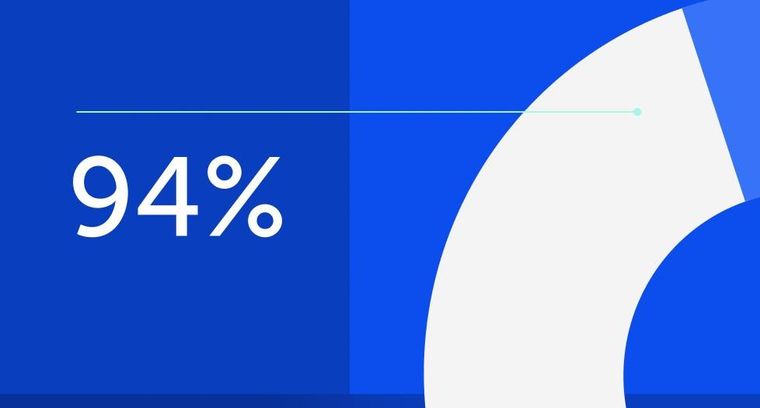
94% of researchers rate our articles as excellent or good
Learn more about the work of our research integrity team to safeguard the quality of each article we publish.
Find out more
REVIEW article
Front. Pharmacol., 20 January 2025
Sec. Experimental Pharmacology and Drug Discovery
Volume 15 - 2024 | https://doi.org/10.3389/fphar.2024.1483792
Glucagon-like peptide-1 (GLP-1) is a 30-amino acid intestinal insulin-stimulating factor, which is mainly secreted by L cells in the distal ileum and colon. It has various physiological functions, such as promoting insulin secretion and synthesis, stimulating β-cell proliferation, inducing islet regeneration, inhibiting β-cell apoptosis and glucagon release, delaying gastric emptying and controlling appetite, etc. It plays a role through a specific GLP-1 receptor (GLP-1R) distributed in many organs or tissues and participates in the regulation of glucose homeostasis in the body. GLP-1 receptor agonists (GLP-1RAs) has the similar physiological function of GLP-1. Because of its structural difference from natural GLP-1, it is not easy to be degraded by dipeptidyl peptidase-4 (DPP-4), thus prolonging the action time. GLP-1RAs have been recognized as a new type of hypoglycemic drugs and widely used in the treatment of type 2 diabetes mellitus (T2DM). Compared with other non-insulin hypoglycemic drugs, it can not only effectively reduce blood glucose and glycosylated hemoglobin (HbA1c), but also protect cardiovascular system, nervous system and kidney function without causing hypoglycemia and weight gain. Therefore, GLP-1RAs has good application prospects and potential for further development.
The idea that substances produced by duodenal mucosa could stimulate and initiate pancreatic endocrine, thereby reducing the amount of urinary glucose in patients with diabetes, was first proposed in 1906 (Moore, 1906). Elrick et al. have shown that compared with intravenous glucose, the increase of plasma insulin level after oral glucose was greater, and the high level of insulin was maintained for a longer time, which provided a basis for intestinal factors to stimulate insulin secretion (Elrick et al., 1964). The enterogenic factor which has the function of reducing blood glucose is named “enteropagin” (Edgard and Jean, 2017). The first identified enteropagin is glucose-dependent insulin-stimulating peptide (GIP). However, immune neutralization of GIP or removal of GIP from intestinal extracts does not completely eliminate the insulin-stimulating activity, indicating that there are other substances with insulin-stimulating activity in the intestine (Drucker, 1998). In the 1980s, after identifying the cDNA sequence and mRNA of proglucagon, two new glucagon-like peptides, glucagon-like peptide-1 (GLP-1) and glucagon-like peptide-2 (GLP-2) (Bell et al., 1983; Lopez et al., 1983; Heinrich et al., 1984), were found in the additional sequence of proglucagon.
GLP-1 is a peptide hormone consisting of 30 amino acids derived from glucagonogen, which is processed by the differentiation of the glucagonogen gene expressed by intestinal epithelial endocrine L-cells, and has two biologically active forms, GLP-1 (7–36 amide) and GLP-1 (7-37), and the intestine is the major source of GLP-1 in plasma (Drucker, 1998; Holst, 2007). Carbohydrate, fat and protein intakes, as well as bile acids, can stimulate the secretion of GLP-1 by intestinal L-cells (Gribble and Reimann, 2021). GLP-1 has a variety of physiological functions, which can regulate blood glucose level by promoting insulin secretion and synthesis, stimulating β-cell proliferation and inducing islet regeneration, inhibiting β-cell apoptosis and glucagon release, delaying gastric emptying and controlling appetite (Müller et al., 2019; Gribble and Reimann, 2021; Drucker and Holst, 2023) (Figure 1). Since the discovery that GLP-1 can improve insulin secretion and glycemic control in patients with type 2 diabetes mellitus (T2DM) in the 1990s (Nauck et al., 1993; Ahrén and Gutniak, 1997; Vella et al., 2000), GLP-1 receptor agonists (GLP1-RAs) have been widely used in clinic.
T2DM is a highly prevalent chronic disease in the world, and its incidence has increased continuously in the past few decades, which has become a major public health problem (Glovaci et al., 2019; Lovic et al., 2020; Taylor et al., 2021). The high incidence and prevalence are attributed to the ageing population and poor lifestyles (Minari et al., 2023). GLP-1RAs, as an important class of drugs for the treatment of T2DM, shows significant advantages in blood glucose control, weight loss, cardiovascular protection and kidney benefit (Ussher and Drucker, 2023; Clark, 2024; Hu et al., 2024). Compared with the previously published articles, thecontents and purposes of this review are different: (1) Focusing on the therapeutic roles of GLP-1RAs in T2DM, the multiple pathways by which GLP-1 regulates blood glucose are described in a more comprehensive and in-depth manner at the mechanism level; (2) The action characteristics of various types of non-insulin hypoglycemic drugs are compared, as well as the advantages, shortcomings, points for attention and challenges of GLP-1RAs in treatment. It is expected that GLP-1RAs can be used more safely, effectively and reasonably for T2DM patients.
GLP-1 is secreted by intestinal L cells and plays a key role in the regulation of blood glucose in a glucose-dependent manner (Zhang et al., 2023). The influencing factors of GLP-1 secretion mainly involve the following aspects: (1) Activation of related receptors. The expression of intestinal endocrine Lcells is regulated by several key receptors activated by short chain fatty acids (SCFAs), specific endogenous cannabinoids and bile acids, and the activation of these receptors increases the secretion of key intestinal peptides, such as GLP-1, GLP-2 and polypeptide YY (PYY) (de Vos et al., 2022). SCFAs receptors belong to the family of G protein-coupled receptors (GPCRs), including GPR41 and GPR43, which are important regulatory receptors for glucose metabolism and lipid metabolism, and can be activated by SCFAs to perform important physiological functions. SCFAs were able to increase the number of L cells in cultured intestinal cells in vitro, leading to an increase in GLP-1 release (Petersen et al., 2014). (2) Structural composition of the microbiota. The imbalance of gut microbiota will reduce the production of SCFAs, weaken the ability to activate SCFAs receptors, and affect GLP-1 secretion, resulting in abnormal glucose metabolism and lipid metabolism (Ma et al., 2019). (3) Dietary factors. In rats fed with high-fat diet, the density of intestinal epithelial cells producing GLP-1, secretory hormone and cholecystokinin reduced, and the expression of intestinal endocrine cells transcription factors encoded by Neurog3 and Neurod1 decreased accordingly (Sakar et al., 2014). In the primary culture cells of high-fat diet mice, transcriptome analysis showed that the gene expression of L cells with many characteristics was impaired (Richards et al., 2016). While a high-fiber diet was able to increase the density of L cells and proglucagon mRNA levels in the colon of obese and leptin-deficient mice (Everard et al., 2011). (4) Roux-en-Y gastric bypass surgery. It leads to a significant increase in postprandial GLP-1 and PYY concentrations in humans and animal models (Jørgensen et al., 2012; Peterli et al., 2012), which may be due to an increase in the number of L cells or in their secretion, or may reflect the flow of nutrients to more densely populated areas of L cells in the distal small intestine.
Sodium-glucose cotransport protein 1 (SGLT1) is a proximal glucose transporter responsible for absorbing glucose across the intestinal brush border, and it realizes glucose concentration gradient transport by coupling the uptake of each glucose molecule to the inward flux of two Na+ ions. This SGLT1-dependent mechanism is the basis for the early elevation of plasma GLP-1 and GIP levels after carbohydrate intake (Zhu et al., 2022). In mice lacking SGLT1, peak glucose-triggered GLP-1 and GIP levels were impaired (Gorboulev et al., 2012), and L cells showed no calcium response to glucose intake (Parker et al., 2012a). GPR119 is a Gαs-coupled receptor. Its activation leads to an increase in the concentration of cyclic adenosine monophosphate (cAMP) in target cells. cAMP is an efficient gastrointestinal hormone secretion stimulator (Gribble and Reimann, 2016; Zhang et al., 2024). Monoacylglycerol binds to GPR119 in L cells to promote the release of GLP-1, GIP and insulin (Chu et al., 2008). The G protein-coupled bile acid receptor (GPBA-R) is a membrane receptor coupled to Gαs signal transduction pathway. Bile acid stimulates GLP-1 secretion from GLUTag cells in a GPBA-R dependent manner. The elevated cAMP and Ca2+ were observed following GPBA-R activation in single GLUTag cell, and bile acid and GPBA-RA increased GLP-1 secretion in intestinal cultures (Parker et al., 2012b). Guanosine and uroguanosine, which target guanosine cyclase (GC-C) receptors in the intestine and kidney, lead to elevated intracellular cGMP concentrations, and high GC-C expression in L cells is associated with regulation of GLP-1 release (Friedlander et al., 2011). These substances may be closely related to the targets of action of GLP-1RAs for the treatment of T2DM.
GLP-1 (7-36)NH2 acts as the active form, and the N-terminal end of this peptide is readily recognized by dipeptidyl peptidase-4 (DPP-4), an exopeptidase released from vascular endothelium and other tissues that can be quickly cut between the 2nd and 3rd amino acids to produce GLP-1 (9-36)NH2. The cycle half-life of GLP-1 (7-36)NH2 is 2–3 min, and the truncated metabolites are usually considered inactive. Approximately 90% of GLP-1 is metabolized by DPP-4 before reaching the central venous circulation (Holst and Deacon, 2005), and the peak circulating concentration of active GLP-1 (7-36)NH2 after a meal in healthy subjects is usually less than 10 pmol/L due to the rapid degradation of DPP-4 (Bak et al., 2014).
