells to apoptosis (Rao et al., 2023). A novel nanoprodrug (DOX@Fc-SS-ATRA NP) combining ferrocene, a differentiation inducer (ATRA), and doxorubicin, enhances TNBC therapy by promoting ferroptosis via the Fenton reaction (Wu C et al., 2023). Furthermore, a small molecule nanoprodrug delivering chemotherapeutics (CPT), Fc, and the GPX4 inhibitor RSL3 inhibits TNBC growth and metastasis through inducing apoptosis and ferroptosis, with Fc and RSL3 amplifying chemotherapy efficacy (Chen et al., 2023).
Advances in pharmacological technologies have led to the development of innovative nanomaterials for breast cancer treatment, valued for their high efficiency and safety. This also includes mitochondria-targeted nanomaterials that deliver ferroptosis-inducing drugs, offering a promising approach to improve therapeutic outcomes. A magnetic nano-photosensitizer complex CSO-SS-Cy7-Hex/SPION/Srfn, capable of accelerating redox reactions and the Fenton reaction, enhances concentration of iron and LPO with reducing GSH levels simultaneously. Through analysis of EMT-related experimental findings, it was observed that the CSO-SS-Cy7-Hex/SPION/Srfn self-assembled complex induced ferroptosis, effectively countering multidrug resistance, invasion, and metastasis in breast cancer (Sang M. et al., 2019). Simvastatin, another ferroptosis-inducing drug, also demonstrated high efficacy with the use of novel nanomaterials. By inhibiting 3-hydroxy-3-methyl-glutaryl-coenzyme A reductase, it regulates cholesterol metabolism via Fe3O4@PCBMA magnetic nanoparticles, downregulating both the mevalonate and GPX4 pathways. This induces ferroptosis in TNBC cells without causing liver or kidney toxicity. These pathways are further linked to mitochondrial glycometabolism and lipid metabolism (Yao X. et al., 2021). A Cu-tetra (4-carboxyphenyl) porphyrin chloride (Fe(III)) Cu-TCPP(Fe)-based MOF nano-system, integrated with Au nanoparticles and loaded with RSL3, blocks GSH biosynthesis by disrupting the pentose phosphate pathway, boosting RSL3-induced ferroptosis (Li K. et al., 2022). Though still in research, these nanomaterials offer promising potential for future BRCA therapies. The incorporation of various ferroptosis-inducing agents into nanoplatforms enhances anticancer efficacy through improved targeted delivery and greater tissue permeability of nanocarriers. Recent reviews highlight these advancements in nanotechnology as promising strategies for breast cancer treatment. Moreover, a recent study demonstrated that Boswellia carterii n-hexane extract (BCHE) significantly inhibited the viability of human breast cancer cells and displayed potent in vivo anti-breast cancer activity without notable toxicity, inducing ferroptosis by upregulating transferrin expression and intracellular Fe2+ levels, downregulating glutathione peroxidase 4 (GPX4), and promoting ROS mediated lipid peroxidation in both breast cancer cells and tumor-bearing mice, suggesting BCHE as a potential therapeutic candidate for ferroptosis-targeted breast cancer treatment (Xie et al., 2024). Furthermore, a study shows that oridonin (ORI), the main active component derived from the Chinese herbal plant Rabdosia rubescens, boosts the anti-proliferative effect of RSL3 on breast cancer cells by facilitating ferroptosis. Mechanistically, it enhanced RSL3-induced ferroptosis in breast cancer cells through activation of the JNK/Nrf2/HO-1 signaling pathway. The combination therapy of RSL3 and ORI suppressed breast cancer cell proliferation, resulting in the accumulation of lipid peroxidation products and iron ions (Ye et al., 2024). In recent years, GSK-3β has been identified as a key tumor suppressor involved in maintaining redox balance. Research by Wang et al. demonstrated that silencing GSK-3β in MDA-MB-231 cells leads to a decrease in DMT1 levels, resulting in lower intracellular ferrous iron accumulation. This reduction subsequently diminishes the sensitivity of these cells to erastin-induced ferroptosis, suggesting that GSK-3β plays a vital role in promoting ferroptosis sensitivity in breast cancer cells (Wang L. et al., 2021). Furthermore, high expression of FADS1/2, enzymes involved in PUFA biosynthesis, was associated with poor prognosis in TNBC and sensitivity to ferroptosis inducers; however, targeting FADS1/2 conferred resistance, which could be reversed by restoring PUFA levels, and inhibiting lipid droplet formation further enhanced ferroptosis, as demonstrated in preclinical models and a patient cohort (Lorito et al., 2024). Moreover, research has shown that BET inhibitors (JQ1 and I-BET151) exert anti-cancer effects in breast cancer by inducing ferroptosis, with NCOA3, as a coactivator, interacting with NR5A2 to counteract BETi-induced ferroptosis. Mechanistically, NR5A2 and NCOA3 cooperate to upregulate NRF2, a transcription factor regulating antioxidant gene expression, and inhibiting NR5A2 or NCOA3 enhances BETi’s anti-cancer effects both in vitro and in vivo. These findings suggest that targeting NR5A2/NCOA3 in combination with BET inhibitors could provide a novel therapeutic strategy for breast cancer treatment (Qiao et al., 2020).
5.2 Cuproptosis in the clinical settings of breast cancer
The discovery of copper-induced cellular death has revealed a new strategy for breast cancer treatment. Promising therapeutics, such as copper ionophores and copper complexes, together with advancements in nanotechnology, have demonstrated significant efficacy in breast cancer treatment (Shanbhag et al., 2021). Copper is a critical factor in the initiation and progression of malignancies, making it a promising target for breast cancer therapy. Copper and its associated proteins, including ATOX1 and CCS, play notable roles in breast cancer progression, metastasis, and poor clinical outcomes (Huffman and O'Halloran, 2001). ATOX1 regulates intracellular copper transport to key proteins such as ATP7A, ATP7B, and SOD1, while also promoting inflammatory neovascularization, wound healing, and the migration of breast cancer cells (Blockhuys and Wittung-Stafshede, 2017). Additionally, CCS facilitates copper transport and inhibits the IGF-1-mediated binding of HIF-1α to hypoxia response elements (HRE), leading to reduced VEGF production and impaired tumor angiogenesis (Seebacher et al., 2016).
A novel approach to breast cancer treatment focuses on copper metabolism, which induces cell death through copper-dependent mechanisms and disrupts copper homeostasis (Tang et al., 2024; Yang Y. et al., 2023). Copper chelators reduce bioavailability by binding copper, while copper ionophores facilitate its intracellular accumulation; these represent the primary strategies in current therapeutic initiatives. Targeting copper-dependent cell death pathways, including cuproptosis, holds potential for improving outcomes in various malignancies, including breast cancer. Recent studies have extensively investigated cuproptosis-related compounds, such as copper ionophores, chelators, and nanomedicines, which exhibit notable anti-cancer effects in breast cancer, as summarized in Table 3.
5.2.1 Copper ionophores
Elevated intracellular copper accumulates in the mitochondria, where it interacts with lipoacylated proteins, causing the oligomerization of DLAT and destabilizing the Fe-S cluster proteins. This disruption leads to cancer cell death, termed cuproptosis (Kahlson and Dixon, 2022). This mechanism has prompted the exploration of copper ionophores, such as DSF and ES, which increase intracellular copper levels and could potentially treat breast cancer. These ionophores form lipophilic complexes with copper, enhancing its cellular accumulation, and thus showing promise for cancer therapy (Lelièvre et al., 2020).
The anti-cancer effects of disulfiram, particularly in breast cancer, have been extensively investigated. DSF, a well-known aldehyde dehydrogenase (ALDH) inhibitor, has demonstrated significant anti-cancer properties, particularly against ALDH-positive breast cancer stem cells (Ni Y. L. et al., 2022). In addition to its targeting of these stem cells, DSF enhances ROS accumulation, thereby effectively overcoming cisplatin resistance in breast cancer cell lines. Such insights may shape future chemotherapeutic strategies (Yang et al., 2019). Research conducted by Swetha et al. indicates that the combination of DSF with docetaxel-loaded nanoparticles enhances the sensitivity of drug-resistant breast cancer cells to docetaxel. This sensitization occurs through the inhibition of the drug efflux pump P-glycoprotein, as well as the targeting of cancer stem cells (CSCs) (Swetha et al., 2023). Zheng et al. demonstrated that DSF enhances PD-L1 expression in TNBC cells, significantly improving the response of mouse breast cancer models to anti-PD-1 antibody therapy. This suggests that combining DSF with anti-PD-1 therapy could be a promising strategy for enhancing TNBC treatment outcomes (Zheng et al., 2021). Furthermore, a combination therapy evident against MCF-7 breast cancer cells, has proven to have a synergistic effect of DOX with hydrazine (Hyd) and DSF to lower the dose of the chemotherapeutic drug (Lafi et al., 2023). DSF couples with copper as a copper ionophore to produce the metabolite bisdiethyldithiocarbamate-copper (CuET), facilitating the transport of copper across the cell membrane (Lu et al., 2021). In addition, cellular damage in breast cancer cell lines resulting from DSF/Cu is associated with apoptosis, ferroptosis, and cuproptosis (Table 3). DSF/Cu inhibits the proliferation of breast cancer stem cells and potentiates the cytotoxic effects of paclitaxel, likely through a combined mechanism of ROS generation and NF-κB pathway suppression (Yip et al., 2011). Acidic pH significantly enhances the cytotoxic effects of the DSF–Cu complex in breast and colon cancer cells. This enhanced toxicity is linked to alterations in cell metabolism, modulation of AKT kinase and NF-κB pathways, and elevated production of ROS (Navrátilová et al., 2013). However, the current clinical effectiveness of DSF/Cu remains ambiguous. Notably, findings from a prospective clinical trial examining DSF paired with Cu for metastatic prostate cancer revealed that DSF was rapidly converted to the inactive metabolite Me-DDC, which resulted in no observable clinical benefits (Zhang T. et al., 2022). In contrast, a Phase II trial indicated that the combination of disulfiram with cisplatin and vinorelbine led to rare long-term survival in two-stage IV lung cancer patients (Nechushtan et al., 2015). Additionally, two separate clinical trials investigating the combination of DSF and Cu for patients with liver metastases (Kelley et al., 2021) and recurrent gliomas (Werlenius et al., 2023) also failed to show significant survival advantages. These divergent results emphasize the need for further research to optimize disulfiram’s therapeutic applications and assess its efficacy across different cancer types, including breast cancer.
ES, a lipophilic copper-binding compound, is capable of chelating extracellular Cu2⁺ ions to form a complex that enables the efficient transport of copper into cells (Gohil, 2021; Guthrie et al., 2020). Once inside the cells, ES exerts anticancer effects primarily by targeting mitochondrial metabolism, inducing oxidative stress, and reducing the expression of CSC markers like CD133 and ALDH (Harrington et al., 2020). Additionally, recent evidence underscores its ability to induce copper-dependent cell death (Tsvetkov et al., 2019), reinforcing its role as a promising agent in breast cancer therapy (Alli and Ford, 2012). Serum lactate dehydrogenase (LDH) levels are closely linked to mitochondrial metabolism, with lower LDH levels correlating to increased sensitivity to ES (Zheng et al., 2022). This observation has significant implications for the use of LDH as a predictive biomarker in cuproptosis-targeting treatments. Notably, in a phase III clinical trial, ES combined with paclitaxel showed limited success overall but demonstrated enhanced efficacy in melanoma patients with lower LDH levels (O'Day et al., 2013), suggesting that mitochondrial metabolism plays a pivotal role in cuproptosis activation. Earlier trials, such as a phase II study, had shown that the addition of ES to paclitaxel significantly prolonged progression-free survival (PFS) in melanoma patients by 41.7%, highlighting its potential as an anti-cancer agent (O'Day et al., 2009). Furthermore, a phase I trial in patients with refractory solid tumors, including Kaposi sarcoma and ovarian cancer, provided partial responses (Berkenblit et al., 2007), further suggesting that ES copper-dependent mechanism could enhance therapeutic outcomes. However, despite promising early-phase results, ES has not consistently demonstrated robust clinical benefits. One potential reason for this is its inability to sufficiently elevate copper levels in tumor cells when used as a monotherapy. This reinforces the need for combinatory strategies and highlights the importance of biomarkers like LDH to optimize patient selection and treatment efficacy.
5.2.2 Small molecular compounds and copper complex
Copper plays a complex and dual role in tumor development. Elevated Cu levels are linked to enhanced tumor cell proliferation and growth, which suggests that tumor cells may employ certain mechanisms to resist cuproptosis. Consequently, targeting Cu homeostasis with small molecular compounds offers a promising strategy to induce or heighten tumor cell sensitivity to cuproptosis. Several recent studies have identified small molecular compounds capable of inducing this copper-dependent cell death. For example, Yang et al. demonstrated that zinc pyrithione disrupts intracellular Cu balance and triggers DLAT oligomerization in TNBC cells, which may contribute to their increased chemosensitivity (Yang X. et al., 2023). Recent research highlights that ferroptosis inducers also potentiate cuproptosis in cancer cells. For example, Sorafenib, the first multi-tyrosine kinase inhibitor approved for various cancers, and erastin, a well-known ferroptosis inducer, have been shown to exacerbate cuproptosis in liver cancer cells (Wang W. et al., 2023), providing new avenues for combined therapeutic strategies. In contrast to copper ionophores, which require copper supplementation, small-molecule compounds like ZnPT that disrupt intracellular copper homeostasis present a more refined therapeutic approach. These compounds can induce cuproptosis in tumor cells without introducing excess copper, minimizing the risk of metal ion imbalances and reducing potential side effects associated with metal toxicity. This method highlights the potential of targeted treatments that leverage intrinsic cellular mechanisms while mitigating adverse effects during therapy.
Copper complexes exhibit diverse catalytic and electrochemical properties (Balsa et al., 2023), positioning them as promising candidates for breast cancer therapy. These complexes exert tumor-inhibitory effects by generating ROS, depleting intracellular glutathione, impairing proteasome function, and inducing DNA damage (Ji et al., 2023), all of which contribute to the selective targeting of cancer cells. Through these mechanisms, copper complexes disrupt cellular homeostasis, promoting cancer cell death and offering potential therapeutic advantages over traditional treatments. Studies have demonstrated that copper complexes like CuHL1 and Cu-PLN inhibit the growth of TNBC cells by triggering cuproptosis. CuHL1 achieves this by downregulating DLAT expression via copper-induced protein lipoylation, while Cu-PLN contributes to cancer cell death by generating ROS, causing DNA damage, and disrupting mitochondrial function, ultimately affecting cellular metabolism (Mukherjee et al., 2023). The study by Xu et al. explored a copper-based complex called HA-Cu, synthesized from disulfonamide-dimethylpyrimidine-phenanthroline-metal, which showed potent inhibitory effects on TNBC (Xu et al., 2024). This complex was effective both in vitro and in vivo, suppressing tumor growth through a synergistic mechanism. It combined antiproliferative, antiangiogenic, anti-inflammatory, and pro-apoptotic properties, inducing cuproptosis in MDA-MB-231 cells by reducing FDX1 expression and increasing HSP70 expression (Xu et al., 2024). Similarly, He et al. developed a copper-chelated cyanine dye to deliver copper ions in various oxidation states, which were tested in 4T1 cells and 4T1 tumor-bearing mice (He et al., 2024). This approach further explored the role of copper in combating TNBC, reinforcing the idea that copper, through various mechanisms like cuproptosis and regulation of key cellular pathways, can be a promising strategy in breast cancer treatment. These mechanisms highlight the potential of copper complexes as targeted therapies against aggressive cancers like breast cancer.
5.2.3 Nano-based therapeutics
Over the past 3 decades, there has been a significant expansion of research in the field of cancer nanotherapeutics (Fan D. et al., 2023). Drugs can be transformed into nanotherapeutics through processes such as dissolution, adsorption, encapsulation, or binding to nanomaterials. These nanotherapeutics leverage unique tumor characteristics such as acidic microenvironments, high levels of GSH and ROS, or tumor-specific surface markers to achieve targeted accumulation and controlled release at the tumor site. This strategic targeting enhances therapeutic precision, significantly reducing off-target effects and the toxicity associated with conventional cancer treatments. The therapeutic effectiveness of chemotherapy can be further improved by combining it with nanocarrier-based methods like photodynamic therapy (PDT), photothermal therapy (PTT), or chemodynamic therapy (CDT), enhancing the overall anticancer efficacy. CDT leverages the Fenton reaction to generate hydroxyl radicals (⋅OH) from overexpressed H₂O₂ within tumor cells, effectively inducing oxidative stress and cell death without the need for external energy sources (Zhao F. et al., 2023).
Given the relatively low selectivity of copper ionophores for tumor cells, employing nanoparticle-based delivery systems can more effectively direct copper to tumor tissues (Zhang C. et al., 2024). This approach heightens cuproptosis activity within tumors while minimizing harm to healthy tissues. Since the identification of cuproptosis, research has increasingly focused on its potential therapeutic applications, particularly in nanoparticle-facilitated copper delivery systems. Researchers have focused on precisely delivering copper, copper ionophores, and other anticancer agents like chemotherapeutic drugs and siRNA to enhance nanomedicine-induced cuproptosis. This targeted approach synergistically increases breast tumor cell damage by sensitizing them to cuproptosis while simultaneously activating additional therapeutic mechanisms (Table 3).
Cu-GA nanoparticles (NPs), which are composed of Cu2⁺, gallic acid, and polyvinylpyrrolidone, have been shown to induce cuproptosis and apoptosis by depleting intracellular GSH levels and increasing ROS production. When combined with CDT, this approach significantly suppresses tumor growth, demonstrating a synergistic effect that enhances therapeutic efficacy (Zhao F. et al., 2023). Similarly, Cu2O@CuBTC-DSF@HA nanocomposites (CCDHs), constructed from Cu₂O, trimesic acid, disulfiram, and hyaluronic acid, offer a unique therapeutic approach by promoting cuproptosis synergistically, without inducing apoptosis. This mode of action increases anti-tumor efficacy while minimizing toxicity, making it a promising strategy for breast cancer treatment (Zhong et al., 2023). SonoCu nanoparticles, a combination of Cu2⁺, a zeolitic imidazolate framework, perfluorocarbon, chlorine-6, and oxygen, have been integrated with sonodynamic therapy (SDT). This system induces cuproptosis specifically in tumor cells while sparing normal cells, leading to strong biosafety and favorable anti-tumor outcomes, which highlights its potential for targeted cancer therapies (Chen K. et al., 2023). HNPs, comprised of DTPH, Cu2⁺, disulfiram, hyaluronan, and artemisinin, also show promise. These particles deplete GSH through their disulfide bonds, sensitizing cancer cells to cuproptosis. By simultaneously inducing cuproptosis, ferroptosis, and apoptosis, HNPs can effectively suppress tumor growth, particularly in 4T1 breast cancer model (Xu W. et al., 2023). CS/MTO-Cu@AMI, a nanocomplex consisting of mitoxantrone, Cu2⁺, amiloride, and chondroitin sulfate, induces cuproptosis and disrupts mitochondrial function in 4T1 breast cancer tumors. By activating the AMPK pathway, this formulation promotes PD-L1 degradation, effectively reducing immune evasion by cancer cells. Furthermore, CS/MTO-Cu@AMI enhances anti-tumor immunity by stimulating the cGAS-STING pathway, which plays a critical role in promoting immune surveillance and response against tumors, making it a promising candidate for combination therapies targeting immune checkpoints (Tian et al., 2023). CuX-P binds to PD-L1 on tumor cells, leading to its internalization, and subsequently triggering cuproptosis. When combined with laser treatment, this process amplifies the anti-tumor immune response by enhancing immune system activation (Liu T. et al., 2023). CuMoO4 Nanodots use prolonged photothermal therapy (PTT) to simultaneously induce ferroptosis and cuproptosis in 4T1 and MCF-7 tumors. This dual-action approach stimulates an immune response through immunogenic cell death (ICD), enhancing its effectiveness as a therapeutic strategy (Zhang J. et al., 2023). ZCProP specifically targets mitochondria, delivering copper ions and prodigiosin, which synergistically induce cuproptosis, ferroptosis, and apoptosis (Deng et al., 2024). The LDH/HA/5-FU nanosheets are designed to selectively target tumor cells, facilitating the rapid release of Cu2⁺ and 5-FU, which together induce apoptosis and cuproptosis while enhancing immune responses. This dual delivery system represents a promising approach that combines Cu-based CDT with chemotherapy for solid tumor treatment (Xia et al., 2024). Complementing this, E-C@DOX NPs effectively inhibit tumor cell stemness and survival pathways, utilizing Cu to disrupt mitochondrial function and trigger cuproptosis, which counters ATP-dependent drug efflux mechanisms, thus reversing DOX resistance (Lu et al., 2024). Additionally, D-CuxOS@Fe–MOF enhances oxidative stress, robustly inducing both ferroptosis and cuproptosis for improved therapeutic response against cancer (Hao et al., 2024). Cu@CDCN further enhances this synergy by integrating photocatalytic hydrogen therapy with anchored Cu2⁺, inducing cuproptosis and mitochondrial dysfunction, ultimately inhibiting tumor cell proliferation and significantly suppressing tumor growth (Ding et al., 2023).
