- 1Department of Endocrinology, Hospital of Chengdu University of Traditional Chinese Medicine, Chengdu, Sichuan, China
- 2Clinical Medical School, Chengdu University of Traditional Chinese Medicine, Chengdu, Sichuan, China
- 3Laboratory of Jinfeng, Chongqing, China
The rising incidence of fibrosis poses a major threat to global public health, and the continuous exploration of natural products for the effective treatment of fibrotic diseases is crucial. Berberine (BBR), an isoquinoline alkaloid, is widely used clinically for its anti-inflammatory, anti-tumor and anti-fibrotic pharmacological effects. Until now, researchers have worked to explore the mechanisms of BBR for the treatment of fibrosis, and multiple studies have found that BBR attenuates fibrosis through different pathways such as TGF-β/Smad, AMPK, Nrf2, PPAR-γ, NF-κB, and Notch/snail axis. This review describes the anti-fibrotic mechanism of BBR and its derivatives, and the safety evaluation and toxicity studies of BBR. This provides important therapeutic clues and strategies for exploring new drugs for the treatment of fibrosis. Nevertheless, more studies, especially clinical studies, are still needed. We believe that with the continuous implementation of high-quality studies, significant progress will be made in the treatment of fibrosis.
1 Introduction
Fibrosis can affect various tissues and organs, with the prevalence of fibrosis-related diseases approaching 5%, and the mortality rate due to fibrosis can be as high as 45% in some regions, resulting in a significant economic burden worldwide (Henderson et al., 2020; Zhao X. et al., 2020). The main pathological changes are excessive deposition of extracellular matrix (ECM), proliferation of fibrous connective tissue and reduction of parenchymal cells in organ tissues, which ultimately leads to structural destruction and functional decline of organs (Friedman et al., 2013; Henderson et al., 2020). Epithelial and endothelial cell damage, inflammatory responses, oxidative stress by various stimuli are common reasons of fibrosis formation and progression (Henderson et al., 2020; Wynn and Ramalingam, 2012; Zhao X. et al., 2020). Common diseases associated with fibrosis include cirrhosis, heart failure, idiopathic pulmonary fibrosis (IPF), nephropathy, diabetes and scleroderma (Zhao X. et al., 2020). For example, hepatic fibrosis (HF) is the result of acute or chronic liver injury, but the result of progressive ECM deposition can lead to histologic cirrhosis (Saxena and Anania, 2015; Zhao X. et al., 2020). Reactive and progressive interstitial fibrosis caused by persistent activation of myocardial fibroblasts leads to myocardial stiffness and ultimately to ventricular dysfunction (Wynn and Ramalingam, 2012). Multiple gene regulatory pathways are critical for driving the function of MFBs, including molecules downstream of the TGF-β receptor, including Smads (Inagaki and Okazaki, 2007; Zhao X. et al., 2020), and extracellular signaling regulators of AMP-activated protein kinase (AMPK), nuclear factor-erythroid 2-related factor 2 (Nrf2), and the inflammatory pathway (Zhao X. et al., 2020), which normally control fibrotic cell motility, proliferation and morphology through well-characterized intracellular signaling pathways (Casaletto and McClatchey, 2012) (Figure 1). Although a small number of drugs are available for the treatment of fibrosis, such as Nidanib and Pirfenidone, long drug cycles, adverse effects, and the lack of drugs targeting different organs remain a challenge to clinical treatment. Therefore, effective therapeutic strategies for fibrosis are urgently required.
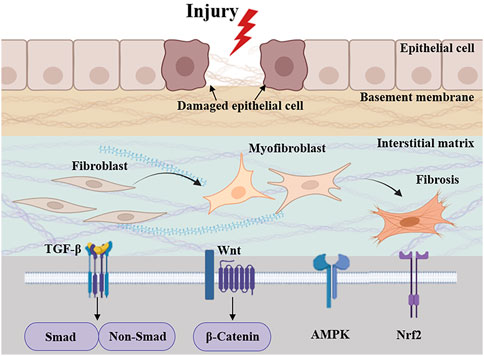
Figure 1. Pathologic processes and signaling cascades associated with fibrosis. Related signaling pathways include TGF-β, AMPK, Nrf2, and inflammatory pathway.
Natural products offer clear advantages in the treatment of diseases due to their high safety profile and diverse efficacy (Liu et al., 2024; Wang et al., 2024a). Rhizoma Coptidis is well known traditional Chinese medicine used in the treatment of fibrosis, inflammation, diabetes, etc. (Ma et al., 2024; Xiao et al., 2021; Yang et al., 2024). Rhizoma Coptidis was first described in Shennong Bencao Jing (Tan et al., 2016), and its anti-diabetic properties were first documented in Note of Elite Physicians (Zhang Q. et al., 2014). Berberine (BBR), one of the representative active ingredients of Rhizoma Coptidis (Shao et al., 2021), is an isoquinoline alkaloid and is also found in the leaves, twigs, barks, rhizomes, roots and stems of large numbers of plants, including Berberis kansuensis C.K. Schneid. (Berberidaceae), Poppyaceae, Coscinium fenestratum (Goetgh.) Colebr. (Menispermaceae), Phellodendron amurense Rupr. (Rutaceae), and Argemone mexicana L. (Papaveraceae) (Feng X. et al., 2019; Habtemariam, 2020; Imenshahidi and Hosseinzadeh, 2016; Singh and Mahajan 2013; Sun et al., 2023; Xu X. et al., 2021). It is an over-the-counter drug for the treatment of bacterial diarrhea and has a long history of medicinal use in both traditional Chinese medicines (Dang et al., 2020; Kong et al., 2020). Multiple studies have demonstrated that BBR has anti-inflammatory, anti-bacterial, anti-viral and anti-fibrotic, and has attracted intensive interest in the treatment of fibrosis of liver, heart, lung, kidney, pancreatic and other organs (Bansod et al., 2020; Che et al., 2019; Chitra et al., 2013; Gao et al., 2024; Tan E. et al., 2023; Xu X. et al., 2021; Zhang Z. et al., 2016).
Literature searches were performed using PubMed, Web of Science, and Google Scholar databases, and no filters were set for the searches in these three databases. The keywords included “berberine”, “fibrosis”, “anti-fibrotic effect”, “pharmacological effect”, “pharmacological mechanism”, “pharmacokinetics”, “safety” and “toxicity”. All articles included were published between 2004 and 2024. The purpose of this review is to focus on the therapeutic role and mechanism studies of BBR in fibrosis, and for this purpose, we read the titles, abstracts, and full text of publications from the past 20 years, removed publications that were not relevant to the topic of this review, and categorized them according to the type of fibrosis and mechanism. We also performed a correlation search for citations to relevant studies and review literature. Duplicates were removed using Endnote 20’s automatic and manual duplicate detection system, and a total of 168 references were finally included.
2 Anti-fibrotic effect of BBR
2.1 Hepatic fibrosis
The main pathological features of HF are activation of hepatic stellate cells (HSCs) and excessive deposition of protofibrillar collagen (Tacke and Weiskirchen, 2012; Yi et al., 2021). After liver injury, multiple factors such as cytokines, chemokines or reactive oxygen species (ROS) induce the differentiation of HSCs from quiescent to myofibroblasts (MFBs), and the overproduction of smooth muscle α-actinin (α-SMA), which ultimately leads to the overproduction and deposition of ECM components and the development of HF (Kong et al., 2012; Sanchez-Valle et al., 2012; Zimmermann and Tacke, 2011). BBR inhibits the activation of HSCs and the generation of α-SMA and suppresses the development of HF through multiple mechanisms (Figure 2).
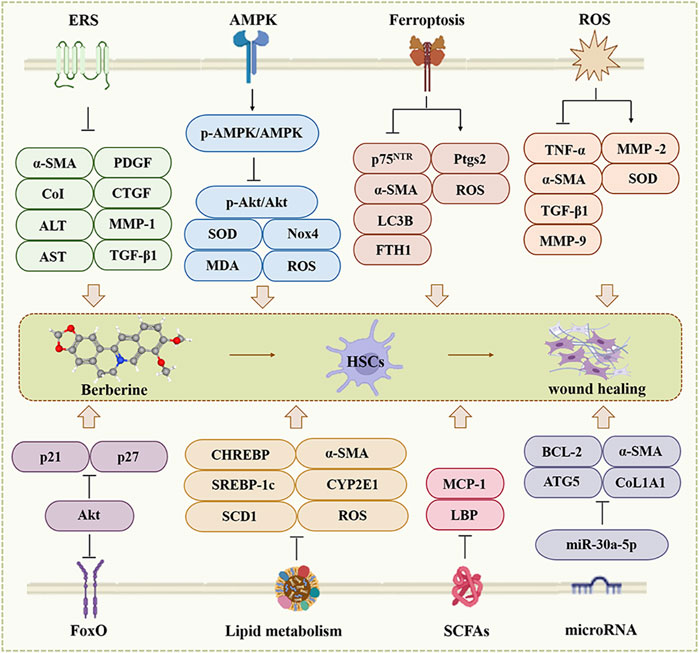
Figure 2. The effect of BBR on the hepatic fibrosis. ERS, AMPK, Ferroptosis, ROS, FoxO, Lipid metabolism, SCFAs and microRNA are involved in BBR for hepatic fibrosis.