In order to solve the problem of rapid inactivation of GLP-1 by DPP-4 degradation, GLP-1RAs and DPP-4 inhibitors have been developed to treat T2DM (Kim and Jang, 2015; Böhm et al., 2017). GLP-1RAs have experienced a research and development process from short-acting to long-acting to ultra-long-acting, and from injection to oral administration. In 1992, exendin-4, which can stimulate insulin secretion, was isolated from Heloderma suspectum venom with 53% homology to mammalian GLP-1, and can resist DPP-4 degradation and has low renal clearance rate (Muttenthaler et al., 2021). The first GLP-1RAs Exenatide synthesized by exendin-4 was approved by the FDA in 2005 (Mariam and Niazi, 2024). Liraglutide is the second GLP-1RA to be approved after exenatide. Liraglutide is a human GLP-1 derivative, which is characterized by the retention of unaltered Ala at position 8, and structural modifications of amino acids at position 26 and 34 (Gong et al., 2024). Beinaglutide is currently the only GLP-1RA with 100% homology with human amino acid sequence (Du et al., 2020). Semaglutide’s introduction of a fatty acid side chain at position 26 Lys allows reversible binding to plasma albumin and reduces renal clearance, and substitution of amino acids at positions 8 and 34 with Aib and Arg avoids its degradation by DPP-4 and prevents fatty acid binding at the wrong site, resulting in a once-weekly dosing (Lau et al., 2015; Gong et al., 2024). The oral form prepared by Semaglutide and absorption enhancer SNAC (sodium N-[8-(2-hydroxybenzoyl) amino] caprylate), became the first oral GLP-1RA approved by the FDA for the treatment of T2DM (Kalra and Sahay, 2020; Aroda et al., 2022). GLP-1RAs can simulate the structure and function of GLP-1, thus play a role similar to GLP-1, and has a longer half-life, can persist and play a role in the body. The classification and usage of GLP-1RAs commonly used in clinic are shown in Table 1.
Transcription factors regulate gene expression by binding to specific enhancer sequences. During the development and maturation of pancreatic β cells, key transcription factors include Pancreatic/duodenal homeobox 1 (Pdx1), neurogenin 3 (NEUROG3), and V-maf musculoaponeurotic fibrosarcoma oncogene homolog A (MafA) (Zhu et al., 2017). Pdx1, in particular, plays a critical role in the development of the pancreas and the function of β cells by binding to regulatory elements and promoting the transcription of the insulin gene (Yang et al., 2012). However, prolonged exposure of β cells to high-glucose environments can lead to decreased expression levels of Pdx1 and MafA, potentially inhibiting insulin biosynthesis and secretion (Kaneto and Matsuoka, 2015). Consequently, patients with T2DM often exhibit reduced Pdx1 expression levels (Ofori et al., 2022). Cordycepin has been found to promote insulin synthesis by upregulating the mRNA levels of insulin, Pdx1, and glucose transporter 1 (GLUT1), as well as the protein expression of Pdx1, GLUT1, Akt, and phosphorylated Akt (Sun et al., 2022).
GLP-1-induced insulin synthesis is initiated by Pdx1. On one hand, GLP-1 activates PKA, increasing the expression of Pdx1 and causing Pdx1 to translocate into the nucleus. Within the nucleus, Pdx1 binds to the insulin promoter, initiating insulin expression and synthesis (Wang et al., 2001; Müller et al., 2019). On the other hand, B-cell translocation gene 2 (BTG2) can upregulate Pdx1-induced insulin gene expression. Studies have shown that GLP-1 treatment significantly increases the expression levels of BTG2, Pdx1, and the insulin gene in pancreatic β cells, indicating that GLP-1 positively regulates insulin gene expression through BTG2, thereby promoting insulin synthesis (Hwang et al., 2013).
GLP-1 stimulates insulin secretion in a glucose-dependent manner through specific receptors expressed on pancreatic βcells.GLP-1R is a family of secretins (class B) that couples GLP-1 to downstream βcell responses, and conformational changes in the receptor upon ligand binding facilitate the interaction between its intracellular region and signaling proteins (Jones et al., 2018). GLP-1R is highly expressed in pancreatic βcells, providing a clear pathway to directly promote insulin secretion. After the knockout of the GLP-1R gene, in transgenic mice expressing human GLP-1R in pancreatic islets and pancreatic ductal cells restored cAMP and serine threonine kinase (Akt) phosphorylation levels in isolated islets, increased GLP-1-stimulated insulin secretion and GLP-1R-dependent β-cell proliferation, and promoted transgenic mice for glucose regulation in response to feeding (Lamont et al., 2012). β-cell-selective knockout of the GLP-1R in mice with impaired glucose tolerance after intraperitoneal injection of glucose and exogenous supplementation of GLP-1 did not induce insulin secretion, but glucose tolerance returned to normal after oral glucose, suggesting that the extra-islet GLP-1R plays a regulatory role in oral glucose tolerance (Smith et al., 2014). GLP-1 is able to stimulate cAMP formation in RIN 1046-38 cells, which in turn causes an increase in insulin mRNA levels and insulin release, and insulin accumulation in the culture fluid was observed by adding GLP-1 (7-37) to RIN 1046-38 cells for 1 h incubation (Drucker et al., 1987). In addition, GLP-1 (7-37) concentrations as low as 50 p.m. stimulated insulin release in perfused rat pancreas (Mojsov et al., 1987).
The activation of the classical cAMP pathway is achieved by releasing irritating G protein subunit Gαs through the exchange of guanine nucleotide and the activation/dissociation of heterogeneous trimer G protein, and in pancreatic βcells, GLP-1 binds to the specific guanine nucleotide-binding protein-coupled receptor GLP-1R, leading to adenylyl cyclase activation and elevated cAMP levels (Mayo et al., 2003; Dalvi et al., 2012). cAMP is an important second messenger driving the acute insulin effect of GLP-1. Overexpression of phosphodiesterase 3B promotes cAMP hydrolysis and reduces GLP-1-induced insulin secretion, an effect evident in both isolated islets and cultured insulinoma cells (Härndahl et al., 2002). cAMP elevation induced by GLP-1 can lead to its downstream protein kinase A (PKA) activation and enhanced signaling via cAMP-activated guanine nucleotide exchange factor (Epac) (Holz, 2004; Doyle and Egan, 2007). cAMP activates PKA, which will phosphorylate the β2 subunit of the L-type VDC channel and the Kir6.2 and SUR1 subunits of the KATP channel, increasing KATP channel sensitivity to ATP and leading to KATP channel closure, cell membrane depolarization and VDC channel opening, followed by Ca2+ influx through VDC channels into βcells to promote the exocytosis of insulin granules and the acute secretion of insulin and into circulation (Bünemann et al., 1999; Müller et al., 2019) (Figure 2). GLP-1-activated PKA inhibits voltage-gated potassium channels, prevents membrane repolarization, and prolongs VDC channel opening to promote Ca2+ inward currents, contributing to GLP-1 stimulate insulin secretion, and the antagonistic effect of GLP-1R activation on Kv currents in βcells requires transactivation of epidermal growth factor receptors, accompanied by signal conduction of cAMP/PKA and phosphatidylinositol-3-kinase (PI3K)/PKCζ signaling (MacDonald et al., 2003). Both Epac1 and Epac2 are expressed in the pancreas (Leech et al., 2000), and under high glucose conditions, Ca2+ flows into βcells through VDC channels, and Epac2 opens RYR calcium channels in the endoplasmic reticulum, further increasing intracellular Ca2+ concentrations and enhancing exocytosis of insulin-secreting vesicles (Holz, 2004; Doyle and Egan, 2007). In isolated perfused rat pancreas, GLP-1 did not stimulate insulin release at glucose concentrations below 2.8 mM and could only promote insulin release when glucose concentrations were above 6.6 mM (Weir et al., 1989). As a result, compared with other hypoglycemic drugs, GLP-1RAs has a lower risk of hypoglycemia. In human islets, GLP-1 promotes increased connectivity between individual βcells to improve calcium kinetics and insulin secretion throughout the islets, and promotes insulin secretion from islet cells when βcells detect both elevated blood glucose levels and hormonal signals from the intestine.
The proliferation potential of pancreatic β cells is very limited. Under normal conditions, new β cells generated through mitosis in adult rats constitute about 3% per day, a rate that rapidly declines with age (Swenne, 1983). However, in vitro, certain nutrients and growth factors can enhance their replicative activity. The size of β cell clusters is a major determinant of the total insulin secretion by the pancreas. Expanding β cell clusters through recruitment of β cell proliferation could help maintain normal blood glucose levels, potentially compensating for β cell loss or dysfunction in diabetes (Sjöholm, 1996).
GLP-1 and GLP-1RAs promote the expansion of β-cell mass by stimulating β-cell proliferation, inducing islet neogenesis, and inhibiting β-cell apoptosis (Wajchenberg, 2007). In isolated rat islets, GLP-1 acts as a growth factor in a dose-dependent manner to increase the incorporation of tritiated thymidine and promotes cell proliferation through the upregulation of PI3K-mediated pathways (Buteau et al., 1999). cAMP is a potent mitogenic factor for β cells, and GLP-1 increases intracellular cAMP levels, thereby promoting β-cell proliferation (Sjöholm, 1996; Susini et al., 1998) (Figure 3). GLP-1 promotes islet neogenesis, lowers blood glucose levels, upregulates Pdx1 expression, and increases β-cell mass in db/db mice (Stoffers et al., 2000). Furthermore, endogenous GLP-1 receptor signaling is crucial for the adaptive proliferative response of islets to metabolic stress and pancreatic injury (Drucker, 2003). In a partially pancreatectomized model of type 2 diabetic rats, continuous treatment with GLP-1RAs for 10 days mitigated the progression of diabetes (Xu et al., 1999). Therefore, GLP-1RAs hold promise as a novel therapeutic approach for diabetic patients with reduced β-cell mass, stimulating β-cell proliferation and differentiation (Iqbal et al., 2021).
Figure 3. The mechanisms of GLP-1 stimulating β cell proliferation. IRS-2: insulin receptor substrate 2; Mek: mitogen-activated extracellular signal-regulated kinase; ERK: extracellular signal-regulated kinase.
