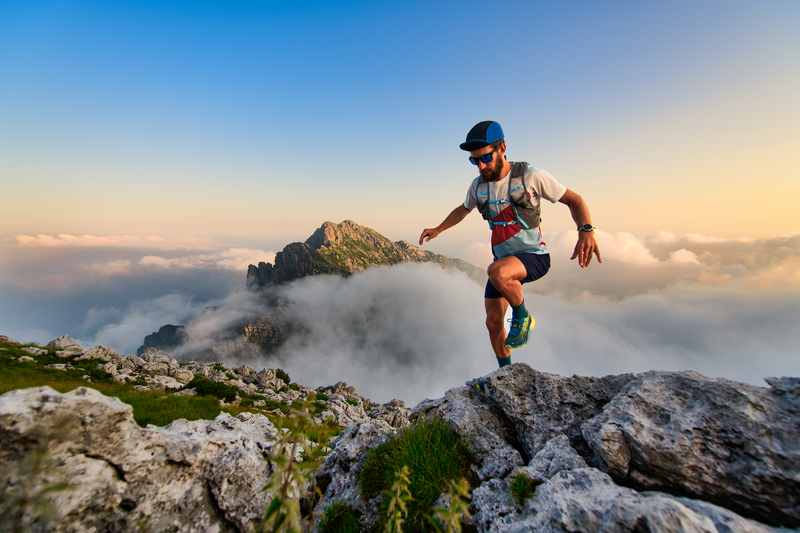
95% of researchers rate our articles as excellent or good
Learn more about the work of our research integrity team to safeguard the quality of each article we publish.
Find out more
REVIEW article
Front. Pharmacol. , 14 November 2024
Sec. Renal Pharmacology
Volume 15 - 2024 | https://doi.org/10.3389/fphar.2024.1477708
LN is a serious complication of systemic lupus erythematosus (SLE), affecting up to 60% of patients with SLE and may lead to end-stage renal disease (ESRD). Macrophages play multifaceted roles in the pathogenesis of LN, including clearance of immune complexes, antigen presentation, regulation of inflammation, and tissue repair. Macrophages are abundant in the glomeruli and tubulointerstitium of LN patients and are positively correlated with serum creatinine levels and the severity of renal pathology. It has been shown that the infiltration of macrophages is closely associated with several clinical indicators, such as serum creatinine and complement C3 levels, anti-dsDNA antibody titers, Austin score, interstitial fibrosis and renal tubular atrophy. Moreover, cytokines expressed by macrophages were upregulated at LN onset and downregulated after remission, suggesting that macrophages may serve as markers of LN pathogenesis and remission. Therapies targeting macrophages have been shown to alleviate LN. There are two main types of macrophages in the kidney: kidney-resident macrophages (KRMs) and monocyte-derived macrophages (MDMs). KRMs and MDMs play different pathological roles in LN, with KRMs promoting leukocyte recruitment at sites of inflammation by expressing monocyte chemokines, while MDMs may exacerbate autoimmune responses by presenting immune complex antigens. Macrophages exhibit high plasticity and can differentiate into various phenotypes in response to distinct environmental stimuli. M1 (proinflammatory) macrophages are linked to the progression of active SLE, whereas the M2 (anti-inflammatory) phenotype is observed during the remission phase of LN. The polarization of macrophages in LN can be manipulated through multiple pathways, such as the modulation of signaling cascades including TLR 2/1, S1P, ERS, metabolic reprogramming, and HMGB1. This paper provides a comprehensive overview of the role of macrophages in the progression of lupus nephritis (LN), and elucidates how these cells and their secretory products function as indicators and therapeutic targets for the disease in the context of diagnosis and treatment of LN.
Systemic lupus erythematosus (SLE) is an autoimmune disease that affects various organs, causing a series of complications. Lupus nephritis (LN) is one of the most serious complications of SLE, occurring in up to 60% of SLE patients (Chan et al., 2023). The prognosis of LN is generally poor, with approximately 7.9% of patients progressing to end-stage renal disease (ESRD) 16.5 years after the onset of LN (Chan et al., 2023), making LN one of the leading causes of death in SLE patients (Wang et al., 2015; Tselios et al., 2019). LN is initiated by the defective clearance of apoptotic cells, as well as the loss of tolerance to autoantigens (Liu and Davidson, 2012; Mistry and Kaplan, 2017). These events lead to abnormal activation of the immune system, which triggers a series of inflammatory responses resulting in renal damage. Macrophages undertake a multifaceted role in the etiology of LN, engaging not merely in the elimination of immune complexes and antigen presentation, but also modulating inflammation and tissue repair via diverse biological pathways. On the one hand, activated macrophages and other antigen-presenting cells phagocytose cellular fragments and present antigens to T and B cells, resulting in the production of antibodies. In combination with autoantibodies and autoantigens, immune complexes are deposited in the kidneys and activate Fc receptors and complement systems, leading to kidney damage (Flores-Mendoza et al., 2018). On the other hand, macrophages, as pivotal players in the innate immune system, possess surface Toll-like receptors (TLRs) that, upon recognizing self-nucleic acids and other autoantigens, possess the potential to exacerbate autoimmune responses (Lind et al., 2022). Macrophages also play a pivotal role in activating and regulating the complement system, thereby significantly influencing the progression of LN (Richoz et al., 2022; Arazi et al., 2019). Macrophages possess the capability to modulate the functionality of neutrophils, encompassing the generation of NETs (neutrophil extracellular traps) and the regulation of LDGs (leukocyte-derived granules), subsequently influencing the inflammatory cascade within lymph nodes (Manda-Handzlik et al., 2023). Furthermore, once activated, macrophages are capable of secreting a diverse series of cytokines, including IL-6, TNF-α, and IL-1β, which subsequently stimulate inflammatory activities within kidneys. As the inflammatory response reaches an advanced stage, macrophages undergo polarization into a reparative phenotype, actively participating in the restoration and repair process of kidney tissue (Nakatani et al., 2010; Kim et al., 2020a). Thus, it is apparent that macrophages are involved in the pathology of LN in many ways.
In this article, we summarize the interactions between macrophages and intrinsic renal cells in LN, the feasibility of the use of macrophages as biomarkers for LN, and the current status of targeting macrophages for the treatment of LN, which may facilitate a better understanding of the roles of macrophages in LN and perhaps provide a reference for further understanding the pathogenesis of LN and the development of related interventions.
Macrophages are members of the innate immune system that are crucial for inflammatory responses and host defense. They are mainly responsible for recognizing, phagocytosing, and degrading invading pathogens and cellular fragments and then presenting the processed antigenic components to other immune cells to drive the immune response (Bajgar and Krejčová, 2023; Muntjewerff et al., 2020). Furthermore, during the early stage of inflammation, macrophages also amplify the inflammatory response by releasing a series of cytokines and chemokines that facilitate the recruitment of their effector cells. In addition to promoting inflammation, macrophages play important roles in tissue repair and fibrosis (Bell and Conway, 2022).
Macrophages are abundant in the glomeruli and tubular interstitium of LN patients (Hu and Chen, 2022; Sahu et al., 2014; Sung et al., 2017). The number of infiltrating macrophages is positively correlated with the level of serum creatinine and the severity of renal pathology (Chen J. et al., 2022). Olmes et al. (2016) analyzed the infiltration of macrophages in the kidneys of patients with type II-V lupus nephritis (classified according to the ISN/RPS system) and reported that the number of macrophages was closely related to several clinical indicators, such as the levels of serum creatinine and complement C3, the anti-dsDNA antibody titer, the Austin score, interstitial fibrosis and tubular atrophy. Moreover, Schiffer et al. (2008) reported that cytokines expressed by activated macrophages are upregulated at proteinuria onset and downregulated after remission induction in NZB/NZW F1 mice, suggesting that activated macrophages may serve as markers of disease onset and remission in LN. In addition, treatments targeting macrophages have been shown to alleviate LN (Chalmers et al., 2015; Chalmers et al., 2017; Zhang et al., 2019). Thus, we can deduce that macrophages play an important role in the process of LN.
There are two types of macrophages in the kidney: kidney-resident macrophages (KRMs) and monocyte-derived macrophages (MDMs). We can distinguish between them by the surface markers, as the former highly expresses F4/80 while the latter highly expresses CD11b (Cheung et al., 2022). KRMs are derived from several different sources, including the yolk sac, fetal liver, and bone marrow (Schulz et al., 2012; Ginhoux and Guilliams, 2016). The first wave of kidney macrophages is derived from erythroid myeloid progenitors (EMPs) in the yolk sac in mice. These EMPs differentiate into premacrophages without monocytic intermediates (Munro and Hughes, 2017). Research in a fate-mapping mouse model revealed that the proportion of yolk sac-derived macrophages in the kidney rapidly decreases beginning at embryonic day 13.5 and is only 2%–3% at birth (Hoeffel et al., 2015). However, fetal monocytes gradually populate the mouse kidney beginning at embryonic day 13.5 (Hoeffel et al., 2015). During this stage, EMPs originating from the yolk sac enter the fetal liver and proliferate rapidly, where they differentiate into monocyte intermediates or premacrophages, which leave the liver to reside in tissues and become KRMs (Hoeffel et al., 2015; Epelman et al., 2014). Notably, fetal monocytes but not yolk sac-derived macrophages give rise to the vast majority of KRMs in the adult kidney (Liu et al., 2020). In addition, bone marrow-derived peripheral monocytes differentiate into macrophages to supplement the KRM pool within a certain limit throughout adulthood (Liu et al., 2020; Dick et al., 2022). Supported by a large amount of evidence, we are familiar with the development of KRMs in mice, yet little is known about this topic in humans. Recently, Bian et al. (2020) analyzed hematopoietic cells from human embryos via single-cell sequencing and reported that early embryonic macrophages in humans exhibit a developmental process similar to that of macrophages in mice.
Comparatively, the source of MDMs is well known. Hematopoietic stem cells (HSCs) in the bone marrow undergo several stages of differentiation to form monoblasts and promonocytes, which enter the circulation and differentiate into mature monocytes. During inflammation, mature monocytes migrate to renal tissue in response to the release of certain chemokines and eventually differentiate into macrophages—a process regulated by macrophage colony-stimulating factor (M-CSF, also known as CSF-1) and granulocyte‒macrophage colony stimulating factor (GM-CSF) (Lenzo et al., 2012; Sun et al., 2018).
Previous studies have employed single-cell RNA sequencing technology to investigate kidney samples derived from LN patients, thereby elucidating the intricate composition of immune cells within the kidney. These investigations have emphasized the distinct functional roles played by various subsets of macrophages in lupus nephritis. Notably, multiple macrophage subsets have been identified, including inflammatory, phagocytic, and M2-like CD16+ macrophages, which exhibit significant differences in their gene expression patterns and functional capabilities. Myeloid cells, particularly macrophages, hold a pivotal role in the development of lupus nephritis (LN). KRMs and MDMs play distinct pathological roles in lupus nephritis (LN). Analysis of the cell fate trajectories of KRMs and MDMs in the MRL/lpr mouse model revealed a disease-associated transcriptional state, underscoring their unique functions in the pathological process. Notably, the MDMs exhibits a pronounced induction of FcγR response genes, potentially exacerbating the autoimmune response by presenting immune complex (IC) opsonin antigens. Conversely, the LN-associated KRMs demonstrates limited immune complex uptake, yet coordinates leukocyte recruitment to inflammatory sites through the expression of monocyte chemokines, thereby driving the progression of LN. Furthermore, KRMs produces niche factors essential for B cell tissue, implying a role in lymphoid aggregates that support autoantibody production, further contributing to the development of autoimmune responses in LN (Richoz et al., 2022; Arazi et al., 2019).
Another type of cell has to be mentioned: patrolling monocytes, also known as non-classical monocytes, play an important role in promoting inflammatory activities in early LN. In the basal state, patrolling monocytes slowly crawl across the vascular endothelium using CX3CR1 and β2 integrins to act as an immune monitor of endothelial cells and surrounding tissues (Auffray et al., 2007). Once stimulated, they exude and invade the surrounding tissues rapidly, producing TNF-α and initiating the classical macrophage differentiation process (Auffray et al., 2007). Patrolling monocytes have recently been found to be present in large numbers in the glomeruli of LN patients and mice, moreover, genetic suppression of patrolling monocytes ameliorated glomerulonephritis (Kuriakose et al., 2019), suggesting a pathogenic role for patrolling monocytes in LN. The immobilized immune complex recruits patrolling monocytes directly by binding to CD16, inducing production of the neutrophil chemotactic factors CXCL2 and TNF-α (Olaru et al., 2018). Subsequently, patrolling monocytes participate in the development of renal inflammation through a series of processes including recruitment of neutrophils, upregulation of IL-6 expression, and differentiation into macrophages (Kuriakose et al., 2019; Finsterbusch et al., 2016; Nomura et al., 2022).