2.1.1 Endoplasmic reticulum stress
Fibrotic signaling triggers transcription of procollagen I, which enters the endoplasmic reticulum and is released into the ECM via the endoplasmic reticulum (ER)-Golgi secretion compartment (Ajoolabady et al., 2023; Maiers et al., 2017). Perturbation of ER export of procollagen I induced ER stress (ERS) and UPR activation, leading to apoptosis in HSCs and upregulates fibrotic genes and Smad2 expression, accelerated progression of HF (Koo et al., 2016; Maiers et al., 2017; Xuan et al., 2021).
Previous studies clarified that 200 mg/kg of BBR for 5 weeks downregulated the expression of α-SMA, CoI, alanine aminotransferase (ALT), azelaic transaminase (AST), platelet-derived growth factor (PDGF), connective tissue growth factor (CTGF), metalloproteinase-1 (MMP-1), and TGF-β1 via ERS, and attenuated chronic liver injury, inflammation and fibrosis in vivo (Zhang Z. et al., 2016). On the other hand, BBR attenuated tunicamycin-induced triglyceride (TG) and collagen deposition in the liver of mice, compared to the tunicamycin group, BBR (75, 150 and 300 mg/kg) all reversed the levels of unfolded protein response (UPR)-related genes (CHOP, GRP78 and ATF6) that it upregulated, mainly by attenuating ERS (Yang et al., 2022). In summary, BBR can improve HF by modulating ERS.
2.1.2 AMPK pathway
AMPK, acts as a “metabolic sensor”, to regulate energy homeostasis and metabolic processes (Hardie et al., 2012; Li et al., 2014; Zhao P. et al., 2020). Researchers find AMPK regulates the activation of HSCs and inhibits TGF-β-induced fibrotic properties of HSCs, ameliorating liver injury and fibrosis (da Silva Morais et al., 2009; Zhao P. et al., 2020).
BBR (50 mg/kg) attenuated carbon tetrachloride (CCl4)-induced hepatic histological changes in mice, such as hepatocyte necrosis, adjacent hepatocyte steatosis, ballooning degeneration, lymphocyte infiltration, pseudofollicular and bridging formation through up-regulating the p-AMPK/AMPK ratio in activated HSCs, thereby decreasing the ratio of p-Akt to total Akt, strongly increased serum and liver tissue superoxidedismutase (SOD) activity in CCl4-induced hepatic injury in mice, decreased serum Malondialdehyde (MDA) level and NADPH oxidase 4 (NOX4) expression in liver tissues, reducing ROS production and effectively preventing HF (Li et al., 2014).
2.1.3 Ferroptosis
Emerging research suggests that ferroptosis is characterized by redox-active iron accumulation, ROS generation, lipid peroxidation, and glutathione depletion, and the iron death inhibitor fer1 helps to inhibit the activation of HSCs in vitro (Yi et al., 2021).
In thioacetamide (TAA) and CCl4 induced HF in mice, compared to the model group, another proposed mechanism of BBR associated anti-fibrotic effect was based on inhibits HSCs activation through ferroptosis, by which BBR reduced expression of hyaluronic acid (HA), p75NTR (HSC activation marker), ALT, AST and iron deposition, as well as lowering of Ishak score (Passino et al., 2007; Yi et al., 2021). Furthermore, treatment with 21 µM BBR for 24 h affected HSCs but not hepatocytes and reduced TAA-induced α-SMA expression, modulating the viability and proliferation of HSCs in a dose- and time-dependent manner (Yi et al., 2021). Besides, studies have shown that BBR treatment can decreased LC3B and FTH1 expression, enhanced Ptgs2 and ROS levels, promoted ferritin hydrolysis, and increased iron overload in HSCs, suggesting that BBR enhances HSCs ferroptosis through inhibition of autophagy, which is beneficial to HF (Yi et al., 2021). In addition, BBR inhibited the proliferation of HSCs in a dose-dependent manner and its IC50 value is 66.86 mM, but combination with sorafenib (10 µM) reduced it to 15.61 µM; molecular docking experiments further demonstrated that BBR binds to PEBP1 (can trigger ferroptosis), with a maximum binding energy of −8.51 kcal/mol; so BBR can promote HSC ferroptosis to alleviate HF through binding to PEBP1 (Xie et al., 2023).
2.1.4 Oxidative stress
Oxidative stress is a vital factor in the pathogenesis of HF. Injured hepatocytes release ROS and inflammatory cytokines which are involved in HSCs activation and recruitment of immune cells to liver tissue (Che et al., 2023; Li N. et al., 2021; Xu et al., 2022). Among them, interleukin 17 (IL-17) promotes ECM production by HSCs and exacerbates HF (Ma et al., 2020; Meng et al., 2012; Seo et al., 2016). Thus, hepatic ROS trigger complex interactions between activated HSCs and recruited immune cells, thereby exacerbating fibrosis and inflammation within the liver.
Accumulating evidences demonstrated that BBR hinder HF through oxidative stress. In the CCL4-induced HF model in Balb/c mice, different doses of BBR (9 and 50 mg/kg) were treated for 2 weeks that ameliorated the increase in plasma enzyme activities and oxidative stress, decreased tumor necrosis factor-α (TNF-α), α-SMA, transforming growth factor beta 1 (TGF-β1) and MMP-9 expression, the levels of MMP-2 is increased, and induced the Cu/Zn SOD activity to be normalization (Domitrovic et al., 2013b). BBR reduced ALT, AST, MDA and hydroxyproline (HYP) levels, as well as increased SOD levels, compared with the CCL4-induced fibrosis group of male Wistar rats (Zhang et al., 2008). BBR (5 µM) can reduced cellular steatosis, hindered ROS, inflammatory cytokines and collagen production in vitro (Rafiei et al., 2023). This indicate that BBR treatment can promote liver repair by ameliorating oxidative stress.
2.1.5 Metabolism
Disorders of lipid metabolism, such as fatty acid and cholesterol metabolism, are one of the pathologic bases of many liver diseases (Arain et al., 2017; Huang et al., 2021; Liu et al., 2022; Scorletti and Carr, 2022). In non-alcoholic fatty liver disease, dysregulation of the lipid balance in the body can lead to a severe accumulation of triacylglycerols in the liver cells, which can progress to non-alcoholic steatohepatitis, hepatic fibrosis and even cirrhosis (Grabner et al., 2021). Lipid metabolism in the liver is regulated by various mechanisms, including peroxisome proliferator-activated receptors (PPARs) and sterol regulatory element binding protein (SREBP) (Grabner et al., 2021).
BBR downregulates the expression of the lipid metabolism-related gene stearoyl coenzyme A desaturase 1 (SCD1) (Yang et al., 2022), reduces TG biosynthesis and enhances TG oxidation to ameliorate hepatic steatosis and HF in vivo (Bansod et al., 2021; Boudaba et al., 2018; Huang et al., 2021; Wang et al., 2016; Zhang et al., 2019). Other studies have reported that BBR supplementation improves total cholesterol, low-density lipoprotein C and high-density lipoprotein C in the blood and accelerates cholesterol excretion through inhibition of adipocyte enhancer-binding protein one or enhancement of cholesterol-binding receptor, which explains its hepatoprotective properties in vivo and in vitro (Derosa et al., 2013; Kong et al., 2004; Wang and Zidichouski, 2018; Wei et al., 2016).
PPARs are considered important metabolic regulators of hepatic lipid metabolism and inflammation (Boyer-Diaz et al., 2021; Chen et al., 2023). PPAR-γ inhibits the activation of HSCs and regulates the expression of genes related to adipogenesis and fibrogenesis to prevent HF progression (Brunner et al., 2019; Perez-Carreon et al., 2010; Sanyal et al., 2010; Yuan et al., 2004). BBR enhances the expression of the gene encoding the FAO carnitine palmitoyl transferase IA by interacting with PPAR-α to inhibit the production of TG in vivo (Yu et al., 2016; Zhou et al., 2008). BBR contains potential agonists of all PPAR isoforms (Xia et al., 2013; Yu et al., 2016), and these could act as ligands to regulate the progression of HF. Taken together, BBR may restore lipid homeostasis and regulate liver function by modulating the PPARs signaling cascade.