Moreover, ECPCP substantially extends the circulation time of the elesclomol–Cu complex, promoting its accumulation at tumor sites and activating cuproptosis. The complex’s Cu2⁺-driven Fenton-like reactions, alongside ROS production from cinnamaldehyde, disrupt redox balance, triggering ICD and enhancing synergy with immunotherapy (Wu et al., 2024). Furthermore, Au@MSN-Cu/PEG/DSF, CJS-Cu NPs, PDA-DTC/Cu NPs, Cu-LDH, Cu-DBCO/CL, and HA-CD@MOF NPs also represent a promising Frontier in breast cancer therapy by leveraging copper’s unique properties for targeted treatment strategies (Table 3) (Chang et al., 2024; Du et al., 2024; Liu et al., 2024; Zheng et al., 2024; Zhou J. et al., 2023; Zhu G. et al., 2024). Additional studies underscore the potential of novel cuproptosis-based nanotherapeutics in breast cancer. M@HMnO2-DP selectively targets tumor cells, delivering DSF to induce cuproptosis independently of external copper sources while disrupting glycolytic pathways and interfering with Fe–S protein synthesis (Zhu J. et al., 2024). Similarly, PCM nano-inducers enhance proteotoxic stress through cuproptosis, leading to the release of mitochondrial DNA that activates the cGAS-STING pathway, thereby initiating a robust immune response that significantly inhibits tumor progression and metastasis (Yu et al., 2024). A novel strategy involving the co-delivery of Cu and erastin in cancer cells has been developed to achieve a synergistic effect by inducing both cuproptosis and ferroptosis (Li Y. et al., 2024). Copper/erastin (CuP/Er) enhances immunogenic cell death, improves antigen presentation, and upregulates PD-L1, promoting T cell proliferation and infiltration. When combined with immune checkpoint inhibitors, it reactivates T cells, leading to effective tumor regression and preventing metastasis in colon adenocarcinoma and triple-negative breast cancer models (Li Y. et al., 2024). This approach underscores the potential of combining multiple cell death pathways to amplify the efficacy of cancer immunotherapies. Expanding on this approach, Huang et al. developed a poly (amidoamine) dendrimer modified with p-carboxybenzenesulfonamide, loaded with copper peroxide nanoparticles and combined with iron-tannic acid networks to form the nanocomposite CuO₂@G5-BS/TF (Huang et al., 2024). This nanocomposite targeted 4T1 tumor cells, facilitating MRI imaging and inducing both ferroptosis and cuproptosis by depleting glutathione and overloading copper and iron. It further neutralized the acidic tumor microenvironment, effectively inhibiting metastasis and offering a dual-functional strategy for TNBC imaging and treatment (Huang et al., 2024). Similarly, the MetaCell system demonstrated the ability to evade immune detection, penetrate breast tumor cells, and promote both cuproptosis and ferroptosis, which resulted in significant anti-tumor effects in vitro and in vivo (Chen K. et al., 2024). Utilizing these therapies, either independently or alongside conventional treatments like chemotherapy and radiotherapy, could greatly enhance breast cancer management. Continued investigation is essential to fully harness their capabilities and improve clinical applications.
5.3 Clinical trials targeting ferroptosis and cuproptosis in breast cancer
Recent clinical trials are investigating ferroptosis and cuproptosis modulation as promising strategies for treating breast cancer, particularly in advanced or treatment-resistant cases (ClinicalTrials.gov). In ferroptosis-targeted approaches, sorafenib, a System Xc-inhibitor, is undergoing a phase II trial in metastatic breast cancer, aiming to disrupt glutathione synthesis and thereby promote ferroptosis (NCT00101400). Similarly, artesunate, an iron-oxidizing agent, is being assessed in a phase I trial for metastatic breast cancer due to its ability to induce oxidative stress in iron-abundant tumor environments (NCT00764036). Neratinib, which activates iron-dependent pathways, is in phase II trials for both HER-2-positive (NCT00300781) and ER-positive breast cancers (NCT05933395), where it may increase susceptibility to ferroptosis in these specific subtypes. Additionally, deferoxamine (DFO), an iron chelator, is being explored in a phase II trial for metastatic triple-negative breast cancer to deplete iron stores and selectively trigger ferroptotic cell death in aggressive tumor cells (NCT05300958).
Copper modulation is also under investigation, showing promise for advanced breast cancer cases. Disulfiram combined with copper supplementation is in phase II trials for metastatic breast cancer that has progressed despite conventional systemic and/or locoregional therapies (NCT03323346), as well as for CTC_EMT-positive, refractory, metastatic hormone receptor-positive, HER-2-negative breast cancer (NCT04265274). Copper depletion strategies are being explored with ATN-224, in a phase II trial for recurrent or advanced estrogen and progesterone receptor-positive breast cancer (NCT00674557). Tetrathiomolybdate, another copper-depleting agent, is under investigation for various breast cancer risk categories: one phase II trial assesses its role in breast cancer with moderate-to-high recurrence risk (NCT00195091), while a phase I/II trial evaluates its effectiveness in reducing relapse risk in high-risk, TNBC (NCT06134375). These trials collectively seek to delineate the therapeutic potential of ferroptosis and cuproptosis modulation, aiming to improve outcomes and overcome resistance in challenging breast cancer subtypes.
6 Pros and cons of ferroptosis and cuproptosis in breast cancer
Breast cancer displays considerable heterogeneity, not only across different patients but also within tumors from the same individual. This diversity is largely defined by differences in hormone receptor status, HER-2 expression, genetic mutations, and the varying characteristics found within individual tumors (Liu S. Q. et al., 2022; Taylor et al., 2024). Hormone receptor-positive breast cancers (ER+ and/or PR+) and TNBC exhibit significant differences in their biology, response to treatments, and overall prognosis (Amgad et al., 2024). Tumors with HER-2 overexpression respond well to therapies specifically targeting HER-2, such as trastuzumab, but may show diminished effectiveness with non-targeted treatment options (Sirhan et al., 2022). Frequent genetic mutations in breast cancer, such as TP53, PIK3CA, and GATA3, play a key role in shaping tumor aggressiveness and influencing treatment outcomes (Martínez-Sáez et al., 2020; Mosele et al., 2020). Furthermore, breast tumors often exhibit intra-tumoral heterogeneity, with distinct cellular subpopulations displaying differences in morphology, growth potential, and gene expression (Martelotto et al., 2014). This molecular diversity drives the tumor’s biological characteristics and significantly affects how it responds to different treatment approaches.
6.1 Advantages
Inducing ferroptosis and cuproptosis represents a compelling therapeutic strategy for targeting breast cancer’s metabolic weaknesses, particularly in treatment-resistant cases. Ferroptosis capitalizes on cancer cells’ iron dependency and susceptibility to lipid peroxidation, leading to oxidative damage and cell death. On the other hand, cuproptosis leverages the toxic accumulation of copper to disrupt mitochondrial function, causing cell death by impairing energy production. These distinct pathways exploit specific vulnerabilities in aggressive breast cancer subtypes, such as TNBC, significantly enhancing the potential for cancer cell eradication. Both paths offer promising solutions for overcoming treatment resistance, a common challenge in breast cancer therapy. Many cancer cells that develop resistance to conventional treatments such as chemotherapy, radiotherapy, or hormonal therapy remain susceptible to ferroptosis and cuproptosis. This opens new avenues for therapy, potentially restoring the effectiveness of existing treatments. Additionally, the selective nature of these cell death mechanisms, which rely on the disruption of iron and copper homeostasis, makes them more likely to affect cancer cells preferentially, thereby minimizing collateral damage to healthy tissues. Furthermore, combining ferroptosis and cuproptosis with conventional therapies, such as chemotherapy and immunotherapy, may result in synergistic effects. By simultaneously targeting cancer cells through disruption of both iron and copper metabolism, along with traditional cytotoxic therapies, the overall therapeutic efficacy can be enhanced. Preclinical studies have shown that ferroptosis not only inhibits tumor growth but also suppresses metastasis in breast cancer models. Although cuproptosis is a newer concept, its disruption of mitochondrial function offers a similar potential for tumor metastasis, particularly in highly proliferative cancer cells. Beyond their cytotoxic effects, ferroptosis has been associated with immunogenic cell death, which may enhance the efficacy of immunotherapies. Similarly, there is growing interest in the potential of cuproptosis to stimulate an immune response, thereby further increasing its therapeutic value. Both pathways demonstrate versatility across breast cancer subtypes, including TNBC and HER-2-positive cancers, by addressing distinct metabolic vulnerabilities—ferroptosis targeting iron metabolism and cuproptosis focusing on mitochondrial activity and copper homeostasis. This versatility highlights their potential as powerful tools in the fight against breast cancer cases (Ai L. et al., 2024; Li Z. et al., 2020; Qi X. et al., 2022; Zhang C. et al., 2024; Zhou et al., 2022; Zhu Z. et al., 2024).
By targeting these regulated cell death pathways, researchers and clinicians can address the heterogeneity of breast cancer, offering new treatment options for resistant or aggressive forms of the disease.
6.2 Disadvantages
Inducing ferroptosis and cuproptosis in breast cancer represents several challenges. Off-target toxicity is a primary concern, as disrupting iron or copper homeostasis could harm healthy tissues, particularly vital organs like the liver and brain, especially with GPX4 inhibitors affecting the development and function of the nervous system and kidneys (Koppula et al., 2021; Liang et al., 2019). This could lead to organ dysfunction and systemic toxicity. Additionally, inflammatory responses triggered by ferroptosis-related lipid peroxidation or cuproptosis-related mitochondrial dysfunction may exacerbate inflammation, potentially promoting tumor progression or treatment resistance. Tumor heterogeneity adds complexity, as cancer cells vary in their susceptibility to ferroptosis (Liang et al., 2019) and cuproptosis, allowing some cells to evade death or develop resistance by enhancing antioxidant defenses or modulating metal metabolism. Moreover, the mechanistic understanding of these pathways in vivo remains incomplete, which could result in unexpected toxicities or interactions with other cell death mechanisms like apoptosis or autophagy. Targeting specificity is another challenge, as developing therapies that induce ferroptosis or cuproptosis selectively in cancer cells without affecting healthy tissues is difficult, increasing the risk of broad cytotoxic effects. Unpredictable interactions with existing treatments, such as chemotherapy, radiotherapy, or immunotherapy, may complicate treatment outcomes and limit their effectiveness. Lastly, prolonged targeting of metal metabolism risks systemic dysregulation, potentially leading to iron overload or copper toxicity, which could cause long-term tissue damage (Chen Z. et al., 2023; Kciuk et al., 2024; Zhou Q. et al., 2024). Addressing these challenges is critical to safely harnessing ferroptosis and cuproptosis for breast cancer treatment.
Ferroptosis and cuproptosis inducers show potential in enhancing chemotherapy effects and overcoming drug resistance in cancer treatment. However, combining these therapies can cause side effects on normal tissues. To address this, there is a need for a drug that co-targets both pathways, responding selectively to conditions in the tumor microenvironment, while minimizing harm to healthy cells. Developing such a compound is challenging and requires careful consideration of various factors, including tumor heterogeneity and potential toxicity.
7 Overview and perspectives
Breast cancer treatment encompasses multiple modalities, including surgery, chemotherapy, radiotherapy, hormone therapy, targeted therapy, and immunotherapy. Recent advances underscore the potential of ferroptosis and cuproptosis to enhance the efficacy of these treatments, particularly in overcoming therapeutic resistance. Chemotherapy remains a cornerstone of treatment for metastatic breast cancer; however, it frequently encounters resistance. The introduction of ferroptosis inducers has shown promise in sensitizing breast cancer cells, especially in TNBC. For instance, combining ferroptosis inducers with anthracyclines (Zhao J. et al., 2023) may enhance therapeutic responses by reversing drug resistance (Zhao J. et al., 2023). In the context of multiple myeloma, the activation of ferroptosis through erastin has been demonstrated to synergize with DOX, thereby enhancing its anti-cancer efficacy (Fu et al., 2022). Nevertheless, a major obstacle remains in inducing ferroptosis to combat anthracycline resistance while minimizing the risk of cardiac toxicity, which continues to be a critical area of research focus. In a similar vein, cuproptosis also contributes to tumor suppression in cancer (Tong et al., 2022). In colorectal cancer, curcumin functions as a copper ionophore, facilitating cuproptosis to exert anti-cancer effects (Yang Y. et al., 2022). Moreover, the combination of ES and 4-octyl itaconate (4-OI) has demonstrated a significant reduction in cell proliferation in oxaliplatin-resistant colorectal cells, primarily through the promotion of cuproptosis via GAPDH inhibition and suppression of aerobic glycolysis (Yang et al., 2023). Additionally, ES has been shown to elevate ROS levels in cisplatin-resistant lung cancer cells, aiding in the eradication of drug-resistant tumor cells (Tong et al., 2022). Lun et al. found that combining low doses of DSF with copper supplementation significantly enhances the effectiveness of temozolomide (TMZ) in vitro. This combination therapy also resulted in improved survival rates in vivo within brain tumor-initiating cell (BTIC) models derived from patients, which are typically resistant to standard treatments and come from both newly diagnosed and recurrent tumors. Thus, the DSF-Cu combination holds potential as an adjuvant therapy for glioblastoma management at initial diagnosis and during recurrence (Lun et al., 2016). In addition, cuproptosis can also be targeted in breast cancer; TNBC’s heightened copper demand allows for the use of copper ionophores, which induce cuproptosis and promote mitochondrial stress, offering a complementary strategy to chemotherapeutics. We have compiled the findings on cuproptosis and its relevance to breast cancer in the previous sections and Table 3.
Radiotherapy, typically utilized postoperatively to eliminate residual cancer cells and reduce recurrence risk, may benefit from the induction of ferroptosis (Lang et al., 2019; Lei G. et al., 2020). Resistance to radiotherapy is a significant factor contributing to its limited effectiveness. The sensitivity of tumors to radiotherapy can differ not only between various tumor types but also among individuals with the same diagnosis, likely due to tumor heterogeneity (Gong et al., 2021; Schaue and McBride, 2015). Recent research into radiotherapy resistance has indicated that ferroptosis inducers (FINs) can enhance the sensitivity of ionizing radiation-resistant cells and xenograft tumors to radiation therapy. Compounds such as erastin, sulfasalazine, FIN56, and RSL3 have demonstrated potential in this regard (Lei G. et al., 2020; Ye et al., 2020; Zhang W. et al., 2021). There is optimism for the development of effective combined therapies that incorporate these drugs with radiotherapy for improved cancer treatment in clinical settings. Moreover, integrating copper-mediated cell death with radiotherapy and chemotherapy has the potential to enhance their anti-cancer effects. Cancer stem cells (CSCs) are involved in treatment resistance (Zhang L. et al., 2023), and their high mitochondrial metabolism makes cuproptosis an appealing strategy to overcome this challenge (Ni et al., 2022). A recent study by Wang et al. demonstrated that combining ionizing radiation with DSF and Cu induced a robust cell stress response in breast cancer. This approach significantly increased immunogenic cell death in both differentiated cancer cells and CSCs, while also promoting the expansion of chimeric antigen receptor T cells (CAR-T) (Wang Y. et al., 2023c), a cutting-edge immunotherapy. Consequently, DSF/Cu may enhance the effectiveness of CAR-T therapy, offering promising new treatment options for breast cancer. It is well-established that immunotherapies have shown great efficacy against numerous tumors. However, resistance to immunotherapies remains a challenge due to low immune cell infiltration or suppression of anti-tumor responses within the TME. The TME plays an immunosuppressive role, with lipid metabolism being a defining aspect closely tied to ferroptosis through lipid peroxidation pathways (Mao et al., 2021; Xia et al., 2021). This intricate interaction between ferroptosis and the TME significantly affects the response to immunotherapy, either enhancing effectiveness or contributing to resistance (Tang D. et al., 2020; Wang et al., 2021). Ferroptosis-targeting therapies can enhance immunotherapy efficacy by overcoming resistance, alleviating suppression of anti-tumor immune cells, and selectively inducing ferroptosis in immunosuppressive cells (Zhai et al., 2024). Cystinase, a ferroptosis inducer (FIN), significantly raises oxidative stress, promoting cancer cell death (Cramer et al., 2017). In ovarian cancer models, combining cystinase with immune checkpoint inhibitors, specifically PD-L1 blockers, has been shown to amplify T cell-driven anti-tumor responses, resulting in enhanced tumor suppression (Xiong et al., 2021). Myeloid-derived suppressor cells (MDSCs) are immunosuppressive cells that accumulate under pathological conditions, inhibiting dendritic cell (DC) functions and T-cell immune responses. In colon cancer, targeting ASAH2 on MDSCs infiltrating tumors with NC06 has been shown to induce ferroptosis in these cells, thereby reducing their presence in tumor cells. This reduction subsequently activates cytotoxic T lymphocytes, enhancing tumor suppression (Zhu et al., 2021). These findings suggest NC06’s potential as a therapeutic approach to modulate the pro-inflammatory immune tumor microenvironment. In the LAR subtype of TNBC, elevated GPX4 levels enhance anti-tumor immunity; however, inhibiting GPX4 induces ferroptosis, particularly when combined with anti-PD1 therapy, leading to greater efficacy compared to monotherapy. Conversely, in glioma and TNBC, GPX4 knockout in B cells suppresses immune responses through lipid peroxidation and ferroptosis (Jiang L. et al., 2023; Yang F. et al., 2023; Yang et al., 2014). Overall, immunotherapy in TNBC exhibits a dual effect influenced by ferroptosis, with these interactions shaped by tumor heterogeneity and the TME.
Given that copper metabolism and cuproptosis significantly influence tumor immunity, targeting cuproptosis may enhance immunotherapy effectiveness. The PD-1/PD-L1 pathway is a key immune checkpoint, and inhibiting PD-1/PD-L1 could improve clinical outcomes for cancer patients (Tiwari et al., 2022). Copper may positively regulate PD-L1 expression in tumors. For example, disulfiram–Cu enhances PD-L1 levels in hepatocellular carcinoma (HCC) cells by inhibiting PARP1 and promoting GSK-3β phosphorylation, which reduces T-cell infiltration. Targeting cuproptosis alongside PD-1/PD-L1 inhibitors could improve therapeutic outcomes. Preclinical studies demonstrate that combining copper ionophores with anti-PD-L1 agents enhances tumor growth suppression in pancreatic ductal adenocarcinoma (PDAC), lung cancer, and HCC (Huang et al., 2023; Li P. et al., 2023; Zhou et al., 2019). In the context of hormone therapy, which targets estrogen receptor-positive (ER+) breast cancers through agents like tamoxifen or aromatase inhibitors, resistance remains a challenge. Inducing ferroptosis in these hormone-dependent cancer cells could overcome resistance by targeting oxidative stress pathways that these cells exploit for survival. Additionally, recent studies suggest that endocrine therapies may increase ferroptosis sensitivity in HR+ cancers by potentially reducing GPX4 activity. The development of the FERscore (Hu et al., 2024), a ferroptosis susceptibility index, highlights a potential therapeutic avenue, suggesting that combining ferroptosis inducers with endocrine therapy could selectively target resistant tumor cells (Li P. et al., 2023). In terms of HER-2-positive breast cancer, ferroptosis holds the potential to overcome treatment resistance. Studies show that inhibiting FGFR4 (Zou et al., 2022) could induce ferroptosis in resistant cells, enhancing sensitivity to therapies like trastuzumab. Additionally, combining ferroptosis inducers with HER-2-targeted treatments boosts ROS production, leading to increased cell death. Neratinib, an irreversible HER-2 inhibitor, has been found to induce ferroptosis in HER-2-positive breast cancer models. By promoting mitochondrial dysfunction and increasing iron-dependent ROS levels, neratinib works synergistically with ferroptosis inducers like RSL3. This combination not only boosts cell death but also helps inhibit brain metastasis in HER-2-positive cases, indicating a dual advantage in targeting both HER-2 and ferroptosis pathways. The therapy pairing neratinib with RSL3 enhances lipid peroxidation and mitochondrial impairment, resulting in increased ferroptotic cell death, providing a promising strategy for improving outcomes in patients with HER-2-positive breast cancer who may not adequately respond to standard treatments (Hu et al., 2024; Park et al., 2023). Integrating ferroptosis-related biomarkers with tumor mutation burden evaluations could improve prognostic insights in HER-2-positive patients. This approach may help identify individuals who are more likely to respond positively to therapies that target ferroptosis in addition to standard anti-HER-2 treatments (Shi et al., 2024). Future research should focus on finding effective combinations of ferroptosis inducers with current HER-2-targeted therapies to improve treatment outcomes. Understanding the mechanisms that enhance efficacy is crucial. Additionally, ongoing clinical trials exploring the relationship between ferroptosis and anti-HER-2 therapies will be essential for validating findings and determining optimal strategies for treating HER-2-positive breast cancer patients.