Macrophages are known to be highly plastic and can polarize into different phenotypes in response to various environmental stimuli. Two macrophage phenotypes were initially reported: M1 (proinflammatory or classically activated) macrophages and M2 (anti-inflammatory or alternatively activated) macrophages (Viola et al., 2019). M1 macrophages, which are generally induced by lipopolysaccharide (LPS), TNF-α and INF-γ, participate in the clearance of pathogens by increasing the level of reactive oxygen species (ROS) via nicotinamide adenine dinucleotide phosphate (NADPH) oxidase upregulation and mitochondrial damage (Kim et al., 2017; West et al., 2011). Furthermore, M1 macrophages can release high levels of proinflammatory cytokines, such as TNF-α, IL-1β, and IL-6, and therefore drive inflammatory activities (Shapouri-Moghaddam et al., 2018). In addition, the enhanced ability of antigen presentation to T cells enables M1 macrophages to activate the adaptive immune system (Arora et al., 2018). In contrast, M2 macrophages are generally anti-inflammatory and play a role in tissue repair (Wynn and Vannella, 2016; Oishi and Manabe, 2018). Fibrosis occurs through the overactivation of M2 macrophages, which function in a paracrine manner or differentiate into myofibroblast-like cells via a process called the macrophage-to-myofibroblast transition (MMT) (Fu et al., 2022; Meng et al., 2016; Luo et al., 2023). On the basis of their phenotype and function, M2 macrophages can be further categorized into four types: M2a, M2b, M2c, and M2d (Arora et al., 2018). Among them, M2a macrophages, which are induced by IL-4 and IL-13, express high levels of CD206 and are involved in tissue repair and fibrosis by producing a series of cytokines, including TGF-α and fibronectin (Toki et al., 2014; Wang et al., 2019). M2b macrophages, termed regulatory macrophages, are induced by immune complexes in combination with toll-like receptor (TLR) agonists or IL-1R agonists, and they increase the expression of the anti-inflammatory cytokine IL-10 after activation (Anderson and Mosser, 2002). M2c macrophages, which are activated by glucocorticoids, TGF-β, or IL-10, effectively inhibit inflammation and resolve tissue repair by releasing substantial amounts of IL-10 (Zizzo et al., 2012; Sapudom et al., 2021; Tang et al., 2017); however, they might contribute to fibrosis via TGF-β production (Ikezumi et al., 2015). In addition, M2c macrophages efficiently clear apoptotic cells by expressing Mer tyrosine kinase (MerTK) (Zizzo et al., 2012). M2d macrophages are induced by TLR agonists synergized with adenosine A2A receptor agonists, and they promote angiogenesis by upregulating the expression of vascular endothelial growth factor (VEGF) (Pinhal-Enfield et al., 2003). Notably, the phenotypes of macrophages are not determined by their origin but rather by the microenvironment in which they are located (Lavin et al., 2014).
Lupus flares are associated with a imbalanced ratio of M1 to M2 macrophages (Funes et al., 2018). M1 macrophages are closely associated with active SLE (Labonte et al., 2018), and polarization of M2 macrophages was observed in situations where LN is alleviated (Zhang et al., 2019). Single-cell sequencing analysis of immune cells in the kidneys of patients with LN revealed a transitional trajectory of inflammatory M1 monocytes to M2 macrophages, in which genes encoding proinflammatory cytokines were progressively downregulated, which may suggest that macrophages shift from a proinflammatory phenotype to an alternatively activated phenotype during the process of LN (Arazi et al., 2019). M1 macrophages are associated with the release of some proinflammatory mediators, including IL-1β, IL-6, TNF-α, PGE2, and ROS (Jing et al., 2020). The proinflammatory mediators expressed by macrophages are involved in tissue injury as well as the recruitment of effector cells that cause further damage to the kidney. Moreover, accumulating evidence shows that M2 macrophages also play an important role in the pathogenesis of LN. During the late stages of inflammation, M2 macrophages contribute to inflammation resolution and tissue repair. The deterioration of mice with SLE after the injection of clodronate, which selectively depletes macrophages, was ameliorated by the adoptive transplantation of M2 macrophages, suggesting that M2 macrophages play a protective role in SLE (Li F. et al., 2015).
Macrophages are polarized via several different pathways in LN. As a type of extracellular vesicle, microparticles (MPs) were recently found to be a source of circulating autoantigens in SLE (Nielsen et al., 2012). Burbano et al. (2019) revealed that microparticle-associated immune complexes (MP-ICs) induce macrophage polarization toward the M1 type, which promotes the activation of T cells and B cells in SLE. In addition, sphingosine-1–phosphate (S1P) is a bioactive lipid whose levels are increased in patients with LN (Patyna et al., 2019). Tian et al. (2023) reported that the binding of S1P to its receptor S1PR1 expressed by macrophages (Müller et al., 2017) induces the polarization of M1 macrophages through the activation of the NLRP3 inflammasome in LN. Moreover, endoplasmic reticulum stress (ERS) is involved in the polarization of macrophages. Kim et al. (2015) revealed that ERS increases the expression of proinflammatory cytokines (such as TNF-α and IL-1 β) in macrophages, suggesting a tendency toward the M1 phenotype. Furthermore, metabolic reprogramming, which is dependent on mTOR and hypoxia-inducible factor (HIF)-1α, was found to contribute to the polarization of macrophages in LN. The IgG immune complex binds to the FcγR expressed by macrophages, resulting in increased glycolysis, which induces macrophage polarization to the M1 phenotype (Jing et al., 2020). Finally, high-mobility group protein B1 (HMGB1) was found to contribute to the polarization of M1 macrophages via the TLR2/4 and NF-κB signaling pathways in patients with SLE (Schaper et al., 2016; Wang et al., 2020). In general, LN can be ameliorated by promoting macrophage polarization toward the M2 phenotype. For example, the TLR2/1 agonist PAM3CSK4 (PAM3) was found to induce the polarization of M2 macrophages, which further reduces the levels of autoantibodies and proteinuria and prolongs survival of lupus model mice (Horuluoglu et al., 2019). Thus, the progression of LN can be manipulated by regulating macrophage polarization (Figure 1).
Figure 1. Roles of macrophages in lupus nephritis (Created with bioRender.com).
As we know, macrophages are essential for immunological tolerance, and the impairment of immunological tolerance is closely related to the generation of autoantibodies to nuclear antigens. In this regard, macrophages in the splenic marginal zone (MZ) are of quite importance. MZ is defined as the transition zone between the red pulp and white pulp of the spleen, with two types of resident macrophages inside- marginal zone macrophages (MZMs) and marginal metallophilic macrophages (MMMs). In detail, MZMs highly express the C-type lectin SIGN-related 1 (SIGNR1) and the scavenger receptor MARCO, while MMMs highly express CD169 (Fujiyama et al., 2019). These macrophages induce immune tolerance by phagocytosis of apoptotic cells (McGaha et al., 2011; Ravishankar et al., 2014).
The tolerogenic function of macrophages in MZ was first described by Miyake et al. (2007), who established human diphtheria toxin receptor (DTR) transgenic mice and revealed that macrophages in MZ are specifically ablated upon injection with diphtheria toxin. They reported that the injection of apoptotic cells failed to induce tolerance in DTR mice and that the clearance of injected apoptotic cells was delayed. Further analysis revealed that macrophages in MZ modulate the selective phagocytosis of apoptotic cells by CD8α+ dendritic cells, which are responsible for immunological tolerance to autoantigens (Miyake et al., 2007). Shinde et al. (2018) revealed that DNA from apoptotic cells activates Aryl hydrocarbon receptor (AhR) in macrophages through TLR9, resulting in the production of IL-10, which is crucial for the inhibition of T-cell responses to autoantigens derived from apoptotic cells. Moreover, the chemokine CCL22 is involved in the mechanisms by which macrophages mediate immunological tolerance. CCL22 induced by apoptotic cells in the MMMs stimulates the accumulation and activation of regulatory T cells and dendritic cells, thereby resulting in the induction of the suppression of apoptotic cell antigens (Ravishankar et al., 2014). Peroxisome proliferator-activated receptor γ (PPARγ) and retinoid X receptor α (RXRα) may also be involved in the clearance of apoptotic cells by macrophages. Roszer et al. (2011) found that mice lacking macrophage expression of PPARγ or RXRα exhibit impaired clearance of apoptotic cells and high levels of autoantibodies to nuclear antigens, which are closely associated with the pathogenesis of LN.
Several studies have demonstrated a reduction in MZMs and the defective clearance of apoptotic debris by MZMs in mice that develop a lupus-like autoimmune disease. For example, the number of MZMs in BXD2 mice is significantly lower than that in C57BL/6 mice, and injected apoptotic cells are cleared more slowly in BXD2 mice than C57BL/6 mice (Li et al., 2013). RT‒PCR analysis revealed that the gene expression of tolerogenic cytokines (such as TGF-β and IL-10) by MZMs was reduced in BXD2 mice (Li et al., 2013), which may be partly responsible for the defective function of MZMs. Erythropoietin (EPO) receptors expressed by macrophages has recently been found to be correlated with the phagocytosis of apoptotic cells. Mechanically, sphingosine 1-phosphate (S1P) released by dying cells activates macrophage EPO signaling, resulting in increased expression of PPARγ, which is essential for the clearance of apoptotic cells (Luo et al., 2016). However, decreases in the levels of EPO and the EPO receptor have been observed in a pristane-induced murine lupus model (Luo et al., 2016).
The impairment of glomerular endothelial cells (ECs) is related to the development of proteinuria in LN (Nawata et al., 2018). EC proliferation, widening of the subendothelial space, and abundant infiltration of CD68+ (a panmacrophage marker) macrophages in the capillary lumina and subendothelial areas were observed in LN patients with endocapillary hypercellularity (EH) (Arai et al., 2021), suggesting that macrophages are involved in EC injury. In addition to secreting TNF-αthat increases the expression of heparinase by glomerular ECs, macrophages also release cathepsin L. Both heparinase and cathepsin L cause damage to the EC glycocalyx (Boels et al., 2017). The degradation of the glycocalyx further contributes to inflammatory cell extravasation and vascular endothelial injury (Yung and Chan, 2023).
Macrophages cause damage to ECs, and ECs play a role in macrophage infiltration. Zoshima et al. (2022) induced EH in mice via antibodies derived from MRL/lpr mice and analyzed the expression of chemokines and chemokine receptors. They reported that CD68+ macrophages, which highly express CCR2 and CX3CR1, are among the major inflammatory cells involved in EH. Moreover, ECs were found to express high levels of CCL2 and CX3CL1, suggesting that ECs are involved in the recruitment of macrophages in LN.
MCs exhibit excessive cell proliferation and increased extracellular matrix secretion in LN, leading to glomerular fibrosis and sclerosis (Tsai et al., 2023). In addition, MCs are important sources of inflammatory mediators involved in the progression of LN. During the early stages of LN, anti-dsDNA antibodies bind to MCs via Annexin II (Yung et al., 2010), resulting in increased release of cytokines, chemokines and fibrotic factors (Nowling, 2022).
Proinflammatory cytokines (e.g., IL-1 and IL-6) expressed by classically activated macrophages induce MC proliferation and extracellular matrix accumulation (Ikezumi et al., 2003). Moreover, MCs play a dual role in the polarization of macrophages in LN. Liao et al. (2018) proposed that MCs activated by IFN-γ induce macrophage polarization toward the M1 phenotype in the acute nephritic phase, whereas during the chronic phase of nephritis, MCs enhance M2 polarization in the presence of growth factors to resolve inflammation and promote tissue repair.
Macrophages and MCs interact with each other through the cytokines they secrete. Sung and Fu (2020) analyzed cytokine signaling in the kidneys of lupus-prone NZM2328 mice via confocal microscopy and revealed that MCs not only are major contributors to IL-6 production in the glomerulus but also release the chemokine M-CSF, which induces the recruitment of macrophages in the glomerulus. In the initial stage of LN, deposited immune complexes promote mesangial cell activation through Fc receptors and complement. IL-6, produced by activated mesangial cells, cooperates with other cytokines to increase levels of chemokine and adhesion molecules, which lead to intraglomerular recruitment of macrophages. Subsequently, TNF-α released by macrophages induces IL-6 production in MCs, and all of these cytokines play crucial roles in events such as extracellular matrix and cellular proliferation in renal tissue (Sung and Fu, 2020).