Apoptosis plays an important role in the pathophysiology of diabetes. The reduction in β-cell mass in patients with T2DM can be attributed to β-cell apoptosis triggered by multiple factors, including islet amyloid deposition, lipotoxicity, glucotoxicity, and inflammation (Costes et al., 2021). Stress pathways induced by these factors, including endoplasmic reticulum stress, oxidative stress, mitochondrial dysfunction, and autophagy, are all involved in the induction of β-cell apoptosis, and these pathways may occur simultaneously at the same level or may interact to further exacerbate β-cell death in the form of superimposed harmful stress. In addition, some non-coding RNAs, such as Bcl-2, Ago, MafA, Pdx1, MTPN, and caspase-3, etc., also play an important role in β-cell apoptosis in T2DM patients by regulating the expression of key β-cell factors (You et al., 2022). β-cell apoptosis is mediated by a series of cascade mechanisms of the cysteine protease family, and current studies on hyperglycemia-induced β-cell apoptosis have focused on the balance between the pro-apoptotic Bcl-2 proteins (Bad, Bid, Bik, and Bax) and the anti-apoptotic Bcl family (Bcl-2 and Bcl-xl) (Tomita, 2016), and apoptosis occurs only when the concentration of the pro-apoptotic protein Bcl-2 on the mitochondrial membrane of the intrinsic pathway exceeds the concentration of the anti-apoptotic protein. In patients with T2DM, where islet neogenesis and β-cell replication are normal, increased apoptosis is the main cause of the decrease in their β-cell numbers, so preventing β-cell apoptosis may become an effective means of treating T2DM (Butler et al., 2003).
GLP-1 has a beneficial effect on β-cell function by promoting β-cell proliferation and neogenesis and reducing apoptosis (Zheng et al., 2022). GLP-1 has anti-apoptotic effects in vivo, and its promotion of cells expressing the GLP-1 receptor directly resists β-cell apoptosis (Drucker, 2003). In addition, GLP-1 increases the activity of protein kinase C (PKC) in islet β cells in a dose-dependent manner, promotes pancreatic β-cell survival, and inhibits mitochondria-dependent apoptosis by regulating Bcl-2/Bax expression levels (Zhang et al., 2015). When GLP-1 binds to GLP-1R on the surface of β-cells, cAMP-dependent PKA is activated and subsequently activates the downstream signal PI3K, which inhibits apoptosis in islet β-cells by blocking the expression of the pro-apoptotic protein Bax (Li et al., 2021) (Figure 4). IKKε phosphorylates targets in the NF-κB signaling pathway, thereby activating the NF-κB pathway. NF-κB is an important nuclear transcription factor, which can promote the regulation of inflammation and apoptosis. The expression levels of IL-6, IKK-ε and NF-κB were elevated in pancreatic cells from T2DM rats, accompanied by increased Bax expression and decreased Bcl-2 expression, as well as decreased pancreatic β-cell function; The GLP-1 receptor agonist liraglutide was able to reduce Bax expression in pancreatic cells from T2DM rats, and Bcl-2 expression was increased, islet tissue structure was improved, insulin-positive expression was increased, and β-cell apoptosis was reduced (Liu et al., 2021). Liraglutide attenuates the inflammatory response by inhibiting IKK-ε/NF-κB, thereby reducing islet β-cell apoptosis.
Considered an anti-insulin hormone, 2022 marks the 100th anniversary of the discovery of glucagon, which is produced in islet α cells through the processing of prohormone-converting enzyme 2 (Pcsk2) and acts through glucagon receptor (GCGR) (Hayashi, 2011). Under normal physiological conditions, glucagon secreted by pancreatic islet α cells plays an important role in maintaining blood glucose homeostasis by regulating hepatic glucose production. Fasting and postprandial hyperglucagonemia in T2DM patients stimulates glucose production in the liver, leading to hyperglycemia. Inhibition of glucagon secretion or antagonism of glucagon receptors has become a potentially effective treatment strategy for T2DM patients (Lund et al., 2014). Both glucagon and GLP-1 receptors belong to the GPCR superfamily. Glucagon acts in the liver via cAMP (Abbas et al., 2020), and glucagon is secreted by α cells as an immediate response to glucose reduction. Compared with the saline group, the physiological dose of GLP-1 injected during the hyperglycemic clamp can significantly reduce the glucagon level in post-transplantation diabetic patients, suggesting that GLP-1 has a clear glucagon inhibitory effect and can restore the altered insulin and glucagon secretion in post-transplantation diabetic patients in a glucose-dependent manner (Halden et al., 2016). Giving exogenous GLP-1 analogues or increasing endogenous GLP-1 levels can enhance the signaling of GLP-1R, and the activation of GLP-1R can effectively inhibit glucagon secretion in human body. The inhibitory effect of GLP-1 on glucagon secretion in vivo was observed only when the blood glucose level was equal to or higher than the fasting level, at higher blood glucose levels, the inhibition of glucagon gradually increased (Holst, 2007), and when the blood glucose concentration was less than 3.7 m mol/L, GLP-1 lost its effect on inhibiting glucagon secretion (Nauck et al., 2002). GLP-1R expression on α cells is low. In pancreas, the main expression sites of GLP-1R are located in pancreatic β cells and δ cells (Richards et al., 2014), and the expression of GLP-1R in α cells was about 0.2% of that in β cells (De Marinis et al., 2010), or even absent (Tornehave et al., 2008). Therefore, indirect regulation, via β- or δ-cell products has been thought to be the primary mechanism by which GLP-1 inhibits glucagon secretion (Gromada et al., 2007; Christensen et al., 2011; Müller et al., 2019; Davis and Sandoval, 2020). However, GLP-1 can activate GCGR in α cells, and GCGR is able to reduce the number of docking granules by coupling and activating inhibitory GTP binding protein (Gi/o), thus inhibiting the secretion of glucagon, which is independent of GLP-1R (Gandasi et al., 2024). The mechanisms of GLP-1 inhibiting glucagon secretion are shown in Figure 5.
Figure 5. Mechanisms of GLP-1 inhibition of glucagon secretion. GLP-1 can activate GCGR in α cells, and GCGR is able to reduce the number of docking granules by coupling and activating Gi/o, thus inhibiting the secretion of glucagon. Gi/o: an inhibitory GTP-binding protein; SG: secretory granules; GCGR: glucagon receptor.
GLP-1RAs has been shown to delay gastric emptying (Jelsing et al., 2012), thereby extending the retention of food in the stomach and small intestine and reducing postprandial glucose levels (Flint et al., 2011). GLP-1 is an energy sensor that reduces intestinal motility, prolonging the retention of food in the gut, contributing to more efficient absorption of nutrients and better bioavailability (Winkler et al., 2019). GLP-1 infusion slows gastric emptying of solid and liquid components of food and alters the distribution of food in the stomach so that a greater proportion of food is retained in the distal stomach (Marathe et al., 2011). Even intravenous administration of GLP-1 at a physiological postprandial dose of 0.3 pmol/kg/min significantly slowed the rate of gastric emptying in healthy subjects, bringing them into a state of “gastroparesis” (Little et al., 2006). Exogenous GLP-1 improves gastric compliance in a dose-dependent manner (Schirra et al., 2002), reduces the frequency and amplitude of gastric antrum and duodenum contraction, inhibits the contractile force of gastric antrum and duodenum, increases pyloric tension, and stimulates pyloric tetanic and phase motion (Schirra et al., 2000). Endogenous GLP-1 affects postprandial blood glucose by slowing gastric emptying and glucose absorption in healthy subjects (Deane et al., 2010) by inhibiting antral and duodenal motility and stimulating pyloric motility (Schirra et al., 2006). Other studies (Delgado-Aros et al., 2002) have shown that GLP-1 can increase fasting and postprandial stomach capacity and delay gastric emptying by inhibiting the cholinergic function of vagus nerve, without inducing postprandial symptoms. GLP-1 also helps with ileal braking, a circuit that regulates the rate of gastric emptying so that the rate of nutrients entering the duodenum is balanced with that of nutrients in the upper small intestine. If the flow of nutrients from the stomach is too fast, duodenum and jejunum absorption is incomplete, and the increased nutrient load in the ileum triggers increased GLP-1 and PYY secretion, which in turn feeds back regulation and further slows gastric emptying, thus restoring balance to the system. To sum up, the potential mechanisms of delayed gastric emptying by GLP-1 are mainly related to: (1) decreasing the contractile force of the gastric antrum and duodenum and increasing the pyloric tension (Schirra et al., 2000; Schirra et al., 2006); (2) inhibiting the cholinergic function of the vagus nerve (Delgado-Aros et al., 2002); (3) ileal braking (Layer et al., 1995; Holst, 2022).
Appetite and weight regulation are controlled by the central nervous system in a fairly complex manner, with the human brain playing a central role in integrating internal and external inputs and regulating energy homeostasis (Farr et al., 2016a). Farr et al. first demonstrated that GLP-1 receptors exist in the parietal cortex, hypothalamus and medulla of the human brain and are involved in regulating food intake (Farr et al., 2016b). GLP1-RAs is clinically used to treat T2DM and promote weight loss in obese individuals. Liraglutide can enter specific brain regions associated with appetite regulation, electrophysiological measurements of mouse brain sections showed that GLP-1 directly stimulated promelanocortin (POMC)/cocaine and amphetamine regulated transcript (CART) neurons and indirectly inhibited neurotransmission of neuropeptide Y (NPY) and agouti-related peptide (AgRP) in arcuate nucleus neurons through GABA-dependent signal transduction. GLP-1R on arcuate nucleus neurons expressing POMC/CART can mediate Liraglutide-induced weight loss (Secher et al., 2014). GLP-1RAs directly stimulate POMC/CART neurons and indirectly inhibit NPY and AgRP to increase measures of satiety and decrease hunger, resulting in reduced energy intake, thereby facilitating weight loss (Ard et al., 2021) (Figure 6). Consumption of high-calorie, high-sugar and high-fat foods is conducive to the development and maintenance of obesity (Berthoud and Zheng, 2012). Obese subjects were given liraglutide 3 mg once a day for 16 weeks. Compared with placebo group, liraglutide increased subjects’ satiety, reduced intake of sweet, salty and high-fat foods, and increased plasma PYY level after meals. As well as reduced fat storage throughout the body, trunk, and upper and lower body, these results suggest that liraglutide has a central role in altering appetite cravings (Kadouh et al., 2020). GLP-1RAs affects the central nervous system, including reducing appetite, improving diet control, increasing satiety, reducing calorie intake, etc. In general, GLP-1RAs provides a highly effective and well-tolerated treatment regimen to help obese patients lose weight while improving weight-related complications (Ard et al., 2021).