Breast cancer cells demonstrate a distinct copper metabolism, requiring elevated copper levels, which makes them more vulnerable to cuproptosis-inducing agents. Research shows that disruptions in copper metabolism are closely linked to BRCA progression. Overexpression of copper transport proteins like SLC31A1 enhances copper uptake, leading to cuproptosis (Wang J. et al., 2024). Alterations in genes related to copper metabolism disrupt homeostasis, resulting in intracellular copper accumulation and triggering cell death through cuproptosis. Modulating the expression of these genes can effectively regulate BRCA cell growth and proliferation. For instance, TNBC is highly sensitive to cuproptosis inducers such as ZnPT, which interferes with copper balance, causing cell death by DLAT aggregation and inhibiting tumor proliferation, migration, and stemness (Yang X. et al., 2023). The interplay between cuproptosis and BRCA emphasizes the importance of molecular heterogeneity in therapeutic responses, with different BRCA subtypes showing varying sensitivity to cuproptosis-inducing treatments (Tang et al., 2024). Cuproptosis, driven by copper accumulation, impacts hormone receptor-positive (HR+) breast cancer prognosis. Elevated levels of genes like DLAT, FDX1, and PDHA1 correlate with poor outcomes, greater tumor aggressiveness, and immune suppression. Treatment strategies could include copper modulation or combining cuproptosis inducers with hormone therapies. However, response variability across patients underlines the need for further research to refine personalized approaches (Li J. et al., 2022; Sha R. et al., 2024; Wang R. et al., 2023; Zhu Z. et al., 2024). Similarly, HER-2-positive BRCA cells, responsive to HER-2-targeted therapies like trastuzumab, warrant further investigation regarding their reaction to cuproptosis inducers (Sha R. et al., 2024). A recent study developed a prognostic model using four CRGs, identifying DLAT as an independent prognostic marker linked to resistance against HER-2-targeted therapy in HER-2-positive breast cancer patients. Combining HER-2-targeted treatments with cuproptosis inducers might enhance efficacy by affecting copper metabolism and HER-2 signaling pathways. TNBC, with its significant reliance on copper, presents a particularly promising target for cuproptosis-based therapies.
A promising avenue for advancing cancer therapy involves highlighting the interplay between ferroptosis and cuproptosis, aiming to target both pathways for improved therapeutic outcomes simultaneously. The close association between ferroptosis and cuproptosis with mitochondrial metabolism underscores the necessity of exploring mitochondria to fully grasp the underlying mechanisms, regulatory mechanisms, and implications for disease (Gao et al., 2024; Wang W. et al., 2023).
Moreover, GSH serves as a pivotal nexus for both ferroptosis and cuproptosis, in addition to its involvement in the mitochondrial TCA cycle. During ferroptosis, GSH functions as an antioxidant, impeding lipid peroxidation. In cuproptosis, GSH acts as a copper chaperone, binding copper to prevent the aggregation of lipoylated proteins. Intriguingly, GSH can suppress both ferroptosis and cuproptosis, indicating a reciprocal regulatory relationship in which GSH likely plays a pivotal role in facilitating communication between these processes (Liu and Chen, 2024; Tsui et al., 2024; Wang J. et al., 2024; Kciuk et al., 2024). Thoroughly comprehending the relationship and mutually influencing aspects of ferroptosis and cuproptosis in cancer should provide a theoretical foundation for synergic treatment approaches.
Moving forward, a crucial area for prospective investigation involves the development of signatures encompassing both ferroptosis and cuproptosis-related ncRNAs. A thorough understanding of the mechanisms underlying the genes associated with ferroptosis and cuproptosis identified in this review is paramount for further elucidation. Several limitations necessitate consideration concerning the ferroptosis and cuproptosis-related gene signatures. First, the studies primarily relied on public databases, inherently introducing selection bias into the study design. Second, despite certain key findings being substantiated by experimental validation, additional experiments are necessary to delve into potential mechanisms, particularly regarding the interaction between cuproptosis and ferroptosis. Evaluating the clinical efficacy of existing gene signatures will be critical for their possible integration into personalized treatment approaches for breast cancer.
Ferroptosis inducers can effectively kill cancer cells, but they may also harm immune cells, thereby suppressing anti-tumor responses. To address this issue, future research should focus on developing small-molecule drugs that selectively trigger ferroptosis in cancer cells while preserving immune function. In parallel, copper chelators and complexes have shown promise in enhancing chemotherapy and preventing resistance in preclinical studies; however, cuproptosis-targeted therapies remain underdeveloped compared to those for copper-related genetic diseases.
Co-targeting ferroptosis and cuproptosis by altering mitochondrial and metal ion homeostasis may personalize cancer treatment by utilizing tumor-specific iron and copper metabolic requirements. This approach aligns with the principles of precision medicine, potentially enhancing patient outcomes and treatment efficacy. Inducing both ferroptosis and cuproptosis can significantly boost the effectiveness of chemotherapy and immunotherapy. Additionally, while radiotherapy is known to trigger ferroptosis (Lei G. et al., 2020), its potential to induce cuproptosis remains unclear. Co-targeting these pathways shows promise over monotherapies, particularly within specific cancer types. However, further research is essential to uncover the underlying molecular mechanisms and address the potential damage to healthy tissues. Developing innovative therapies, especially nanomedicines, will be critical. A deeper understanding of the interactions between these regulated cell death pathways and breast cancer is necessary to enhance future therapeutic strategies.
8 Conclusion
This review highlights the dual-targeting of ferroptosis and cuproptosis as a transformative strategy for breast cancer therapy, focusing on pathways that activate both cell death processes to combat resistance, especially in challenging subtypes like TNBC. Current findings show that inducers of ferroptosis and cuproptosis can enhance sensitivity to traditional treatments, from chemotherapy to immunotherapy, and may address resistance in both advanced and early stages. However, effective clinical translation requires further investigation into tumor-specific iron and copper metabolism and strategies to mitigate potential toxicity to non-cancerous cells. These insights emphasize the potential for precision treatments tailored to exploit metabolic vulnerabilities, advancing the application of targeted therapies and novel combinational approaches for breast cancer management.
Author contributions
MI: Software, Visualization, Writing–original draft, Writing–review and editing. JJ: Data curation, Writing–review and editing. ZZ: Writing–review and editing. SY: Conceptualization, Supervision, Writing–review and editing.
Funding
The author(s) declare that financial support was received for the research, authorship, and/or publication of this article. This work was supported by the Liaoning Province Science and Technology Plan Joint Program (Natural Science Foundation—General Program, grant number 2024-MSLH-571) to SY in 2024.
Acknowledgments
All figures were created with BioRender.com.
Conflict of interest
The authors declare that the research was conducted in the absence of any commercial or financial relationships that could be construed as a potential conflict of interest.
Publisher’s note
All claims expressed in this article are solely those of the authors and do not necessarily represent those of their affiliated organizations, or those of the publisher, the editors and the reviewers. Any product that may be evaluated in this article, or claim that may be made by its manufacturer, is not guaranteed or endorsed by the publisher.
References
Abbasi, U., Abbina, S., Gill, A., Takuechi, L. E., and Kizhakkedathu, J. N. (2021). Role of iron in the molecular pathogenesis of diseases and therapeutic opportunities. ACS Chem. Biol. 16 (6), 945–972. doi:10.1021/acschembio.1c00122
Adams, S., Diéras, V., Barrios, C., Winer, E., Schneeweiss, A., Iwata, H., et al. (2020). Patient-reported outcomes from the phase III IMpassion130 trial of atezolizumab plus nab-paclitaxel in metastatic triple-negative breast cancer. Ann. Oncol. 31 (5), 582–589. doi:10.1016/j.annonc.2020.02.003
Ai, L., Yi, N., Qiu, C., Huang, W., Zhang, K., Hou, Q., et al. (2024). Revolutionizing breast cancer treatment: harnessing the related mechanisms and drugs for regulated cell death (Review). Int. J. Oncol. 64 (5), 46. doi:10.3892/ijo.2024.5634
Ali, A. S., Nazar, M. E., Mustafa, R. M., Hussein, S., Qurbani, K., and Ahmed, S. K. (2024). Impact of heavy metals on breast cancer (Review). World Acad. Sci. J. 6 (1), 4. doi:10.3892/wasj.2023.219
Alli, E., and Ford, J. M. (2012). Breast cancers with compromised DNA repair exhibit selective sensitivity to elesclomol. DNA Repair (Amst) 11 (5), 522–524. doi:10.1016/j.dnarep.2012.02.003
Amgad, M., Hodge, J. M., Elsebaie, M. A. T., Bodelon, C., Puvanesarajah, S., Gutman, D. A., et al. (2024). A population-level digital histologic biomarker for enhanced prognosis of invasive breast cancer. Nat. Med. 30 (1), 85–97. doi:10.1038/s41591-023-02643-7
Anderson, G. J., and Frazer, D. M. (2017). Current understanding of iron homeostasis. Am. J. Clin. Nutr. 106, 1559S–1566S. doi:10.3945/ajcn.117.155804
Angeli, J. P. F., Schneider, M., Proneth, B., Tyurina, Y. Y., Tyurin, V. A., Hammond, V. J., et al. (2014). Inactivation of the ferroptosis regulator Gpx4 triggers acute renal failure in mice. Nat. Cell Biol. 16 (12), 1180–U1120. doi:10.1038/ncb3064
Arnold, M., Morgan, E., Rumgay, H., Mafra, A., Singh, D., Laversanne, M., et al. (2022). Current and future burden of breast cancer: global statistics for 2020 and 2040. Breast 66, 15–23. doi:10.1016/j.breast.2022.08.010
Aron, A. T., Ramos-Torres, K. M., Cotruvo Jr, J. A., and Chang, C. J. (2015). Recognition-and reactivity-based fluorescent probes for studying transition metal signaling in living systems. Accounts Chem. Res. 48 (8), 2434–2442. doi:10.1021/acs.accounts.5b00221
Avagliano, A., Ruocco, M. R., Aliotta, F., Belviso, I., Accurso, A., Masone, S., et al. (2019). Mitochondrial flexibility of breast cancers: a growth advantage and a therapeutic opportunity. Cells 8 (5), 401. doi:10.3390/cells8050401
Bacci, M., Lorito, N., Smiriglia, A., and Morandi, A. (2021). Fat and furious: lipid metabolism in antitumoral therapy response and resistance. Trends Cancer 7 (3), 198–213. doi:10.1016/j.trecan.2020.10.004
Badgley, M. A., Kremer, D. M., Maurer, H. C., DelGiorno, K. E., Lee, H. J., Purohit, V., et al. (2020). Cysteine depletion induces pancreatic tumor ferroptosis in mice. Science 368 (6486), 85–89. doi:10.1126/science.aaw9872
Bai, F., Morcos, F., Sohn, Y. S., Darash-Yahana, M., Rezende, C. O., Lipper, C. H., et al. (2015). The Fe-S cluster-containing NEET proteins mitoNEET and NAF-1 as chemotherapeutic targets in breast cancer. Proc. Natl. Acad. Sci. U. S. A. 112 (12), 3698–3703. doi:10.1073/pnas.1502960112
Balsa, L. M., Baran, E. J., and León, I. E. (2023). Copper complexes as antitumor agents: in vitro and in vivo evidence. Curr. Med. Chem. 30 (5), 510–557. doi:10.2174/0929867328666211117094550
Berkenblit, A., Eder, J. P., Ryan, D. P., Seiden, M. V., Tatsuta, N., Sherman, M. L., et al. (2007). Phase I clinical trial of STA-4783 in combination with paclitaxel in patients with refractory solid tumors. Clin. Cancer Res. 13 (2 Pt 1), 584–590. doi:10.1158/1078-0432.Ccr-06-0964
Bleackley, M. R., and Macgillivray, R. T. (2011). Transition metal homeostasis: from yeast to human disease. Biometals 24 (5), 785–809. doi:10.1007/s10534-011-9451-4
Blockhuys, S., and Wittung-Stafshede, P. (2017). Copper chaperone Atox1 plays role in breast cancer cell migration. Biochem. Biophys. Res. Commun. 483 (1), 301–304. doi:10.1016/j.bbrc.2016.12.148
Bogdan, A. R., Miyazawa, M., Hashimoto, K., and Tsuji, Y. (2016). Regulators of iron homeostasis: new players in metabolism, cell death, and disease. Trends Biochem. Sci. 41 (3), 274–286. doi:10.1016/j.tibs.2015.11.012
Brand, M. D., Orr, A. L., Perevoshchikova, I. V., and Quinlan, C. L. (2013). The role of mitochondrial function and cellular bioenergetics in ageing and disease. Br. J. Dermatol 169 (Suppl. 2), 1–8. doi:10.1111/bjd.12208
Buccarelli, M., Marconi, M., Pacioni, S., De Pascalis, I., D'Alessandris, Q. G., Martini, M., et al. (2018). Inhibition of autophagy increases susceptibility of glioblastoma stem cells to temozolomide by igniting ferroptosis. Cell Death Dis. 9 (8), 841. doi:10.1038/s41419-018-0864-7
Carr, H. S., and Winge, D. R. (2003). Assembly of cytochrome c oxidase within the mitochondrion. Acc. Chem. Res. 36 (5), 309–316. doi:10.1021/ar0200807
Chang, C. J. (2015). Searching for harmony in transition-metal signaling. Nat. Chem. Biol. 11 (10), 744–747. doi:10.1038/nchembio.1913
Chang, J., Yin, W., Zhi, H., Chen, S., Sun, J., Zhao, Y., et al. (2024). Copper deposition in polydopamine nanostructure to promote cuproptosis by catalytically inhibiting copper exporters of tumor cells for cancer immunotherapy. Small 20, 2308565. doi:10.1002/smll.202308565
Chang, V. C., Cotterchio, M., and Khoo, E. (2019). Iron intake, body iron status, and risk of breast cancer: a systematic review and meta-analysis. BMC cancer 19 (1), 543. doi:10.1186/s12885-019-5642-0
Chen, C.-H., Huang, Y.-M., Grillet, L., Hsieh, Y.-C., Yang, Y.-W., and Lo, K.-Y. (2023). Gallium maltolate shows synergism with cisplatin and activates nucleolar stress and ferroptosis in human breast carcinoma cells. Cell. Oncol. 46 (4), 1127–1142. doi:10.1007/s13402-023-00804-x
Chen, D., Cui, Q. C., Yang, H., and Dou, Q. P. (2006). Disulfiram, a clinically used anti-alcoholism drug and copper-binding agent, induces apoptotic cell death in breast cancer cultures and xenografts via inhibition of the proteasome activity. Cancer Res. 66 (21), 10425–10433. doi:10.1158/0008-5472.Can-06-2126
Chen, H.-C., Tang, H.-H., Hsu, W.-H., Wu, S.-Y., Cheng, W.-H., Wang, B.-Y., et al. (2022). Vulnerability of triple-negative breast cancer to saponin Formosanin C-induced ferroptosis. Antioxidants 11 (2), 298. doi:10.3390/antiox11020298
Chen, K., Zhou, A., Zhou, X., He, J., Xu, Y., and Ning, X. (2024). Cellular Trojan Horse initiates bimetallic Fe-Cu MOF-mediated synergistic cuproptosis and ferroptosis against malignancies. Sci. Adv. 10 (15), eadk3201. doi:10.1126/sciadv.adk3201
Chen, K., Zhou, A., Zhou, X., Liu, Y., Xu, Y., and Ning, X. (2023). An intelligent cell-derived nanorobot bridges synergistic crosstalk between sonodynamic therapy and cuproptosis to promote cancer treatment. Nano Lett. 23 (7), 3038–3047. doi:10.1021/acs.nanolett.3c00434
Chen, L., Min, J., and Wang, F. (2022). Copper homeostasis and cuproptosis in health and disease. Signal Transduct. Target Ther. 7 (1), 378. doi:10.1038/s41392-022-01229-y
Chen, T., Liang, L., Wang, Y., Li, X., and Yang, C. (2024). Ferroptosis and cuproptposis in kidney Diseases: dysfunction of cell metabolism. Apoptosis 29 (3), 289–302. doi:10.1007/s10495-023-01928-z
Chen, Y., Yao, Z., Liu, P., Hu, Q., Huang, Y., Ping, L., et al. (2023). A self-assembly nano-prodrug for triple-negative breast cancer combined treatment by ferroptosis therapy and chemotherapy. Acta Biomater. 159, 275–288. doi:10.1016/j.actbio.2023.01.050
Chen, Y.-M., Xu, W., Liu, Y., Zhang, J.-H., Yang, Y.-Y., Wang, Z.-w., et al. (2023). Anomanolide C suppresses tumor progression and metastasis by ubiquitinating GPX4-driven autophagy-dependent ferroptosis in triple negative breast cancer. Int. J. Biol. Sci. 19 (8), 2531–2550. doi:10.7150/ijbs.82120
Chen, Z., Wang, W., Abdul Razak, S. R., Han, T., Ahmad, N. H., and Li, X. (2023). Ferroptosis as a potential target for cancer therapy. Cell Death Dis. 14 (7), 460. doi:10.1038/s41419-023-05930-w
Cheng, M., Liu, P., and Xu, L. X. (2020). Iron promotes breast cancer cell migration via IL-6/JAK2/STAT3 signaling pathways in a paracrine or autocrine IL-6-rich inflammatory environment. J. Inorg. Biochem. 210, 111159. doi:10.1016/j.jinorgbio.2020.111159