SREBP-1c, CHREBP, FAs and C/EBPβ as adipogenic regulators, are involved in the regulation of steatosis, inflammation and fibrosis, however, BBR downregulates their mRNA levels in tunicamycin-induced mice, and mRNA levels of ROS, CYP2E1 (a major mediator of lipid peroxidation), and the expression of TNF-α, IL-6, α-SMA, TIMP-1 and TGF-β1 that are the anti-fibrotic effect of BBR was fully utilized (Zhang Z. et al., 2016).
2.1.6 Gut microbiota
Gut microbiota is a complex system that regulates certain biochemical, physiological and immune responses to maintain the health of the organism (Mishra et al., 2021). It alters the microenvironment of the organism by directly influencing metabolic signaling and energy metabolism either by itself or by producing certain metabolites, which can lead to inflammation, autoimmunity and metabolic disorders (Thursby and Juge, 2017). Thus, a growing body of research suggests that ecological dysregulation of the gut microbiota and impairment of its composition and function are strongly associated with many metabolic diseases (Cai and Kang, 2023; Wang et al., 2021).
Short-chain fatty acids (SCFAs), the end products of anaerobic microbial fermentation of indigestible carbohydrates, have a profound impact on gut function and host energy metabolism (Nicholson et al., 2012). BBR (100 mg/kg) treatment increased the concentration of SCFAs in the gut, increased the production of gut flora-derived butyrate, and decreased lipopolysaccharide (LPS) binding protein (LBP), monocyte chemotactic protein-1 (MCP-1), leptin and lipocalin, and promotes homeostasis of the hepatic microenvironment in vivo (Zhang et al., 2012).
2.1.7 Forkhead box O pathway
Forkhead box O (FoxO) is an essential class of transcription factors involved in numerous biological processes, such as cell cycle, cell proliferation, apoptosis and anti-oxidative stress (Calissi et al., 2021; Sun et al., 2009). p21 and p27 are FoxO-specific transcriptional targets that are associated with G1-phase cell cycle arrest (Roy et al., 2011; Sun et al., 2009). p27 is the key downstream target of FoxO1 to control proliferation and differentiation of HSCs (Sun et al., 2009). The protein kinase B (Akt) signaling cascade controls the transcriptional activity of p21 and p27 through phosphorylating FoxO1 reversing its transcriptional activity (Sun et al., 2009).
This study reported that BBR (5, 10 and 20 μg/mL) induced subcellular redistribution of FoxO1 from the cytoplasm to the nucleus in hepatic stellate cells (CFSCs) in vitro, which reduced the number of activated HSCs, fibrotic septa, and hepatic collagen. Furthermore, it decreases p-FoxO1 (Ser-256) and p-Akt, increases p21 and p27 expression, and inducts G1 blockade, which directly inhibited the CFSC proliferation, which has a protective effect on the liver (Sun et al., 2009).
2.1.8 Another pathway
Numerous studies have proved that enhanced autophagy can induce fibrotic diseases in many organs (Marcelin et al., 2020; Shang et al., 2020; Zhang et al., 2020). After liver injury, increased autophagy promotes the activation of HSCs (Thoen et al., 2011; Wang B. et al., 2017; Zhang et al., 2017). Different microRNAs (miR) involved in various fibrotic diseases. MiR-15 family mainly promotes cell proliferation and apoptosis. MiR-199 and miR-200 families are responsible for ECM deposition and pro-fibrotic cytokine release (Jiang et al., 2017; Schmiedel et al., 2015).
BBR reduced the expression of ATG5, BCL-2, HYP, α-SMA, collagen type I alpha (COL1A1), LC3, ALT, and AST, attenuated collagen deposition and inflammatory cell infiltration, and up-regulating the expression of p53, BAX, and cleaved PARP, and enhanced hepatic repair through up-regulating the expression of miR-30a-5p in vivo and in vitro (Tan Y. et al., 2023). Cyclooxygenase-2 (COX-2) is rapidly synthesized by cells in response to various stimuli involving different pathophysiological processes. BBR can inhibit the expression of COX-2 in vivo and in vitro to protect cells from excessive inflammatory responses, which is beneficial to HF (Feng et al., 2012; Guo et al., 2008; Li et al., 2012; Zeng et al., 2011).
Overall, BBR can mediate different signaling cascades such as ERS, AMPK, ferroptosis, oxidative stress, metabolism, gut microbiota, and FoxO to exert anti-fibrotic effects (Figure 2). However, HF is a complex process, and the activation of HSCs and excessive deposition of collagen fibers are necessary processes that must be taken into account in the future development of new target mechanisms and drugs.
2.2 Myocardial fibrosis
One of the major events that occurs in myocardial fibrosis (MF) when the heart is injured is the activation and differentiation of cardiac fibroblasts (CFs) into MFBs, which contribute to ECM turnover and collagen deposition (Liu et al., 2021). Activated CFs are central cellular effectors of MF and are the main source of ECM proteins in MF (Frangogiannis, 2021). BBR hinders CFs activation and differentiation, and reduces ECM production to achieve anti-MF (Figure 3).
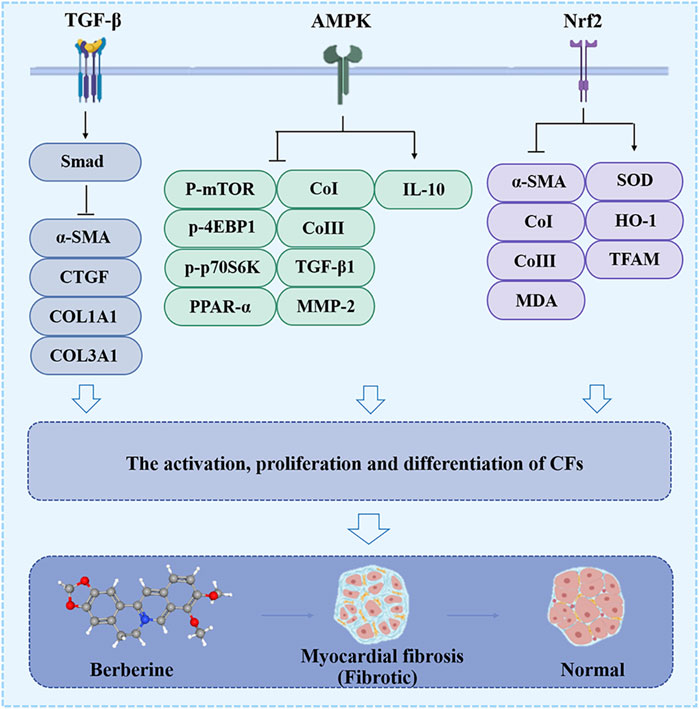
Figure 3. The effect of BBR on the myocardial fibrosis. Related signaling pathways include TGF-β, AMPK and Nrf2 pathway.
2.2.1 TGF-β/smad pathway
Previous studies elaborated that TGF-β producing inflammatory cells are essential in the critical cellular event of CFs activation and ECM deposition (Frangogiannis, 2020; Liu et al., 2021). In isoprenaline (ISO)-induced MF in Sprague-Dawley rats, BBR (10, 30 and 60 mg/kg) attenuated macrophage infiltration, altered macrophage phenotype, reduced the expression of COL1A1, collagen type III alpha (COL3A1), CTGF, TGF-β1, and α-SMA, and inhibited the proliferation of CFs, all of which were achieved by blocking the activation of the TGF-β1/Smad signaling cascade (Che et al., 2019).
2.2.2 AMPK pathway
Several studies have confirmed that BBR downregulates the p-mTOR, p-4EBP1 and p-p70S6K (Thr389), inhibits the proliferation of CFs and their conversion to MFBs, reduces the expression of CoI, CoIII, TGF-β1, MMP-2 and MMP-9, shrinks the size of the MFs, increases the secretion of IL-10, and ultimately inhibits MFs and improves cardiac dysfunction in vivo and in vitro. The pharmacological mechanism of the above results is strongly related to the phosphorylation of AMPK (Ai et al., 2015; Chen et al., 2020).
2.2.3 Nuclear factor-erythroid 2-related factor 2 pathway
Nuclear factor-erythroid 2-related factor 2 (Nrf2) translocate into the nucleus as a transcription factor and induces the expression of downstream anti-oxidant and detoxification enzymes, such as heme oxidase-1 (HO-1), SOD, Glutathione peroxidase (GPx) (Wang et al., 2023). Nrf2 can counterbalances oxidative stress, also affects TGF-β1-mediated phosphorylation of Smad3, which is indispensable for fibrosis development (Kobayashi et al., 2016).