Podocytes are highly differentiated epithelial cells. They extend many tiny processes that overlap each other around the outside of the glomerular basement membrane, forming part of the glomerular filtration barrier. The space between these processes, known as the split diaphragm, is composed mainly of nephrin, podocin, and synaptopodin, which play important roles in maintaining the structure and function of podocytes. Podocyte injury is strongly associated with the development of proteinuria in LN (Wang et al., 2014).
Podocyte injury was detected after coculture with macrophages, as evidenced by decreased synaptopodin expression and increased apoptosis (Zhang et al., 2019). Furthermore, TNF-α and IL-1β released by macrophages reduce nephrin expression in podocytes by inhibiting the promoter of nephrin (Takano et al., 2007). Moreover, TGF-β1 secreted by M2 macrophages may upregulate the expression of Snail1, matrix metalloproteinase (MMP)-7, and MMP-9 via Wnt/β-catenin signaling and downregulate the expression of nephrin, leading to podocyte mesenchymal transition, which leads to podocyte dysfunction (Wang et al., 2011; Li et al., 2008). Recently, Zuo et al. (2021) proposed that MMP-10 can cause damage to podocytes by degrading ZO-1, a podocyte tight junction protein, suggesting that classically activated macrophages may promote podocyte injury via the release of MMP-10 (Roch et al., 2014).
Interactions between parietal epithelial cells and macrophages are rarely discussed. There is evidence that osteopontin expressed by parietal epithelial cells contributes to macrophage infiltration into the kidney (Hartner et al., 2001).
Previously, researchers have focused mainly on glomerular lesions but not tubulointerstitial lesions in LN. However, alterations in tubulointerstitium are receiving increasing attention recently. Renal tubulointerstitial lesions are an independent predictor of prognosis in LN (Obrișcă et al., 2018; Gomes et al., 2021). Moreover, macrophages are abundant in the renal tubulointerstitium of patients with LN (Li et al., 2017), and their number is positively correlated with tubular interstitial inflammation and chronicity indices (Chen J. et al., 2022). In the initial phases of lupus nephritis, macrophages primarily serve a pivotal function in exacerbating inflammatory responses and presenting antigens. In the subsequent phases of inflammatory processes or injuries, macrophages play a pivotal role in facilitating tissue repair through the secretion of growth factors and extracellular matrix remodeling agents, which subsequently contributes to the development of renal tubulointerstitial fibrosis. In chronic inflammation, macrophages can form lymphoid aggregates. Lymphoid aggregates are local immune response centers at the site of inflammation, and are formed by immune cells, including macrophages and lymphocytes. These structures aid in the recruitment and activation of immune cells, maintaining local immune responses. On the one hand, lymphoid aggregates help to localize the immune response to specific areas, preventing the uncontrolled spread of inflammatory reactions. On the other hand, these aggregates provide a platform that promotes interactions between immune cells, including antigen presentation, T cell activation, and the establishment of immune tolerance. Furthermore, immune cells within lymphoid aggregates can not only modulate the intensity and duration of inflammatory responses, but also participating in tissue repair and regeneration processes. The formation of macrophages and lymphoid aggregates has a significant impact on the progression of diseases and the severity of kidney damage (Richoz et al., 2022; Jamaly et al., 2021; Lech and Anders, 2013; Khanal et al., 2023). In addition, macrophage depletion by the administration of diphtheria toxin has been shown to ameliorate tubular injury and interstitial fibrosis in mice with crescentic glomerulonephritis, suggesting that macrophages play an important role in the generation of renal tubulointerstitial lesions (Duffield et al., 2005).
The effects of macrophages on tubular injury are closely related to NF-κB activation. Research has shown that activated NLRP3 in renal tissue-resident macrophages upregulates the expression of IL-33, which promotes inflammatory activities in renal tubular epithelial cells (TECs) via the IL33/ST2/NF-κB pathway. Inhibition of these processes reduces tubular injury and thereby alleviates LN (Ma et al., 2023). Moreover, activation of the NF-κB signaling pathway in macrophages is associated with the release of proinflammatory cytokines (such as IL-6, IL-1 and TNF-α) (Mussbacher et al., 2023). Immunoreactive CD11c+ macrophages derived from circulating monocytes have been identified in the urine of patients with LN. Kim et al. (2020a) reported that CD11c+ macrophages expressing the chemokine receptor CXCR3 are recruited to the renal tubulointerstitium via the chemokine IP-10 produced by TECs. Activated CD11c+ macrophages release high levels of IL-6, which increases fibronectin expression and promotes TEC detachment and apoptosis (Kim et al., 2020a). Among them, fibronectin can be deposited in the tubulointerstitium, inducing the development of interstitial fibrosis (Eddy, 2000), whereas the apoptosis of renal tubular epithelial cells is closely related to tubular atrophy (Havasi and Borkan, 2011). In addition, macrophage exosomes enriched with miR-155 were identified by Zhang Z. et al. (2022). These exosomes can promote inflammatory activities in tubular epithelial cells by downregulating the expression of SOCS-1, a negative regulator of NF-κB, and the inhibition of miR-155 ameliorates renal tubular injury in vivo and in vitro (Zhang Z. et al., 2022).
Analysis of animal models revealed that M2 macrophages are crucial for the fibrotic phase of kidney disease (Han et al., 2013; Kushiyama et al., 2011). Macrophages produce a series of cytokines, such as IL-1, matrix metalloproteinases, and platelet-derived growth factor, which promote the proliferation and activation of fibroblasts (Tang et al., 2019). Myofibroblasts are activated fibroblasts that produce pathogenic collagen, contributing to the development of tubulointerstitial fibrosis (Klingberg et al., 2013). Interestingly, M2 macrophages can also differentiate into myofibroblasts via a process known as the macrophage–myofibroblast transition (MMT), which is regulated by TGF-β/Smad3 signaling, resulting in the production of collagen (Wang et al., 2016).
Renal tubular epithelial cells are not only targets but also drivers of inflammatory activities in LN. They promote macrophage proliferation and survival through the expression of CSF-1 and IL-34 (Wada et al., 2019) and macrophage recruitment through chemokines such as CCL2 and CX3CL1 (Jia et al., 2022a; Jia et al., 2022b; Kassianos et al., 2015). In addition, exosomes produced by TECs were recently shown to be associated with the activation of inflammatory macrophages (Lv et al., 2020; Lv et al., 2018; Li et al., 2019). Once internalized by macrophages, these exosomes, which can be enriched either with microRNAs or CCL2, promote the polarization and migration of M1 macrophages.
The traditional indicators used to test for LN include serum creatinine, urinary protein, anti-dsDNA antibodies and complement C3/4. However, these indicators cannot directly reflect LN flares or distinguish between active and chronic disease. Renal biopsy is the gold standard for the diagnosis of LN, and repeat renal biopsy is recommended for monitoring disease status and guiding the treatment of LN patients (Pakozdi et al., 2018; Marinaki et al., 2020; Lledó-Ibáñez et al., 2022). Nevertheless, renal biopsy is an invasive procedure that cannot be performed anytime or anywhere, which limits its clinical application. Therefore, safe, noninvasive and simple methods are needed for the diagnosis and monitoring of LN.
Intraglomerular CD68+ macrophages can be used as biomarkers for EH to reduce variation due to observer subjectivity. Bos et al. (2022) reported that the number of CD68+ macrophages in the glomerulus was significantly correlated with EH in LN. They reported that when 7 or more CD68+ macrophages were present in a glomerulus, the diagnostic sensitivity and specificity of intraglomerular CD68+ macrophages for EH were 88% and 67%, respectively.
Urinary CD11c+ macrophages derived from circulating monocytes are abundant in the urine of patients with active proliferative LN and are significantly associated with the serum anti-dsDNA antibody titer, renal tubular atrophy and interstitial fibrosis (Kim et al., 2020b). More importantly, the number of urinary CD11c+ macrophages is significantly lower in patients who achieved a complete or partial renal response than in those who did not achieve a renal response after 6 months of treatment (Kim et al., 2020b). These results suggest that the number of urinary CD11c+ macrophages may serve as a biomarker reflecting the clinical and pathological characteristics, as well as the treatment response, of patients with LN.
Soluble CD163 is the most discussed macrophage product. It is derived from the cleavage of the CD163 macrophage receptor (Møller et al., 2010) and can be detected in the urine of LN patients (Endo et al., 2016). Compared with those in SLE patients without LN, patients with other glomerular diseases, and healthy controls, the levels of urinary soluble CD163 in patients with active LN were shown to be significantly greater (Mejia-Vilet et al., 2020; Zhang et al., 2020), suggesting that urinary soluble CD163 may serve as a biomarker of LN. Urinary CD163 begins to rise 6 months before the onset of LN and is not only closely related to the Systemic Lupus Erythematosus Disease Activity Index (SLEDAI) and renal histopathological changes but also reflects the effectiveness of treatment (Mejia-Vilet et al., 2020; Zhang et al., 2020; Gupta et al., 2021), making it an excellent and non-invasive marker during LN follow-up. In contrast, the percentage of CD163+ macrophages in the renal tubulointerstitial compartment is positively correlated with the chronicity index in LN (Allam et al., 2020).
Current treatments for LN tend to be glucocorticoids and immunosuppressants (Table 1), which not only exhibit variable efficacy but also lead to potentially serious adverse events. Given the role of macrophages in the pathogenesis of LN and the promising results of macrophage-associated targeted therapies in a variety of animal models, treatments targeting macrophages, including the promotion of phagocytosis by macrophages, the inhibition of monocyte/macrophage recruitment, the modulation of macrophage polarization and the inhibition of macrophage cytokine production, may be potential interventions for LN. However, most of the experiments evaluating these treatments have been performed in animals and need to be confirmed by further clinical trials.
Since EPO signaling plays an important role in the clearance of apoptotic cells by macrophages, interference with EPO signaling may be a promising approach for LN intervention. Using a lupus-prone mouse model, Luo et al. (2016) found that recombinant human EPO or PPARγ agonists reduced autoantibody production and improved renal function. However, the application of EPO agonists may increase the risk of several adverse effects, including hypertension, thromboembolism, and cardiovascular events (Sonia et al., 2023). Hence, an EPO derivative named ARA290 that possesses the anti-inflammatory and cytoprotective properties of EPO but has no effect on the hematopoietic system was developed (Brines et al., 2008). ARA290 has been reported to significantly promote the phagocytosis of apoptotic cells by macrophages in lupus-prone mice, in addition to inhibiting the activation of M1 macrophages and the production of inflammatory cytokines (such as IL-6 and TNF-α), all of which contribute to the alleviation of renal inflammation in LN (Huang et al., 2018).
Moreover, the inhibition of monocyte/macrophage recruitment alleviates renal inflammation by directly reducing the number of inflammatory macrophages. Mizoribine is a purine synthesis inhibitor that blocks macrophage infiltration by decreasing OPN expression, thereby ameliorating fibrosis in animal models (Takahashi et al., 2009; Sato et al., 2001). In patients with LN, mizoribine significantly inhibits infiltration of macrophages in the glomeruli and reduces the chronicity index, with no serious adverse events observed (Tanaka et al., 2010). However, the sample size of that trial was insufficient, and further large-scale studies are needed. As an alcohol withdrawal drug, disulfiram has recently been reported to negatively regulate monocyte/macrophage migration by inhibiting FRONUT, a cytoplasmic protein that promotes chemotaxis by interacting with CCR2 and CCR5 (Terashima et al., 2020; Toda et al., 2009). In addition, disulfiram reduces the production of cytokines and chemokines (for example, TNF-α and CCL2) in macrophages and thereby alleviates renal pathological injury and proteinuria (Toda et al., 2022). In addition, treatment of lupus-prone mice with GW2580, a selective inhibitor of CSF-1 receptor kinase, depletes macrophages in the glomeruli and significantly reduces albuminuria and the levels of serum creatinine, as well as renal tissue damage (Chalmers et al., 2015; Chalmers et al., 2017). Recently, (5R)-5-hydroxytriptolide was shown to reduce the infiltration of immune cells (such as macrophages, T cells, and neutrophils) in MRL/lpr mice by inhibiting the expression of chemokines. Moreover, reductions in serum creatinine levels and proteinuria, reduced renal damage, and increased life spans were also observed in mice treated with (5R)-5-hydroxytriptolide (Zhang et al., 2017). PI3Kδ, which is expressed mainly in hematopoietic cells (Vanhaesebroeck et al., 1997), regulates the differentiation and cytokine production of B and T cells (Okkenhaug and Fruman, 2010). Surprisingly, PI3Kδ inhibitors reduced the number of B cells and T cells in MRL/lpr mice while inhibiting the ability of macrophages to cross the glomerular basement membrane, which is critical for intrarenal macrophage infiltration (Suárez-Fueyo et al., 2014). PI3Kδ inhibitors are not only effective at ameliorating kidney damage and prolonging the life span of mice but also safe (Suárez-Fueyo et al., 2014), making them promising drugs for the treatment of LN. Other drugs that inhibit the infiltration of macrophages and a variety of other immune cells include CCR1 antagonists, which prolong survival in NZB/W mice by alleviating renal pathological injury and delaying fatal proteinuria (Bignon et al., 2014).