Chipurupalli, S., Jiang, P., Liu, X., Santos, J. L., Marcato, P., and Rosen, K. V. (2023). Three-dimensional growth sensitizes breast cancer cells to treatment with ferroptosis-promoting drugs. Cell Death Dis. 14 (9), 580. doi:10.1038/s41419-023-06106-2
Chu, M., An, X., Fu, C., Yu, H., Zhang, D., Li, Q., et al. (2023). Disulfiram/copper induce ferroptosis in triple-negative breast cancer cell line MDA-MB-231. Front. Biosci. (Landmark Ed.) 28 (8), 186. doi:10.31083/j.fbl2808186
Courtney, D., Davey, M. G., Moloney, B. M., Barry, M. K., Sweeney, K., McLaughlin, R. P., et al. (2022). Breast cancer recurrence: factors impacting occurrence and survival. Ir. J. Med. Sci. (1971) 191 (6), 2501–2510. doi:10.1007/s11845-022-02926-x
Cramer, S. L., Saha, A., Liu, J., Tadi, S., Tiziani, S., Yan, W., et al. (2017). Systemic depletion of L-cyst(e)ine with cyst(e)inase increases reactive oxygen species and suppresses tumor growth. Nat. Med. 23 (1), 120–127. doi:10.1038/nm.4232
Członkowska, A., Litwin, T., Dusek, P., Ferenci, P., Lutsenko, S., Medici, V., et al. (2018). Wilson disease. Nat. Rev. Dis. Prim. 4 (1), 21. doi:10.1038/s41572-018-0018-3
Dai, Y., Zhu, L., Li, X., Zhang, F., Chen, K., Jiao, G., et al. (2024). A biomimetic cuproptosis amplifier for targeted NIR-II fluorescence/photoacoustic imaging-guided synergistic NIR-II photothermal immunotherapy. Biomaterials 305, 122455. doi:10.1016/j.biomaterials.2023.122455
de Bie, P., Muller, P., Wijmenga, C., and Klomp, L. W. (2007). Molecular pathogenesis of Wilson and Menkes disease: correlation of mutations with molecular defects and disease phenotypes. J. Med. Genet. 44 (11), 673–688. doi:10.1136/jmg.2007.052746
Deng, J., Zhuang, H., Shao, S., Zeng, X., Xue, P., Bai, T., et al. (2024). Mitochondrial-targeted copper delivery for cuproptosis-based synergistic cancer therapy. Adv. Healthc. Mater. 13, 2304522. doi:10.1002/adhm.202304522
Denoyer, D., Masaldan, S., La Fontaine, S., and Cater, M. A. (2015). Targeting copper in cancer therapy: 'Copper that Cancer. Metallomics 7 (11), 1459–1476. doi:10.1039/c5mt00149h
Ding, H., Ren, F., Liu, P., Feng, Y., Ma, X., Shen, Z., et al. (2023). Cu2+-Anchored Carbon nano-photocatalysts for visible water splitting to boost hydrogen cuproptosis. Angew. Chem. Int. Ed. 62 (44), e202311549. doi:10.1002/anie.202311549
Dixon, S. J., Lemberg, K. M., Lamprecht, M. R., Skouta, R., Zaitsev, E. M., Gleason, C. E., et al. (2012). Ferroptosis: an iron-dependent form of nonapoptotic cell death. Cell 149 (5), 1060–1072. doi:10.1016/j.cell.2012.03.042
Doll, S., Proneth, B., Tyurina, Y. Y., Panzilius, E., Kobayashi, S., Ingold, I., et al. (2017). ACSL4 dictates ferroptosis sensitivity by shaping cellular lipid composition. Nat. Chem. Biol. 13 (1), 91–98. doi:10.1038/nchembio.2239
Dong, X., Li, Y., Sheng, X., Zhou, W., Sun, A., and Dai, H. (2024). Mitochondria-related signaling pathways involved in breast cancer regulate ferroptosis. Genes. Dis. 11 (1), 358–366. doi:10.1016/j.gendis.2023.03.019
Du, C., Guo, X., Qiu, X., Jiang, W., Wang, X., An, H., et al. (2024). Self-reinforced bimetallic mito-jammer for Ca(2+) overload-mediated cascade mitochondrial damage for cancer cuproptosis sensitization. Adv. Sci. (Weinh) 11 (15), e2306031. doi:10.1002/advs.202306031
Eaton, J. W., and Qian, M. (2002). Molecular bases of cellular iron toxicity. Free Radic. Biol. Med. 32 (9), 833–840. doi:10.1016/s0891-5849(02)00772-4
Emad, B., WalyEldeen, A. A., Hassan, H., Sharaky, M., Abdelhamid, I. A., Ibrahim, S. A., et al. (2023). Yttrium Oxide nanoparticles induce cytotoxicity, genotoxicity, apoptosis, and ferroptosis in the human triple-negative breast cancer MDA-MB-231 cells. BMC cancer 23 (1), 1151. doi:10.1186/s12885-023-11649-w
Fan, D., Cao, Y., Cao, M., Wang, Y., Cao, Y., and Gong, T. (2023). Nanomedicine in cancer therapy. Signal Transduct. Target Ther. 8 (1), 293. doi:10.1038/s41392-023-01536-y
Fan, Y., Luo, C., Wang, Y., Wang, Z., Wang, C., Zhong, X., et al. (2023). A nomogram based on cuproptosis-related genes predicts 7-year relapse-free survival in patients with estrogen receptor-positive early breast cancer. Front. Oncol. 13, 1111480. doi:10.3389/fonc.2023.1111480
Fang, X., Ardehali, H., Min, J., and Wang, F. (2023). The molecular and metabolic landscape of iron and ferroptosis in cardiovascular disease. Nat. Rev. Cardiol. 20 (1), 7–23. doi:10.1038/s41569-022-00735-4
Fang, Y., Chen, X., Tan, Q., Zhou, H., Xu, J., and Gu, Q. (2021). Inhibiting ferroptosis through disrupting the NCOA4-FTH1 interaction: a new mechanism of action. ACS Cent. Sci. 7 (6), 980–989. doi:10.1021/acscentsci.0c01592
Farnie, G., and Clarke, R. B. (2007). Mammary stem cells and breast cancer--role of Notch signalling. Stem Cell Rev. 3 (2), 169–175. doi:10.1007/s12015-007-0023-5
Feng, A., He, L., Chen, T., and Xu, M. (2022). A novel cuproptosis-related lncRNA nomogram to improve the prognosis prediction of gastric cancer. Front. Oncol. 12, 957966. doi:10.3389/fonc.2022.957966
Feng, W., Xiao, Y., Zhao, C., Zhang, Z., Liu, W., Ma, J., et al. (2022). New deferric amine compounds efficiently chelate excess iron to treat iron overload disorders and to prevent ferroptosis. Adv. Sci. (Weinh) 9 (29), e2202679. doi:10.1002/advs.202202679
Forbes, A. (2009). Iron and parenteral nutrition. Gastroenterology 137 (5 Suppl. l), S47–S54. doi:10.1053/j.gastro.2009.08.013
Fu, B., Shao, R., Wang, H., Chen, G., Bai, S., and Wang, H. (2022). Integrated assessment of the clinical and biological value of ferroptosis-related genes in multiple myeloma. Cancer Cell Int. 22 (1), 326. doi:10.1186/s12935-022-02742-4
Gao, M., Monian, P., Pan, Q., Zhang, W., Xiang, J., and Jiang, X. (2016). Ferroptosis is an autophagic cell death process. Cell Res. 26 (9), 1021–1032. doi:10.1038/cr.2016.95
Gao, Y., Jin, F., Zhang, P., Zheng, C., Zheng, X., Xie, J., et al. (2024). Elesclomol-copper synergizes with imidazole ketone erastin by promoting cuproptosis and ferroptosis in myelodysplastic syndromes. Biomed. Pharmacother. 175, 116727. doi:10.1016/j.biopha.2024.116727
Ge, A., He, Q., Zhao, D., Li, Y., Chen, J., Deng, Y., et al. (2024). Mechanism of ferroptosis in breast cancer and research progress of natural compounds regulating ferroptosis. J. Cell Mol. Med. 28 (1), e18044. doi:10.1111/jcmm.18044
Ge, E. J., Bush, A. I., Casini, A., Cobine, P. A., Cross, J. R., DeNicola, G. M., et al. (2022). Connecting copper and cancer: from transition metal signalling to metalloplasia. Nat. Rev. Cancer 22 (2), 102–113. doi:10.1038/s41568-021-00417-2
Gohil, V. M. (2021). Repurposing elesclomol, an investigational drug for the treatment of copper metabolism disorders. Expert Opin. Investig. Drugs 30 (1), 1–4. doi:10.1080/13543784.2021.1840550
Gong, L., Zhang, Y., Liu, C., Zhang, M., and Han, S. (2021). Application of radiosensitizers in cancer radiotherapy. Int. J. nanomedicine 16, 1083–1102. doi:10.2147/IJN.S290438
Grasso, M., Bond, G. J., Kim, Y. J., Boyd, S., Matson Dzebo, M., Valenzuela, S., et al. (2021). The copper chaperone CCS facilitates copper binding to MEK1/2 to promote kinase activation. J. Biol. Chem. 297 (6), 101314. doi:10.1016/j.jbc.2021.101314
Guo, J., Cheng, J., Zheng, N., Zhang, X., Dai, X., Zhang, L., et al. (2021). Copper promotes tumorigenesis by activating the PDK1-AKT oncogenic pathway in a copper transporter 1 dependent manner. Adv. Sci. (Weinh) 8 (18), e2004303. doi:10.1002/advs.202004303
Guo, Q., Qiu, P., Pan, K., and Lin, J. (2022). Comprehensive analysis of cuproptosis-related long non-coding RNA signature and personalized therapeutic strategy of breast cancer patients. Front. Oncol. 12, 1081089. doi:10.3389/fonc.2022.1081089
Gururaja Rao, S. (2017). Mitochondrial changes in cancer. Handb. Exp. Pharmacol. 240, 211–227. doi:10.1007/164_2016_40
Guthrie, L. M., Soma, S., Yuan, S., Silva, A., Zulkifli, M., Snavely, T. C., et al. (2020). Elesclomol alleviates Menkes pathology and mortality by escorting Cu to cuproenzymes in mice. Science 368 (6491), 620–625. doi:10.1126/science.aaz8899
Han, D., Wu, G., Chang, C., Zhu, F., Xiao, Y., Li, Q., et al. (2015). Disulfiram inhibits TGF-β-induced epithelial-mesenchymal transition and stem-like features in breast cancer via ERK/NF-κB/Snail pathway. Oncotarget 6 (38), 40907–40919. doi:10.18632/oncotarget.5723
Hao, C., Huang, L., Zhang, H., Xu, L., Sun, M., Kuang, H., et al. (2024). Chiral CuxOS@ Fe-MOFs for enhanced cancer therapy. Adv. Funct. Mater. 34 (10), 2312795. doi:10.1002/adfm.202312795
Harrington, B. S., Ozaki, M. K., Caminear, M. W., Hernandez, L. F., Jordan, E., Kalinowski, N. J., et al. (2020). Drugs targeting tumor-initiating cells prolong survival in a post-surgery, post-chemotherapy ovarian cancer relapse model. Cancers (Basel) 12 (6), 1645. doi:10.3390/cancers12061645
Hasan, N. M., Gupta, A., Polishchuk, E., Yu, C. H., Polishchuk, R., Dmitriev, O. Y., et al. (2012). Molecular events initiating exit of a copper-transporting ATPase ATP7B from the trans-Golgi network. J. Biol. Chem. 287 (43), 36041–36050. doi:10.1074/jbc.M112.370403
Hassannia, B., Vandenabeele, P., and Vanden Berghe, T. (2019). Targeting ferroptosis to iron out cancer. Cancer Cell 35 (6), 830–849. doi:10.1016/j.ccell.2019.04.002
Hatori, Y., and Lutsenko, S. (2016). The role of copper chaperone Atox1 in coupling redox homeostasis to intracellular copper distribution. Antioxidants (Basel) 5 (3), 25. doi:10.3390/antiox5030025
He, T., Tang, Q., Ren, Q., Liu, Y., He, G., Pan, Y., et al. (2024). Different valence states of copper ion delivery against triple-negative breast cancer. ACS Nano 18 (7), 5434–5445. doi:10.1021/acsnano.3c10226
Hentze, M. W., Muckenthaler, M. U., Galy, B., and Camaschella, C. (2010). Two to tango: regulation of Mammalian iron metabolism. Cell 142 (1), 24–38. doi:10.1016/j.cell.2010.06.028
Heuberger, D. M., Wolint, P., Jang, J. H., Itani, S., Jungraithmayr, W., Waschkies, C. F., et al. (2022). High-affinity Cu(I)-Chelator with potential anti-tumorigenic action-A proof-of-principle experimental study of human H460 tumors in the CAM assay. Cancers (Basel) 14 (20), 5122. doi:10.3390/cancers14205122
Hirota, K. (2019). An intimate crosstalk between iron homeostasis and oxygen metabolism regulated by the hypoxia-inducible factors (HIFs). Free Radic. Biol. Med. 133, 118–129. doi:10.1016/j.freeradbiomed.2018.07.018
Hirschhorn, T., and Stockwell, B. R. (2019). The development of the concept of ferroptosis. Free Radic. Biol. Med. 133, 130–143. doi:10.1016/j.freeradbiomed.2018.09.043
Hu, K., Qiu, J., Hu, Y., Wang, Y., Yu, C., and Wu, Y. (2024). Efficacy of FERscore in predicting sensitivity to ferroptosis inducers in breast cancer. NPJ Breast Cancer 10 (1), 74. doi:10.1038/s41523-024-00685-9
Hu, X., He, Y., Han, Z., Liu, W., Liu, D., Zhang, X., et al. (2022). PNO1 inhibits autophagy-mediated ferroptosis by GSH metabolic reprogramming in hepatocellular carcinoma. Cell Death Dis. 13 (11), 1010. doi:10.1038/s41419-022-05448-7
Huang, H., Guo, H., Liu, J., Ni, C., Xia, L., Cao, X., et al. (2024). Dendrimer/metal-phenolic nanocomplexes encapsulating CuO2 for targeted magnetic resonance imaging and enhanced ferroptosis/cuproptosis/chemodynamic therapy by regulating the tumor microenvironment. Acta Biomater. 183, 252–263. doi:10.1016/j.actbio.2024.05.035
Huang, S., Xie, P., Huang, X., Chen, Z., Yang, J., Wang, J., et al. (2023). Disulfiram combined with chemoimmunotherapy potentiates pancreatic cancer treatment efficacy through the activation of cGAS-STING signaling pathway via suppressing PARP1 expression. Am. J. Cancer Res. 13 (5), 2055–2065. doi:10.3892/ijo.2022.5385
Huang, T., Liu, Y., Li, J., Shi, B., Shan, Z., Shi, Z., et al. (2022). Insights into prognosis and immune infiltration of cuproptosis-related genes in breast cancer. Front. Immunol. 13, 1054305. doi:10.3389/fimmu.2022.1054305
Huang, X. (2008). Does iron have a role in breast cancer? Lancet Oncol. 9 (8), 803–807. doi:10.1016/s1470-2045(08)70200-6
Huffman, D. L., and O'Halloran, T. V. (2001). Function, structure, and mechanism of intracellular copper trafficking proteins. Annu. Rev. Biochem. 70, 677–701. doi:10.1146/annurev.biochem.70.1.677
Hunsaker, E. W., and Franz, K. J. (2019). Emerging opportunities to manipulate metal trafficking for therapeutic benefit. Inorg. Chem. 58 (20), 13528–13545. doi:10.1021/acs.inorgchem.9b01029
Islam, S., Hoque, N., Nasrin, N., Hossain, M., Rizwan, F., Biswas, K., et al. (2022). Iron overload and breast cancer: iron chelation as a potential therapeutic approach. Life (Basel) 12 (7), 963. doi:10.3390/life12070963
Javadov, S., Kozlov, A. V., and Camara, A. K. S. (2020). Mitochondria in health and diseases. Cells 9 (5), 1177. doi:10.3390/cells9051177
Ji, P., Wang, P., Chen, H., Xu, Y., Ge, J., Tian, Z., et al. (2023). Potential of copper and copper compounds for anticancer applications. Pharm. (Basel) 16 (2), 234. doi:10.3390/ph16020234
Jia, C. L., Yang, F., and Li, R. (2021). Prognostic model construction and immune microenvironment analysis of breast cancer based on ferroptosis-related lncRNAs. Int. J. Gen. Med. 14, 9817–9831. doi:10.2147/ijgm.S342783
Jian, J., Yang, Q., Dai, J., Eckard, J., Axelrod, D., Smith, J., et al. (2011). Effects of iron deficiency and iron overload on angiogenesis and oxidative stress-a potential dual role for iron in breast cancer. Free Radic. Biol. Med. 50 (7), 841–847. doi:10.1016/j.freeradbiomed.2010.12.028
Jiang, B., Zhu, H., Feng, W., Wan, Z., Qi, X., He, R., et al. (2022). Database mining detected a cuproptosis-related prognostic signature and a related regulatory Axis in breast cancer. Dis. Markers 2022, 9004830. doi:10.1155/2022/9004830
Jiang, L., Gao, X.-M., and Cao, J. (2023). The achilles heel of TNBCs: ferroptosis heterogeneity. Cell metab. 35 (1), 1–2. doi:10.1016/j.cmet.2022.11.014
Jiang, L., Kon, N., Li, T., Wang, S. J., Su, T., Hibshoosh, H., et al. (2015). Ferroptosis as a p53-mediated activity during tumour suppression. Nature 520 (7545), 57–62. doi:10.1038/nature14344
Jiang, R., Manson, J. E., Meigs, J. B., Ma, J., Rifai, N., and Hu, F. B. (2004). Body iron stores in relation to risk of type 2 diabetes in apparently healthy women. Jama 291 (6), 711–717. doi:10.1001/jama.291.6.711
Jiang, X., Stockwell, B. R., and Conrad, M. (2021). Ferroptosis: mechanisms, biology and role in disease. Nat. Rev. Mol. Cell Biol. 22 (4), 266–282. doi:10.1038/s41580-020-00324-8
Jiang, Z., Lim, S.-O., Yan, M., Hsu, J. L., Yao, J., Wei, Y., et al. (2021). TYRO3 induces anti–PD-1/PD-L1 therapy resistance by limiting innate immunity and tumoral ferroptosis. J. Clin. investigation 131 (8), e139434. doi:10.1172/JCI139434
Jiang, Z., Sha, G., Zhang, W., Zhang, Z., Liu, T., Wang, D., et al. (2023). The huge potential of targeting copper status in the treatment of colorectal cancer. Clin. Transl. Oncol. 25 (7), 1977–1990. doi:10.1007/s12094-023-03107-7
Jiang, Z. R., Yang, L. H., Jin, L. Z., Yi, L. M., Bing, P. P., Zhou, J., et al. (2022). Identification of novel cuproptosis-related lncRNA signatures to predict the prognosis and immune microenvironment of breast cancer patients. Front. Oncol. 12, 988680. doi:10.3389/fonc.2022.988680
Jin, J., Ma, M., Shi, S., Wang, J., Xiao, P., Yu, H. F., et al. (2022). Copper enhances genotoxic drug resistance via ATOX1 activated DNA damage repair. Cancer Lett. 536, 215651. doi:10.1016/j.canlet.2022.215651
Jomova, K., Makova, M., Alomar, S. Y., Alwasel, S. H., Nepovimova, E., Kuca, K., et al. (2022). Essential metals in health and disease. Chem. Biol. Interact. 367, 110173. doi:10.1016/j.cbi.2022.110173
Kahlson, M. A., and Dixon, S. J. (2022). Copper-induced cell death. Science 375 (6586), 1231–1232. doi:10.1126/science.abo3959
Kciuk, M., Gielecińska, A., Kałuzińska-Kołat, Ż., Yahya, E. B., and Kontek, R. (2024). Ferroptosis and cuproptosis: metal-dependent cell death pathways activated in response to classical chemotherapy - significance for cancer treatment? Biochim. Biophys. Acta Rev. Cancer 1879 (4), 189124. doi:10.1016/j.bbcan.2024.189124
Kelley, K. C., Grossman, K. F., Brittain-Blankenship, M., Thorne, K. M., Akerley, W. L., Terrazas, M. C., et al. (2021). A Phase 1 dose-escalation study of disulfiram and copper gluconate in patients with advanced solid tumors involving the liver using S-glutathionylation as a biomarker. BMC cancer 21 (1), 510. doi:10.1186/s12885-021-08242-4
Kim, J. Y., Cho, Y., Oh, E., Lee, N., An, H., Sung, D., et al. (2016). Disulfiram targets cancer stem-like properties and the HER2/Akt signaling pathway in HER2-positive breast cancer. Cancer Lett. 379 (1), 39–48. doi:10.1016/j.canlet.2016.05.026
Kim, R., Hashimoto, A., Markosyan, N., Tyurin, V. A., Tyurina, Y. Y., Kar, G., et al. (2022). Ferroptosis of tumour neutrophils causes immune suppression in cancer. Nature 612 (7939), 338–346. doi:10.1038/s41586-022-05443-0