Recent studies have elucidated that in the DOX-induced MF model in male Sprague-Dawley rats, BBR (60 mg/kg) targeting Nrf2 can downregulated the expression of α-SMA, CoI, CoIII, and MDA, inhibited the differentiation of CFs to MFBs, and increased SOD activity, HO-1, and mitochondrial transcription factor A (TFAM), rescuing mitochondrial morphology damage and loss of membrane potential (Wang et al., 2023), further protecting the normal functioning of the heart.
Taken together, the conversion of CFs to MFBs occurs at a central event in CF, and BBR prevents this event through the TGF-β/Smad, AMPK, and Nrf2 pathways (Figure 3). Hitherto, there is still a critical lack of research on the target and mechanism of BBR in MF treatment.
2.3 Pulmonary fibrosis
Pulmonary fibrosis (PF) is a heterogeneous lung mesenchymal disorder that includes persistent epithelial injury, abnormal wound healing, and excessive ECM deposition (Dong and Ma, 2016). MFBs, fibroblasts, and other cell types that secrete numerous of ECM into the alveolar structures, continued differentiation, proliferation, and migration of fibroblasts triggered by a variety of fibrotic mediators contribute to the formation of fibrotic foci and subsequent lung structural remodeling (Wang et al., 2024b). However, BBR plays a unique role in the treatment of PF (Figure 4).
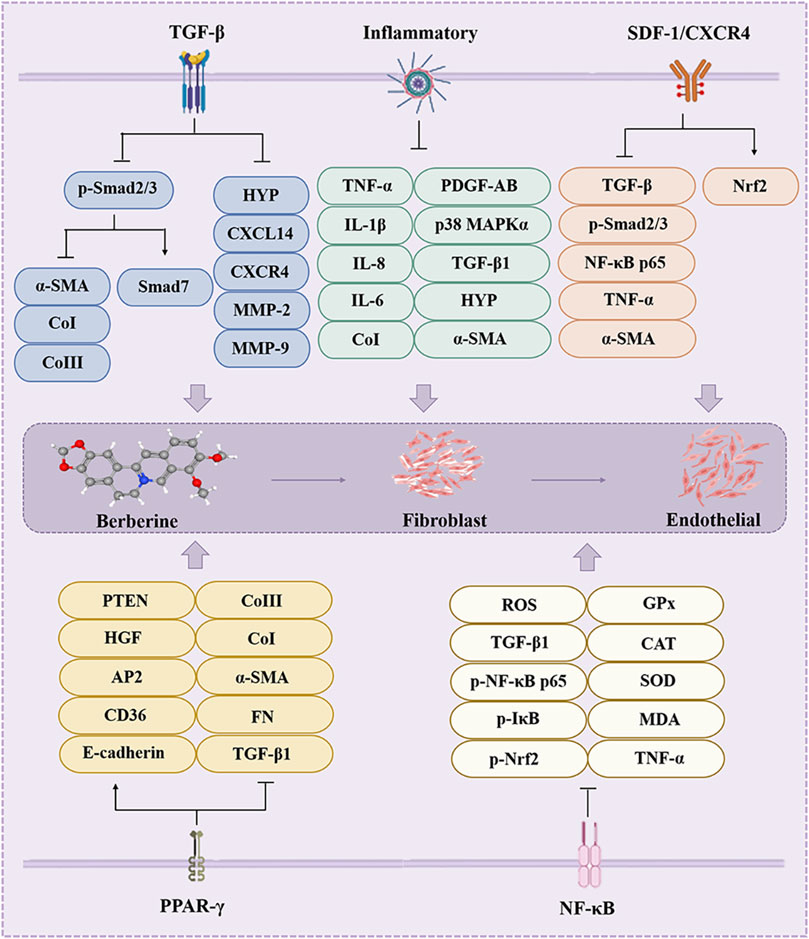
Figure 4. The effect of BBR on the pulmonary fibrosis. Inflammatory, AMPK, TGF-β, Nrf2, Notch/Snail and SIRT2 are involved in BBR for pulmonary fibrosis.
2.3.1 TGF-β pathway
TGF-β, a critical inducer of epithelial-mesenchymal transition (EMT), promotes EMT in alveolar epithelial and endothelial cells, restores tissue morphology and structure, and induces fibroblasts to convert to MFBs, synthesize and secrete ECM (Li et al., 2011; Tew et al., 2020). TGF-β-mediated Smad and non-Smad signaling cascades are thought to be major players in accelerated PF(Higashiyama et al., 2007; Wynn, 2011). In the BLM-induced PF in male Wistar albino rats, BBR (200 mg/kg) reverses bleomycin (BLM)-induced lung ultrastructural changes, enhances Smad7 expression, and downregulates α-SMA, CoI and CoIII expression through inhibits BLM-induced elevation of p-Smad 2/3 (Chitra et al., 2015; Tan E. et al., 2023; Palanivel et al., 2015). Another study clarified that BBR reduced the levels of HYP, CXCL14, CXCR4, CoI/III, MMP-2, MMP-9, α-SMA and p-Smad 2/3, and prevented the activation of Smad2/3, ensured recovery of lung status and function (Li et al., 2019).
2.3.2 Inflammatory
Pathologically, PF is always first accompanied by an inflammatory response (Feng F. et al., 2019), inflammatory cells multitask at the wound site by facilitating wound debridement and producing chemokines and growth factors (Eming et al., 2017).
In the PM2.5-induced MF in male C57BL/6 mice and BLM-induecd MF in male Kunming mice, BBR (50 mg/kg) treatment reduces inflammatory cell aggregation in the lungs and decreases the expression of pro-inflammatory cytokines TNF-α, IL-8, IL-1β, and IL-6; it also downregulates the levels of COI, COIII, TGF-β1, PDGF-AB, HYP, α-SMA, p38 MAPKα, and p38 MAPKα (pT180/Y182) and inhibits collagen production and deposition (Alnuqaydan et al., 2022; Beik et al., 2023; Tew et al., 2020; Yang et al., 2023; Zhao et al., 2023).
2.3.3 Stromal cell-derived factor-1/CXCR4 pathway
CXCL12 is involved in the mobilization of bone marrow-derived stem cells through Stromal cell-derived factor-1 (SDF-1) receptor C-X-C chemokine receptor 4 (CXCR4), which is abundantly expressed on a wide range of cells and may be considered a permanent reservoir for fibroblasts (Ahmedy et al., 2023).
Recently, a study found that in the BLM-induecd MF in albino male mice, BBR (5 mg/kg) greatly inhibited BLM-induced weight loss and elevated lung index, preserved lung structure and attenuated bronchoalveolar injury, and ameliorated lung injury, which through reduced expression of SDF1 and CXCR4 (Ahmedy et al., 2023). And further inhibited the expression of TGF-β, p-Smad2/3, α-SMA, NF-κB p65, TNF-α, IL-6, MDA, GSH, SOD and CAT, effectively attenuated oxidative stress, enhanced the expression of Nrf2, and ultimately attenuated epithelial mesenchymal transition of PF (Ahmedy et al., 2023).
2.3.4 PPAR-γ pathway
BBR can directly enter the cytoplasm of fibroblasts and act as a PPAR-γ agonist, up-regulating the nuclear translocation, DNA-binding activity, and transcriptional activity of PPAR-γ, and reversing PM2.5-induced collagen deposition, the expression of fibroblast markers (TGF-β1, FN, α-SMA, COI, and COIII), and the expression of E cadherin expression upregulation, promotes CD36 and AP2 mRNA expression, HGF and PTEN protein levels, and attenuates oxidative and inflammatory factor-mediated PF in BLM-induecd female ICR mice (Guan et al., 2018; Zhao et al., 2023).
2.3.5 Nuclear factor-κB pathway
The BBR-mediated nuclear factor-κB (NF-κB) pathway attenuated the extent of lung oxidative damage and PF. In BLM-induecd male wistar albino rats, BBR (200 mg/kg) action was manifested as inhibition of TGF-β1, ROS, iNOS, TNF-α, MDA, OH, NO, and myeloperoxidase (MPO) levels through downregulation of the phosphorylation of Nrf2, IκB, and NF-κB p65; inhibition of HYP and histamine release, significant reduction of mast cell recruitment, and reversal of BLM-induced SOD, CAT, GPx and GR activities, and restored the levels of non-enzymatic antioxidant status of glutathione, vitamin A, vitamin C and vitamin E (Chitra et al., 2013; Sun et al., 2022).
The special structure of the lung poses a challenge for the treatment of PF, and although BBR can protect the lung structure and reduce lung damage through various pathways (such as TGF-β) (Figure 4), researchers are encouraged to conduct in-depth studies, especially clinical studies, to provide stronger and more comprehensive evidence for the treatment of fibrotic diseases with BBR.