GLP-1RAs can be divided into two categories: animal GLP-1 and its derivatives and human GLP-1 and its derivatives, which has experienced a development process from short-acting to long-acting to ultra-long-acting (Ji, 2022). Compared with placebo, GLP-1RAs treatment in T2DM patients significantly reduced the risk of death from cardiovascular disease and fatal/non-fatal stroke in cardiovascular outcome trials (Qin and Song, 2022). GLP-1RAs contributes to weight loss and lower glycosylated hemoglobin (HbA1c), and has a renal protective effect and is preferred in patients with higher cardiovascular risk T2DM and those considering weight loss (Ng et al., 2022). In addition, GLP-1RAs also have the effects of anti-inflammation, neuroprotection, lowering blood pressure, improving blood lipid profile and protecting liver (CSE and CDS, 2020; Zhao et al., 2021; Ajabnoor et al., 2023) and can benefit specific subgroups of T2DM patients: T2DM patients at risk of cardiovascular disease, kidney disease, non-alcoholic fatty liver disease, cognitive impairment, etc. Compared with other non-insulin hypoglycaemic agents, GLP-1RAs have the advantage of lowering HbA1c by a large margin and improving cardiovascular system and renal function without causing hypoglycaemia and weight gain (Table 2). In GLP-1RAs, Semaglutide is better than Dulaglutide in lowering HbA1c concentration (Chen et al., 2023). And Semaglutide effectively reduces low-density lipoprotein and total cholesterol levels, and performs best in weight loss (Xie et al., 2023; Yao et al., 2024).
However, as polypeptide drugs, GLP-1RAs are easily hydrolyzed by enzymes in the gastrointestinal environment. Coupled with their high molecular weight and high polarity, it is usually difficult to penetrate the gastrointestinal epithelial cell membrane, resulting in low oral bioavailability. Most of the GLP-1RAs on the market are injection forms, and the development of oral dosage forms is very important to shape the potential of GLP-1RAs market in the future. Common side effects of GLP-1RAs include gastrointestinal reactions such as nausea, vomiting and diarrhea (Chin et al., 2023; Aldhaleei et al., 2024), which may lead to a decline in the quality of life and even lead to drug withdrawal in some patients. Although the risk of hypoglycemia in GLP-1RAs is low, when used in combination with other hypoglycemic drugs or improper dose adjustment, patients may still have symptoms of hypoglycemia (Wolffenbuttel et al., 2023). In addition, long-term use of GLP-1RAs may also have potential safety risks, such as pancreatitis (Shetty et al., 2022) and thyroid diseases (Bezin et al., 2023), which require continuous monitoring and evaluation.
T2DM is a chronic disease characterized by hyperglycemia, which eventually leads to microvascular damage and macrovascular events, as well as complications related to peripheral vascular diseases. Patients with obesity, hypertension, lipid disorders and various other risk factors have significantly increased rates of complications (Nauck et al., 2021b). In addition to a healthy lifestyle and prevention and treatment specifically aimed at diabetes-related complications, blood glucose control remains the primary approach to T2DM management.
GLP-1RAs may be the drugs of choice for the treatment of diabetes because of their cardioprotective, neuroprotective and renal protective activities other than lowering blood glucose, while not causing side effects of hypoglycemia and weight gain, with a proven efficacy and safety (Sharma et al., 2018; Nauck et al., 2021a). Other benefits of ultra-long-acting GLP-1RAs include less fluctuation in blood concentration, improved gastrointestinal tolerance, and a simpler and more convenient once-a-week dosing regimen that improves the compliance of patients and the persistence of treatment (Gentilella et al., 2019). Although a large number of short-term and medium-term clinical studies support the safety and efficacy of GLP-1RAs, there are insufficient data on the presence of delayed side effects with their long-term (decades) use (Guirguis et al., 2024). The response of different patients to GLP-1RAs may be different, and some patients may not be able to achieve the expected hypoglycemic effect. This may be related to genetic background, disease progression, islet function, obesity, lifestyle and other factors. It is a challenge to predict the individual response to GLP-1RAs through accurate medical treatment and optimize the treatment plan accordingly. T2DM patients are often accompanied by other comorbidities and need to take multiple drugs at the same time. The interactions between GLP-1RAs and other drugs may affect drug efficacy or increase the risk of adverse reactions, as well as the dose adjustment of the combination of hypoglycemic drugs, which put forward higher requirements for clinicians. GLP-1RAs usually belong to biological agents, and the production processes are complex, resulting in relatively expensive drug price. For some patients, especially those who do not have adequate medical insurance coverage, long-term use of such drugs may impose a heavy financial burden. Therefore, it is particularly important to reduce the costs of GLP-1RAs research and development.
Overall, GLP-1RAs is a relatively safe and effective new hypoglycemic drug, which helps to delay the occurrence and development of diabetes. At present, several kinds of intestinal hormone co-agonists are under development and have made progress through clinical trials. GLP-1-GIP co-agonist tirzepatide was approved by the US Food and Drug Administration in 2022 for the treatment of T2DM (Nogueiras et al., 2023). With the gradual deepening of the research and the continuous improvement of clinical status, GLP-1RAs brings new hope for the comprehensive treatment of T2DM patients.
XH: Conceptualization, Data curation, Formal Analysis, Methodology, Writing–original draft. WZ: Conceptualization, Investigation, Methodology, Writing–original draft. PHL: Conceptualization, Software, Writing–original draft. YJZ: Conceptualization, Formal Analysis, Writing–original draft. GHL: Methodology, Writing–original draft. HYS: Visualization, Writing–original draft. BNL: Project administration, Supervision, Writing–review and editing. ZRP: Funding acquisition, Project administration, Supervision, Writing–review and editing.
The author(s) declare that financial support was received for the research, authorship, and/or publication of this article. This work was supported by the National Natural Science Foundation of China (Grant No. 82374431).
The authors declare that the research was conducted in the absence of any commercial or financial relationships that could be construed as a potential conflict of interest.
All claims expressed in this article are solely those of the authors and do not necessarily represent those of their affiliated organizations, or those of the publisher, the editors and the reviewers. Any product that may be evaluated in this article, or claim that may be made by its manufacturer, is not guaranteed or endorsed by the publisher.
GLP-1, glucagon-like peptide-1; GLP-1R, glucagon-like peptide-1 receptor; GLP-1RAs, glucagon-like peptide-1 receptor agonists; T2DM, type 2 diabetes mellitus; GIP, glucose-dependent insulin-stimulating peptide; GLP-2, glucagon-like peptide-2; GCGR, glucagon receptor; DPP-4, dipeptidyl peptidase-4; SCFAs, short chain fatty acids; GPCRs, G protein-coupled receptors; PYY, polypeptide YY; SGLT1, sodium-glucose cotransport protein 1; cAMP, cyclic adenosine monophosphate; GC-C, guanosine cyclase; Akt, serine threonine kinase; PKA, protein kinase A; Epac, guanine nucleotide exchange factor; PI3K, phosphatidylinositol-3-kinase; Pdx1, Pancreatic/duodenal homologous protein 1; NEUROG3, neurogenin 3; MafA, V-maf musculoaponeurotic fibrosarcoma oncogene homolog A; GLUT1, glucose transporter protein 1; BTG2, B-cell translocation gene 2; PKC, protein kinase C; POMC, promelanocortin; CART, cocaine and amphetamine regulated transcript; HbA1c, glycosylated hemoglobin.