Kim, Y. J., Kim, J. Y., Lee, N., Oh, E., Sung, D., Cho, T. M., et al. (2017a). Disulfiram suppresses cancer stem-like properties and STAT3 signaling in triple-negative breast cancer cells. Biochem. Biophys. Res. Commun. 486 (4), 1069–1076. doi:10.1016/j.bbrc.2017.03.164
Kim, Y. J., Lee, N., Kim, Y. J., Cho, Y., An, H., Oh, E., et al. (2017b). Disulfiram induces anoikis and suppresses lung colonization in triple-negative breast cancer via calpain activation. Cancer Lett. 386, 151–160. doi:10.1016/j.canlet.2016.11.022
Klimenko, O. V. (2017). Small non-coding RNAs as regulators of structural evolution and carcinogenesis. Noncoding RNA Res. 2 (2), 88–92. doi:10.1016/j.ncrna.2017.06.002
Kohgo, Y., Ikuta, K., Ohtake, T., Torimoto, Y., and Kato, J. (2008). Body iron metabolism and pathophysiology of iron overload. Int. J. Hematol. 88, 7–15. doi:10.1007/s12185-008-0120-5
Koppula, P., Zhuang, L., and Gan, B. (2021). Cystine transporter SLC7A11/xCT in cancer: ferroptosis, nutrient dependency, and cancer therapy. Protein Cell 12 (8), 599–620. doi:10.1007/s13238-020-00789-5
Kuo, M. T., Fu, S., Savaraj, N., and Chen, H. H. (2012). Role of the human high-affinity copper transporter in copper homeostasis regulation and cisplatin sensitivity in cancer chemotherapy. Cancer Res. 72 (18), 4616–4621. doi:10.1158/0008-5472.Can-12-0888
Kuo, Y. M., Zhou, B., Cosco, D., and Gitschier, J. (2001). The copper transporter CTR1 provides an essential function in mammalian embryonic development. Proc. Natl. Acad. Sci. U. S. A. 98 (12), 6836–6841. doi:10.1073/pnas.111057298
Lafi, Z., Alshaer, W., Gharaibeh, L., Alqudah, D. A., AlQuaissi, B., Bashaireh, B., et al. (2023). Synergistic combination of doxorubicin with hydralazine, and disulfiram against MCF-7 breast cancer cell line. PLoS One 18 (9), e0291981. doi:10.1371/journal.pone.0291981
Lang, X., Green, M. D., Wang, W., Yu, J., Choi, J. E., Jiang, L., et al. (2019). Radiotherapy and immunotherapy promote tumoral lipid oxidation and ferroptosis via synergistic repression of SLC7A11. Cancer Discov. 9 (12), 1673–1685. doi:10.1158/2159-8290.CD-19-0338
Lee, S., Hwang, N., Seok, B. G., Lee, S., Lee, S. J., and Chung, S. W. (2023). Autophagy mediates an amplification loop during ferroptosis. Cell Death Dis. 14 (7), 464. doi:10.1038/s41419-023-05978-8
Lei, G., Zhang, Y., Koppula, P., Liu, X., Zhang, J., Lin, S. H., et al. (2020). The role of ferroptosis in ionizing radiation-induced cell death and tumor suppression. Cell Res. 30 (2), 146–162. doi:10.1038/s41422-019-0263-3
Lelièvre, P., Sancey, L., Coll, J. L., Deniaud, A., and Busser, B. (2020). The multifaceted roles of copper in cancer: a trace metal element with dysregulated metabolism, but also a target or a bullet for therapy. Cancers (Basel) 12 (12), 3594. doi:10.3390/cancers12123594
Levine, B., and Kroemer, G. (2019). Biological functions of autophagy genes: a disease perspective. Cell 176 (1-2), 11–42. doi:10.1016/j.cell.2018.09.048
Li, D., and Li, Y. (2020). The interaction between ferroptosis and lipid metabolism in cancer. Signal Transduct. Target. Ther. 5 (1), 108. doi:10.1038/s41392-020-00216-5
Li, D., Wu, X., Fan, X., Cheng, C., Li, D., and Zhang, W. (2022). Comprehensive analysis of cuproptosis-related lncRNAs in the prognosis and therapy response of patients with bladder cancer. Ann. Transl. Med. 10 (22), 1232. doi:10.21037/atm-22-5294
Li, F., Yang, Y., Zhang, X., Yu, J., and Yu, Y. (2024). A novel prognostic model of breast cancer based on cuproptosis-related lncRNAs. Discov. Oncol. 15 (1), 35. doi:10.1007/s12672-024-00888-3
Li, H., Li, L., Xue, C., Huang, R., Hu, A., An, X., et al. (2021). A novel ferroptosis-related gene signature predicts overall survival of breast cancer patients. Biol. (Basel) 10 (2), 151. doi:10.3390/biology10020151
Li, J., Li, P. T., Wu, W., Ding, B. N., Wen, Y. G., Cai, H. L., et al. (2024). POU2F2-mediated upregulation of lncRNA PTPRG-AS1 inhibits ferroptosis in breast cancer via miR-376c-3p/SLC7A11 axis. Epigenomics 16 (4), 215–231. doi:10.2217/epi-2023-0100
Li, J., Liu, J., Li, J., Feng, A., Nie, Y., Yang, Z., et al. (2023a). A risk prognostic model for patients with esophageal squamous cell carcinoma basing on cuproptosis and ferroptosis. J. Cancer Res. Clin. Oncol. 149 (13), 11647–11659. doi:10.1007/s00432-023-05005-5
Li, J., Liu, J., Xu, Y., Wu, R., Chen, X., Song, X., et al. (2021). Tumor heterogeneity in autophagy-dependent ferroptosis. Autophagy 17 (11), 3361–3374. doi:10.1080/15548627.2021.1872241
Li, J., Wu, F., Li, C., Sun, S., Feng, C., Wu, H., et al. (2022). The cuproptosis-related signature predicts prognosis and indicates immune microenvironment in breast cancer. Front. Genet. 13, 977322. doi:10.3389/fgene.2022.977322
Li, J., Zhang, W., Ma, X., Wei, Y., Zhou, F., Li, J., et al. (2023b). Cuproptosis/ferroptosis-related gene signature is correlated with immune infiltration and predict the prognosis for patients with breast cancer. Front. Pharmacol. 14, 1192434. doi:10.3389/fphar.2023.1192434
Li, K., Lin, C., Li, M., Xu, K., He, Y., Mao, Y., et al. (2022). Multienzyme-like reactivity cooperatively impairs glutathione peroxidase 4 and ferroptosis suppressor protein 1 pathways in triple-negative breast cancer for sensitized ferroptosis therapy. ACS Nano 16 (2), 2381–2398. doi:10.1021/acsnano.1c08664
Li, L., Li, L., and Sun, Q. (2022). High expression of cuproptosis-related SLC31A1 gene in relation to unfavorable outcome and deregulated immune cell infiltration in breast cancer: an analysis based on public databases. BMC Bioinforma. 23 (1), 350. doi:10.1186/s12859-022-04894-6
Li, L., Zhou, H., and Zhang, C. (2024). Cuproptosis in cancer: biological implications and therapeutic opportunities. Cell. and Mol. Biol. Lett. 29 (1), 91. doi:10.1186/s11658-024-00608-3
Li, P., Sun, Q., Bai, S., Wang, H., and Zhao, L. (2023). Combination of the cuproptosis inducer disulfiram and anti-PD-L1 abolishes NSCLC resistance by ATP7B to regulate the HIF-1 signaling pathway. Int. J. Mol. Med. 53 (2), 19. doi:10.3892/ijmm.2023.5343
Li, Q., Liu, H., Jin, Y., Yu, Y., Wang, Y., Wu, D., et al. (2023). Analysis of a new therapeutic target and construction of a prognostic model for breast cancer based on ferroptosis genes. Comput. Biol. Med. 165, 107370. doi:10.1016/j.compbiomed.2023.107370
Li, W., Zhang, X., Chen, Y., and Pang, D. (2022). Identification of cuproptosis-related patterns and construction of a scoring system for predicting prognosis, tumor microenvironment-infiltration characteristics, and immunotherapy efficacy in breast cancer. Front. Oncol. 12, 966511. doi:10.3389/fonc.2022.966511
Li, X., Ma, Z., and Mei, L. (2022). Cuproptosis-related gene SLC31A1 is a potential predictor for diagnosis, prognosis and therapeutic response of breast cancer. Am. J. Cancer Res. 12 (8), 3561–3580.
Li, Y., Cao, Y., Xiao, J., Shang, J., Tan, Q., Ping, F., et al. (2020). Inhibitor of apoptosis-stimulating protein of p53 inhibits ferroptosis and alleviates intestinal ischemia/reperfusion-induced acute lung injury. Cell Death Differ. 27 (9), 2635–2650. doi:10.1038/s41418-020-0528-x
Li, Y., Chen, H., Liao, J., Chen, K., Javed, M. T., Qiao, N., et al. (2021). Long-term copper exposure promotes apoptosis and autophagy by inducing oxidative stress in pig testis. Environ. Sci. Pollut. Res. Int. 28 (39), 55140–55153. doi:10.1007/s11356-021-14853-y
Li, Y., Li, T., Zhai, D., Xie, C., Kuang, X., Lin, Y., et al. (2022). Quantification of ferroptosis pathway status revealed heterogeneity in breast cancer patients with distinct immune microenvironment. Front. Oncol. 12, 956999. doi:10.3389/fonc.2022.956999
Li, Y., and Li, Z. (2021). Potential mechanism underlying the role of mitochondria in breast cancer drug resistance and its related treatment prospects. Front. Oncol. 11, 629614. doi:10.3389/fonc.2021.629614
Li, Y., Liu, J., Chen, Y., Weichselbaum, R. R., and Lin, W. (2024). Nanoparticles synergize ferroptosis and cuproptosis to potentiate cancer immunotherapy. Adv. Sci. 11, 2310309. doi:10.1002/advs.202310309
Li, Y., Na, F., and Pei, J. (2023). Construction and characterization of a cuproptosis- and immune checkpoint-based LncRNAs signature for breast cancer risk stratification. Breast Cancer 30 (3), 393–411. doi:10.1007/s12282-023-01434-9
Li, Z., Chen, L., Chen, C., Zhou, Y., Hu, D., Yang, J., et al. (2020). Targeting ferroptosis in breast cancer. Biomark. Res. 8 (1), 58. doi:10.1186/s40364-020-00230-3
Li, Z., Zhang, H., Wang, X., Wang, Q., Xue, J., Shi, Y., et al. (2022). Identification of cuproptosis-related subtypes, characterization of tumor microenvironment infiltration, and development of a prognosis model in breast cancer. Front. Immunol. 13, 996836. doi:10.3389/fimmu.2022.996836
Liang, C., Zhang, X., Yang, M., and Dong, X. (2019). Recent progress in ferroptosis inducers for cancer therapy. Adv. Mater 31 (51), e1904197. doi:10.1002/adma.201904197
Liang, D., Minikes, A. M., and Jiang, X. (2022). Ferroptosis at the intersection of lipid metabolism and cellular signaling. Mol. Cell 82 (12), 2215–2227. doi:10.1016/j.molcel.2022.03.022
Liang, W., and Ferrara, N. (2020). Iron metabolism in the tumor microenvironment: contributions of innate immune cells. Front. Immunol. 11, 626812. doi:10.3389/fimmu.2020.626812
Liao, P., Wang, W., Wang, W., Kryczek, I., Li, X., Bian, Y., et al. (2022). CD8+ T cells and fatty acids orchestrate tumor ferroptosis and immunity via ACSL4. Cancer Cell 40 (4), 365–378.e6. doi:10.1016/j.ccell.2022.02.003
Lill, R., and Freibert, S. A. (2020). Mechanisms of mitochondrial iron-sulfur protein biogenesis. Annu. Rev. Biochem. 89, 471–499. doi:10.1146/annurev-biochem-013118-111540
Lin, C., He, J., Tong, X., and Song, L. (2023). Copper homeostasis-associated gene PRNP regulates ferroptosis and immune infiltration in breast cancer. PLoS One 18 (8), e0288091. doi:10.1371/journal.pone.0288091
Ling, Y. Y., Wang, W. J., Hao, L., Wu, X. W., Liang, J. H., Zhang, H., et al. (2022). Self-amplifying iridium (III) photosensitizer for ferroptosis-mediated immunotherapy against transferrin receptor-overexpressing cancer. Small 18 (49), 2203659. doi:10.1002/smll.202203659
Lippard, S. J. (1999). Free copper ions in the cell? Science 284 (5415), 748–749. doi:10.1126/science.284.5415.748
Liu, J., Kuang, F., Kroemer, G., Klionsky, D. J., Kang, R., and Tang, D. (2020). Autophagy-dependent ferroptosis: machinery and regulation. Cell Chem. Biol. 27 (4), 420–435. doi:10.1016/j.chembiol.2020.02.005
Liu, J., Liu, Y., Wang, Y., Li, C., Xie, Y., Klionsky, D. J., et al. (2023). TMEM164 is a new determinant of autophagy-dependent ferroptosis. Autophagy 19 (3), 945–956. doi:10.1080/15548627.2022.2111635
Liu, N., and Chen, M. (2024). Crosstalk between ferroptosis and cuproptosis: from mechanism to potential clinical application. Biomed. Pharmacother. 171, 116115. doi:10.1016/j.biopha.2023.116115
Liu, S., Zhao, Y., Zhang, J., and Liu, Z. (2023). Application of single-cell RNA sequencing analysis of novel breast cancer phenotypes based on the activation of ferroptosis-related genes. Funct. Integr. Genomics 23 (2), 173. doi:10.1007/s10142-023-01086-0
Liu, S. Q., Gao, Z. J., Wu, J., Zheng, H. M., Li, B., Sun, S., et al. (2022). Single-cell and spatially resolved analysis uncovers cell heterogeneity of breast cancer. J. Hematol. Oncol. 15 (1), 19. doi:10.1186/s13045-022-01236-0
Liu, T., Zhou, Z., Zhang, M., Lang, P., Li, J., Liu, Z., et al. (2023). Cuproptosis-immunotherapy using PD-1 overexpressing T cell membrane-coated nanosheets efficiently treats tumor. J. Control. Release 362, 502–512. doi:10.1016/j.jconrel.2023.08.055
Liu, X., Luo, B., Wu, X., and Tang, Z. (2023). Cuproptosis and cuproptosis-related genes: emerging potential therapeutic targets in breast cancer. Biochim. Biophys. Acta Rev. Cancer 1878 (6), 189013. doi:10.1016/j.bbcan.2023.189013
Liu, Y., Hu, Y., Jiang, Y., Bu, J., and Gu, X. (2022). Targeting ferroptosis, the achilles' heel of breast cancer: a review. Front. Pharmacol. 13, 1036140. doi:10.3389/fphar.2022.1036140
Liu, Y., Niu, R., Zhao, H., Wang, Y., Song, S., Zhang, H., et al. (2024). Single-site nanozymes with a highly conjugated coordination structure for antitumor immunotherapy via cuproptosis and cascade-enhanced T lymphocyte activity. J. Am. Chem. Soc. 146 (6), 3675–3688. doi:10.1021/jacs.3c08622
Lorito, N., Subbiani, A., Smiriglia, A., Bacci, M., Bonechi, F., Tronci, L., et al. (2024). FADS1/2 control lipid metabolism and ferroptosis susceptibility in triple-negative breast cancer. EMBO Mol. Med. 16 (7), 1533–1559. doi:10.1038/s44321-024-00090-6
Louandre, C., Ezzoukhry, Z., Godin, C., Barbare, J. C., Mazière, J. C., Chauffert, B., et al. (2013). Iron-dependent cell death of hepatocellular carcinoma cells exposed to sorafenib. Int. J. Cancer 133 (7), 1732–1742. doi:10.1002/ijc.28159
Lu, C., Li, X., Ren, Y., and Zhang, X. (2021). Disulfiram: a novel repurposed drug for cancer therapy. Cancer Chemother. Pharmacol. 87 (2), 159–172. doi:10.1007/s00280-020-04216-8
Lu, S., Tian, H., Li, B., Li, L., Jiang, H., Gao, Y., et al. (2024). An ellagic acid coordinated copper-based nanoplatform for efficiently overcoming cancer chemoresistance by cuproptosis and synergistic inhibition of cancer cell stemness. Small 20 (17), 2309215. doi:10.1002/smll.202309215
Lu, Y. J., Gong, Y., Li, W. J., Zhao, C. Y., and Guo, F. (2022). The prognostic significance of a novel ferroptosis-related gene model in breast cancer. Ann. Transl. Med. 10 (4), 184. doi:10.21037/atm-22-479
Luis, G., Godfroid, A., Nishiumi, S., Cimino, J., Blacher, S., Maquoi, E., et al. (2021). Tumor resistance to ferroptosis driven by Stearoyl-CoA Desaturase-1 (SCD1) in cancer cells and Fatty Acid Biding Protein-4 (FABP4) in tumor microenvironment promote tumor recurrence. Redox Biol. 43, 102006. doi:10.1016/j.redox.2021.102006
Lun, X., Wells, J. C., Grinshtein, N., King, J. C., Hao, X., Dang, N.-H., et al. (2016). Disulfiram when combined with copper enhances the therapeutic effects of temozolomide for the treatment of glioblastoma. Clin. Cancer Res. 22 (15), 3860–3875. doi:10.1158/1078-0432.CCR-15-1798
Luo, M., Fu, A., Wu, R., Wei, N., Song, K., Lim, S., et al. (2022). High expression of G6PD increases doxorubicin resistance in triple negative breast cancer cells by maintaining GSH level. Int. J. Biol. Sci. 18 (3), 1120–1133. doi:10.7150/ijbs.65555
Luo, Y., Yan, P., Li, X., Hou, J., Wang, Y., and Zhou, S. (2021). pH-sensitive polymeric vesicles for GOx/BSO delivery and synergetic starvation-ferroptosis therapy of tumor. Biomacromolecules 22 (10), 4383–4394. doi:10.1021/acs.biomac.1c00960
Ma, M., Kong, P., Huang, Y., Wang, J., Liu, X., Hu, Y., et al. (2022). Activation of MAT2A-ACSL3 pathway protects cells from ferroptosis in gastric cancer. Free Radic. Biol. Med. 181, 288–299. doi:10.1016/j.freeradbiomed.2022.02.015
Ma, S., Dielschneider, R. F., Henson, E. S., Xiao, W., Choquette, T. R., Blankstein, A. R., et al. (2017). Ferroptosis and autophagy induced cell death occur independently after siramesine and lapatinib treatment in breast cancer cells. PLoS One 12 (8), e0182921. doi:10.1371/journal.pone.0182921
MacDonald, R. S. (2000). The role of zinc in growth and cell proliferation. J. Nutr. 130 (Suppl. l), 1500S–1508s. doi:10.1093/jn/130.5.1500S
Magtanong, L., Ko, P. J., To, M., Cao, J. Y., Forcina, G. C., Tarangelo, A., et al. (2019). Exogenous monounsaturated fatty acids promote a ferroptosis-resistant cell state. Cell Chem. Biol. 26 (3), 420–432. doi:10.1016/j.chembiol.2018.11.016
Mao, C., Wang, M., Zhuang, L., and Gan, B. (2024). Metabolic cell death in cancer: ferroptosis, cuproptosis, disulfidptosis, and beyond. Protein Cell 15, 642–660. doi:10.1093/procel/pwae003
Mao, X., Xu, J., Wang, W., Liang, C., Hua, J., Liu, J., et al. (2021). Crosstalk between cancer-associated fibroblasts and immune cells in the tumor microenvironment: new findings and future perspectives. Mol. cancer 20, 131–230. doi:10.1186/s12943-021-01428-1
Marques, O., da Silva, B. M., Porto, G., and Lopes, C. (2014). Iron homeostasis in breast cancer. Cancer Lett. 347 (1), 1–14. doi:10.1016/j.canlet.2014.01.029
Martelotto, L. G., Ng, C. K., Piscuoglio, S., Weigelt, B., and Reis-Filho, J. S. (2014). Breast cancer intra-tumor heterogeneity. Breast Cancer Res. 16 (3), 210. doi:10.1186/bcr3658
Martínez-Sáez, O., Chic, N., Pascual, T., Adamo, B., Vidal, M., González-Farré, B., et al. (2020). Frequency and spectrum of PIK3CA somatic mutations in breast cancer. Breast Cancer Res. 22 (1), 45. doi:10.1186/s13058-020-01284-9
Mattick, J. S., Amaral, P. P., Carninci, P., Carpenter, S., Chang, H. Y., Chen, L.-L., et al. (2023). Long non-coding RNAs: definitions, functions, challenges and recommendations. Nat. Rev. Mol. Cell Biol. 24 (6), 430–447. doi:10.1038/s41580-022-00566-8