2.4 Renal fibrosis
Renal fibrosis (RF) is a common manifestation of various chronic kidney diseases and representative events include increased matrix production and inhibited degradation to promote intercellular matrix interactions, cyst-like cell and fibroblast activation, tubular epithelial-mesenchymal transition, MFBs activation, immune cell infiltration and apoptosis (Sun et al., 2022). Almost all cells in the kidney are associated with the fibrotic process, including fibroblasts, tubular epithelial cells, endothelial cells, lymphocytes and macrophages (Sun et al., 2022). Thus, BBR may achieve renal protection through these cellular interactions and associated factors (Figure 5).
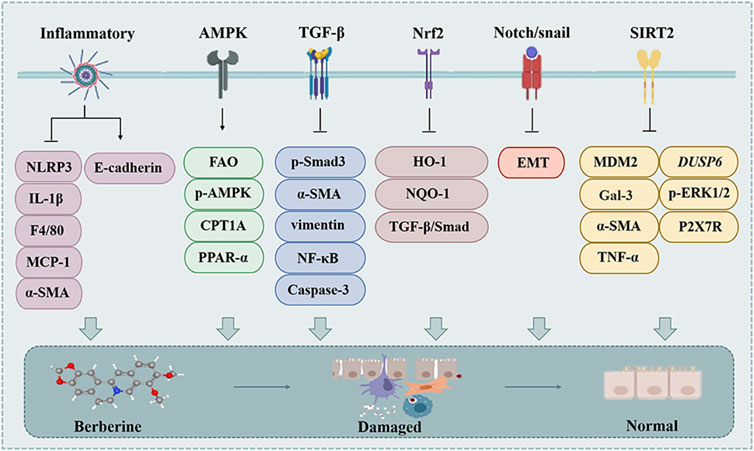
Figure 5. The effect of BBR on the renal fibrosis. Related signaling pathways include TGF-β, inflammatory, SDF-1/CXCR4, PPAR-γ and NF-κB pathway.
2.4.1 TGF-β pathway
TGF-β1 is a major driver of RF, and is thought to act either directly or indirectly on a variety of cell types of the kidney to promote the fibrotic process (Meng et al., 2016).
BBR intervention can downregulate TGF-β, p-Smad3, α-SMA, vimentin, NF-κB mRNA and protein levels, relieves renal injury and inhibits RF in vivo and in vitro (Hassanein et al., 2022; Li and Zhang, 2017; Wang et al., 2014). BBR alleviates adriamycin (DOX)-induced RF through reduced TGF-β, caspase-3 and NF-κB expression and collagen deposition in vivo, ultimately slowing down the progression of RF (Ibrahim Fouad and Ahmed, 2021; Song et al., 2024).
2.4.2 Inflammatory
RF pathogenesis mainly includes inflammation-induced tubular epithelial cell (TEC) injury, and inhibition of inflammatory progression can delay or reverse RF (Song et al., 2024; Tan E. et al., 2023). BBR (30 μM and 50 mg/kg) attenuated the extent of interstitial fibrosis and histopathological damage through inhibiting the activation of NLRP3 inflammasome and IL-1β level, which reduced the expression of F4/80, MCP-1, CoI, CoIV, and α-SMA, upregulated the level of E-cadherin, and alleviated the abnormalities of serum creatinine and urea nitrogen levels in vivo and in vitro (Song et al., 2024; Tan E. et al., 2023). In summary, BBR has a protective effect on RF.
2.4.3 AMPK pathway
AMPK is a key factor contributing to the onset and progression of renal interstitial fibrosis. It can enhance mitochondrial FAO in response to decreased ATP levels (Li et al., 2022). BBR (30 μM and 50 mg/kg) intervention reversed the downregulation of p-AMPK in the kidney and reversed the downregulation of FAO-associated proteins in vivo and in vitro, including CPT1A and PPAR-α (Tan E. et al., 2023).
2.4.4 Nrf2 pathway
On the one hand, BBR (200 mg/kg) was found to attenuate RF induced by STZ in C57BL/6J mice by activating the Nrf2 signaling pathway in Diabetic Nephropathy (DN); on the other hand, knockdown of Nrf2 not only counteracts BBR-induced HO-1 and NQO-1 expression, but also reverses the inhibitory effect of BBR on high glucose (HG)-induced TGF-β/Smad signaling activation and anti-fibrotic effect (Hassanein et al., 2022; Zhang X. et al., 2016).
2.4.5 Notch/snail pathway
The Notch pathway has been shown to mediate cellular fibrosis such as EMT in epithelial cells in DN and is associated with TGF-β1 (Yang et al., 2017). Snail1 expression is directly regulated by the Notch signaling, and the notch/snail pathway is an important mechanism in renal interstitial fibrosis in DN (Yang et al., 2017).
BBR (30 μM and 150 mg/kg) blocked HG-induced EMT events, and inhibited expression of notch and snail1 in renal tubular epithelial cells, suppressing tubular EMT and renal interstitial fibrosis in vivo and in vitro (Yang et al., 2017).
2.4.6 Sirtuin 2/murine double minute2 pathway
Class III histone deacetylase Sirtuin 2 (SIRT2) is a vital member of nicotinamide adenine dinucleotide-dependent protein deacetylases, which occupy a key position in inflammation and fibrogenesis (Ahmedy et al., 2022). BBR (200 mg/kg) inhibited cisplatin-induced SIRT2 and Murine double minute2 (MDM2) expression, further reduced hemagglutinin-3 (Gal-3), α-SMA, TNF-α, DUSP6, and reduced the mRNA levels of P2X7R and p-ERK1/2 to achieve relief of RF induced by cisplatin in female Wistar rats (Ahmedy et al., 2022).
During the development of RF, fibrotic tissue replaces nephrons in the kidneys, and the problem of increased tissue stiffness brought about by excessive accumulation of ECM hinders drug distribution and efficacy. Although various studies have shown that BBR has multiple targets and mechanisms for targeting RF (Figure 5), the above problems cannot be ignored and more researchers are called to consider them in future studies.
2.5 Pancreatic fibrosis
Pancreatic fibrosis is primarily associated with the activation of pancreatic stellate cells (PSCs), which results in the secretion of excess ECM proteins (An et al., 2023; Masamune et al., 2009). Pro-fibrotic mediators activate quiescent PSCs into MFBs, such as TGF-β (Masamune et al., 2009). AMPK is a metabolite sensor protein that is predominantly expressed in all organs and ameliorates inflammation and fibrosis by regulating macrophage polarization.
In the cerulein-induced pancreatic fibrosis in male Swiss albino mice, BBR dose-dependently decreased levels of pancreatic MDA, nitrite, TNF-α, IL-6, IL-1β, TGF-β1, α-SMA, COL1A, COL3A, and p-Smad2/3, reduced inflammatory cell infiltration, vesicular cell atrophy, exocrine pancreatic vacuolization, ECM deposition and EMT program; increased the content of GSH, and the expression of E-cadherin, p-AMPKα, p-AMPKβ, ACC and Smad7 expression (Bansod et al., 2020). Taken together, BBR prevented the progression of chronic pancreatitis and associated fibrosis in an AMPK-dependent manner by inhibiting TGF-β/Smad signaling and regulating macrophage polarization (Bansod et al., 2020).
2.6 Adipose tissue fibrosis
In the presence of overnutrition, adipose tissue undergoes rapid dynamic remodeling through adipocyte hypertrophy and hyperplasia (Koenen et al., 2021; Sun et al., 2011), accompanied by elevated accumulation of immune cells and overproduction of ECM, leading to the development of fibrosis (Li et al., 2022; Wang et al., 2018). Adipose tissue fibrosis is a hallmark of obesity-related adipose tissue dysfunction (Hu et al., 2018).
2.6.1 AMPK pathway
Previous studies have shown that TGF-β signaling is associated with adipose ECM remodeling and that inhibition of TGF-β by activated AMPK alleviates adipose tissue fibrosis (Wang et al., 2018; Xu X. et al., 2021). Besides, BBR inhibits TGF-β1/Smad3 in HFD-induced white adipose tissues by attenuating macrophage infiltration and polarization, and by activating the AMPK pathway. TGF-β1/Smad3 signaling and ameliorated obesity-associated adipose tissue fibrosis (Wang et al., 2018; Xu X. et al., 2021). Notably, the inhibitory effect of BBR on adipose tissue fibrosis was blocked by compound C (an AMPK inhibitor) (Wang et al., 2018; Xu X. et al., 2021). The C57BL/6 mice adipose tissue fibrosis model used in the above study was induced by the HFD. The above results provide sufficient evidence that BBR attenuates adipose tissue fibrosis through AMPK pathway.