Metabolic reprogramming is a key regulator of macrophage polarization. M1 macrophages typically use glycolysis to generate energy, while M2 macrophages use oxidative phosphorylation (Galván-Peña and O’Neill, 2014). Jing et al. (2020) treated primary human renal macrophages and nephrotoxic serum-challenged mice with glycolysis inhibitors and reported that the release of the proinflammatory mediator IL-1β by macrophages was reduced; moreover, the mice presented reduced serum urea levels and IL-1β-mediated inflammatory cell recruitment to the kidney. These findings suggest that manipulating macrophage metabolism may interfere with the progression of LN. In addition, mivebresib, a BRD4 inhibitor, and Serp-1, serpin encoded by myxomavirus, have recently been shown to play roles in SLE-associated diffuse alveolar hemorrhages by inhibiting the polarization of M1 macrophages, showing promise for LN treatment (He et al., 2023; Zhuang et al., 2021). Liver X receptor-alpha achieves a similar effect by promoting the polarization of M2 macrophages (Han et al., 2018). It has also been reported that supplementation with serum amyloid p component (SAP) can alleviate LN in mice by shifting macrophages from a proinflammatory M2b phenotype to an anti-inflammatory M2a phenotype (Zhang et al., 2011). However, supplementation with mannose-binding lectin (MBL) inhibits the MAPK and NF-κB signaling pathways, which in turn reduces M2b macrophage polarization and exerts beneficial effects on the clinical and pathological parameters of LN patients (Cai et al., 2013). Other drugs that inhibit M1 macrophage polarization, including Sedum sarmentosum Bunge extract (Lu et al., 2019), and drugs that promote M2 macrophage polarization, including the TLR2/1 agonist PAM3CSK4 (Horuluoglu et al., 2019), the AhR agonist indole-3-carbinol (Mohammadi et al., 2018), the PPAR γ agonist pioglitazone (Mohammadi et al., 2017), and total peony glucosides (Liang et al., 2021), have been shown to have therapeutic effects on SLE or LN. Mesenchymal stem cells (MSCs), including bone marrow-derived MSCs (BM-MSCs), umbilical cord-derived MCs (UC-MSCs) and adipose tissue-derived MSCs (AT-MSCs), play multiple roles in LN, one of which is the induction of an anti-inflammatory phenotype in macrophages. In vivo and in vitro studies have shown that human UC-MSCs can not only reduce macrophage infiltration but also induce the polarization of anti-inflammatory macrophages to prevent podocyte injury in lupus-prone mice (Zhang et al., 2019). Deng et al. (2015) proposed that the reduced levels of macrophage CD206 and phagocytic activity in patients with SLE can be restored by human UC-MSCs, in which IL-6 plays a key regulatory role. BM-MSCs have also been reported to significantly reduce the number of macrophages in rats with chronic renal failure (Caldas et al., 2015). Interestingly, it has been suggested that MSC-derived exosomes possess immunomodulatory effects similar to those of MSCs. In vitro experiments demonstrated that exosomes derived from AT-MSCs could induce macrophages to differentiate into an immunosuppressive phenotype, which is related to an increase in the number of regulatory T (Treg) cells (Özdemir et al., 2019). Treg cells are protective cells that contribute to the alleviation of SLE (Scheinecker et al., 2020). Moreover, macrophages incubated with MSC-derived exosomes exhibited reduced proliferative capacity, decreased expression of LPS-induced inflammatory cytokines, and an increased percentage of M2 macrophages (Sun et al., 2022). Moreover, MRL/lpr mice injected with MSC-derived exosomes presented reduced deposition of glomerular collagen fibers and complement C3, increased levels of serum IL-10, and prolonged survival, suggesting that MSC-derived exosomes may ameliorate LN by inducing M2 macrophage polarization (Sun et al., 2022). Specifically, the mechanism by which MSC-derived exosomes induce the M2 phenotype in macrophages may be partially attributable to the downregulation of NOTCH1 expression by miR-146a-5p in exosomes (Chen X. et al., 2022). In addition, Zhang M. et al. (2022) showed that exosomes derived from BM-MSCs may promote M2 macrophage polarization via the miR-16 and miR-21 they carry, resulting in a reduction in antibodies and complement deposition in the glomerular mesangial and endocapillary regions. In addition, exosome-treated macrophages increase the expression of CCL20, which is closely related to the expansion of Treg cells (Zhang M. et al., 2022). All of these factors have been shown to contribute to the amelioration of LN.
Glucocorticoids are the most widely used drugs in the treatment of LN. As reviewed by Ehrchen et al. (2019), they act not only by reducing the expression of proinflammatory mediators (such as IL-1β, IL-6, IL-12 and TNF-α) but also by inducing the expression of anti-inflammatory mediators (Fkbp5, MKP-1, Tsc22d3, Per1, adenosine receptor 3a and formyl peptide receptor) in monocytes/macrophages. These effects are essential for ameliorating inflammation in the kidney. Cf-02, a synthetic compound, has recently shown favorable therapeutic effects in NZB/WF1 mice with acute-onset LN that induced by LPS intraperitoneal injections (Yang et al., 2021). Specifically, cf.-02 reduces the infiltration of inflammatory cells, including macrophages, and inhibits the activation of the macrophage NLRP3 inflammasome, which ultimately ameliorates renal function, proteinuria, and renal histopathology (Yang et al., 2021). Additionally, paeoniflorin (Ji et al., 2018), luteolin (Ding et al., 2023), and quercitrin (Li et al., 2016) have been found to be potent anti-inflammatory agents that reduce the production of proinflammatory cytokines by inhibiting NF-κB activation in macrophages, suggesting potential therapies for LN. Isogarcinol, a natural compound extracted from Garcinia mangostana L., has been shown to be an immunomodulatory drug with low cytotoxicity (Cen et al., 2013). Li et al. reported that isogarcinol abrogated the increased secretion of IL-1 β, IL-6 and TNF-α in macrophages induced by LPS, and decreased expression of proinflammatory cytokines was also observed in the kidneys of mice treated with isogarcinol (Li W. et al., 2015), suggesting that isogarcinol may be a candidate anti-inflammatory drug for kidney protection in LN.
Macrophages have various roles in lupus nephritis (LN), including inflammatory responses, tissue repair, immune tolerance, and their interaction with intrinsic cells in the kidney. Macrophages have cellular interactions with glomerular endothelial cells, mesangial cells, podocytes, and renal tubular epithelial cells to affect the progression of LN. The polarization status of macrophages is closely correlated with the pathogenesis of LN, where M1 type macrophages are associated with disease activity, while M2 type macrophages are associated with the resolution of inflammation and tissue repair. Moreover, the potential of macrophages and their products as biomarkers in the diagnosis and monitoring of LN, and therapeutic strategies targeting macrophages provide new perspectives for the treatment of LN. Macrophages with different polarization statuses participate in different stages of LN development. A comprehensive understanding of the mechanisms by which macrophages are involved in the development of LN is crucial for exploring treatments for LN, but the heterogeneity of macrophages poses numerous challenges to our research. Current therapies targeting macrophages have shown promising results in lupus animal models; however, these effects may not be observed in humans. Key issues to be addressed in future research include the identification of optimal macrophage-targeted therapeutic strategies, the evaluation of the safety and efficacy of these therapies in humans, and the development of new biomarkers to improve the diagnosis and treatment of LN.
In conclusion, macrophages play complex roles in LN, which not only participate in the pathogenesis of the disease, but may also serve as new therapeutic targets. By gaining insight into the role of macrophages in LN, we can develop more effective and safer treatments for LN patients.
YC: Writing–original draft, Investigation. LL: Writing–original draft, Visualization, Methodology. YY: Writing–original draft, Conceptualization. YH: Writing–review and editing. WH: Writing–review and editing. HK: Writing–review and editing. Z-YG: Writing–review and editing. GS: Writing–review and editing.
The author(s) declare that financial support was received for the research, authorship, and/or publication of this article. This research was supported by the Basic Public Welfare Research Project of Wenzhou Science and Technology Bureau (Y2023116). Wenzhou association for science and technology service technology innovation (kjfw16).
The authors declare that the research was conducted in the absence of any commercial or financial relationships that could be construed as a potential conflict of interest.
All claims expressed in this article are solely those of the authors and do not necessarily represent those of their affiliated organizations, or those of the publisher, the editors and the reviewers. Any product that may be evaluated in this article, or claim that may be made by its manufacturer, is not guaranteed or endorsed by the publisher.