Menshawey, R., Menshawey, E., Alserr, A. H. K., and Abdelmassih, A. F. (2020). Low iron mitigates viral survival: insights from evolution, genetics, and pandemics-a review of current hypothesis. Egypt J. Med. Hum. Genet. 21 (1), 75. doi:10.1186/s43042-020-00114-z
Mizushima, N., and Komatsu, M. (2011). Autophagy: renovation of cells and tissues. Cell 147 (4), 728–741. doi:10.1016/j.cell.2011.10.026
Mosele, F., Stefanovska, B., Lusque, A., Tran Dien, A., Garberis, I., Droin, N., et al. (2020). Outcome and molecular landscape of patients with PIK3CA-mutated metastatic breast cancer. Ann. Oncol. 31 (3), 377–386. doi:10.1016/j.annonc.2019.11.006
Mou, Y., Wang, J., Wu, J., He, D., Zhang, C., Duan, C., et al. (2019). Ferroptosis, a new form of cell death: opportunities and challenges in cancer. J. Hematol. and Oncol. 12, 34–16. doi:10.1186/s13045-019-0720-y
Mukherjee, S., Sawant, A. V., Prassanawar, S. S., and Panda, D. (2023). Copper-plumbagin complex produces potent anticancer effects by depolymerizing microtubules and inducing reactive oxygen species and DNA damage. ACS Omega 8 (3), 3221–3235. doi:10.1021/acsomega.2c06691
Muñoz, M., García-Erce, J. A., and Remacha, A. F. (2011b). Disorders of iron metabolism. Part 1: molecular basis of iron homoeostasis. J. Clin. Pathol. 64 (4), 281–286. doi:10.1136/jcp.2010.079046
Muñoz, M., García-Erce, J. A., and Remacha Á, F. (2011a). Disorders of iron metabolism. Part II: iron deficiency and iron overload. J. Clin. Pathol. 64 (4), 287–296. doi:10.1136/jcp.2010.086991
Nagini, S. (2017). Breast cancer: current molecular therapeutic targets and new players. Anti-Cancer Agents Med. Chem. (Former. Curr. Med. Chemistry-Anti-Cancer Agents) 17 (2), 152–163. doi:10.2174/1871520616666160502122724
Navrátilová, J., Hankeová, T., Beneš, P., and Šmarda, J. (2013). Acidic pH of tumor microenvironment enhances cytotoxicity of the disulfiram/Cu2+ complex to breast and colon cancer cells. Chemotherapy 59 (2), 112–120. doi:10.1159/000353915
Nechushtan, H., Hamamreh, Y., Nidal, S., Gotfried, M., Baron, A., Shalev, Y. I., et al. (2015). A phase IIb trial assessing the addition of disulfiram to chemotherapy for the treatment of metastatic non-small cell lung cancer. Oncologist 20 (4), 366–367. doi:10.1634/theoncologist.2014-0424
Nelson, R. L. (1992). Dietary iron and colorectal cancer risk. Free Radic. Biol. Med. 12 (2), 161–168. doi:10.1016/0891-5849(92)90010-e
Ni, Y. L., Chien, P. J., Hsieh, H. C., Shen, H. T., Lee, H. T., Chen, S. M., et al. (2022). Disulfiram/copper suppresses cancer stem cell activity in differentiated thyroid cancer cells by inhibiting BMI1 expression. Int. J. Mol. Sci. 23 (21), 13276. doi:10.3390/ijms232113276
O'Day, S., Gonzalez, R., Lawson, D., Weber, R., Hutchins, L., Anderson, C., et al. (2009). Phase II, randomized, controlled, double-blinded trial of weekly elesclomol plus paclitaxel versus paclitaxel alone for stage IV metastatic melanoma. J. Clin. Oncol. 27 (32), 5452–5458. doi:10.1200/jco.2008.17.1579
O'Day, S. J., Eggermont, A. M., Chiarion-Sileni, V., Kefford, R., Grob, J. J., Mortier, L., et al. (2013). Final results of phase III SYMMETRY study: randomized, double-blind trial of elesclomol plus paclitaxel versus paclitaxel alone as treatment for chemotherapy-naive patients with advanced melanoma. J. Clin. Oncol. 31 (9), 1211–1218. doi:10.1200/jco.2012.44.5585
Pagès, A., Dotu, I., Pallarès-Albanell, J., Martí, E., Guigó, R., and Eyras, E. (2018). The discovery potential of RNA processing profiles. Nucleic Acids Res. 46 (3), e15. doi:10.1093/nar/gkx1115
Palumaa, P., Kangur, L., Voronova, A., and Sillard, R. (2004). Metal-binding mechanism of Cox17, a copper chaperone for cytochrome c oxidase. Biochem. J. 382 (Pt 1), 307–314. doi:10.1042/bj20040360
Pan, Y., Zhang, Q., Zhang, H., and Kong, F. (2023). Prognostic and immune microenvironment analysis of cuproptosis-related LncRNAs in breast cancer. Funct. Integr. Genomics 23 (1), 38. doi:10.1007/s10142-023-00963-y
Pardini, B., and Dragomir, M. P. (2021). SCIRT lncRNA blocks the shot of breast cancer cells self-renewal mechanism. Cancer Res. 81 (3), 535–536. doi:10.1158/0008-5472.Can-20-3903
Park, S. Y., Jeong, K. J., Poire, A., Zhang, D., Tsang, Y. H., Blucher, A. S., et al. (2023). Irreversible HER2 inhibitors overcome resistance to the RSL3 ferroptosis inducer in non-HER2 amplified luminal breast cancer. Cell Death and Dis. 14 (8), 532. doi:10.1038/s41419-023-06042-1
Passaro, A., Al Bakir, M., Hamilton, E. G., Diehn, M., André, F., Roy-Chowdhuri, S., et al. (2024). Cancer biomarkers: emerging trends and clinical implications for personalized treatment. Cell 187 (7), 1617–1635. doi:10.1016/j.cell.2024.02.041
Paul, B. T., Manz, D. H., Torti, F. M., and Torti, S. V. (2017). Mitochondria and Iron: current questions. Expert Rev. Hematol. 10 (1), 65–79. doi:10.1080/17474086.2016.1268047
Pavithra, V., Sathisha, T. G., Kasturi, K., Mallika, D. S., Amos, S. J., and Ragunatha, S. (2015). Serum levels of metal ions in female patients with breast cancer. J. Clin. Diagn Res. 9 (1), BC25–c27. doi:10.7860/jcdr/2015/11627.5476
Pinnix, Z. K., Miller, L. D., Wang, W., D'Agostino, R., Kute, T., Willingham, M. C., et al. (2010). Ferroportin and iron regulation in breast cancer progression and prognosis. Sci. Transl. Med. 2 (43), 43ra56. doi:10.1126/scisignal.3001127
Porporato, P. E., Filigheddu, N., Pedro, J. M. B., Kroemer, G., and Galluzzi, L. (2018). Mitochondrial metabolism and cancer. Cell Res. 28 (3), 265–280. doi:10.1038/cr.2017.155
Prá, D., Franke, S. I., Henriques, J. A., and Fenech, M. (2012). Iron and genome stability: an update. Mutat. Res. 733 (1-2), 92–99. doi:10.1016/j.mrfmmm.2012.02.001
Prasad, A. S. (2014). Zinc: an antioxidant and anti-inflammatory agent: role of zinc in degenerative disorders of aging. J. Trace Elem. Med. Biol. 28 (4), 364–371. doi:10.1016/j.jtemb.2014.07.019
Prasad Panda, S., and Kesharwani, A. (2023). Micronutrients/miRs/ATP networking in mitochondria: clinical intervention with ferroptosis, cuproptosis, and calcium burden. Mitochondrion 71, 1–16. doi:10.1016/j.mito.2023.05.003
Qi, X., Wan, Z., Jiang, B., Ouyang, Y., Feng, W., Zhu, H., et al. (2022). Inducing ferroptosis has the potential to overcome therapy resistance in breast cancer. Front. Immunol. 13, 1038225. doi:10.3389/fimmu.2022.1038225
Qiao, J., Chen, Y., Mi, Y., Jin, H., Huang, T., Liu, L., et al. (2020). NR5A2 synergizes with NCOA3 to induce breast cancer resistance to BET inhibitor by upregulating NRF2 to attenuate ferroptosis. Biochem. Biophys. Res. Commun. 530 (2), 402–409. doi:10.1016/j.bbrc.2020.05.069
Qiao, L., Zhu, G., Jiang, T., Qian, Y., Sun, Q., Zhao, G., et al. (2024). Self-destructive copper carriers induce pyroptosis and cuproptosis for efficient tumor immunotherapy against dormant and recurrent tumors. Adv. Mater. 36 (8), 2308241. doi:10.1002/adma.202308241
Ramchandani, D., Berisa, M., Tavarez, D. A., Li, Z., Miele, M., Bai, Y., et al. (2021). Copper depletion modulates mitochondrial oxidative phosphorylation to impair triple negative breast cancer metastasis. Nat. Commun. 12 (1), 7311. doi:10.1038/s41467-021-27559-z
Rao, Z., Xia, Y., Jia, Q., Zhu, Y., Wang, L., Liu, G., et al. (2023). Iron-based metal-organic framework co-loaded with buthionine sulfoximine and oxaliplatin for enhanced cancer chemo-ferrotherapy via sustainable glutathione elimination. J. nanobiotechnology 21 (1), 265. doi:10.1186/s12951-023-01998-w
Rappa, G., Gamcsik, M. P., Mitina, R. L., Baum, C., Fodstad, O., and Lorico, A. (2003). Retroviral transfer of MRP1 and gamma-glutamyl cysteine synthetase modulates cell sensitivity to L-buthionine-S,R-sulphoximine (BSO): new rationale for the use of BSO in cancer therapy. Eur. J. Cancer 39 (1), 120–128. doi:10.1016/s0959-8049(02)00447-1
Ren, X., Li, Y., Zhou, Y., Hu, W., Yang, C., Jing, Q., et al. (2021). Overcoming the compensatory elevation of NRF2 renders hepatocellular carcinoma cells more vulnerable to disulfiram/copper-induced ferroptosis. Redox Biol. 46, 102122. doi:10.1016/j.redox.2021.102122
Rishi, G., Huang, G., and Subramaniam, V. N. (2021). Cancer: the role of iron and ferroptosis. Int. J. Biochem. Cell Biol. 141, 106094. doi:10.1016/j.biocel.2021.106094
Rowland, E. A., Snowden, C. K., and Cristea, I. M. (2018). Protein lipoylation: an evolutionarily conserved metabolic regulator of health and disease. Curr. Opin. Chem. Biol. 42, 76–85. doi:10.1016/j.cbpa.2017.11.003
Rufaida Mustafa Ahmed, M., and Nazik Elmalaika Obaid Seid Ahmed, H. (2017). Changes in serum iron, total iron binding capacity and transferrin saturation percent in Sudanese females newly diagnosed with breast cancer at khartoum oncology hospital: a case-control study. Sudan J. Med. Sci. (SJMS) 12 (3), 119–132. doi:10.18502/sjms.v12i3.915
Russell, S. J., Zhao, C., Biondic, S., Menezes, K., Hagemann-Jensen, M., Librach, C. L., et al. (2024). An atlas of small non-coding RNAs in human preimplantation development. Nat. Commun. 15 (1), 8634. doi:10.1038/s41467-024-52943-w
Sang, M., Luo, R., Bai, Y., Dou, J., Zhang, Z., Liu, F., et al. (2019). Mitochondrial membrane anchored photosensitive nano-device for lipid hydroperoxides burst and inducing ferroptosis to surmount therapy-resistant cancer. Theranostics 9 (21), 6209–6223. doi:10.7150/thno.36283
Schaue, D., and McBride, W. H. (2015). Opportunities and challenges of radiotherapy for treating cancer. Nat. Rev. Clin. Oncol. 12 (9), 527–540. doi:10.1038/nrclinonc.2015.120
Scheurer, T., Steffens, J., Markert, A., Du Marchie Sarvaas, M., Roderburg, C., Rink, L., et al. (2022). The human long noncoding RNAs CoroMarker, MALAT1, CDR1as, and LINC00460 in whole blood of individuals after controlled short-term exposure with ultrafine metal fume particles at workplace conditions, and in human macrophages in vitro. J. Occup. Med. Toxicol. 17 (1), 15. doi:10.1186/s12995-022-00356-0
Schmidt, K., Ralle, M., Schaffer, T., Jayakanthan, S., Bari, B., Muchenditsi, A., et al. (2018). ATP7A and ATP7B copper transporters have distinct functions in the regulation of neuronal dopamine-β-hydroxylase. J. Biol. Chem. 293 (52), 20085–20098. doi:10.1074/jbc.RA118.004889
Schneider, S. A., and Bhatia, K. P. (2013). Excess iron harms the brain: the syndromes of neurodegeneration with brain iron accumulation (NBIA). J. Neural Transm. (Vienna) 120 (4), 695–703. doi:10.1007/s00702-012-0922-8
Scimeca, M., and Bonanno, E. (2018). New highlight in breast cancer development: the key role of hepcidin and iron metabolism. Ann. Transl. Med. 6 (Suppl. 1), S56. doi:10.21037/atm.2018.10.30
Seebacher, N., Lane, D. J., Richardson, D. R., and Jansson, P. J. (2016). Turning the gun on cancer: utilizing lysosomal P-glycoprotein as a new strategy to overcome multi-drug resistance. Free Radic. Biol. Med. 96, 432–445. doi:10.1016/j.freeradbiomed.2016.04.201
Sempos, C. T., Looker, A. C., Gillum, R. F., and Makuc, D. M. (1994). Body iron stores and the risk of coronary heart disease. N. Engl. J. Med. 330 (16), 1119–1124. doi:10.1056/nejm199404213301604
Sha, R., Dong, X., Yan, S., Dai, H., Sun, A., You, L., et al. (2024). Cuproptosis-related genes predict prognosis and trastuzumab therapeutic response in HER2-positive breast cancer. Sci. Rep. 14 (1), 2908. doi:10.1038/s41598-024-52638-8
Sha, R., Xu, Y., Yuan, C., Sheng, X., Wu, Z., Peng, J., et al. (2021). Predictive and prognostic impact of ferroptosis-related genes ACSL4 and GPX4 on breast cancer treated with neoadjuvant chemotherapy. EBioMedicine 71, 103560. doi:10.1016/j.ebiom.2021.103560
Sha, S., Si, L., Wu, X., Chen, Y., Xiong, H., Xu, Y., et al. (2022). Prognostic analysis of cuproptosis-related gene in triple-negative breast cancer. Front. Immunol. 13, 922780. doi:10.3389/fimmu.2022.922780
Shanbhag, V. C., Gudekar, N., Jasmer, K., Papageorgiou, C., Singh, K., and Petris, M. J. (2021). Copper metabolism as a unique vulnerability in cancer. Biochim. Biophys. Acta Mol. Cell Res. 1868 (2), 118893. doi:10.1016/j.bbamcr.2020.118893
Shen, L., He, Y., Fang, C., Qiu, H., Chen, Q., Huang, F., et al. (2022). Cuproptosis-associated genes and immune microenvironment characterization in breast cancer. Med. Baltim. 101 (50), e32301. doi:10.1097/md.0000000000032301
Shen, S., Yang, D., Yang, Y., Chen, Y., Xiong, J., and Hu, X. (2022). A novel prognostic ferroptosis-related lncRNA signature associated with immune landscape in invasive breast cancer. Dis. Markers 2022, 9168556. doi:10.1155/2022/9168556
Shen, Y., Li, D., Liang, Q., Yang, M., Pan, Y., and Li, H. (2022). Cross-talk between cuproptosis and ferroptosis regulators defines the tumor microenvironment for the prediction of prognosis and therapies in lung adenocarcinoma. Front. Immunol. 13, 1029092. doi:10.3389/fimmu.2022.1029092
Shi, B., Zhang, W., Wang, T., and Cui, Z. (2023). The therapeutic and prognostic role of cuproptosis-related genes in triple negative breast cancer. BMC Bioinforma. 24 (1), 223. doi:10.1186/s12859-023-05348-3
Shi, J. Y., Che, X., Wen, R., Hou, S. J., Xi, Y. J., Feng, Y. Q., et al. (2024). Ferroptosis biomarkers predict tumor mutation burden's impact on prognosis in HER2-positive breast cancer. World J. Clin. Oncol. 15 (3), 391–410. doi:10.5306/wjco.v15.i3.391
Shivnani, P., Shekhawat, S., and Prajapati, A. (2023). Cancer Cachexia and breast cancer stem cell signalling - a crosstalk of signalling molecules. Cell Signal 110, 110847. doi:10.1016/j.cellsig.2023.110847
Sirhan, Z., Thyagarajan, A., and Sahu, R. P. (2022). The efficacy of tucatinib-based therapeutic approaches for HER2-positive breast cancer. Mil. Med. Res. 9 (1), 39. doi:10.1186/s40779-022-00401-3
Skrott, Z., Mistrik, M., Andersen, K. K., Friis, S., Majera, D., Gursky, J., et al. (2017). Alcohol-abuse drug disulfiram targets cancer via p97 segregase adaptor NPL4. Nature 552 (7684), 194–199. doi:10.1038/nature25016
Smal, M., Memoli, D., Alexandrova, E., Di Rosa, D., D’Agostino, Y., Russo, F., et al. (2024). Small non-coding RNA transcriptomic profiling in adult and fetal human brain. Sci. Data 11 (1), 767. doi:10.1038/s41597-024-03604-6
Sohn, Y. S., Tamir, S., Song, L., Michaeli, D., Matouk, I., Conlan, A. R., et al. (2013). NAF-1 and mitoNEET are central to human breast cancer proliferation by maintaining mitochondrial homeostasis and promoting tumor growth. Proc. Natl. Acad. Sci. U. S. A. 110 (36), 14676–14681. doi:10.1073/pnas.1313198110
Song, S., Zhang, M., Xie, P., Wang, S., and Wang, Y. (2022). Comprehensive analysis of cuproptosis-related genes and tumor microenvironment infiltration characterization in breast cancer. Front. Immunol. 13, 978909. doi:10.3389/fimmu.2022.978909
Steinbrueck, A., Sedgwick, A. C., Brewster, J. T., Yan, K. C., Shang, Y., Knoll, D. M., et al. (2020). Transition metal chelators, pro-chelators, and ionophores as small molecule cancer chemotherapeutic agents. Chem. Soc. Rev. 49 (12), 3726–3747. doi:10.1039/c9cs00373h
Stevens, R. G., Jones, D. Y., Micozzi, M. S., and Taylor, P. R. (1988). Body iron stores and the risk of cancer. N. Engl. J. Med. 319 (16), 1047–1052. doi:10.1056/nejm198810203191603
Stockwell, B. R. (2022). Ferroptosis turns 10: emerging mechanisms, physiological functions, and therapeutic applications. Cell 185 (14), 2401–2421. doi:10.1016/j.cell.2022.06.003
Sui, S., Xu, S., and Pang, D. (2022). Emerging role of ferroptosis in breast cancer: new dawn for overcoming tumor progression. Pharmacol. Ther. 232, 107992. doi:10.1016/j.pharmthera.2021.107992
Sun, C., Liu, P., Pei, L., Zhao, M., and Huang, Y. (2022). Propofol inhibits proliferation and augments the anti-tumor effect of doxorubicin and paclitaxel partly through promoting ferroptosis in triple-negative breast cancer cells. Front. Oncol. 12, 837974. doi:10.3389/fonc.2022.837974
Sun, L., Wang, H., Yu, S., Zhang, L., Jiang, J., and Zhou, Q. (2022). Herceptin induces ferroptosis and mitochondrial dysfunction in H9c2 cells. Int. J. Mol. Med. 49 (2), 17. doi:10.3892/ijmm.2021.5072
Swetha, K. L., Paul, M., Maravajjala, K. S., Kumbham, S., Biswas, S., and Roy, A. (2023). Overcoming drug resistance with a docetaxel and disulfiram loaded pH-sensitive nanoparticle. J. Control Release 356, 93–114. doi:10.1016/j.jconrel.2023.02.023
Tang, D., Chen, X., Kang, R., and Kroemer, G. (2021). Ferroptosis: molecular mechanisms and health implications. Cell Res. 31 (2), 107–125. doi:10.1038/s41422-020-00441-1
Tang, D., Chen, X., and Kroemer, G. (2022). Cuproptosis: a copper-triggered modality of mitochondrial cell death. Cell Res. 32 (5), 417–418. doi:10.1038/s41422-022-00653-7
Tang, D., Kepp, O., and Kroemer, G. (2020). Ferroptosis becomes immunogenic: implications for anticancer treatments. Oncoimmunology 10 (1), 1862949. doi:10.1080/2162402X.2020.1862949