2.6.2 Hypoxia-inducible factor 1α pathway
Hypoxia is an early event in adipose tissue dysfunction, and hypoxic conditions promote the expression of hypoxia-inducible factor 1α (HIF-1α). HIF-1α-induced transcriptional program that leads to the enhanced synthesis of ECM components and ultimately promotes the development of fibrosis in white adipose tissue (Hu et al., 2018). BBR attenuated HFD-induced fibrosis and fibroblast proliferation through reducing CoI, α-SMA, platelet-derived growth factor receptor α (PDGFR-α) and HIF-1α expression, and inhibiting aberrant ECM protein synthesis in vivo. All of the above results were achieved by reversing HFD-induced HIF-1α activation and transcription by BBR (Hu et al., 2018).
2.6.3 Another pathway
Adipose tissue macrophages are found in up to 50% of adipose tissue in obese rodents and humans. Under obesity, the M2 macrophage phenotype is transformed into M1 macrophage phenotype. BBR regulates macrophage infiltration and polarization by decreasing the expression of iNOS, COX-2, IL-1β, IFN-γ, F4/80, MCP-1, and MMP-1α, reducing abnormal ECM deposition to reduce inflammation and fibrosis in adipose tissue through down-regulating the expression of COI, α-SMA, α-SMA, MMP-9 and TIMP-1 in vivo (Li et al., 2022).
2.7 Epidural fibrosis
Epidural fibrosis (EF) is the result of a physiological cyclic defense response during wound healing, a cycle in which fibroblasts in the healing area proliferate rapidly in response to inflammatory mediators and growth factors, and an excessive healing response leads to increased formation of scar tissue, resulting in excessive and disorganized matrix deposition (Keskin et al., 2022).
During wound healing, immune cells (such as monocytes and macrophages) together with fibroblasts and smooth muscle cells produce high levels of TGF-β1, further causes proliferation and accumulation of fibroblasts in the ECM, leading to a vicious cycle of EF after laminectomy (Gorgulu et al., 2004). In the Laminectomy-induced EF in Wistar albino rats, it's worth noting that BBR downregulates the expression of TGF-β1 further attenuate EF (Gorgulu et al., 2004). It has also been shown that BBR prevents apoptosis in human-derived myeloid cells by reducing oxidative stress induced by autophagy and endoplasmic reticulum stress induced by Ca2+ dysregulation (Luo et al., 2019). Other study found that BBR reduced HYP expression stopping the development of EF (Keskin et al., 2022).
Briefly, BBR plays a unique role in pancreatic, adipose tissue, and epidural fibrosis using different mechanisms, but relevant studies are insufficient to illustrate the therapeutic role of BBR in various types of organ fibrosis. Therefore, studies on the anti-fibrotic effects of BBR are indispensable in the future.
3 Anti-fibrotic effects of BBR derivatives
The absolute bioavailability of BBR is low and more than half of the original BBR is not absorbed by the intestinal tract, however, BBR is converted by the intestinal flora into absorbable metabolites such as compounds like dihydroberberberine (dhBBR), oxyberberine (OBB), canadine, and others (Chen et al., 2011; Feng et al., 2015; Liu et al., 2010). Previous studies have demonstrated that dhBBR reduces the release of caspase-1, apoptosis-associated speck-like protein (ASC), and IL-1β through NLRP3 inflammatory vesicle-associated mechanisms to inhibit pyroptosis (a form of programmed cell death that occurs in HF) (de Carvalho Ribeiro and Szabo, 2022; Xu L. et al., 2021). OBB treatment has been reported to increase SOD, catalase (CAT), and GPx activities, and decrease ROS, MDA, and MPO concentrations, thereby reducing oxidative stress (Dou et al., 2021). Therefore, OBB-mediated recovery of liver function may impede the progression of liver disease and promote liver regeneration (Dou et al., 2021). OBB has also been clarified to ameliorate pathological deterioration of adipocytes and hepatocytes via the AMPK pathway and to stimulate energy expenditure to control lipid homeostasis at a smaller dose than BBR. In addition, OBB was shown to inhibit macrophage migration and promote phenotypic conversion of M1 macrophages to M2 macrophages, ultimately reducing the inflammatory burden of the liver (Li Q. P. et al., 2021).
BBR hydrochloride (BH), which is another BBR derivative, ameliorated PM2.5-induced PF by inhibiting oxidative stress and inflammation (Zhao et al., 2023). BH concentration-dependently decreased the expression of TGF-β1, CTGF, ICAM-1, IL-1β, and p-P38, exerting anti-inflammatory and anti-fibrotic effects (Zhao et al., 2023). Postoperative adhesions are a common cause of peritoneal fibrosis. Studies have delayed that in the Surgery-induced peritoneal fibrosis in male Sprague-Dawley rats, BH reduces the expression levels of IL-1β, IL-6, TGF-β, TNF-α, ICAM-1, p-JNK and p-NF-κB, prevents adhesions after abdominal surgery, and reduces inflammation and prevents peritoneal fibrosis through inhibiting TAK1/JNK and TAK1/NF-κB signaling (Zhang Y. et al., 2014).
Additionally, it has been reported that proto-berberine alkaloids attenuate skin fibrosis by modulating mitochondrial dehydrogenase activity, cell proliferation, collagen production, and the ability of inflammatory cytokines (IL-1β and IL-6) production in vitro (Pietra et al., 2015).
4 Pharmacokinetics
BBR has a low oral bioavailability due to poor intestinal absorption and rapid metabolism, Hua et al. used the liquid chromatography-electrospray ionization-mass spectrometry method to demonstrate that plasma concentrations were only 0.4 ng/mL at an oral dose of 400 mg (Hua et al., 2007). Oral bioavailability of BBR is hampered by intestinal first-pass elimination, hepatic distribution and p-glycoprotein (Pg-P) pumps (Imenshahidi and Hosseinzadeh, 2016; Liu et al., 2010). After 4 h of oral administration of BBR (200 mg/kg) to rats, the distribution of BBR in organs was at least 10–30 times higher than that in plasma, with the highest distribution in the liver, followed by the kidney, this may provide an explanation for the pharmacologic effects of BBR in clinical human disease (Kumar et al., 2015; Tan et al., 2013; Wang et al., 2005). The metabolites of BBR are mainly berberrubine (up to 65.1%), thalifendine, demethylene-berberine and jatrorrhizine, which are ultimately excreted in urine, bile and feces (Feng X. et al., 2019).
The bioavailability of BBR is the biggest hindrance to its clinical application, and several strategies have been found to improve the bioavailability of BBR (Imenshahidi and Hosseinzadeh, 2016). For example, Chitosan-N-Acetylcysteine and β-cyclodextrin modulated Pg-P activity to further enhanced the intestinal absorption of BBR (Imenshahidi and Hosseinzadeh, 2016). Chen et al. found that 2.5% D-α-tocopherol polyethylene glycol 1,000 succinate increased the area under the curve (AUC) (0–36) of BBR up to 1.9-fold, possibly through inhibition of Pg-P activity (Chen et al., 2011). Godugu et al. prepared a mucoadhesive microparticle formulation of BBR that enhanced the AUC of BBR up to 6.98-fold (Godugu et al., 2014), whereas oral BBR microemulsion formulation increased the bioavailability of BBR up to 6.47-fold (Gui et al., 2008). Wang et al. improved the oral bioavailability of BBR up to 2.4-fold by an anhydrous reverse micelle delivery system (Wang et al., 2011). Current clinical studies could not adequately address the issue of BBR bioavailability, and there is still a need to construct more drug formulations to address this challenge in the future, so as to increase the utilization and the application range of BBR.
5 Safety and toxicology
In general, BBR is virtually safe at routine doses, with low toxicity and side effects (Imenshahidi and Hosseinzadeh, 2016; Liu et al., 2016), such as only mild gastrointestinal reactions (diarrhea and constipation) reported in clinical studies (Zhang et al., 2010). On the one hand, BBR may prevent toxic reactions and side effects associated with some anti-tumor and analgesic drugs, such as cisplatin, cyclophosphamide, bleomycin, and acetaminophen (Chitra et al., 2013; Domitrovic et al., 2013a; Germoush and Mahmoud, 2014). On the other hand, in some cases, other adverse effects may occur. 10 mg/kg of BBR can suppress immune function in mice (Mahmoudi et al., 2016), and BBR interaction with macrolides and statins may lead to cardiac arrhythmias and reduce the efficacy of the drug (Feng P. et al., 2018; Holy et al., 2009; Zhi et al., 2015). Therefore, we conclude that BBR is safe for use in oral formulations based on traditional dosages and indications.