Abbas, G., Haq, Q. M. I., Hamaed, A., Al-Sibani, M., and Hussain, H. (2020). Glucagon and glucagon-like peptide-1 receptors: promising therapeutic targets for an effective management of diabetes mellitus. Curr. Pharm. Des. 26 (4), 501–508. doi:10.2174/1381612826666200131143231
Ahrén, B., and Gutniak, M. (1997). No correlation between insulin and islet amyloid polypeptide after stimulation with glucagon-like peptide-1 in type 2 diabetes. Eur. J. Endocrinol. 137 (6), 643–649. doi:10.1530/eje.0.1370643
Ajabnoor, G. M. A., Hashim, K. T., Alzahrani, M. M., Alsuheili, A. Z., Alharbi, A. F., Alhozali, A. M., et al. (2023). The possible effect of the long-term use of glucagon-like peptide-1 receptor agonists (GLP-1RA) on Hba1c and lipid profile in type 2 diabetes mellitus: a retrospective study in KAUH, Jeddah, Saudi Arabia. Diseases 11 (1), 50. doi:10.3390/diseases11010050
Aldhaleei, W. A., Abegaz, T. M., and Bhagavathula, A. S. (2024). Glucagon-like peptide-1 receptor agonists associated gastrointestinal adverse events: a cross-sectional analysis of the national institutes of health all of us cohort. Pharmaceuticals 17 (2), 199. doi:10.3390/ph17020199
Ard, J., Fitch, A., Fruh, S., and Herman, L. (2021). Weight loss and maintenance related to the mechanism of action of glucagon-like peptide 1 receptor agonists. Adv. Ther. 38 (6), 2821–2839. doi:10.1007/s12325-021-01710-0
Aroda, V. R., Blonde, L., and Pratley, R. E. (2022). A new era for oral peptides: SNAC and the development of oral semaglutide for the treatment of type 2 diabetes. Rev. Endocr. Metabolic Disord. 23 (5), 979–994. doi:10.1007/s11154-022-09735-8
Bak, M. J., Wewer Albrechtsen, N. J., Pedersen, J., Knop, F. K., Vilsbøll, T., Jørgensen, N. B., et al. (2014). Specificity and sensitivity of commercially available assays for glucagon-like peptide-1 (GLP-1): implications for GLP-1 measurements in clinical studies. Diabetes Obes. and Metabolism 16 (11), 1155–1164. doi:10.1111/dom.12352
Bell, G. I., Santerre, R. F., and Mullenbach, G. T. (1983). Hamster preproglucagon contains the sequence of glucagon and two related peptides. Nature 302 (5910), 716–718. doi:10.1038/302716a0
Berthoud, H.-R., and Zheng, H. (2012). Modulation of taste responsiveness and food preference by obesity and weight loss. Physiology and Behav. 107 (4), 527–532. doi:10.1016/j.physbeh.2012.04.004
Bezin, J., Gouverneur, A., Pénichon, M., Mathieu, C., Garrel, R., Hillaire-Buys, D., et al. (2023). GLP-1 receptor agonists and the risk of thyroid cancer. Diabetes Care 46 (2), 384–390. doi:10.2337/dc22-1148
Böhm, A., Wagner, R., Machicao, F., Holst, J. J., Gallwitz, B., Stefan, N., et al. (2017). DPP4 gene variation affects GLP-1 secretion, insulin secretion, and glucose tolerance in humans with high body adiposity. Plos One 12 (7), e0181880. doi:10.1371/journal.pone.0181880
Bünemann, M., Gerhardstein, B. L., Gao, T., and Hosey, M. M. (1999). Functional regulation of L-type calcium channels via protein kinase A-mediated phosphorylation of the beta(2) subunit. J. Biol. Chem. 274 (48), 33851–33854. doi:10.1074/jbc.274.48.33851
Buteau, J., Roduit, R., Susini, S., and Prentki, M. (1999). Glucagon-like peptide-1 promotes DNA synthesis, activates phosphatidylinositol 3-kinase and increases transcription factor pancreatic and duodenal homeobox gene 1 (PDX-1) DNA binding activity in beta (INS-1)-cells. Diabetologia 42 (7), 856–864. doi:10.1007/s001250051238
Butler, A. E., Janson, J., Bonner-Weir, S., Ritzel, R., Rizza, R. A., and Butler, P. C. (2003). Beta-cell deficit and increased beta-cell apoptosis in humans with type 2 diabetes. Diabetes 52 (1), 102–110. doi:10.2337/diabetes.52.1.102
Chen, H., Li, X., Chen, J., Ren, T., Zhang, Y., Wang, Y., et al. (2023). Comparative efficacy and safety of glucagon-like peptide 1 receptor agonists for the treatment of type 2 diabetes: a network meta-analysis. Medicina 102 (27), e34122. doi:10.1097/MD.0000000000034122
Chin, R., Nagaoka, S., Nakasawa, H., Tanaka, Y., and Inagaki, N. (2023). Safety and effectiveness of dulaglutide 0.75 mg in Japanese patients with type 2 diabetes in real-world clinical practice: 36 month post-marketing observational study. J. Diabetes Investigation 14 (2), 247–258. doi:10.1111/jdi.13932
Christensen, M., Bagger, J. I., Vilsboll, T., and Knop, F. K. (2011). The alpha-cell as target for type 2 diabetes therapy. Rev. Diabet. Stud. 8 (3), 369–381. doi:10.1900/rds.2011.8.369
Chu, Z.-L., Carroll, C., Alfonso, J., Gutierrez, V., He, H., Lucman, A., et al. (2008). A role for intestinal endocrine cell-expressed G protein-coupled receptor 119 in glycemic control by enhancing glucagon-like peptide-1 and glucose-dependent insulinotropic peptide release. Endocrinology 149 (5), 2038–2047. doi:10.1210/en.2007-0966
Clark, L. (2024). GLP-1 receptor agonists: a review of glycemic benefits and beyond. JAAPA 37 (4), 1–4. doi:10.1097/01.Jaa.0001007388.97793.41
Costes, S., Bertrand, G., and Ravier, M. A. (2021). Mechanisms of beta-cell apoptosis in type 2 diabetes-prone situations and potential protection by GLP-1-based therapies. Int. J. Mol. Sci. 22 (10), 5303. doi:10.3390/ijms22105303
Cse, C. S. o.E. (2018). Expert consensus of combined oral hypoglycemic medication for Chinese adult type 2 diabetes. Drug Eval. 15 (A01), 5–14+22. doi:10.3969/j.issn.1672-2809.2018.z1.001
Cse, C. S. o.E., and Cds, C. D. S. (2020). Consensus recommendations on utilizing glucagon-like peptide-1 (GLP-1) receptor agonists in the treatment of type 2 diabetes mellitus. Chin. J. Intern. Med. 59 (11), 836–846. doi:10.3760/cma.j.cn112138-20200704-00646
Dalvi, P. S., Nazarians-Armavil, A., Purser, M. J., and Belsham, D. D. (2012). Glucagon-like peptide-1 receptor agonist, exendin-4, regulates feeding-associated neuropeptides in hypothalamic neurons in vivo and in vitro. Endocrinology 153 (5), 2208–2222. doi:10.1210/en.2011-1795
Davis, E. M., and Sandoval, D. A. (2020). Glucagon-like peptide-1: actions and influence on pancreatic hormone function. Compr. Physiol. 10 (2), 577–595. doi:10.1002/cphy.c190025
Deane, A. M., Nguyen, N. Q., Stevens, J. E., Fraser, R. J. L., Holloway, R. H., Besanko, L. K., et al. (2010). Endogenous glucagon-like peptide-1 slows gastric emptying in healthy subjects, attenuating postprandial glycemia. J. Clin. Endocrinol. and Metabolism 95 (1), 215–221. doi:10.1210/jc.2009-1503
Delgado-Aros, S., Kim, D.-Y., Burton, D. D., Thomforde, G. M., Stephens, D., Brinkmann, B. H., et al. (2002). Effect of GLP-1 on gastric volume, emptying, maximum volume ingested, and postprandial symptoms in humans. Am. J. Physiology-Gastrointestinal Liver Physiology 282 (3), G424–G431. doi:10.1152/ajpgi.2002.282.3.G424
De Marinis, Y. Z., Salehi, A., Ward, C. E., Zhang, Q., Abdulkader, F., Bengtsson, M., et al. (2010). GLP-1 inhibits and adrenaline stimulates glucagon release by differential modulation of N- and L-type Ca2+ channel-dependent exocytosis. Cell Metab. 11 (6), 543–553. doi:10.1016/j.cmet.2010.04.007
de Vos, W. M., Tilg, H., Van Hul, M., and Cani, P. D. (2022). Gut microbiome and health: mechanistic insights. Gut 71 (5), 1020–1032. doi:10.1136/gutjnl-2021-326789
Doyle, M. E., and Egan, J. M. (2007). Mechanisms of action of glucagon-like peptide 1 in the pancreas. Pharmacol. and Ther. 113 (3), 546–593. doi:10.1016/j.pharmthera.2006.11.007
Drucker, D. J. (2003). Glucagon-like peptides: regulators of cell proliferation, differentiation, and apoptosis. Mol. Endocrinol. 17 (2), 161–171. doi:10.1210/me.2002-0306
Drucker, D. J., and Holst, J. J. (2023). The expanding incretin universe: from basic biology to clinical translation. Diabetologia 66 (10), 1765–1779. doi:10.1007/s00125-023-05906-7
Drucker, D. J., Philippe, J., Mojsov, S., Chick, W. L., and Habener, J. F. (1987). Glucagon-like peptide I stimulates insulin gene expression and increases cyclic AMP levels in a rat islet cell line. Proc. Natl. Acad. Sci. U. S. A. 84 (10), 3434–3438. doi:10.1073/pnas.84.10.3434
Du, X., Jin, J., Shang, Q., Kong, X., Tang, L., and Liu, W. (2020). Advances in the study of marketed glucagon-like peptide-1 receptor agonists. Drug Eval. Res. 43 (3), 559–564. doi:10.7501/j.issn.1674-6376.2020.03.037
Edgard, Z., and Jean, L. B. (2017). Contributions a l’étude des variations physiologiques de la sécrétion interne du pancréas relations entre les sécrétions externe et interne du pancréas. Arch. Int. Physiol. 31 (1), 20–44. doi:10.1080/13813455.1929.11864670
Elrick, H., Stimmler, L., Hlad Jr, C. J., and Arai, Y. (1964). Plasma insulin response to oral and intravenous glucose administration. J. Of Clin. Endocrinol. and Metabolism 24, 1076–1082. doi:10.1210/jcem-24-10-1076
Everard, A., Lazarevic, V., Derrien, M., Girard, M., Muccioli, G. G., Neyrinck, A. M., et al. (2011). Responses of gut microbiota and glucose and lipid metabolism to prebiotics in genetic obese and diet-induced leptin-resistant mice. Diabetes 60 (11), 2775–2786. doi:10.2337/db11-0227