Tang, D., Kroemer, G., and Kang, R. (2024). Targeting cuproplasia and cuproptosis in cancer. Nat. Rev. Clin. Oncol. 21 (5), 370–388. doi:10.1038/s41571-024-00876-0
Tang, X., Ding, H., Liang, M., Chen, X., Yan, Y., Wan, N., et al. (2021). Curcumin induces ferroptosis in non-small-cell lung cancer via activating autophagy. Thorac. Cancer 12 (8), 1219–1230. doi:10.1111/1759-7714.13904
Taylor, B. C., Sun, X., Gonzalez-Ericsson, P. I., Sanchez, V., Sanders, M. E., Wescott, E. C., et al. (2024). NKG2A is a therapeutic vulnerability in immunotherapy resistant MHC-I heterogeneous triple-negative breast cancer. Cancer Discov. 14 (2), 290–307. doi:10.1158/2159-8290.Cd-23-0519
Theil, E. C. (2000). Targeting mRNA to regulate iron and oxygen metabolism. Biochem. Pharmacol. 59 (1), 87–93. doi:10.1016/s0006-2952(99)00300-7
Tian, H., Duan, J., Li, B., Qin, S., Nice, E. C., Zhang, W., et al. (2023). Clinical chemotherapeutic agent coordinated copper-based nanoadjuvants for efficiently sensitizing cancer chemo-immunotherapy by cuproptosis-mediated mitochondrial metabolic reprogramming. Adv. Funct. Mater. 33 (51), 2306584. doi:10.1002/adfm.202306584
Tiwari, A., Trivedi, R., and Lin, S. Y. (2022). Tumor microenvironment: barrier or opportunity towards effective cancer therapy. J. Biomed. Sci. 29 (1), 83. doi:10.1186/s12929-022-00866-3
Tong, X., Tang, R., Xiao, M., Xu, J., Wang, W., Zhang, B., et al. (2022). Targeting cell death pathways for cancer therapy: recent developments in necroptosis, pyroptosis, ferroptosis, and cuproptosis research. J. Hematol. and Oncol. 15 (1), 174. doi:10.1186/s13045-022-01392-3
Torti, S. V., and Torti, F. M. (2013). Cellular iron metabolism in prognosis and therapy of breast cancer. Crit. Rev. Oncog. 18 (5), 435–448. doi:10.1615/critrevoncog.2013007784
Toyokuni, S. (1996). Iron-induced carcinogenesis: the role of redox regulation. Free Radic. Biol. Med. 20 (4), 553–566. doi:10.1016/0891-5849(95)02111-6
Traeger, L., Wiegand, S. B., Sauer, A. J., Corman, B. H. P., Peneyra, K. M., Wunderer, F., et al. (2022). UBA6 and NDFIP1 regulate the degradation of ferroportin. Haematologica 107 (2), 478–488. doi:10.3324/haematol.2021.278530
Tsang, T., Davis, C. I., and Brady, D. C. (2021). Copper biology. Curr. Biol. 31 (9), R421–r427. doi:10.1016/j.cub.2021.03.054
Tsui, K. H., Hsiao, J. H., Lin, L. T., Tsang, Y. L., Shao, A. N., Kuo, C. H., et al. (2024). The cross-communication of cuproptosis and regulated cell death in human pathophysiology. Int. J. Biol. Sci. 20 (1), 218–230. doi:10.7150/ijbs.84733
Tsvetkov, P., Coy, S., Petrova, B., Dreishpoon, M., Verma, A., Abdusamad, M., et al. (2022). Copper induces cell death by targeting lipoylated TCA cycle proteins. Science 375 (6586), 1254–1261. doi:10.1126/science.abf0529
Tsvetkov, P., Detappe, A., Cai, K., Keys, H. R., Brune, Z., Ying, W., et al. (2019). Mitochondrial metabolism promotes adaptation to proteotoxic stress. Nat. Chem. Biol. 15 (7), 681–689. doi:10.1038/s41589-019-0291-9
Tümer, Z., and Møller, L. B. (2010). Menkes disease. Eur. J. Hum. Genet. 18 (5), 511–518. doi:10.1038/ejhg.2009.187
Wang, D., Liang, W., Huo, D., Wang, H., Wang, Y., Cong, C., et al. (2023). SPY1 inhibits neuronal ferroptosis in amyotrophic lateral sclerosis by reducing lipid peroxidation through regulation of GCH1 and TFR1. Cell Death Differ. 30 (2), 369–382. doi:10.1038/s41418-022-01089-7
Wang, D., Wei, G., Ma, J., Cheng, S., Jia, L., Song, X., et al. (2021). Identification of the prognostic value of ferroptosis-related gene signature in breast cancer patients. BMC cancer 21 (1), 645. doi:10.1186/s12885-021-08341-2
Wang, F., Tang, C., Gao, X., and Xu, J. (2020). Identification of a six-gene signature associated with tumor mutation burden for predicting prognosis in patients with invasive breast carcinoma. Ann. Transl. Med. 8 (7), 453. doi:10.21037/atm.2020.04.02
Wang, G., Xie, L., Li, B., Sang, W., Yan, J., Li, J., et al. (2021). A nanounit strategy reverses immune suppression of exosomal PD-L1 and is associated with enhanced ferroptosis. Nat. Commun. 12 (1), 5733. doi:10.1038/s41467-021-25990-w
Wang, J., Li, J., Liu, J., Chan, K. Y., Lee, H. S., Lin, K. N., et al. (2024). Interplay of ferroptosis and cuproptosis in cancer: dissecting metal-driven mechanisms for therapeutic potentials. Cancers (Basel) 16 (3), 512. doi:10.3390/cancers16030512
Wang, J., Li, S., Guo, Y., Zhao, C., Chen, Y., Ning, W., et al. (2023a). Cuproptosis-related gene SLC31A1 expression correlates with the prognosis and tumor immune microenvironment in glioma. Funct. Integr. Genomics 23 (3), 279. doi:10.1007/s10142-023-01210-0
Wang, J., Luo, L. Z., Liang, D. M., Guo, C., Huang, Z. H., Sun, G. Y., et al. (2023b). Progress in the research of cuproptosis and possible targets for cancer therapy. World J. Clin. Oncol. 14 (9), 324–334. doi:10.5306/wjco.v14.i9.324
Wang, L., Chai, X., Wan, R., Zhang, H., Zhou, C., Xiang, L., et al. (2020). Disulfiram chelated with copper inhibits the growth of gastric cancer cells by modulating stress response and wnt/β-catenin signaling. Front. Oncol. 10, 595718. doi:10.3389/fonc.2020.595718
Wang, L., Chen, Y., Zhao, J., Luo, D., and Tian, W. (2022). Analysis and prediction model of ferroptosis related genes in breast cancer. Transl. Cancer Res. 11 (7), 1970–1976. doi:10.21037/tcr-21-2686
Wang, L., Ouyang, S., Li, B., Wu, H., and Wang, F. (2021). GSK-3β manipulates ferroptosis sensitivity by dominating iron homeostasis. Cell Death Discov. 7 (1), 334. doi:10.1038/s41420-021-00726-3
Wang, N., Gu, Y., Li, L., Chi, J., Liu, X., Xiong, Y., et al. (2022). Identification of novel prognostic risk signature of breast cancer based on ferroptosis-related genes. Sci. Rep. 12 (1), 13766. doi:10.1038/s41598-022-18044-8
Wang, R., Xu, K., Chen, Q., Hu, Q., Zhang, J., and Guan, X. (2023). Cuproptosis engages in c-Myc-mediated breast cancer stemness. J. Transl. Med. 21 (1), 409. doi:10.1186/s12967-023-04204-5
Wang, W., Lu, K., Jiang, X., Wei, Q., Zhu, L., Wang, X., et al. (2023). Ferroptosis inducers enhanced cuproptosis induced by copper ionophores in primary liver cancer. J. Exp. Clin. Cancer Res. 42 (1), 142. doi:10.1186/s13046-023-02720-2
Wang, Y., Chen, Y., Zhang, J., Yang, Y., Fleishman, J. S., Wang, Y., et al. (2024). Cuproptosis: a novel therapeutic target for overcoming cancer drug resistance. Drug Resist Updat 72, 101018. doi:10.1016/j.drup.2023.101018
Wang, Y., Drum, D. L., Sun, R., Zhang, Y., Yu, L., Jia, L., et al. (2023c). Stressed target cancer cells drive nongenetic reprogramming of CAR T cells and tumor microenvironment, overcoming multiple obstacles of CAR T therapy for solid tumors. Res. Square.
Wang, Y., Pang, X., Liu, Y., Mu, G., and Wang, Q. (2023d). SOCS1 acts as a ferroptosis driver to inhibit the progression and chemotherapy resistance of triple-negative breast cancer. Carcinogenesis 44 (8-9), 708–715. doi:10.1093/carcin/bgad060
Wang, Y., Xu, Y., and Zhang, Y. (2022). A novel ferroptosis-related long noncoding RNA signature for relapse free survival prediction in patients with breast cancer. Med. Baltim. 101 (31), e29573. doi:10.1097/md.0000000000029573
Wang, Z., Jin, D., Zhou, S., Dong, N., Ji, Y., An, P., et al. (2023e). Regulatory roles of copper metabolism and cuproptosis in human cancers. Front. Oncol. 13, 1123420. doi:10.3389/fonc.2023.1123420
Wang, Z., Li, R., Hou, N., Zhang, J., Wang, T., Fan, P., et al. (2023f). PRMT5 reduces immunotherapy efficacy in triple-negative breast cancer by methylating KEAP1 and inhibiting ferroptosis. J. Immunother. cancer 11 (6), e006890. doi:10.1136/jitc-2023-006890
Ward, D. M., and Cloonan, S. M. (2019). Mitochondrial iron in human health and disease. Annu. Rev. Physiol. 81, 453–482. doi:10.1146/annurev-physiol-020518-114742
Wedam, R., Greer, Y. E., Wisniewski, D. J., Weltz, S., Kundu, M., Voeller, D., et al. (2023). Targeting mitochondria with ClpP agonists as a novel therapeutic opportunity in breast cancer. Cancers (Basel) 15 (7), 1936. doi:10.3390/cancers15071936
Weekley, C. M., and He, C. (2017). Developing drugs targeting transition metal homeostasis. Curr. Opin. Chem. Biol. 37, 26–32. doi:10.1016/j.cbpa.2016.12.011
Wei, T., Zhu, N., Jiang, W., and Xing, X. L. (2022). Development and validation of ferroptosis- and immune-related lncRNAs signatures for breast infiltrating duct and lobular carcinoma. Front. Oncol. 12, 844642. doi:10.3389/fonc.2022.844642
Werlenius, K., Kinhult, S., Solheim, T. S., Magelssen, H., Löfgren, D., Mudaisi, M., et al. (2023). Effect of disulfiram and copper plus chemotherapy vs chemotherapy alone on survival in patients with recurrent glioblastoma: a randomized clinical trial. JAMA Netw. Open 6 (3), e234149. doi:10.1001/jamanetworkopen.2023.4149
Witkowska, D., Słowik, J., and Chilicka, K. (2021). Heavy metals and human health: possible exposure pathways and the competition for protein binding sites. Molecules 26 (19), 6060. doi:10.3390/molecules26196060
Wright, G. S. A. (2020). Molecular and pharmacological chaperones for SOD1. Biochem. Soc. Trans. 48 (4), 1795–1806. doi:10.1042/bst20200318
Wu, C., Zhang, F., Li, B., Li, Z., Xie, X., Huang, Y., et al. (2023). A self-assembly nano-prodrug for combination therapy in triple-negative breast cancer stem cells. Small 19 (41), 2301600. doi:10.1002/smll.202301600
Wu, H., Zhang, Z., Cao, Y., Hu, Y., Li, Y., Zhang, L., et al. (2024). A self-amplifying ROS-responsive nanoplatform for simultaneous cuproptosis and cancer immunotherapy. Adv. Sci. 11, 2401047. doi:10.1002/advs.202401047
Wu, S., Pan, R., Lu, J., Wu, X., Xie, J., Tang, H., et al. (2022). Development and verification of a prognostic ferroptosis-related gene model in triple-negative breast cancer. Front. Oncol. 12, 896927. doi:10.3389/fonc.2022.896927
Wu, W., Yu, L., Jiang, Q., Huo, M., Lin, H., Wang, L., et al. (2019). Enhanced tumor-specific disulfiram chemotherapy by in situ Cu(2+) chelation-initiated nontoxicity-to-toxicity transition. J. Am. Chem. Soc. 141 (29), 11531–11539. doi:10.1021/jacs.9b03503
Wu, X., Zhang, Y., Liang, G., and Ye, H. (2023). Cuproptosis-related lncRNAs potentially predict prognosis and therapy sensitivity of breast cancer. Front. Pharmacol. 14, 1199883. doi:10.3389/fphar.2023.1199883
Xia, L., Oyang, L., Lin, J., Tan, S., Han, Y., Wu, N., et al. (2021). The cancer metabolic reprogramming and immune response. Mol. cancer 20, 28–21. doi:10.1186/s12943-021-01316-8
Xia, Y., Gu, M., Wang, J., Zhang, X., Shen, T., Shi, X., et al. (2024). Tumor microenvironment-activated, immunomodulatory nanosheets loaded with copper (II) and 5-FU for synergistic chemodynamic therapy and chemotherapy. J. Colloid Interface Sci. 653, 137–147. doi:10.1016/j.jcis.2023.09.042
Xie, J., Huang, H., Wei, X., Tan, P., Ouyang, L., Wang, L., et al. (2024). Boswellia carterii n-hexane extract suppresses breast cancer growth via induction of ferroptosis by downregulated GPX4 and upregulated transferrin. Sci. Rep. 14 (1), 14307. doi:10.1038/s41598-024-65170-6
Xie, J., Yang, Y., Gao, Y., and He, J. (2023). Cuproptosis: mechanisms and links with cancers. Mol. Cancer 22 (1), 46. doi:10.1186/s12943-023-01732-y
Xie, Y., Wang, B., Zhao, Y., Tao, Z., Wang, Y., Chen, G., et al. (2022). Mammary adipocytes protect triple-negative breast cancer cells from ferroptosis. J. Hematol. and Oncol. 15 (1), 72. doi:10.1186/s13045-022-01297-1
Xiong, H., Wang, C., Wang, Z., Jiang, Z., Zhou, J., and Yao, J. (2019). Intracellular cascade activated nanosystem for improving ER+ breast cancer therapy through attacking GSH-mediated metabolic vulnerability. J. Control. Release 309, 145–157. doi:10.1016/j.jconrel.2019.07.029
Xiong, R., He, R., Liu, B., Jiang, W., Wang, B., Li, N., et al. (2021). Ferroptosis: a new promising target for lung cancer therapy. Oxid. Med. Cell Longev. 2021, 8457521. doi:10.1155/2021/8457521
Xu, B. B., Jin, N., Liu, J. C., Liao, A. Q., Lin, H. Y., and Qin, X. Y. (2024). Arene-arene coupled disulfamethazines (or sulfadiazine)-phenanthroline-metal(II) complexes were synthesized by in situ reactions and inhibited the growth and development of triple-negative breast cancer through the synergistic effect of antiangiogenesis, anti-inflammation, pro-apoptosis, and cuproptosis. J. Med. Chem. 67 (9), 7088–7111. doi:10.1021/acs.jmedchem.3c02432
Xu, C., Chen, Y., Yu, Q., Song, J., Jin, Y., and Gao, X. (2023). Compounds targeting ferroptosis in breast cancer: progress and their therapeutic potential. Front. Pharmacol. 14, 1243286. doi:10.3389/fphar.2023.1243286
Xu, L., Hu, Y., and Liu, W. (2022). Pyroptosis-mediated molecular subtypes are characterized by distinct tumor microenvironment infiltration characteristics in breast cancer. J. Inflamm. Res. 15, 345–362. doi:10.2147/jir.S349186
Xu, L., Wang, S., Zhang, D., Wu, Y., Shan, J., Zhu, H., et al. (2023). Machine learning- and WGCNA-mediated double analysis based on genes associated with disulfidptosis, cuproptosis and ferroptosis for the construction and validation of the prognostic model for breast cancer. J. Cancer Res. Clin. Oncol. 149 (18), 16511–16523. doi:10.1007/s00432-023-05378-7
Xu, Q. T., Wang, Z. W., Cai, M. Y., Wei, J. F., and Ding, Q. (2022). A novel cuproptosis-related prognostic 2-lncRNAs signature in breast cancer. Front. Pharmacol. 13, 1115608. doi:10.3389/fphar.2022.1115608
Xu, R., Wang, W., and Zhang, W. (2023). Ferroptosis and the bidirectional regulatory factor p53. Cell Death Discov. 9 (1), 197. doi:10.1038/s41420-023-01517-8
Xu, S., Liu, D., Chang, T., Wen, X., Ma, S., Sun, G., et al. (2022). Cuproptosis-associated lncRNA establishes new prognostic profile and predicts immunotherapy response in clear cell renal cell carcinoma. Front. Genet. 13, 938259. doi:10.3389/fgene.2022.938259
Xu, S., Min, J., and Wang, F. (2021). Ferroptosis: an emerging player in immune cells. Sci. Bull. 66 (22), 2257–2260. doi:10.1016/j.scib.2021.02.026
Xu, W., Wang, Y., Hou, G., Wang, J., Wang, T., Qian, J., et al. (2023). Tumor microenvironment responsive hollow nanoplatform for triple amplification of oxidative stress to enhance cuproptosis-based synergistic cancer therapy. Adv. Healthc. Mater 12 (13), e2202949. doi:10.1002/adhm.202202949
Xu, Y., Pan, S., Jiang, W., Xue, F., and Zhu, X. (2020). Effects of propofol on the development of cancer in humans. Cell Prolif. 53 (8), e12867. doi:10.1111/cpr.12867
Xu, Z., Jiang, S., Ma, J., Tang, D., Yan, C., and Fang, K. (2021). Comprehensive analysis of ferroptosis-related LncRNAs in breast cancer patients reveals prognostic value and relationship with tumor immune microenvironment. Front. Surg. 8, 742360. doi:10.3389/fsurg.2021.742360
Xue, Q., Kang, R., Klionsky, D. J., Tang, D., Liu, J., and Chen, X. (2023a). Copper metabolism in cell death and autophagy. Autophagy 19 (8), 2175–2195. doi:10.1080/15548627.2023.2200554
Xue, Q., Yan, D., Chen, X., Li, X., Kang, R., Klionsky, D. J., et al. (2023b). Copper-dependent autophagic degradation of GPX4 drives ferroptosis. Autophagy 19 (7), 1982–1996. doi:10.1080/15548627.2023.2165323
Yang, F., Liao, J., Yu, W., Qiao, N., Guo, J., Han, Q., et al. (2021). Exposure to copper induces mitochondria-mediated apoptosis by inhibiting mitophagy and the PINK1/parkin pathway in chicken (Gallus gallus) livers. J. Hazard Mater 408, 124888. doi:10.1016/j.jhazmat.2020.124888
Yang, F., Xiao, Y., Ding, J. H., Jin, X., Ma, D., Li, D. Q., et al. (2023). Ferroptosis heterogeneity in triple-negative breast cancer reveals an innovative immunotherapy combination strategy. Cell Metab. 35 (1), 84–100.e8. doi:10.1016/j.cmet.2022.09.021
Yang, L., Yang, P., Lip, G. Y., and Ren, J. (2023). Copper homeostasis and cuproptosis in cardiovascular disease therapeutics. Trends Pharmacol. Sci. 44, 573–585. doi:10.1016/j.tips.2023.07.004
Yang, M., Zheng, H., Xu, K., Yuan, Q., Aihaiti, Y., Cai, Y., et al. (2022). A novel signature to guide osteosarcoma prognosis and immune microenvironment: cuproptosis-related lncRNA. Front. Immunol. 13, 919231. doi:10.3389/fimmu.2022.919231
Yang, Q., Liu, W., Zhang, S., and Liu, S. (2020). The cardinal roles of ferroportin and its partners in controlling cellular iron in and out. Life Sci. 258, 118135. doi:10.1016/j.lfs.2020.118135
Yang, W., Wang, Y., Huang, Y., Yu, J., Wang, T., Li, C., et al. (2023). 4-Octyl itaconate inhibits aerobic glycolysis by targeting GAPDH to promote cuproptosis in colorectal cancer. Biomed. and Pharmacother. 159, 114301. doi:10.1016/j.biopha.2023.114301
Yang, W. S., SriRamaratnam, R., Welsch, M. E., Shimada, K., Skouta, R., Viswanathan, V. S., et al. (2014). Regulation of ferroptotic cancer cell death by GPX4. Cell 156 (1), 317–331. doi:10.1016/j.cell.2013.12.010
Yang, X., Deng, L., Diao, X., Yang, S., Zou, L., Yang, Q., et al. (2023). Targeting cuproptosis by zinc pyrithione in triple-negative breast cancer. iScience 26 (11), 108218. doi:10.1016/j.isci.2023.108218