Allam, M., Fathy, H., Allah, D. A., and Salem, M. A. E. (2020). Lupus nephritis: correlation of immunohistochemical expression of C4d, CD163-positive M2c-like macrophages and Foxp3-expressing regulatory T cells with disease activity and chronicity. Lupus 29 (8), 943–953. doi:10.1177/0961203320932663
Anderson, C. F., and Mosser, D. M. (2002). A novel phenotype for an activated macrophage: the type 2 activated macrophage. J. Leukoc. Biol. 72 (1), 101–106. doi:10.1189/jlb.72.1.101
Arai, M., Mii, A., Kashiwagi, T., Shimizu, A., and Sakai, Y. (2021). The severity of glomerular endothelial cell injury is associated with infiltrating macrophage heterogeneity in endocapillary proliferative glomerulonephritis. Sci. Rep. 11 (1), 13339. doi:10.1038/s41598-021-92655-5
Arazi, A., Rao, D. A., Berthier, C. C., Davidson, A., Liu, Y., Hoover, P. J., et al. (2019). The immune cell landscape in kidneys of patients with lupus nephritis. Nat. Immunol. 20 (7), 902–914. doi:10.1038/s41590-019-0398-x
Arora, S., Dev, K., Agarwal, B., Das, P., and Syed, M. A. (2018). Macrophages: their role, activation and polarization in pulmonary diseases. Immunobiology 223 (4-5), 383–396. doi:10.1016/j.imbio.2017.11.001
Auffray, C., Fogg, D., Garfa, M., Elain, G., Join-Lambert, O., Kayal, S., et al. (2007). Monitoring of blood vessels and tissues by a population of monocytes with patrolling behavior. Science 317 (5838), 666–670. doi:10.1126/science.1142883
Bajgar, A., and Krejčová, G. (2023). On the origin of the functional versatility of macrophages. Front. Physiol. 14, 1128984. doi:10.3389/fphys.2023.1128984
Bell, R. M. B., and Conway, B. R. (2022). Macrophages in the kidney in health, injury and repair. Int. Rev. Cell Mol. Biol. 367, 101–147. doi:10.1016/bs.ircmb.2022.01.005
Bian, Z., Gong, Y., Huang, T., Lee, C. Z. W., Bian, L., Bai, Z., et al. (2020). Deciphering human macrophage development at single-cell resolution. Nature 582 (7813), 571–576. doi:10.1038/s41586-020-2316-7
Bignon, A., Gaudin, F., Hémon, P., Tharinger, H., Mayol, K., Walzer, T., et al. (2014). CCR1 inhibition ameliorates the progression of lupus nephritis in NZB/W mice. J. Immunol. 192 (3), 886–896. doi:10.4049/jimmunol.1300123
Boels, M. G. S., Koudijs, A., Avramut, M. C., Sol, W. M. P. J., Wang, G., van Oeveren-Rietdijk, A. M., et al. (2017). Systemic monocyte chemotactic protein-1 inhibition modifies renal macrophages and restores glomerular endothelial glycocalyx and barrier function in diabetic nephropathy. Am. J. Pathol. 187 (11), 2430–2440. doi:10.1016/j.ajpath.2017.07.020
Bos, E. M. J., Sangle, S. R., Wilhelmus, S., Wolterbeek, R., Jordan, N., D'Cruz, D., et al. (2022). Use of glomerular CD68+ cells as a surrogate marker for endocapillary hypercellularity in lupus nephritis. Kidney Int. Rep. 7 (4), 841–847. doi:10.1016/j.ekir.2021.12.030
Brines, M., Patel, N. S. A., Villa, P., Brines, C., Mennini, T., De Paola, M., et al. (2008). Nonerythropoietic, tissue-protective peptides derived from the tertiary structure of erythropoietin. Proc. Natl. Acad. Sci. U. S. A. 105 (31), 10925–10930. doi:10.1073/pnas.0805594105
Burbano, C., Villar-Vesga, J., Vásquez, G., Muñoz-Vahos, C., Rojas, M., and Castaño, D. (2019). Proinflammatory differentiation of macrophages through microparticles that form immune complexes leads to T- and B-cell activation in systemic autoimmune diseases. Front. Immunol. 10, 2058. doi:10.3389/fimmu.2019.02058
Cai, Y., Zhang, W., and Xiong, S. (2013). Mannose-binding lectin blunts macrophage polarization and ameliorates lupus nephritis. PLoS One 8 (4), e62465. doi:10.1371/journal.pone.0062465
Caldas, H. C., de Paula Couto, T. A. P., Fernandes, I. M. M., Baptista, M. A. S. F., Kawasaki-Oyama, R. S., Goloni-Bertollo, E. M., et al. (2015). Comparative effects of mesenchymal stem cell therapy in distinct stages of chronic renal failure. Clin. Exp. Nephrol. 19 (5), 783–789. doi:10.1007/s10157-015-1079-1
Cen, J., Shi, M., Yang, Y., Fu, Y., Zhou, H., Wang, M., et al. (2013). Isogarcinol is a new immunosuppressant. PLoS One 8 (6), e66503. doi:10.1371/journal.pone.0066503
Chalmers, S. A., Chitu, V., Herlitz, L. C., Sahu, R., Stanley, E. R., and Putterman, C. (2015). Macrophage depletion ameliorates nephritis induced by pathogenic antibodies. J. Autoimmun. 57, 42–52. doi:10.1016/j.jaut.2014.11.007
Chalmers, S. A., Wen, J., Shum, J., Doerner, J., Herlitz, L., and Putterman, C. (2017). CSF-1R inhibition attenuates renal and neuropsychiatric disease in murine lupus. Clin. Immunol. 185, 100–108. doi:10.1016/j.clim.2016.08.019
Chan, S. C. W., Wang, Y. F., Yap, D. Y. H., Chan, T. M., Lau, Y. L., Lee, P. P. W., et al. (2023). Risk and Factors associated with disease manifestations in systemic lupus erythematosus - lupus nephritis (RIFLE-LN): a ten-year risk prediction strategy derived from a cohort of 1652 patients. Front. Immunol. 14, 1200732. doi:10.3389/fimmu.2023.1200732
Chen J., J., Cui, L., Ouyang, J., Wang, J., and Xu, W. (2022). Clinicopathological significance of tubulointerstitial CD68 macrophages in proliferative lupus nephritis. Clin. Rheumatol. 41 (9), 2729–2736. doi:10.1007/s10067-022-06214-y
Chen, X., Su, C., Wei, Q., Sun, H., Xie, J., and Nong, G. (2022). Exosomes derived from human umbilical cord mesenchymal stem cells alleviate diffuse alveolar hemorrhage associated with systemic lupus erythematosus in mice by promoting M2 macrophage polarization via the microRNA-146a-5p/NOTCH1 Axis. Immunol. Invest. 51 (7), 1975–1993. doi:10.1080/08820139.2022.2090261
Cheung, M. D., Erman, E. N., Moore, K. H., Lever, J. M., Li, Z., LaFontaine, J. R., et al. (2022). Resident macrophage subpopulations occupy distinct microenvironments in the kidney. JCI Insight 7 (20), e161078. doi:10.1172/jci.insight.161078
Deng, W., Chen, W., Zhang, Z., Huang, S., Kong, W., Sun, Y., et al. (2015). Mesenchymal stem cells promote CD206 expression and phagocytic activity of macrophages through IL-6 in systemic lupus erythematosus. Clin. Immunol. 161 (2), 209–216. doi:10.1016/j.clim.2015.07.011
Dick, S. A., Wong, A., Hamidzada, H., Nejat, S., Nechanitzky, R., Vohra, S., et al. (2022). Three tissue resident macrophage subsets coexist across organs with conserved origins and life cycles. Sci. Immunol. 7 (67), eabf7777. doi:10.1126/sciimmunol.abf7777
Ding, T., Yi, T., Li, Y., Zhang, W., Wang, X., Liu, J., et al. (2023). Luteolin attenuates lupus nephritis by regulating macrophage oxidative stress via HIF-1α pathway. Eur. J. Pharmacol. 953, 175823. doi:10.1016/j.ejphar.2023.175823
Duffield, J. S., Tipping, P. G., Kipari, T., Cailhier, J. F., Clay, S., Lang, R., et al. (2005). Conditional ablation of macrophages halts progression of crescentic glomerulonephritis. Am. J. Pathol. 167 (5), 1207–1219. doi:10.1016/S0002-9440(10)61209-6
Eddy, A. A. (2000). Molecular basis of renal fibrosis. Pediatr. Nephrol. 15 (3-4), 290–301. doi:10.1007/s004670000461
Ehrchen, J. M., Roth, J., and Barczyk-Kahlert, K. (2019). More than suppression: glucocorticoid action on monocytes and macrophages. Front. Immunol. 10, 2028. doi:10.3389/fimmu.2019.02028
Endo, N., Tsuboi, N., Furuhashi, K., Shi, Y., Du, Q., Abe, T., et al. (2016). Urinary soluble CD163 level reflects glomerular inflammation in human lupus nephritis. Nephrol. Dial. Transpl. 31 (12), 2023–2033. doi:10.1093/ndt/gfw214
Epelman, S., Lavine, K. J., Beaudin, A. E., Sojka, D. K., Carrero, J. A., Calderon, B., et al. (2014). Embryonic and adult-derived resident cardiac macrophages are maintained through distinct mechanisms at steady state and during inflammation. Immunity 40 (1), 91–104. doi:10.1016/j.immuni.2013.11.019
Finsterbusch, M., Hall, P., Li, A., Devi, S., Westhorpe, C. L. V., Kitching, A. R., et al. (2016). Patrolling monocytes promote intravascular neutrophil activation and glomerular injury in the acutely inflamed glomerulus. Proc. Natl. Acad. Sci. U. S. A. 113 (35), E5172–E5181. doi:10.1073/pnas.1606253113
Flores-Mendoza, G., Sansón, S. P., Rodríguez-Castro, S., Crispín, J. C., and Rosetti, F. (2018). Mechanisms of tissue injury in lupus nephritis. Trends Mol. Med. 24 (4), 364–378. doi:10.1016/j.molmed.2018.02.003
Fu, H., Gu, Y. H., Tan, J., Yang, Y. N., and Wang, G. H. (2022). CircACTR2 in macrophages promotes renal fibrosis by activating macrophage inflammation and epithelial-mesenchymal transition of renal tubular epithelial cells. Cell Mol. Life Sci. 79 (5), 253. doi:10.1007/s00018-022-04247-9
Fujiyama, S., Nakahashi-Oda, C., Abe, F., Wang, Y., Sato, K., and Shibuya, A. (2019). Identification and isolation of splenic tissue-resident macrophage sub-populations by flow cytometry. Int. Immunol. 31 (1), 51–56. doi:10.1093/intimm/dxy064
Funes, S. C., Rios, M., Escobar-Vera, J., and Kalergis, A. M. (2018). Implications of macrophage polarization in autoimmunity. Immunology 154 (2), 186–195. doi:10.1111/imm.12910
Galván-Peña, S., and O'Neill, L. A. (2014). Metabolic reprograming in macrophage polarization. Front. Immunol. 5, 420. doi:10.3389/fimmu.2014.00420
Ginhoux, F., and Guilliams, M. (2016). Tissue-resident macrophage ontogeny and homeostasis. Immunity 44 (3), 439–449. doi:10.1016/j.immuni.2016.02.024
Gomes, M. F., Mardones, C., Xipell, M., Blasco, M., Solé, M., Espinosa, G., et al. (2021). The extent of tubulointerstitial inflammation is an independent predictor of renal survival in lupus nephritis. J. Nephrol. 34 (6), 1897–1905. doi:10.1007/s40620-021-01007-z
Gupta, R., Yadav, A., and Aggarwal, A. (2021). Urinary soluble CD163 is a good biomarker for renal disease activity in lupus nephritis. Clin. Rheumatol. 40 (3), 941–948. doi:10.1007/s10067-020-05343-6
Han, S., Zhuang, H., Shumyak, S., Wu, J., Xie, C., Li, H., et al. (2018). Liver X receptor agonist therapy prevents diffuse alveolar hemorrhage in murine lupus by repolarizing macrophages. Front. Immunol. 9, 135. doi:10.3389/fimmu.2018.00135
Han, Y., Tesch, G. H., Manthey, C. L., and Nikolic-Paterson, D. J. (2013). Role of macrophages in the fibrotic phase of rat crescentic glomerulonephritis. Am. J. Physiol. Ren. Physiol. 304 (8), F1043–F1053. doi:10.1152/ajprenal.00389.2012
Hartner, A., Porst, M., Gauer, S., Pröls, F., Veelken, R., and Hilgers, K. F. (2001). Glomerular osteopontin expression and macrophage infiltration in glomerulosclerosis of DOCA-salt rats. Am. J. Kidney Dis. 38 (1), 153–164. doi:10.1053/ajkd.2001.25209
Havasi, A., and Borkan, S. C. (2011). Apoptosis and acute kidney injury. Kidney Int. 80 (1), 29–40. doi:10.1038/ki.2011.120
He, X., Jiang, L., Hu, L., Du, P., Zhu, M., Wu, H., et al. (2023). Mivebresib alleviates systemic lupus erythematosus-associated diffuse alveolar hemorrhage via inhibiting infiltration of monocytes and M1 polarization of macrophages. Int. Immunopharmacol. 120, 110305. doi:10.1016/j.intimp.2023.110305
Hoeffel, G., Chen, J., Lavin, Y., Low, D., Almeida, F. F., See, P., et al. (2015). C-Myb(+) erythro-myeloid progenitor-derived fetal monocytes give rise to adult tissue-resident macrophages. Immunity 42 (4), 665–678. doi:10.1016/j.immuni.2015.03.011