Farr, O. M., Li, C.-s.R., and Mantzoros, C. S. (2016a). Central nervous system regulation of eating: insights from human brain imaging. Metabolism 65 (5), 699–713. doi:10.1016/j.metabol.2016.02.002
Farr, O. M., Sofopoulos, M., Tsoukas, M. A., Dincer, F., Thakkar, B., Sahin-Efe, A., et al. (2016b). GLP-1 receptors exist in the parietal cortex, hypothalamus and medulla of human brains and the GLP-1 analogue liraglutide alters brain activity related to highly desirable food cues in individuals with diabetes: a crossover, randomised, placebo-controlled trial. Diabetologia 59 (5), 954–965. doi:10.1007/s00125-016-3874-y
Flint, A., Kapitza, C., Hindsberger, C., and Zdravkovic, M. (2011). The once-daily human glucagon-like peptide-1 (GLP-1) analog liraglutide improves postprandial glucose levels in type 2 diabetes patients. Adv. Ther. 28 (3), 213–226. doi:10.1007/s12325-010-0110-x
Friedlander, R. S., Moss, C. E., Mace, J., Parker, H. E., Tolhurst, G., Habib, A. M., et al. (2011). Role of phosphodiesterase and adenylate cyclase isozymes in murine colonic glucagon-like peptide 1 secreting cells. Br. J. Pharmacol. 163 (2), 261–271. doi:10.1111/j.1476-5381.2010.01107.x
Gandasi, N. R., Gao, R., Kothegala, L., Pearce, A., Santos, C., Acreman, S., et al. (2024). GLP-1 metabolite GLP-1(9–36) is a systemic inhibitor of mouse and human pancreatic islet glucagon secretion. Diabetologia 67 (3), 528–546. doi:10.1007/s00125-023-06060-w
Gao, Y., Yang, G., and Zhou, Y. (2014). Expert consensus on the application of oral hypoglycemic drugs in patients with cardiovascular disease complicated with diabetes mellitus. Diabetes World 8 (10), 440–446. doi:10.3969/j.issn.1672-7851.2013.10.002
Gentilella, R., Pechtner, V., Corcos, A., and Consoli, A. (2019). Glucagon-like peptide-1 receptor agonists in type 2 diabetes treatment: are they all the same? Diabetes/Metabolism Res. Rev. 35 (1), e3070. doi:10.1002/dmrr.3070
Glovaci, D., Fan, W., and Wong, N. D. (2019). Epidemiology of diabetes mellitus and cardiovascular disease. Curr. Cardiol. Rep. 21 (4), 21. doi:10.1007/s11886-019-1107-y
Gong, B., Yao, Z., Zhou, C., Wang, W., Sun, L., and Han, J. (2024). Glucagon-like peptide-1 analogs: miracle drugs are blooming? Eur. J. Med. Chem. 269, 116342. doi:10.1016/j.ejmech.2024.116342
Gorboulev, V., Schürmann, A., Vallon, V., Kipp, H., Jaschke, A., Klessen, D., et al. (2012). Na(+)-D-glucose cotransporter SGLT1 is pivotal for intestinal glucose absorption and glucose-dependent incretin secretion. Diabetes 61 (1), 187–196. doi:10.2337/db11-1029
Gribble, F. M., and Reimann, F. (2016). Enteroendocrine cells: chemosensors in the intestinal epithelium. Annu. Rev. Physiology 78 (1), 277–299. doi:10.1146/annurev-physiol-021115-105439
Gribble, F. M., and Reimann, F. (2021). Metabolic Messengers: glucagon-like peptide 1. Nat. Metab. 3 (2), 142–148. doi:10.1038/s42255-020-00327-x
Gromada, J., Franklin, I., and Wollheim, C. B. (2007). Alpha-cells of the endocrine pancreas: 35 years of research but the enigma remains. Endocr. Rev. 28 (1), 84–116. doi:10.1210/er.2006-0007
Guirguis, A., Chiappini, S., Papanti P, G. D., Vickers-Smith, R., Harris, D., Corkery, J. M., et al. (2024). Exploring the association between suicidal thoughts, self-injury, and GLP-1 receptor agonists in weight loss treatments: insights from pharmacovigilance measures and unmasking analysis. Eur. Neuropsychopharmacol. 82, 82–91. doi:10.1016/j.euroneuro.2024.02.003
Halden, T. A. S., Egeland, E. J., Åsberg, A., Hartmann, A., Midtvedt, K., Khiabani, H. Z., et al. (2016). GLP-1 restores altered insulin and glucagon secretion in posttransplantation diabetes. Diabetes Care 39 (4), 617–624. doi:10.2337/dc15-2383
Härndahl, L., Jing, X.-J., Ivarsson, R., Degerman, E., Ahrén, B., Manganiello, V. C., et al. (2002). Important role of phosphodiesterase 3B for the stimulatory action of cAMP on pancreatic beta-cell exocytosis and release of insulin. J. Biol. Chem. 277 (40), 37446–37455. doi:10.1074/jbc.M205401200
Hayashi, Y. (2011). Metabolic impact of glucagon deficiency. Diabetes, Obes. Metabolism 13 (s1), 151–157. doi:10.1111/j.1463-1326.2011.01456.x
Heinrich, G., Gros, P., Lund, P. K., Bentley, R. C., and Habener, J. F. (1984). Pre-proglucagon messenger ribonucleic acid: nucleotide and encoded amino acid sequences of the rat pancreatic complementary deoxyribonucleic acid. Endocrinology 115 (6), 2176–2181. doi:10.1210/endo-115-6-2176
Holst, J. J. (2007). The physiology of glucagon-like peptide 1. Physiol. Rev. 87 (4), 1409–1439. doi:10.1152/physrev.00034.2006
Holst, J. J. (2022). Discovery of the GI effects of GLP-1: an historical perspective. Dig. Dis. Sci. 67 (7), 2716–2720. doi:10.1007/s10620-022-07519-3
Holst, J. J., and Deacon, C. F. (2005). Glucagon-like peptide-1 mediates the therapeutic actions of DPP-IV inhibitors. Diabetologia 48 (4), 612–615. doi:10.1007/s00125-005-1705-7
Holz, G. G. (2004). Epac: a new cAMP-binding protein in support of glucagon-like peptide-1 receptor-mediated signal transduction in the pancreatic beta-cell. Diabetes 53 (1), 5–13. doi:10.2337/diabetes.53.1.5
Hu, E.-H., Tsai, M.-L., Lin, Y., Chou, T.-S., and Chen, T.-H. (2024). A review and meta-analysis of the safety and efficacy of using glucagon-like peptide-1 receptor agonists. Medicina 60 (3), 357. doi:10.3390/medicina60030357
Hwang, S.-L., Kwon, O., Kim, S.-G., Lee, I.-K., and Kim, Y. D. (2013). B-cell translocation gene 2 positively regulates GLP-1-stimulated insulin secretion via induction of PDX-1 in pancreatic β-cells. Exp. and Mol. Med. 45 (5), e25. doi:10.1038/emm.2013.47
Iqbal, A. M., Imamudeen, N., Basheer, A., Menon, S., Mohan, G., Sani, T. N., et al. (2021). Efficacy and cardiovascular safety of GLP-1 receptor analogues. Curr. Drug Saf. 16 (2), 197–206. doi:10.2174/1574886315999201208212356
Jelsing, J., Vrang, N., Hansen, G., Raun, K., Tang-Christensen, M., and Knudsen, L. B. (2012). Liraglutide: short-lived effect on gastric emptying—long lasting effects on body weight. Diabetes, Obes. Metabolism 14 (6), 531–538. doi:10.1111/j.1463-1326.2012.01557.x
Ji, L. (2022). Evidence and expert guidance of glucagon-like peptide-1 receptor agonist weekly preparation in China. Chin. J. Diabetes 30 (6), 405–411. doi:10.3969/j.issn.1006-6187.2022.06.002
Jones, B., Bloom, S. R., Buenaventura, T., Tomas, A., and Rutter, G. A. (2018). Control of insulin secretion by GLP-1. Peptides 100, 75–84. doi:10.1016/j.peptides.2017.12.013
Jørgensen, N. B., Jacobsen, S. H., Dirksen, C., Bojsen-Møller, K. N., Naver, L., Hvolris, L., et al. (2012). Acute and long-term effects of Roux-en-Y gastric bypass on glucose metabolism in subjects with Type 2 diabetes and normal glucose tolerance. Am. J. Physiology-Endocrinology Metabolism 303 (1), E122–E131. doi:10.1152/ajpendo.00073.2012
Kadouh, H., Chedid, V., Halawi, H., Burton, D. D., Clark, M. M., Khemani, D., et al. (2020). GLP-1 analog modulates appetite, taste preference, gut hormones, and regional body fat stores in adults with obesity. J. Clin. Endocrinol. and Metabolism 105 (5), 1552–1563. doi:10.1210/clinem/dgz140
Kalra, S., and Sahay, R. (2020). A review on semaglutide: an oral glucagon-like peptide 1 receptor agonist in management of type 2 diabetes mellitus. Diabetes Ther. 11 (9), 1965–1982. doi:10.1007/s13300-020-00894-y
Kaneto, H., and Matsuoka, T.-a. (2015). Role of pancreatic transcription factors in maintenance of mature β-cell function. Int. J. Mol. Sci. 16 (3), 6281–6297. doi:10.3390/ijms16036281
Kim, K.-S., and Jang, H.-J. (2015). Medicinal plants qua glucagon-like peptide-1 secretagogue via intestinal nutrient sensors. Evidence-Based Complementary Altern. Med. 2015, 171742. doi:10.1155/2015/171742
Lamont, B. J., Li, Y., Kwan, E., Brown, T. J., Gaisano, H., and Drucker, D. J. (2012). Pancreatic GLP-1 receptor activation is sufficient for incretin control of glucose metabolism in mice. J. Clin. Investigation 122 (1), 388–402. doi:10.1172/jci42497
Lau, J., Bloch, P., Schäffer, L., Pettersson, I., Spetzler, J., Kofoed, J., et al. (2015). Discovery of the once-weekly glucagon-like peptide-1 (GLP-1) analogue semaglutide. J. Med. Chem. 58 (18), 7370–7380. doi:10.1021/acs.jmedchem.5b00726
Layer, P., Holst, J. J., Grandt, D., and Goebell, H. (1995). Ileal release of glucagon-like peptide-1 (GLP-1). Association with inhibition of gastric acid secretion in humans. Dig. Dis. Sci. 40 (5), 1074–1082. doi:10.1007/BF02064202
Leech, C. A., Holz, G. G., Chepurny, O., and Habener, J. F. (2000). Expression of cAMP-regulated guanine nucleotide exchange factors in pancreatic beta-cells. Biochem. Biophysical Res. Commun. 278 (1), 44–47. doi:10.1006/bbrc.2000.3763
Li, D.-j., Yue, Q., Liu, L., Che, K.-k., Liu, X.-m., and Hu, C.-h. (2021). Brexpiprazole caused glycolipid metabolic disorder by inhibiting GLP1/GLP1R signaling in rats. Acta Pharmacol. Sin. 42 (8), 1267–1279. doi:10.1038/s41401-021-00680-x