6 Discussion
Although a small number of drugs are available for the treatment of fibrosis, such as Nidanib and Pirfenidone, long drug cycles, adverse effects, and the lack of drugs targeting different organs remain a challenge to clinical treatment. Therefore, effective therapeutic strategies for fibrosis are urgently required. Natural products have become a hotspot for research and new drug development in recent years due to their advantages of multiple actions, targets and pathways, and more and more natural products have been reported with the utilization of various biological techniques. Studies over the past 2 decades have convincingly demonstrated the therapeutic effects of BBR on various types of fibrosis. The efficacy of BBR in the treatment of different types of fibrosis is mediated by its multi-target pharmacological profile, including the modulation of the TGF-β/Smad, inflammation, AMPK, and Nrf2 pathways, among others (Table 1). Although numerous studies have described the antifibrotic effects of BBR, most of them are based on cellular and animal studies, and comprehensive clinical studies are still lacking.
As mentioned earlier, the low bioavailability of BBR is the biggest hindrance to its practical application, and its preparation into modern oral dosage forms is essential, such as a mucoadhesive microparticle formulation and microemulsion formulation, as it reduces side effects and achieves better therapeutic effect (Feng X. et al., 2019). Besides, the development and advancement of various biological technologies can also solve this problem, such as an anhydrous reverse micelle delivery system, polymer materials and nanotechnology. Generally, BBR at routine doses is almost safe with low toxicity and side effects, the adverse effects of BBR under special circumstances may also limit the clinical application of BBR, so it needs to be used according to conventional dosage and indications.
Interestingly, despite the poor bioavailability of BBR, this does not explain why it is so effective in vivo. Growing research suggests that BBR may target the gut microbiota as a target for multifunctional action, exerting therapeutic effects by reversing the structure and value of the gut microbiota under pathological conditions (Feng X. et al., 2019). A study on beagle dogs showed that continuous oral administration of BBR for 7 days upregulated the levels of butyrate (a product of bacteria) and nitroreductases-producing bacteria in the plasma (Feng R. et al., 2018). Wang et al. (2017) found different bioavailability of BBR in obese and lean animals due to different structural distribution and values of microbiota. Alioga et al. (2016) studied the differential expression of gut microbiota between populations from different countries, emphasizing that the gut microbiota is closely related to inter-individual and inter-ethnic differences in drug metabolism. BBR is rapidly metabolized in vivo, and the gut microbiota enhances the bioactivity of BBR by converting it to dhBBR. Nevertheless, the effects of dhBBR treatment given alone differed from those described above, suggesting that the anti-fibrotic effect of BBR is not only due to its metabolites. Another study confirmed that the intestinal absorption of dhBBR in the body is 5-fold higher than that of BBR, and the absorbed dhBBR is oxidized to BBR and re-enters the bloodstream (Feng et al., 2015). There is also a study that explains this, where BBR undergoes extrahepatic metabolism and intestinal conversion to OBB, which are mainly transported intracellularly in a protein-bound form to form hepatocyte-targeted and enterohepatic circulations (Huang et al., 2023). We believe that the distribution of BBR and its bioactive metabolites in vivo may partially explain this.
7 Final considerations and prospect
Natural products are derivatives of traditional Chinese medicines and are the material basis for the pharmacological effects. They have the advantages of multiple actions, multiple targets and multiple pathways, and have become a hot spot for research and new drug development in recent years. BBR has attracted much attention due to its multiple pharmacological effects. Numerous studies on BBR affecting various types of fibrosis have found that its mechanisms of action include, but are not limited to the regulation of collagen homeostasis, anti-inflammation, anti-oxidative stress, and the prevention of tissue damage through different signaling cascades, and the mechanisms of action vary in different tissues or organs. We are only reviewing the completed studies with some limitations. Firstly, the bioavailability of BBR is insufficient, and the discovery of good drug carriers is important for improving its solubility, enhancing tissue targeting, and expanding the range of clinical applications. Secondly, further comprehensive studies, especially clinical trials, are urgently needed, which are essential for elucidating other mechanisms and molecular targets of BBR in the treatment of fibrosis as well as evaluating its efficacy and safety.
Author contributions
XL: Writing–original draft, Writing–review and editing. QL: Writing–original draft, Writing–review and editing. YW: Supervision, Writing–review and editing. SX: Writing–review and editing. RY: Conceptualization, Supervision, Writing–review and editing.
Funding
The author(s) declare that financial support was received for the research, authorship, and/or publication of this article. This work is supported by the Sichuan Provincial Cadre Healthcare Scientific Research Program (ZH 2024-501), Observations on the efficacy of Yin Huang Qing Re lotion in preventing incontinence dermatitis in elderly people (22HL18).
Conflict of interest
The authors declare that the research was conducted in the absence of any commercial or financial relationships that could be construed as a potential conflict of interest.
Publisher’s note
All claims expressed in this article are solely those of the authors and do not necessarily represent those of their affiliated organizations, or those of the publisher, the editors and the reviewers. Any product that may be evaluated in this article, or claim that may be made by its manufacturer, is not guaranteed or endorsed by the publisher.
References
Ahmedy, O. A., El-Tanbouly, D. M., Al-Mokaddem, A. K., and El-Said, Y. A. M. (2022). Insights into the role of P2X7R/DUSP6/ERK1/2 and SIRT2/MDM2 signaling in the nephroprotective effect of berberine against cisplatin-induced renal fibrosis in rats. Life Sci. 309, 121040. doi:10.1016/j.lfs.2022.121040
Ahmedy, O. A., Kamel, M. W., Abouelfadl, D. M., Shabana, M. E., and Sayed, R. H. (2023). Berberine attenuates epithelial mesenchymal transition in bleomycin-induced pulmonary fibrosis in mice via activating A(2a)R and mitigating the SDF-1/CXCR4 signaling. Life Sci. 322, 121665. doi:10.1016/j.lfs.2023.121665
Ai, F., Chen, M., Yu, B., Yang, Y., Xu, G., Gui, F., et al. (2015). Berberine regulates proliferation, collagen synthesis and cytokine secretion of cardiac fibroblasts via AMPK-mTOR-p70S6K signaling pathway. Int. J. Clin. Exp. Pathol. 8 (10), 12509–12516.