Yang, Y., Du, J., Huang, Y. F., He, W., Liu, L., Li, D., et al. (2024a). Identification of TFR2 as a novel ferroptosis-related gene that serves an important role in prognosis and progression of triple-negative breast cancer. Oncol. Lett. 27 (2), 43. doi:10.3892/ol.2023.14176
Yang, Y., Fan, H., and Guo, Z. (2024b). Modulation of metal homeostasis for cancer therapy. ChemPlusChem 89 (6), e202300624. doi:10.1002/cplu.202300624
Yang, Y., Liang, S., Geng, H., Xiong, M., Li, M., Su, Q., et al. (2022). Proteomics revealed the crosstalk between copper stress and cuproptosis, and explored the feasibility of curcumin as anticancer copper ionophore. Free Radic. Biol. Med. 193, 638–647. doi:10.1016/j.freeradbiomed.2022.11.023
Yang, Z., Guo, F., Albers, A. E., Sehouli, J., and Kaufmann, A. M. (2019). Disulfiram modulates ROS accumulation and overcomes synergistically cisplatin resistance in breast cancer cell lines. Biomed. Pharmacother. 113, 108727. doi:10.1016/j.biopha.2019.108727
Yang Y, Y., Li, M., Chen, G., Liu, S., Guo, H., Dong, X., et al. (2023). Dissecting copper biology and cancer treatment:‘Activating Cuproptosis or suppressing Cuproplasia. Coord. Chem. Rev. 495, 215395. doi:10.1016/j.ccr.2023.215395
Yao, X., Xie, R., Cao, Y., Tang, J., Men, Y., Peng, H., et al. (2021). Simvastatin induced ferroptosis for triple-negative breast cancer therapy. J. Nanobiotechnology 19 (1), 311. doi:10.1186/s12951-021-01058-1
Yao, Z. Y., Xing, C., Cai, S., and Xing, X. L. (2022). Development and validation of ferroptosis-related lncRNAs as prognosis and diagnosis biomarkers for breast cancer. Biomed. Res. Int. 2022, 2390764. doi:10.1155/2022/2390764
Ye, L. F., Chaudhary, K. R., Zandkarimi, F., Harken, A. D., Kinslow, C. J., Upadhyayula, P. S., et al. (2020). Radiation-induced lipid peroxidation triggers ferroptosis and synergizes with ferroptosis inducers. ACS Chem. Biol. 15 (2), 469–484. doi:10.1021/acschembio.9b00939
Ye, S., Hu, X., Sun, S., Su, B., Cai, J., and Jiang, J. (2024). Oridonin promotes RSL3-induced ferroptosis in breast cancer cells by regulating the oxidative stress signaling pathway JNK/Nrf2/HO-1. Eur. J. Pharmacol. 974, 176620. doi:10.1016/j.ejphar.2024.176620
Yin, L., Liu, P., Jin, Y., Ning, Z., Yang, Y., and Gao, H. (2022). Ferroptosis-related small-molecule compounds in cancer therapy: strategies and applications. Eur. J. Med. Chem. 244, 114861. doi:10.1016/j.ejmech.2022.114861
Yin, L., and Tang, Y. (2022). Predicting prognosis and clinical features of the tumor microenvironment based on ferroptosis score in patients with breast cancer. Sci. Rep. 12 (1), 10611. doi:10.1038/s41598-022-14964-7
Ying, Y., Man, L., Gang, C., Suyi, L., Houqi, G., Xiongwei, D., et al. (2023). Dissecting copper biology and cancer treatment: ‘Activating Cuproptosis or suppressing Cuproplasia’. Coord. Chem. Rev. 495, 215395. doi:10.1016/j.ccr.2023.215395
Yip, N. C., Fombon, I. S., Liu, P., Brown, S., Kannappan, V., Armesilla, A. L., et al. (2011). Disulfiram modulated ROS-MAPK and NFκB pathways and targeted breast cancer cells with cancer stem cell-like properties. Br. J. Cancer 104 (10), 1564–1574. doi:10.1038/bjc.2011.126
Yu, X., Li, B., Yan, J., Li, W., Tian, H., Wang, G., et al. (2024). Cuproptotic nanoinducer-driven proteotoxic stress potentiates cancer immunotherapy by activating the mtDNA–cGAS–STING signaling. Biomaterials 307, 122512. doi:10.1016/j.biomaterials.2024.122512
Yu, Z., Zhou, R., Zhao, Y., Pan, Y., Liang, H., Zhang, J. S., et al. (2019). Blockage of SLC31A1-dependent copper absorption increases pancreatic cancer cell autophagy to resist cell death. Cell Prolif. 52 (2), e12568. doi:10.1111/cpr.12568
Yuan, H. J., Xue, Y. T., and Liu, Y. (2022). Cuproptosis, the novel therapeutic mechanism for heart failure: a narrative review. Cardiovasc Diagn Ther. 12 (5), 681–692. doi:10.21037/cdt-22-214
Zarjou, A., Bolisetty, S., Joseph, R., Traylor, A., Apostolov, E. O., Arosio, P., et al. (2013). Proximal tubule H-ferritin mediates iron trafficking in acute kidney injury. J. Clin. investigation 123 (10), 4423–4434. doi:10.1172/JCI67867
Zechner, R., Zimmermann, R., Eichmann, T. O., Kohlwein, S. D., Haemmerle, G., Lass, A., et al. (2012). FAT SIGNALS--lipases and lipolysis in lipid metabolism and signaling. Cell Metab. 15 (3), 279–291. doi:10.1016/j.cmet.2011.12.018
Zeng, L., Ding, S., Cao, Y., Li, C., Zhao, B., Ma, Z., et al. (2023). A MOF-based potent ferroptosis inducer for enhanced radiotherapy of triple negative breast cancer. ACS Nano 17 (14), 13195–13210. doi:10.1021/acsnano.3c00048
Zha, J., Chen, F., Dong, H., Shi, P., Yao, Y., Zhang, Y., et al. (2014). Disulfiram targeting lymphoid malignant cell lines via ROS-JNK activation as well as Nrf2 and NF-kB pathway inhibition. J. Transl. Med. 12, 163. doi:10.1186/1479-5876-12-163
Zhai, X., Lin, Y., Zhu, L., Wang, Y., Zhang, J., Liu, J., et al. (2024). Ferroptosis in cancer immunity and immunotherapy: multifaceted interplay and clinical implications. Cytokine Growth Factor Rev. 75, 101–109. doi:10.1016/j.cytogfr.2023.08.004
Zhang, C., Huang, T., and Li, L. (2024). Targeting cuproptosis for cancer therapy: mechanistic insights and clinical perspectives. J. Hematol. Oncol. 17 (1), 68. doi:10.1186/s13045-024-01589-8
Zhang, D., Yang, S., Li, Y., Yao, J., Ruan, J., Zheng, Y., et al. (2020). Prediction of overall survival among female patients with breast cancer using a prognostic signature based on 8 DNA repair-related genes. JAMA Netw. Open 3 (10), e2014622. doi:10.1001/jamanetworkopen.2020.14622
Zhang, H., Chen, D., Ringler, J., Chen, W., Cui, Q. C., Ethier, S. P., et al. (2010). Disulfiram treatment facilitates phosphoinositide 3-kinase inhibition in human breast cancer cells in vitro and in vivo. Cancer Res. 70 (10), 3996–4004. doi:10.1158/0008-5472.Can-09-3752
Zhang, H., Zhu, S., Zhou, H., Li, R., Xia, X., and Xiong, H. (2023). Identification of MTHFD2 as a prognostic biomarker and ferroptosis regulator in triple-negative breast cancer. Front. Oncol. 13, 1098357. doi:10.3389/fonc.2023.1098357
Zhang, J., Peng, L., Hao, Y., Yang, H., Zhao, W., and Mao, C. (2023). Biodegradable CuMoO4 Nanodots with multienzyme activities for multimodal treatment of tumor. Adv. Healthc. Mater. 12 (22), 2300167. doi:10.1002/adhm.202300167
Zhang, K., Ping, L., Du, T., Liang, G., Huang, Y., Li, Z., et al. (2021). A ferroptosis-related lncRNAs signature predicts prognosis and immune microenvironment for breast cancer. Front. Mol. Biosci. 8, 678877. doi:10.3389/fmolb.2021.678877
Zhang, L., Chen, W., Liu, S., and Chen, C. (2023). Targeting breast cancer stem cells. Int. J. Biol. Sci. 19 (2), 552–570. doi:10.7150/ijbs.76187
Zhang, N., Ping, W., Rao, K., Zhang, Z., Huang, R., Zhu, D., et al. (2024). Biomimetic copper-doped polypyrrole nanoparticles induce glutamine metabolism inhibition to enhance breast cancer cuproptosis and immunotherapy. J. Control. Release 371, 204–215. doi:10.1016/j.jconrel.2024.05.045
Zhang, S., Chen, Y., Guo, W., Yuan, L., Zhang, D., Xu, Y., et al. (2014). Disordered hepcidin-ferroportin signaling promotes breast cancer growth. Cell Signal 26 (11), 2539–2550. doi:10.1016/j.cellsig.2014.07.029
Zhang, T., Kephart, J., Bronson, E., Anand, M., Daly, C., Spasojevic, I., et al. (2022). Prospective clinical trial of disulfiram plus copper in men with metastatic castration-resistant prostate cancer. Prostate 82 (7), 858–866. doi:10.1002/pros.24329
Zhang, X., Ge, X., Jiang, T., Yang, R., and Li, S. (2022). Research progress on immunotherapy in triple-negative breast cancer. Int. J. Oncol. 61 (2), 1–13.
Zhang, X., and Zhang, Q. (2023). A novel ferroptosis-related gene signature associated with cuproptosis for predicting overall survival in breast cancer patients. Acta Biochim. Pol. 70 (4), 875–884. doi:10.18388/abp.2020_6722
Zhang, Y., Qian, Y., Zhang, J., Yan, W., Jung, Y. S., Chen, M., et al. (2017). Ferredoxin reductase is critical for p53-dependent tumor suppression via iron regulatory protein 2. Genes. Dev. 31 (12), 1243–1256. doi:10.1101/gad.299388.117
Zhang, Z., Guo, M., Shen, M., Kong, D., Zhang, F., Shao, J., et al. (2020). The BRD7-P53-SLC25A28 axis regulates ferroptosis in hepatic stellate cells. Redox Biol. 36, 101619. doi:10.1016/j.redox.2020.101619
Zhang, Z., Lu, M., Chen, C., Tong, X., Li, Y., Yang, K., et al. (2021). Holo-lactoferrin: the link between ferroptosis and radiotherapy in triple-negative breast cancer. Theranostics 11 (7), 3167–3182. doi:10.7150/thno.52028
Zhao, F., Yu, H., Liang, L., Wang, C., Shi, D., Zhang, X., et al. (2023). Redox homeostasis disruptors based on metal-phenolic network nanoparticles for chemo/chemodynamic synergistic tumor therapy through activating apoptosis and cuproptosis. Adv. Healthc. Mater 12 (29), e2301346. doi:10.1002/adhm.202301346
Zhao, J., Zhang, N., Ma, X., Li, M., and Feng, H. (2023). The dual role of ferroptosis in anthracycline-based chemotherapy includes reducing resistance and increasing toxicity. Cell Death Discov. 9 (1), 184. doi:10.1038/s41420-023-01483-1
Zheng, J., and Conrad, M. (2020). The metabolic underpinnings of ferroptosis. Cell Metab. 32 (6), 920–937. doi:10.1016/j.cmet.2020.10.011
Zheng, J., Ge, H., Guo, M., Zhang, T., Hu, Q., Yao, Q., et al. (2024). Photoinduced cuproptosis with tumor-specific for metastasis-inhibited cancer therapy. Small 20 (10), 2304407. doi:10.1002/smll.202304407
Zheng, P., Zhou, C., Lu, L., Liu, B., and Ding, Y. (2022). Elesclomol: a copper ionophore targeting mitochondrial metabolism for cancer therapy. J. Exp. Clin. Cancer Res. 41 (1), 271. doi:10.1186/s13046-022-02485-0
Zheng, Q., Shi, S., Zhang, N., and Chen, H. (2023). A novel cuproptosis-related genes model in breast cancer prognosis. Med. Baltim. 102 (31), e34507. doi:10.1097/md.0000000000034507
Zheng, X., Liu, Z., Mi, M., Wen, Q., Wu, G., and Zhang, L. (2021). Disulfiram improves the anti-PD-1 therapy efficacy by regulating PD-L1 expression via epigenetically reactivation of IRF7 in triple negative breast cancer. Front. Oncol. 11, 734853. doi:10.3389/fonc.2021.734853
Zhong, J., Zheng, X., Wen, Y., Wang, S.-B., Zhan, G., and Chen, A.-Z. (2023). In situ sacrificial growth of metastable copper-enriched nanomedicine for cuproptosis-based synergistic cancer therapy. Chem. Eng. J. 474, 145795. doi:10.1016/j.cej.2023.145795
Zhou, B., Guo, L., Zhang, B., Liu, S., Zhang, K., Yan, J., et al. (2019). Disulfiram combined with copper induces immunosuppression via PD-L1 stabilization in hepatocellular carcinoma. Am. J. Cancer Res. 9 (11), 2442–2455.
Zhou, J., Yu, Q., Song, J., Li, S., Li, X. L., Kang, B., et al. (2023). Photothermally triggered copper payload release for cuproptosis-promoted cancer synergistic therapy. Angew. Chem. Int. Ed. 62 (12), e202213922. doi:10.1002/anie.202213922
Zhou, L., Wong, C., Liu, Y., Jiang, W., and Yang, Q. (2023). Development and validation of stable ferroptosis- and pyroptosis-related signatures in predicting prognosis and immune status in breast cancer. J. Cell Mol. Med. 27 (23), 3827–3838. doi:10.1111/jcmm.17958
Zhou, Q., Meng, Y., Li, D., Yao, L., Le, J., Liu, Y., et al. (2024). Ferroptosis in cancer: from molecular mechanisms to therapeutic strategies. Signal Transduct. Target Ther. 9 (1), 55. doi:10.1038/s41392-024-01769-5
Zhou, T. J., Zhang, M. M., Liu, D. M., Huang, L. L., Yu, H. Q., Wang, Y., et al. (2024). Glutathione depletion and dihydroorotate dehydrogenase inhibition actuated ferroptosis-augment to surmount triple-negative breast cancer. Biomaterials 305, 122447. doi:10.1016/j.biomaterials.2023.122447
Zhou, Z., Deng, J., Pan, T., Zhu, Z., Zhou, X., Lv, C., et al. (2022). Prognostic significance of cuproptosis-related gene signatures in breast cancer based on transcriptomic data analysis. Cancers (Basel) 14 (23), 5771. doi:10.3390/cancers14235771
Zhu, B., Wang, S., Wang, R., and Wang, X. (2022). Identification of molecular subtypes and a six-gene risk model related to cuproptosis for triple negative breast cancer. Front. Genet. 13, 1022236. doi:10.3389/fgene.2022.1022236
Zhu, H., Klement, J. D., Lu, C., Redd, P. S., Yang, D., Smith, A. D., et al. (2021). Asah2 represses the p53–Hmox1 axis to protect myeloid-derived suppressor cells from ferroptosis. J. Immunol. 206 (6), 1395–1404. doi:10.4049/jimmunol.2000500
Zhu, J., Chen, Q., Gu, K., Meng, Y., Ji, S., Zhao, Y., et al. (2022). Correlation between ferroptosis-related gene signature and immune landscape, prognosis in breast cancer. J. Immunol. Res. 2022, 6871518. doi:10.1155/2022/6871518
Zhu, J., You, Y., Zhang, W., Wang, W., Jiang, M., Pu, F., et al. (2024). Sensitizing cuproptosis by endogenous copper-triggered bioorthogonal nanoremodeler. Nano Today 55, 102196. doi:10.1016/j.nantod.2024.102196
Zhu, L., Tian, Q., Jiang, S., Gao, H., Yu, S., Zhou, Y., et al. (2021). A novel ferroptosis-related gene signature for overall survival prediction in patients with breast cancer. Front. Cell Dev. Biol. 9, 670184. doi:10.3389/fcell.2021.670184
Zhu, Z., Zhu, K., Zhang, J., Zhou, Y., and Zhang, Q. (2024). Elucidating the evolving role of cuproptosis in breast cancer progression. Int. J. Biol. Sci. 20 (12), 4872–4887. doi:10.7150/ijbs.98806
Zhu G., G., Wang, M., Qiao, L., Xie, Y., Wang, J., Li, L., et al. (2024). Lysosomal rupture-mediated “broken window effect” to amplify cuproptosis and pyroptosis for high-efficiency cancer immunotherapy. Adv. Funct. Mater. 34, 2400496. doi:10.1002/adfm.202400496
Zou, Y., Zheng, S., Xie, X., Ye, F., Hu, X., Tian, Z., et al. (2022). N6-methyladenosine regulated FGFR4 attenuates ferroptotic cell death in recalcitrant HER2-positive breast cancer. Nat. Commun. 13 (1), 2672. doi:10.1038/s41467-022-30217-7
Glossary
BRCA breast cancer
Fe iron
Cu copper
DNA deoxyribonucleic acid
ROS reactive oxygen species
Fe3+ ferric iron
Fe2+ ferrous iron
STEAP3 six-transmembrane epithelial antigen of prostate 3
TFR1 transferrin receptor protein 1
MFRN mitoferrin
FTH1 ferritin heavy chain 1
NCOA4 nuclear receptor coactivator 4
FPN ferroportin
Cu+ cuprous ion
Cu2+ cupric ion
CTR1 copper transporter 1
Copper ATPases copper-transporting P-type ATPases
BCSCs breast cancer stem cells
DSF/Cu disulfiram/copper
RAS-RAF-MEK-ERK RAS-RAF-Mitogen-activated protein kinase kinase-extracellular signal-regulated kinase
RTK receptor tyrosine kinase
TCA tricarboxylic acid
DLAT dihydrolipoamide S-acetyltransferase
TGN trans-Golgi network
PRNP prion protein
ATP adenosine triphosphate
CISD1 CDGSH iron-sulfur domain-containing protein 1
CISD2 CDGSH iron-sulfur domain-containing protein 2
DBT dihydrolipoyllysine-residue acetyltransferase component of pyruvate dehydrogenase complex
GCSH glycine cleavage system H protein
DLST dihydrolipoamide succinyltransferase
MCF mitochondrial carrier family
SCD1 stearoyl-CoA Desaturase-1
DMOCPTL dimethylaminomicheliolide
ncRNAs non-coding RNAs
lncRNAs long non-coding RNAs
miRNA microRNA
circRNA circular RNA
tsRNA tRNA-derived small RNA
FRGs ferroptosis-related genes
CRGs cuproptosis-related genes
F/CRGs ferroptosis/cuproptosis-related genes
ER+EBC estrogen receptor-positive early breast cancer
iDFS invasive disease-free survival
RFS relapse-free survival
GPX4 glutathione peroxidase 4
GSH glutathione
ALDH aldehyde Dehydrogenase
EMT epithelial-mesenchymal transition
TNBC triple-Negative Breast Cancer
DOX doxorubicin
NPs nanoparticles
CDT chemodynamic therapy
SDT sonodynamic therapy
PTT photothermal therapy
ICD immunogenic cell death
ECM extracellular matrix
mtDNA mitochondrial DNA
LPO lipid peroxidation.
Keywords: ferroptosis, cuproptosis, breast cancer, interplay, immune infiltration, prognosis, cross talk
Citation: Imam M, Ji J, Zhang Z and Yan S (2025) Targeting the initiator to activate both ferroptosis and cuproptosis for breast cancer treatment: progress and possibility for clinical application. Front. Pharmacol. 15:1493188. doi: 10.3389/fphar.2024.1493188
Received: 08 September 2024; Accepted: 12 November 2024;
Published: 10 January 2025.
Edited by:
Xuelin Zhou, Capital Medical University, ChinaReviewed by:
Nianping Liu, Stanford University, United StatesChenxu Guo, Massachusetts General Hospital Cancer Center, United States
Xiaoyan Tang, University of California, San Francisco, United States
Copyright © 2025 Imam, Ji, Zhang and Yan. This is an open-access article distributed under the terms of the Creative Commons Attribution License (CC BY). The use, distribution or reproduction in other forums is permitted, provided the original author(s) and the copyright owner(s) are credited and that the original publication in this journal is cited, in accordance with accepted academic practice. No use, distribution or reproduction is permitted which does not comply with these terms.
*Correspondence: Shunchao Yan, eWFuc2NAY211LmVkdS5jbg==