Little, T. J., Pilichiewicz, A. N., Russo, A., Phillips, L., Jones, K. L., Nauck, M. A., et al. (2006). Effects of intravenous glucagon-like peptide-1 on gastric emptying and intragastric distribution in healthy subjects: relationships with postprandial glycemic and insulinemic responses. J. Clin. Endocrinol. and Metabolism 91 (5), 1916–1923. doi:10.1210/jc.2005-2220
Liu, J., and Zhu, D. (2022). Consensus of Chinese experts on oral hypoglycemic drugs combined with initial insulin in the treatment of type 2 diabetes mellitus. Chin. J. Diabetes 30 (5), 321–331. doi:10.3969/j.issn.1006⁃6187.2022.05.001
Liu, Y. T., He, T., Li, H. Q., and Jiang, P. (2021). Liraglutide improves pancreatic islet β cell apoptosis in rats with type 2 diabetes mellitus by inhibiting the IKKε/NF-κB pathway. Eur. Rev. Med. Pharmacol. Sci. 25 (14), 4818–4828. doi:10.26355/eurrev_202107_26395
Lopez, L. C., Frazier, M. L., Su, C. J., Kumar, A., and Saunders, G. F. (1983). Mammalian pancreatic preproglucagon contains three glucagon-related peptides. Proc. Natl. Acad. Sci. U. S. A. 80 (18), 5485–5489. doi:10.1073/pnas.80.18.5485
Lovic, D., Piperidou, A., Zografou, I., Grassos, H., Pittaras, A., and Manolis, A. (2020). The growing epidemic of diabetes mellitus. Curr. Vasc. Pharmacol. 18 (2), 104–109. doi:10.2174/1570161117666190405165911
Lund, A., Bagger, J. I., Christensen, M., Knop, F. K., and Vilsbøll, T. (2014). Glucagon and type 2 diabetes: the return of the alpha cell. Curr. Diabetes Rep. 14 (12), 555. doi:10.1007/s11892-014-0555-4
Ma, Q., Li, Y., Li, P., Wang, M., Wang, J., Tang, Z., et al. (2019). Research progress in the relationship between type 2 diabetes mellitus and intestinal flora. Biomed. and Pharmacother. 117, 109138. doi:10.1016/j.biopha.2019.109138
MacDonald, P. E., Wang, X., Xia, F., El-kholy, W., Targonsky, E. D., Tsushima, R. G., et al. (2003). Antagonism of rat beta-cell voltage-dependent K+ currents by exendin 4 requires dual activation of the cAMP/protein kinase A and phosphatidylinositol 3-kinase signaling pathways. J. Biol. Chem. 278 (52), 52446–52453. doi:10.1074/jbc.M307612200
Marathe, C. S., Rayner, C. K., Jones, K. L., and Horowitz, M. (2011). Effects of GLP-1 and incretin-based therapies on gastrointestinal motor function. Exp. Diabetes Res. 2011, 1–10. doi:10.1155/2011/279530
Mariam, Z., and Niazi, S. K. (2024). Glucagon-like peptide agonists: a prospective review. Endocrinol. Diabetes and Metabolism 7 (1), e462. doi:10.1002/edm2.462
Mayo, K. E., Miller, L. J., Bataille, D., Dalle, S., Göke, B., Thorens, B., et al. (2003). International union of Pharmacology. XXXV. The glucagon receptor family. Pharmacol. Rev. 55 (1), 167–194. doi:10.1124/pr.55.1.6
Minari, T. P., Tácito, L. H. B., Yugar, L. B. T., Ferreira-Melo, S. E., Manzano, C. F., Pires, A. C., et al. (2023). Nutritional strategies for the management of type 2 diabetes mellitus: a narrative review. Nutrients 15 (24), 5096. doi:10.3390/nu15245096
Mojsov, S., Weir, G. C., and Habener, J. F. (1987). Insulinotropin: glucagon-like peptide I (7-37) co-encoded in the glucagon gene is a potent stimulator of insulin release in the perfused rat pancreas. J. Clin. Investigation 79 (2), 616–619. doi:10.1172/JCI112855
Moore, B. (1906). On the treatment of Diabetus mellitus by acid extract of Duodenal Mucous Membrane. Biochem. J. 1 (1), 28–38. doi:10.1042/bj0010028
Müller, T. D., Finan, B., Bloom, S. R., D'Alessio, D., Drucker, D. J., Flatt, P. R., et al. (2019). Glucagon-like peptide 1 (GLP-1). Mol. Metab. 30, 72–130. doi:10.1016/j.molmet.2019.09.010
Muttenthaler, M., King, G. F., Adams, D. J., and Alewood, P. F. (2021). Trends in peptide drug discovery. Nat. Rev. Drug Discov. 20 (4), 309–325. doi:10.1038/s41573-020-00135-8
Nauck, M. A., Heimesaat, M. M., Behle, K., Holst, J. J., Nauck, M. S., Ritzel, R., et al. (2002). Effects of glucagon-like peptide 1 on counterregulatory hormone responses, cognitive functions, and insulin secretion during hyperinsulinemic, stepped hypoglycemic clamp experiments in healthy volunteers. J. Clin. Endocrinol. and Metabolism 87 (3), 1239–1246. doi:10.1210/jcem.87.3.8355
Nauck, M. A., Kleine, N., Orskov, C., Holst, J. J., Willms, B., and Creutzfeldt, W. (1993). Normalization of fasting hyperglycaemia by exogenous glucagon-like peptide 1 (7-36 amide) in type 2 (non-insulin-dependent) diabetic patients. Diabetologia 36 (8), 741–744. doi:10.1007/BF00401145
Nauck, M. A., Quast, D. R., Wefers, J., and Meier, J. J. (2021a). GLP-1 receptor agonists in the treatment of type 2 diabetes – state-of-the-art. Mol. Metab. 46, 101102. doi:10.1016/j.molmet.2020.101102
Nauck, M. A., Wefers, J., and Meier, J. J. (2021b). Treatment of type 2 diabetes: challenges, hopes, and anticipated successes. Lancet Diabetes and Endocrinol. 9 (8), 525–544. doi:10.1016/s2213-8587(21)00113-3
Ng, E., Shaw, J. E., Wood, A., Maple-Brown, L. J., and Hare, M. J. (2022). Glucagon-like peptide-1 receptor agonist (GLP1-RA) therapy in type 2 diabetes. Aust. J. General Pract. 51 (7), 513–518. doi:10.31128/AJGP-07-21-6057
Nogueiras, R., Nauck, M. A., and Tschöp, M. H. (2023). Gut hormone co-agonists for the treatment of obesity: from bench to bedside. Nat. Metab. 5 (6), 933–944. doi:10.1038/s42255-023-00812-z
Ofori, J. K., Karagiannopoulos, A., Barghouth, M., Nagao, M., Andersson, M. E., Salunkhe, V. A., et al. (2022). The highly expressed calcium-insensitive synaptotagmin-11 and synaptotagmin-13 modulate insulin secretion. Acta Physiol. 236 (1), e13857. doi:10.1111/apha.13857
Parker, H. E., Adriaenssens, A., Rogers, G., Richards, P., Koepsell, H., Reimann, F., et al. (2012a). Predominant role of active versus facilitative glucose transport for glucagon-like peptide-1 secretion. Diabetologia 55 (9), 2445–2455. doi:10.1007/s00125-012-2585-2
Parker, H. E., Wallis, K., le Roux, C. W., Wong, K. Y., Reimann, F., and Gribble, F. M. (2012b). Molecular mechanisms underlying bile acid-stimulated glucagon-like peptide-1 secretion. Br. J. Pharmacol. 165 (2), 414–423. doi:10.1111/j.1476-5381.2011.01561.x
Peterli, R., Steinert, R. E., Woelnerhanssen, B., Peters, T., Christoffel-Courtin, C., Gass, M., et al. (2012). Metabolic and hormonal changes after laparoscopic roux-en-Y gastric bypass and sleeve gastrectomy: a randomized, prospective trial. Obes. Surg. 22 (5), 740–748. doi:10.1007/s11695-012-0622-3
Petersen, N., Reimann, F., Bartfeld, S., Farin, H. F., Ringnalda, F. C., Vries, R. G. J., et al. (2014). Generation of L Cells in mouse and human small intestine organoids. Diabetes 63 (2), 410–420. doi:10.2337/db13-0991
Qin, J., and Song, L. (2022). Glucagon-like peptide-1 (GLP-1) receptor agonists and cardiovascular events in patients with type 2 diabetes mellitus: a meta-analysis of double-blind, randomized, placebo-controlled clinical trials. BMC Endocr. Disord. 22 (1), 125. doi:10.1186/s12902-022-01036-0
Richards, P., Pais, R., Habib, A. M., Brighton, C. A., Yeo, G. S. H., Reimann, F., et al. (2016). High fat diet impairs the function of glucagon-like peptide-1 producing L-cells. Peptides 77, 21–27. doi:10.1016/j.peptides.2015.06.006
Richards, P., Parker, H. E., Adriaenssens, A. E., Hodgson, J. M., Cork, S. C., Trapp, S., et al. (2014). Identification and characterization of GLP-1 receptor–expressing cells using a new transgenic mouse model. Diabetes 63 (4), 1224–1233. doi:10.2337/db13-1440
Sakar, Y., Duca, F. A., Langelier, B., Devime, F., Blottiere, H., Delorme, C., et al. (2014). Impact of high-fat feeding on basic helix–loop–helix transcription factors controlling enteroendocrine cell differentiation. Int. J. Obes. 38 (11), 1440–1448. doi:10.1038/ijo.2014.20
Schirra, J., Houck, P., Wank, U., Arnold, R., Göke, B., and Katschinski, M. (2000). Effects of glucagon-like peptide-1(7-36)amide on antro-pyloro-duodenal motility in the interdigestive state and with duodenal lipid perfusion in humans. Gut 46 (5), 622–631. doi:10.1136/gut.46.5.622
Schirra, J., Nicolaus, M., Roggel, R., Katschinski, M., Storr, M., Woerle, H. J., et al. (2006). Endogenous glucagon-like peptide 1 controls endocrine pancreatic secretion and antro-pyloro-duodenal motility in humans. Gut 55 (2), 243–251. doi:10.1136/gut.2004.059741
Schirra, J., Wank, U., Arnold, R., Göke, B., and Katschinski, M. (2002). Effects of glucagon-like peptide-1(7–36)amide on motility and sensation of the proximal stomach in humans. Gut 50 (3), 341–348. doi:10.1136/gut.50.3.341
Secher, A., Jelsing, J., Baquero, A. F., Hecksher-Sørensen, J., Cowley, M. A., Dalbøge, L. S., et al. (2014). The arcuate nucleus mediates GLP-1 receptor agonist liraglutide-dependent weight loss. J. Clin. Investigation 124 (10), 4473–4488. doi:10.1172/jci75276