Horuluoglu, B., Bayik, D., Kayraklioglu, N., Goguet, E., Kaplan, M. J., and Klinman, D. M. (2019). PAM3 supports the generation of M2-like macrophages from lupus patient monocytes and improves disease outcome in murine lupus. J. Autoimmun. 99, 24–32. doi:10.1016/j.jaut.2019.01.004
Hu, W., and Chen, X. (2022). Identification of hub ferroptosis-related genes and immune infiltration in lupus nephritis using bioinformatics. Sci. Rep. 12 (1), 18826. doi:10.1038/s41598-022-23730-8
Huang, B., Jiang, J., Luo, B., Zhu, W., Liu, Y., Wang, Z., et al. (2018). Non-erythropoietic erythropoietin-derived peptide protects mice from systemic lupus erythematosus. J. Cell Mol. Med. 22 (7), 3330–3339. doi:10.1111/jcmm.13608
Ikezumi, Y., Hurst, L. A., Masaki, T., Atkins, R. C., and Nikolic-Paterson, D. J. (2003). Adoptive transfer studies demonstrate that macrophages can induce proteinuria and mesangial cell proliferation. Kidney Int. 63 (1), 83–95. doi:10.1046/j.1523-1755.2003.00717.x
Ikezumi, Y., Suzuki, T., Yamada, T., Hasegawa, H., Kaneko, U., Hara, M., et al. (2015). Alternatively activated macrophages in the pathogenesis of chronic kidney allograft injury. Pediatr. Nephrol. 30 (6), 1007–1017. doi:10.1007/s00467-014-3023-0
Jamaly, S., Rakaee, M., Abdi, R., Tsokos, G. C., and Fenton, K. A. (2021). Interplay of immune and kidney resident cells in the formation of tertiary lymphoid structures in lupus nephritis. Autoimmun. Rev. 20 (12), 102980. doi:10.1016/j.autrev.2021.102980
Ji, L., Hou, X., Liu, W., Deng, X., Jiang, Z., Huang, K., et al. (2018). Paeoniflorin inhibits activation of the IRAK1-NF-κB signaling pathway in peritoneal macrophages from lupus-prone MRL/lpr mice. Microb. Pathog. 124, 223–229. doi:10.1016/j.micpath.2018.08.051
Jia, P., Xu, S., Ren, T., Pan, T., Wang, X., Zhang, Y., et al. (2022b). LncRNA IRAR regulates chemokines production in tubular epithelial cells thus promoting kidney ischemia-reperfusion injury. Cell Death Dis. 13 (6), 562. doi:10.1038/s41419-022-05018-x
Jia, P., Xu, S., Wang, X., Wu, X., Ren, T., Zou, Z., et al. (2022a). Chemokine CCL2 from proximal tubular epithelial cells contributes to sepsis-induced acute kidney injury. Am. J. Physiol. Ren. Physiol. 323 (2), F107–f119. doi:10.1152/ajprenal.00037.2022
Jing, C., Castro-Dopico, T., Richoz, N., Tuong, Z. K., Ferdinand, J. R., Lok, L. S. C., et al. (2020). Macrophage metabolic reprogramming presents a therapeutic target in lupus nephritis. Proc. Natl. Acad. Sci. U. S. A. 117 (26), 15160–15171. doi:10.1073/pnas.2000943117
Kassianos, A. J., Wang, X., Sampangi, S., Afrin, S., Wilkinson, R., and Healy, H. (2015). Fractalkine-CX3CR1-dependent recruitment and retention of human CD1c+ myeloid dendritic cells by in vitro-activated proximal tubular epithelial cells. Kidney Int. 87 (6), 1153–1163. doi:10.1038/ki.2014.407
Khanal, S., Wieland, A., and Gunderson, A. J. (2023). Mechanisms of tertiary lymphoid structure formation: cooperation between inflammation and antigenicity. Front. Immunol. 14, 1267654. doi:10.3389/fimmu.2023.1267654
Kim, J., Jeong, J. H., Jung, J., Jeon, H., Lee, S., Lim, J. S., et al. (2020a). Immunological characteristics and possible pathogenic role of urinary CD11c+ macrophages in lupus nephritis. Rheumatol. (Oxford) 59 (8), 2135–2145. doi:10.1093/rheumatology/keaa053
Kim, J., Lee, J. S., Go, H., Lim, J. S., Oh, J. S., Kim, Y. G., et al. (2020b). Clinical and histological significance of urinary CD11c(+) macrophages in lupus nephritis. Arthritis Res. Ther. 22 (1), 173. doi:10.1186/s13075-020-02265-1
Kim, S., Joe, Y., Kim, H. J., Kim, Y. S., Jeong, S. O., Pae, H. O., et al. (2015). Endoplasmic reticulum stress-induced IRE1α activation mediates cross-talk of GSK-3β and XBP-1 to regulate inflammatory cytokine production. J. Immunol. 194 (9), 4498–4506. doi:10.4049/jimmunol.1401399
Kim, S. Y., Jeong, J. M., Kim, S. J., Seo, W., Kim, M. H., Choi, W. M., et al. (2017). Pro-inflammatory hepatic macrophages generate ROS through NADPH oxidase 2 via endocytosis of monomeric TLR4-MD2 complex. Nat. Commun. 8 (1), 2247. doi:10.1038/s41467-017-02325-2
Klingberg, F., Hinz, B., and White, E. S. (2013). The myofibroblast matrix: implications for tissue repair and fibrosis. J. Pathol. 229 (2), 298–309. doi:10.1002/path.4104
Kuriakose, J., Redecke, V., Guy, C., Zhou, J., Wu, R., Ippagunta, S. K., et al. (2019). Patrolling monocytes promote the pathogenesis of early lupus-like glomerulonephritis. J. Clin. Invest. 129 (6), 2251–2265. doi:10.1172/JCI125116
Kushiyama, T., Oda, T., Yamada, M., Higashi, K., Yamamoto, K., Sakurai, Y., et al. (2011). Alteration in the phenotype of macrophages in the repair of renal interstitial fibrosis in mice. Nephrol. (Carlton) 16 (5), 522–535. doi:10.1111/j.1440-1797.2010.01439.x
Labonte, A. C., Kegerreis, B., Geraci, N. S., Bachali, P., Madamanchi, S., Robl, R., et al. (2018). Identification of alterations in macrophage activation associated with disease activity in systemic lupus erythematosus. PLoS One 13 (12), e0208132. doi:10.1371/journal.pone.0208132
Lavin, Y., Winter, D., Blecher-Gonen, R., David, E., Keren-Shaul, H., Merad, M., et al. (2014). Tissue-resident macrophage enhancer landscapes are shaped by the local microenvironment. Cell 159 (6), 1312–1326. doi:10.1016/j.cell.2014.11.018
Lech, M., and Anders, H. J. (2013). The pathogenesis of lupus nephritis. J. Am. Soc. Nephrol. 24 (9), 1357–1366. doi:10.1681/ASN.2013010026
Lenzo, J. C., Turner, A. L., Cook, A. D., Vlahos, R., Anderson, G. P., Reynolds, E. C., et al. (2012). Control of macrophage lineage populations by CSF-1 receptor and GM-CSF in homeostasis and inflammation. Immunol. Cell Biol. 90 (4), 429–440. doi:10.1038/icb.2011.58
Li, F., Yang, Y., Zhu, X., Huang, L., and Xu, J. (2015). Macrophage polarization modulates development of systemic lupus erythematosus. Cell Physiol. Biochem. 37 (4), 1279–1288. doi:10.1159/000430251
Li, H., Wu, Q., Li, J., Yang, P., Zhu, Z., Luo, B., et al. (2013). Cutting Edge: defective follicular exclusion of apoptotic antigens due to marginal zone macrophage defects in autoimmune BXD2 mice. J. Immunol. 190 (9), 4465–4469. doi:10.4049/jimmunol.1300041
Li, J., Yu, Y. F., Liu, C. H., and Wang, C. M. (2017). Significance of M2 macrophages in glomerulonephritis with crescents. Pathol. Res. Pract. 213 (9), 1215–1220. doi:10.1016/j.prp.2017.04.011
Li, W., Li, H., Zhang, M., Wang, M., Zhong, Y., Wu, H., et al. (2016). Quercitrin ameliorates the development of systemic lupus erythematosus-like disease in a chronic graft-versus-host murine model. Am. J. Physiol. Ren. Physiol. 311 (1), F217–F226. doi:10.1152/ajprenal.00249.2015
Li, W., Li, H., Zhang, M., Zhong, Y., Wang, M., Cen, J., et al. (2015). Isogarcinol extracted from Garcinia mangostana L. Ameliorates systemic lupus erythematosus-like disease in a murine model. J. Agric. Food Chem. 63 (38), 8452–8459. doi:10.1021/acs.jafc.5b03425
Li, Y., Kang, Y. S., Dai, C., Kiss, L. P., Wen, X., and Liu, Y. (2008). Epithelial-to-mesenchymal transition is a potential pathway leading to podocyte dysfunction and proteinuria. Am. J. Pathol. 172 (2), 299–308. doi:10.2353/ajpath.2008.070057
Li, Z. L., Lv, L. L., Tang, T. T., Wang, B., Feng, Y., Zhou, L. T., et al. (2019). HIF-1α inducing exosomal microRNA-23a expression mediates the cross-talk between tubular epithelial cells and macrophages in tubulointerstitial inflammation. Kidney Int. 95 (2), 388–404. doi:10.1016/j.kint.2018.09.013
Liang, C. L., Jiang, H., Feng, W., Liu, H., Han, L., Chen, Y., et al. (2021). Total glucosides of paeony ameliorate pristane-induced lupus nephritis by inducing PD-1 ligands(+) macrophages via activating IL-4/STAT6/PD-L2 signaling. Front. Immunol. 12, 683249. doi:10.3389/fimmu.2021.683249
Liao, W. Q., Cui, S. Y., Ouyang, Q., Mei, Y., Cai, G. Y., Fu, B., et al. (2018). Modulation of macrophage polarization by human glomerular mesangial cells in response to the stimuli in renal microenvironment. J. Interferon Cytokine Res. 38 (12), 566–577. doi:10.1089/jir.2018.0093
Lind, N. A., Rael, V. E., Pestal, K., Liu, B., and Barton, G. M. (2022). Regulation of the nucleic acid-sensing Toll-like receptors. Nat. Rev. Immunol. 22 (4), 224–235. doi:10.1038/s41577-021-00577-0
Liu, F., Dai, S., Feng, D., Qin, Z., Peng, X., Sakamuri, S. S. V. P., et al. (2020). Distinct fate, dynamics and niches of renal macrophages of bone marrow or embryonic origins. Nat. Commun. 11 (1), 2280. doi:10.1038/s41467-020-16158-z
Liu, Z., and Davidson, A. (2012). Taming lupus-a new understanding of pathogenesis is leading to clinical advances. Nat. Med. 18 (6), 871–882. doi:10.1038/nm.2752
Lledó-Ibáñez, G. M., Xipell, M., Ferreira, M., Solé, M., Garcia-Herrera, A., Cervera, R., et al. (2022). Kidney biopsy in lupus nephritis after achieving clinical renal remission: paving the way for renal outcome assessment. Clin. Kidney J. 15 (11), 2081–2088. doi:10.1093/ckj/sfac150
Lu, H., Cheng, S., Wu, C., Zheng, S., Hong, W., Liu, L., et al. (2019). Sedum sarmentosum Bunge extract alleviates inflammation and kidney injury via inhibition of M1-macrophage polarization. Phytomedicine 62, 152976. doi:10.1016/j.phymed.2019.152976
Luo, B., Gan, W., Liu, Z., Shen, Z., Wang, J., Shi, R., et al. (2016). Erythropoeitin signaling in macrophages promotes dying cell clearance and immune tolerance. Immunity 44 (2), 287–302. doi:10.1016/j.immuni.2016.01.002
Luo, L., Wang, S., Hu, Y., Wang, L., Jiang, X., Zhang, J., et al. (2023). Precisely regulating M2 subtype macrophages for renal fibrosis resolution. ACS Nano 17 (22), 22508–22526. doi:10.1021/acsnano.3c05998
Lv, L. L., Feng, Y., Wen, Y., Wu, W. J., Ni, H. F., Li, Z. L., et al. (2018). Exosomal CCL2 from tubular epithelial cells is critical for albumin-induced tubulointerstitial inflammation. J. Am. Soc. Nephrol. 29 (3), 919–935. doi:10.1681/ASN.2017050523
Lv, L. L., Feng, Y., Wu, M., Wang, B., Li, Z. L., Zhong, X., et al. (2020). Exosomal miRNA-19b-3p of tubular epithelial cells promotes M1 macrophage activation in kidney injury. Cell Death Differ. 27 (1), 210–226. doi:10.1038/s41418-019-0349-y
Ma, Q., Xu, M., Jing, X., Qiu, J., Huang, S., Yan, H., et al. (2023). Honokiol suppresses the aberrant interactions between renal resident macrophages and tubular epithelial cells in lupus nephritis through the NLRP3/IL-33/ST2 axis. Cell Death Dis. 14 (3), 174. doi:10.1038/s41419-023-05680-9
Manda-Handzlik, A., Cieloch, A., Kuźmicka, W., Mroczek, A., Stelmaszczyk-Emmel, A., Demkow, U., et al. (2023). Secretomes of M1 and M2 macrophages decrease the release of neutrophil extracellular traps. Sci. Rep. 13 (1), 15633. doi:10.1038/s41598-023-42167-1
Marinaki, S., Kapsia, E., Liapis, G., Gakiopoulou, H., Skalioti, C., Kolovou, K., et al. (2020). Clinical impact of repeat renal biopsies in patients with lupus nephritis: renal biopsy is essential especially later in the course of the disease. Eur. J. Rheumatol. 7 (1), 2–8. doi:10.5152/eurjrheum.2019.18146
McGaha, T. L., Chen, Y., Ravishankar, B., van Rooijen, N., and Karlsson, M. C. I. (2011). Marginal zone macrophages suppress innate and adaptive immunity to apoptotic cells in the spleen. Blood 117 (20), 5403–5412. doi:10.1182/blood-2010-11-320028
Mejia-Vilet, J. M., Zhang, X. L., Cruz, C., Cano-Verduzco, M. L., Shapiro, J. P., Nagaraja, H. N., et al. (2020). Urinary soluble CD163: a novel noninvasive biomarker of activity for lupus nephritis. J. Am. Soc. Nephrol. 31 (6), 1335–1347. doi:10.1681/ASN.2019121285
Meng, X. M., Wang, S., Huang, X. R., Yang, C., Xiao, J., Zhang, Y., et al. (2016). Inflammatory macrophages can transdifferentiate into myofibroblasts during renal fibrosis. Cell Death Dis. 7 (12), e2495. doi:10.1038/cddis.2016.402
Mistry, P., and Kaplan, M. J. (2017). Cell death in the pathogenesis of systemic lupus erythematosus and lupus nephritis. Clin. Immunol. 185, 59–73. doi:10.1016/j.clim.2016.08.010
Miyake, Y., Asano, K., Kaise, H., Uemura, M., Nakayama, M., and Tanaka, M. (2007). Critical role of macrophages in the marginal zone in the suppression of immune responses to apoptotic cell-associated antigens. J. Clin. Invest. 117 (8), 2268–2278. doi:10.1172/JCI31990
Mohammadi, S., Memarian, A., Sedighi, S., Behnampour, N., and Yazdani, Y. (2018). Immunoregulatory effects of indole-3-carbinol on monocyte-derived macrophages in systemic lupus erythematosus: a crucial role for aryl hydrocarbon receptor. Autoimmunity 51 (5), 199–209. doi:10.1080/08916934.2018.1494161
Mohammadi, S., Saghaeian-Jazi, M., Sedighi, S., and Memarian, A. (2017). Immunomodulation in systemic lupus erythematosus: induction of M2 population in monocyte-derived macrophages by pioglitazone. Lupus 26 (12), 1318–1327. doi:10.1177/0961203317701842
Møller, H. J., Nielsen, M. J., Maniecki, M. B., Madsen, M., and Moestrup, S. K. (2010). Soluble macrophage-derived CD163: a homogenous ectodomain protein with a dissociable haptoglobin-hemoglobin binding. Immunobiology 215 (5), 406–412. doi:10.1016/j.imbio.2009.05.003
Müller, J., von Bernstorff, W., Heidecke, C. D., and Schulze, T. (2017). Differential S1P receptor profiles on M1-and M2-polarized macrophages affect macrophage cytokine production and migration. Biomed. Res. Int. 2017, 7584621. doi:10.1155/2017/7584621
Munro, D. A. D., and Hughes, J. (2017). The origins and functions of tissue-resident macrophages in kidney development. Front. Physiol. 8, 837. doi:10.3389/fphys.2017.00837
Muntjewerff, E. M., Meesters, L. D., and van den Bogaart, G. (2020). Antigen cross-presentation by macrophages. Front. Immunol. 11, 1276. doi:10.3389/fimmu.2020.01276
Mussbacher, M., Derler, M., Basílio, J., and Schmid, J. A. (2023). NF-κB in monocytes and macrophages - an inflammatory master regulator in multitalented immune cells. Front. Immunol. 14, 1134661. doi:10.3389/fimmu.2023.1134661
Nakatani, K., Yoshimoto, S., Iwano, M., Asai, O., Samejima, K. i., Sakan, H., et al. (2010). Fractalkine expression and CD16+ monocyte accumulation in glomerular lesions: association with their severity and diversity in lupus models. Am. J. Physiol. Ren. Physiol. 299 (1), F207–F216. doi:10.1152/ajprenal.00482.2009
Nawata, A., Hisano, S., Shimajiri, S., Wang, K. Y., Tanaka, Y., and Nakayama, T. (2018). Podocyte and endothelial cell injury lead to nephrotic syndrome in proliferative lupus nephritis. Histopathology 72 (7), 1084–1092. doi:10.1111/his.13454
Nielsen, C. T., Østergaard, O., Stener, L., Iversen, L. V., Truedsson, L., Gullstrand, B., et al. (2012). Increased IgG on cell-derived plasma microparticles in systemic lupus erythematosus is associated with autoantibodies and complement activation. Arthritis Rheum. 64 (4), 1227–1236. doi:10.1002/art.34381
Nomura, A., Mizuno, M., Noto, D., Aoyama, A., Kuga, T., Murayama, G., et al. (2022). Different spatial and temporal roles of monocytes and monocyte-derived cells in the pathogenesis of an imiquimod induced lupus model. Front. Immunol. 13, 764557. doi:10.3389/fimmu.2022.764557
Nowling, T. K. (2022). Mesangial cells in lupus nephritis. Curr. Rheumatol. Rep. 23 (12), 83. doi:10.1007/s11926-021-01048-0
Obrișcă, B., Jurubiță, R., Andronesi, A., Sorohan, B., Achim, C., Bobeica, R., et al. (2018). Histological predictors of renal outcome in lupus nephritis: the importance of tubulointerstitial lesions and scoring of glomerular lesions. Lupus 27 (9), 1455–1463. doi:10.1177/0961203318776109
Oishi, Y., and Manabe, I. (2018). Macrophages in inflammation, repair and regeneration. Int. Immunol. 30 (11), 511–528. doi:10.1093/intimm/dxy054
Okkenhaug, K., and Fruman, D. A. (2010). PI3Ks in lymphocyte signaling and development. Curr. Top. Microbiol. Immunol. 346, 57–85. doi:10.1007/82_2010_45
Olaru, F., Döbel, T., Lonsdorf, A. S., Oehrl, S., Maas, M., Enk, A. H., et al. (2018). Intracapillary immune complexes recruit and activate slan-expressing CD16+ monocytes in human lupus nephritis. JCI Insight 3 (11), e96492. doi:10.1172/jci.insight.96492
Olmes, G., Büttner-Herold, M., Ferrazzi, F., Distel, L., Amann, K., and Daniel, C. (2016). CD163+ M2c-like macrophages predominate in renal biopsies from patients with lupus nephritis. Arthritis Res. Ther. 18, 90. doi:10.1186/s13075-016-0989-y
Özdemir, R., Özdemir, A. T., Sarıboyacı, A. E., Uysal, O., Tuğlu, M. İ., and Kırmaz, C. (2019). The investigation of immunomodulatory effects of adipose tissue mesenchymal stem cell educated macrophages on the CD4 T cells. Immunobiology 224 (4), 585–594. doi:10.1016/j.imbio.2019.04.002
Pakozdi, A., Pyne, D., Sheaff, M., and Rajakariar, R. (2018). Utility of a repeat renal biopsy in lupus nephritis: a single centre experience. Nephrol. Dial. Transpl. 33 (3), 507–513. doi:10.1093/ndt/gfx019
Patyna, S., Büttner, S., Eckes, T., Obermüller, N., Bartel, C., Braner, A., et al. (2019). Blood ceramides as novel markers for renal impairment in systemic lupus erythematosus. Prostagl. Other Lipid Mediat 144, 106348. doi:10.1016/j.prostaglandins.2019.106348
Pinhal-Enfield, G., Ramanathan, M., Hasko, G., Vogel, S. N., Salzman, A. L., Boons, G. J., et al. (2003). An angiogenic switch in macrophages involving synergy between Toll-like receptors 2, 4, 7, and 9 and adenosine A(2A) receptors. Am. J. Pathol. 163 (2), 711–721. doi:10.1016/S0002-9440(10)63698-X
Ravishankar, B., Shinde, R., Liu, H., Chaudhary, K., Bradley, J., Lemos, H. P., et al. (2014). Marginal zone CD169+ macrophages coordinate apoptotic cell-driven cellular recruitment and tolerance. Proc. Natl. Acad. Sci. U. S. A. 111 (11), 4215–4220. doi:10.1073/pnas.1320924111
Richoz, N., Tuong, Z. K., Loudon, K. W., Patiño-Martínez, E., Ferdinand, J. R., Portet, A., et al. (2022). Distinct pathogenic roles for resident and monocyte-derived macrophages in lupus nephritis. JCI Insight 7 (21), e159751. doi:10.1172/jci.insight.159751
Roch, T., Akymenko, O., Krüger, A., Jung, F., and Lendlein, A. (2014). Expression pattern analysis and activity determination of matrix metalloproteinase derived from human macrophage subsets. Clin. Hemorheol. Microcirc. 58 (1), 147–158. doi:10.3233/CH-141885
Roszer, T., Menéndez-Gutiérrez, M. P., Lefterova, M. I., Alameda, D., Núñez, V., Lazar, M. A., et al. (2011). Autoimmune kidney disease and impaired engulfment of apoptotic cells in mice with macrophage peroxisome proliferator-activated receptor gamma or retinoid X receptor alpha deficiency. J. Immunol. 186 (1), 621–631. doi:10.4049/jimmunol.1002230
Sahu, R., Bethunaickan, R., Singh, S., and Davidson, A. (2014). Structure and function of renal macrophages and dendritic cells from lupus-prone mice. Arthritis Rheumatol. 66 (6), 1596–1607. doi:10.1002/art.38410
Sapudom, J., Karaman, S., Mohamed, W. K. E., Garcia-Sabaté, A., Quartey, B. C., and Teo, J. C. M. (2021). 3D in vitro M2 macrophage model to mimic modulation of tissue repair. NPJ Regen. Med. 6 (1), 83. doi:10.1038/s41536-021-00193-5
Sato, N., Shiraiwa, K., Kai, K., Watanabe, A., Ogawa, S., Kobayashi, Y., et al. (2001). Mizoribine ameliorates the tubulointerstitial fibrosis of obstructive nephropathy. Nephron 89 (2), 177–185. doi:10.1159/000046065
Schaper, F., de Leeuw, K., Horst, G., Bootsma, H., Limburg, P. C., Heeringa, P., et al. (2016). High mobility group box 1 skews macrophage polarization and negatively influences phagocytosis of apoptotic cells. Rheumatol. (Oxford) 55 (12), 2260–2270. doi:10.1093/rheumatology/kew324
Scheinecker, C., Göschl, L., and Bonelli, M. (2020). Treg cells in health and autoimmune diseases: new insights from single cell analysis. J. Autoimmun. 110, 102376. doi:10.1016/j.jaut.2019.102376
Schiffer, L., Bethunaickan, R., Ramanujam, M., Huang, W., Schiffer, M., Tao, H., et al. (2008). Activated renal macrophages are markers of disease onset and disease remission in lupus nephritis. J. Immunol. 180 (3), 1938–1947. doi:10.4049/jimmunol.180.3.1938
Schulz, C., Gomez Perdiguero, E., Chorro, L., Szabo-Rogers, H., Cagnard, N., Kierdorf, K., et al. (2012). A lineage of myeloid cells independent of Myb and hematopoietic stem cells. Science 336 (6077), 86–90. doi:10.1126/science.1219179
Shapouri-Moghaddam, A., Mohammadian, S., Vazini, H., Taghadosi, M., Esmaeili, S. A., Mardani, F., et al. (2018). Macrophage plasticity, polarization, and function in health and disease. J. Cell Physiol. 233 (9), 6425–6440. doi:10.1002/jcp.26429
Shinde, R., Hezaveh, K., Halaby, M. J., Kloetgen, A., Chakravarthy, A., da Silva Medina, T., et al. (2018). Apoptotic cell-induced AhR activity is required for immunological tolerance and suppression of systemic lupus erythematosus in mice and humans. Nat. Immunol. 19 (6), 571–582. doi:10.1038/s41590-018-0107-1
Sonia, S. N., George, S., Shahi, S. R., Ali, Z., Abaza, A., Jamil, A., et al. (2023). An overview of safety and efficacy between hypoxia-inducible factor-prolyl-hydroxylase inhibitors and erythropoietin-stimulating agents in treating anemia in chronic kidney disease patients. Cureus 15 (7), e42045. doi:10.7759/cureus.42045
Suárez-Fueyo, A., Rojas, J. M., Cariaga, A. E., García, E., Steiner, B. H., Barber, D. F., et al. (2014). Inhibition of PI3Kδ reduces kidney infiltration by macrophages and ameliorates systemic lupus in the mouse. J. Immunol. 193 (2), 544–554. doi:10.4049/jimmunol.1400350
Sun, L., Rautela, J., Delconte, R. B., Souza-Fonseca-Guimaraes, F., Carrington, E. M., Schenk, R. L., et al. (2018). GM-CSF quantity has a selective effect on granulocytic vs. Monocytic myeloid development and function. Front. Immunol. 9, 1922. doi:10.3389/fimmu.2018.01922
Sun, W., Yan, S., Yang, C., Yang, J., Wang, H., Li, C., et al. (2022). Mesenchymal stem cells-derived exosomes ameliorate lupus by inducing M2 macrophage polarization and regulatory T cell expansion in MRL/lpr mice. Immunol. Invest. 51 (6), 1785–1803. doi:10.1080/08820139.2022.2055478