Sharma, D., Verma, S., Vaidya, S., Kalia, K., and Tiwari, V. (2018). Recent updates on GLP-1 agonists: current advancements and challenges. Biomed. and Pharmacother. 108, 952–962. doi:10.1016/j.biopha.2018.08.088
Shetty, R., Basheer, F. T., Poojari, P. G., Thunga, G., Chandran, V. P., and Acharya, L. D. (2022). Adverse drug reactions of GLP-1 agonists: a systematic review of case reports. Diabetes and Metabolic Syndrome Clin. Res. and Rev. 16 (3), 102427. doi:10.1016/j.dsx.2022.102427
Si, H., Zhao, L., Cai, S., Wang, K., Wang, J., Gao, F., et al. (2023). Medication guidelines for glucagon-like peptide-1 receptor agonist (2023 edition). China Pharm. 34 (11), 1281–1292. doi:10.6039/j.issn.1001-0408.2023.11.01
Sjöholm, A. (1996). Diabetes mellitus and impaired pancreatic beta-cell proliferation. J. Intern. Med. 239 (3), 211–220. doi:10.1046/j.1365-2796.1996.377740000.x
Smith, E. P., An, Z., Wagner, C., Lewis, A. G., Cohen, E. B., Li, B., et al. (2014). The role of β cell glucagon-like peptide-1 signaling in glucose regulation and response to diabetes drugs. Cell Metab. 19 (6), 1050–1057. doi:10.1016/j.cmet.2014.04.005
Stoffers, D. A., Kieffer, T. J., Hussain, M. A., Drucker, D. J., Bonner-Weir, S., Habener, J. F., et al. (2000). Insulinotropic glucagon-like peptide 1 agonists stimulate expression of homeodomain protein IDX-1 and increase islet size in mouse pancreas. Diabetes 49 (5), 741–748. doi:10.2337/diabetes.49.5.741
Sun, H., Zhang, A., Gong, Y., Sun, W., Yan, B., Lei, S., et al. (2022). Improving effect of cordycepin on insulin synthesis and secretion in normal and oxidative-damaged INS-1 cells. Eur. J. Pharmacol. 920, 174843. doi:10.1016/j.ejphar.2022.174843
Susini, S., Roche, E., Prentki, M., and Schlegel, W. (1998). Glucose and glucoincretin peptides synergize to induce c-fos, c-jun,junB,zif-268, and nur-77gene expression in pancreatic β(INS-1) cells. FASEB J. 12 (12), 1173–1182. doi:10.1096/fasebj.12.12.1173
Swenne, I. (1983). Effects of aging on the regenerative capacity of the pancreatic B-cell of the rat. Diabetes 32 (1), 14–19. doi:10.2337/diab.32.1.14
Taylor, S. I., Yazdi, Z. S., and Beitelshees, A. L. (2021). Pharmacological treatment of hyperglycemia in type 2 diabetes. J. Clin. Investigation 131 (2), e142243. doi:10.1172/jci142243
Tomita, T. (2016). Apoptosis in pancreatic β-islet cells in Type 2 diabetes. Bosnian J. Basic Med. Sci. 16 (3), 162–179. doi:10.17305/bjbms.2016.919
Tornehave, D., Kristensen, P., Rømer, J., Knudsen, L. B., and Heller, R. S. (2008). Expression of the GLP-1 receptor in mouse, rat, and human pancreas. J. Histochem. and Cytochem. 56 (9), 841–851. doi:10.1369/jhc.2008.951319
Ussher, J. R., and Drucker, D. J. (2023). Glucagon-like peptide 1 receptor agonists: cardiovascular benefits and mechanisms of action. Nat. Rev. Cardiol. 20 (7), 463–474. doi:10.1038/s41569-023-00849-3
Vella, A., Shah, P., Basu, R., Basu, A., Holst, J. J., and Rizza, R. A. (2000). Effect of glucagon-like peptide 1(7-36) amide on glucose effectiveness and insulin action in people with type 2 diabetes. Diabetes 49(4), 611–617. doi:10.2337/diabetes.49.4.611
Wajchenberg, B. L. (2007). beta-cell failure in diabetes and preservation by clinical treatment. Endocr. Rev. 28 (2), 187–218. doi:10.1210/10.1210/er.2006-0038
Wang, X., Zhou, J., Doyle, M. E., and Egan, J. M. (2001). Glucagon-like peptide-1 causes pancreatic duodenal homeobox-1 protein translocation from the cytoplasm to the nucleus of pancreatic beta-cells by a cyclic adenosine monophosphate/protein kinase A-dependent mechanism. Endocrinology 142 (5), 1820–1827. doi:10.1210/endo.142.5.8128
Weir, G. C., Mojsov, S., Hendrick, G. K., and Habener, J. F. (1989). Glucagonlike peptide I (7-37) actions on endocrine pancreas. Diabetes 38 (3), 338–342. doi:10.2337/diab.38.3.338
Winkler, G., Hajós, P., and Kiss, J. T. (2019). A glükagonszerű peptid-1 (GLP1) és a gyomor-bél rendszer. GLP1-receptor-agonisták - túlértékelt gyomor-elfelejtődött bél- („ileal brake) hatás? Orvosi Hetil. 160 (49), 1927–1934. doi:10.1556/650.2019.31615
Wolffenbuttel, B. H. R., Brugts, M. P., Catarig, A.-M., Clark, A., Kok, M., Lieverse, A. G., et al. (2023). Once-weekly semaglutide use in type 2 diabetes: real-world data from the SURE Netherlands observational study. Adv. Ther. 40 (3), 920–933. doi:10.1007/s12325-022-02385-x
Xie, Z., Hu, J., Gu, H., Li, M., and Chen, J. (2023). Comparison of the efficacy and safety of 10 glucagon-like peptide-1 receptor agonists as add-on to metformin in patients with type 2 diabetes: a systematic review. Front. Endocrinol. 14, 1244432. doi:10.3389/fendo.2023.1244432
Xu, G., Stoffers, D. A., Habener, J. F., and Bonner-Weir, S. (1999). Exendin-4 stimulates both beta-cell replication and neogenesis, resulting in increased beta-cell mass and improved glucose tolerance in diabetic rats. Diabetes 48 (12), 2270–2276. doi:10.2337/diabetes.48.12.2270
Yang, B. T., Dayeh, T. A., Volkov, P. A., Kirkpatrick, C. L., Malmgren, S., Jing, X., et al. (2012). Increased DNA methylation and decreased expression of PDX-1 in pancreatic islets from patients with type 2 diabetes. Mol. Endocrinol. 26 (7), 1203–1212. doi:10.1210/me.2012-1004
Yao, H., Zhang, A., Li, D., Wu, Y., Wang, C.-Z., Wan, J.-Y., et al. (2024). Comparative effectiveness of GLP-1 receptor agonists on glycaemic control, body weight, and lipid profile for type 2 diabetes: systematic review and network meta-analysis. Bmj 384, e076410. doi:10.1136/bmj-2023-076410
You, S., Zheng, J., Chen, Y., and Huang, H. (2022). Research progress on the mechanism of beta-cell apoptosis in type 2 diabetes mellitus. Front. Endocrinol. 13, 976465. doi:10.3389/fendo.2022.976465
Zeng, Y., Wang, Y., and Zhang, H. (2024). Consensus of medical experts on clinical application of glucagon-like peptide-1 receptor agonist (GLP-1RA). Pharm. Today 34 (10), 721–735. doi:10.12048/j.issn.1674-229X.2024.10.001
Zhang, C., Li, J., Wang, L., Ma, J., Li, X., Wu, Y., et al. (2024). Terazosin, a repurposed GPR119 agonist, ameliorates mitophagy and β-cell function in NAFPD by inhibiting MST1-Foxo3a signalling pathway. Cell Prolif., e13764. doi:10.1111/cpr.13764
Zhang, L., Wang, Y., Wang, J., Liu, Y., and Yin, Y. (2015). Protein kinase C pathway mediates the protective effects of glucagon-like peptide-1 on the apoptosis of islet β-cells. Mol. Med. Rep. 12 (5), 7589–7594. doi:10.3892/mmr.2015.4355
Zhang, Q., Ye, J., and Wang, X. (2023). Progress in the contrary effects of glucagon-like peptide-1 and chemerin on obesity development. Exp. Biol. Med. 248 (22), 2020–2029. doi:10.1177/15353702231214270
Zhao, X., Wang, M., Wen, Z., Lu, Z., Cui, L., Fu, C., et al. (2021). GLP-1 receptor agonists: beyond their pancreatic effects. Front. Endocrinol. 12, 721135. doi:10.3389/fendo.2021.721135
Zheng, W., Li, L., and Li, H. (2022). Phytochemicals modulate pancreatic islet β cell function through glucagon-like peptide-1-related mechanisms. Biochem. Pharmacol. 197, 114817. doi:10.1016/j.bcp.2021.114817
Zhu, H., Cai, H., Wang, X., Chen, T., Zhen, C., Zhang, Z., et al. (2022). Sodium-glucose co-transporter 1 (SGLT1) differentially regulates gluconeogenesis and GLP-1 receptor (GLP-1R) expression in different diabetic rats: a preliminary validation of the hypothesis of “SGLT1 bridge” as an indication for “surgical diabetes”. Ann. Transl. Med. 10 (8), 481. doi:10.21037/atm-22-1769
Keywords: GLP-1, GLP-1RAs, T2DM, glucose, mechanism
Citation: He X, Zhao W, Li P, Zhang Y, Li G, Su H, Lu B and Pang Z (2025) Research progress of GLP-1RAs in the treatment of type 2 diabetes mellitus. Front. Pharmacol. 15:1483792. doi: 10.3389/fphar.2024.1483792
Received: 20 August 2024; Accepted: 23 December 2024;
Published: 20 January 2025.
Edited by:
Ewa Krystyna Szczepanska-Sadowska, Medical University of Warsaw, PolandReviewed by:
Per M. Hellström, Uppsala University, SwedenCopyright © 2025 He, Zhao, Li, Zhang, Li, Su, Lu and Pang. This is an open-access article distributed under the terms of the Creative Commons Attribution License (CC BY). The use, distribution or reproduction in other forums is permitted, provided the original author(s) and the copyright owner(s) are credited and that the original publication in this journal is cited, in accordance with accepted academic practice. No use, distribution or reproduction is permitted which does not comply with these terms.
*Correspondence: ZongRan Pang, enJwYW5nQDE2My5jb20=; BiNan Lu, YmluYW5sdUBtdWMuZWR1LmNu
Disclaimer: All claims expressed in this article are solely those of the authors and do not necessarily represent those of their affiliated organizations, or those of the publisher, the editors and the reviewers. Any product that may be evaluated in this article or claim that may be made by its manufacturer is not guaranteed or endorsed by the publisher.
Research integrity at Frontiers
Learn more about the work of our research integrity team to safeguard the quality of each article we publish.