Sung, S. J., and Fu, S. M. (2020). Interactions among glomerulus infiltrating macrophages and intrinsic cells via cytokines in chronic lupus glomerulonephritis. J. Autoimmun. 106, 102331. doi:10.1016/j.jaut.2019.102331
Sung, S. J., Ge, Y., Dai, C., Wang, H., Fu, S. M., Sharma, R., et al. (2017). Dependence of glomerulonephritis induction on novel intraglomerular alternatively activated bone marrow-derived macrophages and mac-1 and PD-L1 in lupus-prone NZM2328 mice. J. Immunol. 198 (7), 2589–2601. doi:10.4049/jimmunol.1601565
Takahashi, S., Taniguchi, Y., Nakashima, A., Arakawa, T., Kawai, T., Doi, S., et al. (2009). Mizoribine suppresses the progression of experimental peritoneal fibrosis in a rat model. Nephron Exp. Nephrol. 112 (2), e59–e69. doi:10.1159/000213896
Takano, Y., Yamauchi, K., Hayakawa, K., Hiramatsu, N., Kasai, A., Okamura, M., et al. (2007). Transcriptional suppression of nephrin in podocytes by macrophages: roles of inflammatory cytokines and involvement of the PI3K/Akt pathway. FEBS Lett. 581 (3), 421–426. doi:10.1016/j.febslet.2006.12.051
Tanaka, H., Oki, E., Tsuruga, K., Aizawa-Yashiro, T., Ito, Y., Sato, N., et al. (2010). Mizoribine attenuates renal injury and macrophage infiltration in patients with severe lupus nephritis. Clin. Rheumatol. 29 (9), 1049–1054. doi:10.1007/s10067-010-1484-5
Tang, L., Zhang, H., Wang, C., Li, H., Zhang, Q., and Bai, J. (2017). M2A and M2C macrophage subsets ameliorate inflammation and fibroproliferation in acute lung injury through interleukin 10 pathway. Shock 48 (1), 119–129. doi:10.1097/SHK.0000000000000820
Tang, P. M., Nikolic-Paterson, D. J., and Lan, H. Y. (2019). Macrophages: versatile players in renal inflammation and fibrosis. Nat. Rev. Nephrol. 15 (3), 144–158. doi:10.1038/s41581-019-0110-2
Terashima, Y., Toda, E., Itakura, M., Otsuji, M., Yoshinaga, S., Okumura, K., et al. (2020). Targeting FROUNT with disulfiram suppresses macrophage accumulation and its tumor-promoting properties. Nat. Commun. 11 (1), 609. doi:10.1038/s41467-020-14338-5
Tian, J., Chang, S., Wang, J., Chen, J., Xu, H., Huang, T., et al. (2023). S1P/S1PR1 axis promotes macrophage M1 polarization through NLRP3 inflammasome activation in Lupus nephritis. Mol. Immunol. 160, 55–66. doi:10.1016/j.molimm.2023.06.006
Toda, E., Sawada, A., Takeuchi, K., Wakamatsu, K., Ishikawa, A., Kuwahara, N., et al. (2022). Inhibition of the chemokine signal regulator FROUNT by disulfiram ameliorates crescentic glomerulonephritis. Kidney Int. 102 (6), 1276–1290. doi:10.1016/j.kint.2022.07.031
Toda, E., Terashima, Y., Sato, T., Hirose, K., Kanegasaki, S., and Matsushima, K. (2009). FROUNT is a common regulator of CCR2 and CCR5 signaling to control directional migration. J. Immunol. 183 (10), 6387–6394. doi:10.4049/jimmunol.0803469
Toki, D., Zhang, W., Hor, K. L. M., Liuwantara, D., Alexander, S. I., Yi, Z., et al. (2014). The role of macrophages in the development of human renal allograft fibrosis in the first year after transplantation. Am. J. Transpl. 14 (9), 2126–2136. doi:10.1111/ajt.12803
Tsai, C. Y., Li, K. J., Shen, C. Y., Lu, C. H., Lee, H. T., Wu, T. H., et al. (2023). Decipher the immunopathological mechanisms and set up potential therapeutic strategies for patients with lupus nephritis. Int. J. Mol. Sci. 24 (12), 10066. doi:10.3390/ijms241210066
Tselios, K., Gladman, D. D., Sheane, B. J., Su, J., and Urowitz, M. (2019). All-cause, cause-specific and age-specific standardised mortality ratios of patients with systemic lupus erythematosus in Ontario, Canada over 43 years (1971-2013). Ann. Rheum. Dis. 78 (6), 802–806. doi:10.1136/annrheumdis-2018-214802
Vanhaesebroeck, B., Welham, M. J., Kotani, K., Stein, R., Warne, P. H., Zvelebil, M. J., et al. (1997). P110delta, a novel phosphoinositide 3-kinase in leukocytes. Proc. Natl. Acad. Sci. U. S. A. 94 (9), 4330–4335. doi:10.1073/pnas.94.9.4330
Viola, A., Munari, F., Sánchez-Rodríguez, R., Scolaro, T., and Castegna, A. (2019). The metabolic signature of macrophage responses. Front. Immunol. 10, 1462. doi:10.3389/fimmu.2019.01462
Wada, Y., Gonzalez-Sanchez, H. M., Weinmann-Menke, J., Iwata, Y., Ajay, A. K., Meineck, M., et al. (2019). IL-34-Dependent intrarenal and systemic mechanisms promote lupus nephritis in MRL-fas(lpr) mice. J. Am. Soc. Nephrol. 30 (2), 244–259. doi:10.1681/ASN.2018090901
Wang, D., Dai, C., Li, Y., and Liu, Y. (2011). Canonical Wnt/β-catenin signaling mediates transforming growth factor-β1-driven podocyte injury and proteinuria. Kidney Int. 80 (11), 1159–1169. doi:10.1038/ki.2011.255
Wang, J., Li, R., Peng, Z., Hu, B., Rao, X., and Li, J. (2020). HMGB1 participates in LPS-induced acute lung injury by activating the AIM2 inflammasome in macrophages and inducing polarization of M1 macrophages via TLR2, TLR4, and RAGE/NF-κB signaling pathways. Int. J. Mol. Med. 45 (1), 61–80. doi:10.3892/ijmm.2019.4402
Wang, L. X., Zhang, S. X., Wu, H. J., Rong, X. L., and Guo, J. (2019). M2b macrophage polarization and its roles in diseases. J. Leukoc. Biol. 106 (2), 345–358. doi:10.1002/JLB.3RU1018-378RR
Wang, S., Meng, X. M., Ng, Y. Y., Ma, F. Y., Zhou, S., Zhang, Y., et al. (2016). TGF-β/Smad3 signalling regulates the transition of bone marrow-derived macrophages into myofibroblasts during tissue fibrosis. Oncotarget 7 (8), 8809–8822. doi:10.18632/oncotarget.6604
Wang, Y., Yu, F., Song, D., Wang, S. X., and Zhao, M. H. (2014). Podocyte involvement in lupus nephritis based on the 2003 ISN/RPS system: a large cohort study from a single centre. Rheumatol. (Oxford) 53 (7), 1235–1244. doi:10.1093/rheumatology/ket491
Wang, Z., Wang, Y., Zhu, R., Tian, X., Xu, D., Wang, Q., et al. (2015). Long-term survival and death causes of systemic lupus erythematosus in China: a systemic review of observational studies. Med. (Baltimore) 94 (17), e794. doi:10.1097/MD.0000000000000794
West, A. P., Brodsky, I. E., Rahner, C., Woo, D. K., Erdjument-Bromage, H., Tempst, P., et al. (2011). TLR signalling augments macrophage bactericidal activity through mitochondrial ROS. Nature 472 (7344), 476–480. doi:10.1038/nature09973
Wynn, T. A., and Vannella, K. M. (2016). Macrophages in tissue repair, regeneration, and fibrosis. Immunity 44 (3), 450–462. doi:10.1016/j.immuni.2016.02.015
Yang, S. R., Hua, K. F., Yang, C. Y., Chen, A., Weng, J. C., Tsai, Y. L., et al. (2021). Cf-02, a novel benzamide-linked small molecule, blunts NF-κB activation and NLRP3 inflammasome assembly and improves acute onset of accelerated and severe lupus nephritis in mice. Faseb J. 35 (8), e21785. doi:10.1096/fj.202100047R
Yung, S., and Chan, T. M. (2023). Endothelial cell activation and glycocalyx shedding - potential as biomarkers in patients with lupus nephritis. Front. Immunol. 14, 1251876. doi:10.3389/fimmu.2023.1251876
Yung, S., Cheung, K. F., Zhang, Q., and Chan, T. M. (2010). Anti-dsDNA antibodies bind to mesangial annexin II in lupus nephritis. J. Am. Soc. Nephrol. 21 (11), 1912–1927. doi:10.1681/ASN.2009080805
Zhang, L. Y., Li, H., Wu, Y. W., Cheng, L., Yan, Y. X., Yang, X. Q., et al. (2017). (5R)-5-hydroxytriptolide ameliorates lupus nephritis in MRL/lpr mice by preventing infiltration of immune cells. Am. J. Physiol. Ren. Physiol. 312 (4), F769–F777. doi:10.1152/ajprenal.00649.2016
Zhang, M., Johnson-Stephenson, T. K., Wang, W., Wang, Y., Li, J., Li, L., et al. (2022). Mesenchymal stem cell-derived exosome-educated macrophages alleviate systemic lupus erythematosus by promoting efferocytosis and recruitment of IL-17(+) regulatory T cell. Stem Cell Res. Ther. 13 (1), 484. doi:10.1186/s13287-022-03174-7
Zhang, T., Li, H., Vanarsa, K., Gidley, G., Mok, C. C., Petri, M., et al. (2020). Association of urine sCD163 with proliferative lupus nephritis, fibrinoid necrosis, cellular crescents and intrarenal M2 macrophages. Front. Immunol. 11, 671. doi:10.3389/fimmu.2020.00671
Zhang, W., Xu, W., and Xiong, S. (2011). Macrophage differentiation and polarization via phosphatidylinositol 3-kinase/Akt-ERK signaling pathway conferred by serum amyloid P component. J. Immunol. 187 (4), 1764–1777. doi:10.4049/jimmunol.1002315
Zhang, Z., Chen, H., Zhou, L., Li, C., Lu, G., and Wang, L. (2022). Macrophage-derived exosomal miRNA-155 promotes tubular injury in ischemia-induced acute kidney injury. Int. J. Mol. Med. 50 (3), 116. doi:10.3892/ijmm.2022.5172
Zhang, Z., Niu, L., Tang, X., Feng, R., Yao, G., Chen, W., et al. (2019). Mesenchymal stem cells prevent podocyte injury in lupus-prone B6.MRL-Faslpr mice via polarizing macrophage into an anti-inflammatory phenotype. Nephrol. Dial. Transpl. 34 (4), 597–605. doi:10.1093/ndt/gfy195
Zhuang, H., Han, S., Lu, L., and Reeves, W. H. (2021). Myxomavirus serpin alters macrophage function and prevents diffuse alveolar hemorrhage in pristane-induced lupus. Clin. Immunol. 229, 108764. doi:10.1016/j.clim.2021.108764
Zizzo, G., Hilliard, B. A., Monestier, M., and Cohen, P. L. (2012). Efficient clearance of early apoptotic cells by human macrophages requires M2c polarization and MerTK induction. J. Immunol. 189 (7), 3508–3520. doi:10.4049/jimmunol.1200662
Zoshima, T., Baba, T., Tanabe, Y., Ishida, Y., Nakatani, K., Nagata, M., et al. (2022). CCR2-and CCR5-mediated macrophage infiltration contributes to glomerular endocapillary hypercellularity in antibody-induced lupus nephritis. Rheumatol. (Oxford) 61 (7), 3033–3048. doi:10.1093/rheumatology/keab825
Keywords: M1 macrophages, M2 macrophages, SLE, lupus nephritis, LN
Citation: Cheng Y, Liu L, Ye Y, He Y, Hu W, Ke H, Guo Z-Y and Shao G (2024) Roles of macrophages in lupus nephritis. Front. Pharmacol. 15:1477708. doi: 10.3389/fphar.2024.1477708
Received: 08 August 2024; Accepted: 29 October 2024;
Published: 14 November 2024.
Edited by:
Edgar Jaimes, Memorial Sloan Kettering Cancer Center, United StatesReviewed by:
Dan Jane-wit, Yale University, United StatesCopyright © 2024 Cheng, Liu, Ye, He, Hu, Ke, Guo and Shao. This is an open-access article distributed under the terms of the Creative Commons Attribution License (CC BY). The use, distribution or reproduction in other forums is permitted, provided the original author(s) and the copyright owner(s) are credited and that the original publication in this journal is cited, in accordance with accepted academic practice. No use, distribution or reproduction is permitted which does not comply with these terms.
*Correspondence: Guojian Shao, d3pzaGFvZ2pAMTYzLmNvbQ==; Zhi-Yong Guo, ZHJndW96aGl5b25nQDE2My5jb20=
†These authors have contributed equally to this work and share first authorship
Disclaimer: All claims expressed in this article are solely those of the authors and do not necessarily represent those of their affiliated organizations, or those of the publisher, the editors and the reviewers. Any product that may be evaluated in this article or claim that may be made by its manufacturer is not guaranteed or endorsed by the publisher.
Research integrity at Frontiers
Learn more about the work of our research integrity team to safeguard the quality of each article we publish.