Ajoolabady, A., Kaplowitz, N., Lebeaupin, C., Kroemer, G., Kaufman, R. J., Malhi, H., et al. (2023). Endoplasmic reticulum stress in liver diseases. Hepatology 77 (2), 619–639. doi:10.1002/hep.32562
Alnuqaydan, A. M., Almutary, A. G., Azam, M., Manandhar, B., De Rubis, G., Madheswaran, T., et al. (2022). Phytantriol-based berberine-loaded liquid crystalline nanoparticles attenuate inflammation and oxidative stress in lipopolysaccharide-induced RAW264.7 macrophages. Nanomater. (Basel) 12 (23), 4312. doi:10.3390/nano12234312
Alolga, R. N., Fan, Y., Chen, Z., Liu, L. W., Zhao, Y. J., Li, J., et al. (2016). Significant pharmacokinetic differences of berberine are attributable to variations in gut microbiota between Africans and Chinese. Sci. Rep. 6, 27671. doi:10.1038/srep27671
An, J., Jiang, T., Qi, L., and Xie, K. (2023). Acinar cells and the development of pancreatic fibrosis. Cytokine Growth Factor Rev. 71-72, 40–53. doi:10.1016/j.cytogfr.2023.05.003
Arain, S. Q., Talpur, F. N., Channa, N. A., Ali, M. S., and Afridi, H. I. (2017). Serum lipid profile as a marker of liver impairment in hepatitis B Cirrhosis patients. Lipids Health Dis. 16 (1), 51. doi:10.1186/s12944-017-0437-2
Bansod, S., Doijad, N., and Godugu, C. (2020). Berberine attenuates severity of chronic pancreatitis and fibrosis via AMPK-mediated inhibition of TGF-β1/Smad signaling and M2 polarization. Toxicol. Appl. Pharmacol. 403, 115162. doi:10.1016/j.taap.2020.115162
Bansod, S., Saifi, M. A., and Godugu, C. (2021). Molecular updates on berberine in liver diseases: bench to bedside. Phytother. Res. 35 (10), 5459–5476. doi:10.1002/ptr.7181
Beik, A., Najafipour, H., Joukar, S., Rajabi, S., Masoumi-Ardakani, Y., Dabiri, S., et al. (2023). Beneficial effects of berberine against pulmonary complications of experimental pulmonary arterial hypertension in rats and some relevant mechanisms. Pulm. Circ. 13 (1), e12207. doi:10.1002/pul2.12207
Boudaba, N., Marion, A., Huet, C., Pierre, R., Viollet, B., and Foretz, M. (2018). AMPK Re-activation suppresses hepatic steatosis but its downregulation does not promote fatty liver development. EBioMedicine 28, 194–209. doi:10.1016/j.ebiom.2018.01.008
Boyer-Diaz, Z., Aristu-Zabalza, P., Andres-Rozas, M., Robert, C., Ortega-Ribera, M., Fernandez-Iglesias, A., et al. (2021). Pan-PPAR agonist lanifibranor improves portal hypertension and hepatic fibrosis in experimental advanced chronic liver disease. J. Hepatol. 74 (5), 1188–1199. doi:10.1016/j.jhep.2020.11.045
Brunner, K. T., Henneberg, C. J., Wilechansky, R. M., and Long, M. T. (2019). Nonalcoholic fatty liver disease and obesity treatment. Curr. Obes. Rep. 8 (3), 220–228. doi:10.1007/s13679-019-00345-1
Cai, Y., and Kang, Y. (2023). Gut microbiota and metabolites in diabetic retinopathy: insights into pathogenesis for novel therapeutic strategies. Biomed. Pharmacother. 164, 114994. doi:10.1016/j.biopha.2023.114994
Calissi, G., Lam, E. W., and Link, W. (2021). Therapeutic strategies targeting FOXO transcription factors. Nat. Rev. Drug Discov. 20 (1), 21–38. doi:10.1038/s41573-020-0088-2
Casaletto, J. B., and McClatchey, A. I. (2012). Spatial regulation of receptor tyrosine kinases in development and cancer. Nat. Rev. Cancer 12 (6), 387–400. doi:10.1038/nrc3277
Che, Y., Shen, D. F., Wang, Z. P., Jin, Y. G., Wu, Q. Q., Wang, S. S., et al. (2019). Protective role of berberine in isoprenaline-induced cardiac fibrosis in rats. BMC Cardiovasc Disord. 19 (1), 219. doi:10.1186/s12872-019-1198-9
Che, Z., Zhou, Z., Li, S. Q., Gao, L., Xiao, J., and Wong, N. K. (2023). ROS/RNS as molecular signatures of chronic liver diseases. Trends Mol. Med. 29 (11), 951–967. doi:10.1016/j.molmed.2023.08.001
Chen, H., Tan, H., Wan, J., Zeng, Y., Wang, J., Wang, H., et al. (2023). PPAR-gamma signaling in nonalcoholic fatty liver disease: pathogenesis and therapeutic targets. Pharmacol. Ther. 245, 108391. doi:10.1016/j.pharmthera.2023.108391
Chen, W., Miao, Y. Q., Fan, D. J., Yang, S. S., Lin, X., Meng, L. K., et al. (2011). Bioavailability study of berberine and the enhancing effects of TPGS on intestinal absorption in rats. AAPS PharmSciTech 12 (2), 705–711. doi:10.1208/s12249-011-9632-z
Chen, X., Jiang, X., Cheng, C., Chen, J., Huang, S., Xu, M., et al. (2020). Berberine attenuates cardiac hypertrophy through inhibition of mTOR signaling pathway. Cardiovasc Drugs Ther. 34 (4), 463–473. doi:10.1007/s10557-020-06977-z
Chitra, P., Saiprasad, G., Manikandan, R., and Sudhandiran, G. (2013). Berberine attenuates bleomycin induced pulmonary toxicity and fibrosis via suppressing NF-κB dependant TGF-β activation: a biphasic experimental study. Toxicol. Lett. 219 (2), 178–193. doi:10.1016/j.toxlet.2013.03.009
Dang, Y., An, Y., He, J., Huang, B., Zhu, J., Gao, M., et al. (2020). Berberine ameliorates cellular senescence and extends the lifespan of mice via regulating p16 and cyclin protein expression. Aging Cell 19 (1), e13060. doi:10.1111/acel.13060
da Silva Morais, A., Abarca-Quinones, J., Guigas, B., Viollet, B., Starkel, P., Horsmans, Y., et al. (2009). Development of hepatic fibrosis occurs normally in AMPK-deficient mice. Clin. Sci. (Lond). 118 (6), 411–420. doi:10.1042/CS20090293
de Carvalho Ribeiro, M., and Szabo, G. (2022). Role of the inflammasome in liver disease. Annu. Rev. Pathol. 17, 345–365. doi:10.1146/annurev-pathmechdis-032521-102529
Derosa, G., D'Angelo, A., Bonaventura, A., Bianchi, L., Romano, D., and Maffioli, P. (2013). Effects of berberine on lipid profile in subjects with low cardiovascular risk. Expert Opin. Biol. Ther. 13 (4), 475–482. doi:10.1517/14712598.2013.776037
Domitrovic, R., Cvijanovic, O., Pernjak-Pugel, E., Skoda, M., Mikelic, L., and Crncevic-Orlic, Z. (2013a). Berberine exerts nephroprotective effect against cisplatin-induced kidney damage through inhibition of oxidative/nitrosative stress, inflammation, autophagy and apoptosis. Food Chem. Toxicol. 62, 397–406. doi:10.1016/j.fct.2013.09.003
Domitrovic, R., Jakovac, H., Marchesi, V. V., and Blazekovic, B. (2013b). Resolution of liver fibrosis by isoquinoline alkaloid berberine in CCl₄-intoxicated mice is mediated by suppression of oxidative stress and upregulation of MMP-2 expression. J. Med. Food 16 (6), 518–528. doi:10.1089/jmf.2012.0175
Dong, J., and Ma, Q. (2016). Myofibroblasts and lung fibrosis induced by carbon nanotube exposure. Part Fibre Toxicol. 13 (1), 60. doi:10.1186/s12989-016-0172-2
Dou, Y., Huang, R., Li, Q., Liu, Y., Li, Y., Chen, H., et al. (2021). Oxyberberine, an absorbed metabolite of berberine, possess superior hypoglycemic effect via regulating the PI3K/Akt and Nrf2 signaling pathways. Biomed. Pharmacother. 137, 111312. doi:10.1016/j.biopha.2021.111312
Eming, S. A., Wynn, T. A., and Martin, P. (2017). Inflammation and metabolism in tissue repair and regeneration. Science 356 (6342), 1026–1030. doi:10.1126/science.aam7928
Feng, A. W., Gao, W., Zhou, G. R., Yu, R., Li, N., Huang, X. L., et al. (2012). Berberine ameliorates COX-2 expression in rat small intestinal mucosa partially through PPARγ pathway during acute endotoxemia. Int. Immunopharmacol. 12 (1), 182–188. doi:10.1016/j.intimp.2011.11.009
Feng, F., Cheng, P., Zhang, H., Li, N., Qi, Y., Wang, H., et al. (2019a). The protective role of tanshinone IIA in silicosis rat model via TGF-β1/smad signaling suppression, NOX4 inhibition and Nrf2/ARE signaling activation. Drug Des. Devel Ther. 13, 4275–4290. doi:10.2147/DDDT.S230572
Feng, P., Zhao, L., Guo, F., Zhang, B., Fang, L., Zhan, G., et al. (2018a). The enhancement of cardiotoxicity that results from inhibiton of CYP 3A4 activity and hERG channel by berberine in combination with statins. Chem. Biol. Interact. 293, 115–123. doi:10.1016/j.cbi.2018.07.022
Feng, R., Shou, J. W., Zhao, Z. X., He, C. Y., Ma, C., Huang, M., et al. (2015). Transforming berberine into its intestine-absorbable form by the gut microbiota. Sci. Rep. 5, 12155. doi:10.1038/srep12155
Feng, R., Zhao, Z. X., Ma, S. R., Guo, F., Wang, Y., and Jiang, J. D. (2018b). Gut microbiota-regulated pharmacokinetics of berberine and active metabolites in beagle dogs after oral administration. Front. Pharmacol. 9, 214. doi:10.3389/fphar.2018.00214
Feng, X., Sureda, A., Jafari, S., Memariani, Z., Tewari, D., Annunziata, G., et al. (2019b). Berberine in cardiovascular and metabolic diseases: from mechanisms to therapeutics. Theranostics 9 (7), 1923–1951. doi:10.7150/thno.30787
Frangogiannis, N. (2020). Transforming growth factor-β in tissue fibrosis. J. Exp. Med. 217 (3), e20190103. doi:10.1084/jem.20190103
Frangogiannis, N. G. (2021). Cardiac fibrosis. Cardiovasc Res. 117 (6), 1450–1488. doi:10.1093/cvr/cvaa324
Friedman, S. L., Sheppard, D., Duffield, J. S., and Violette, S. (2013). Therapy for fibrotic diseases: nearing the starting line. Sci. Transl. Med. 5 (167), 167sr1. doi:10.1126/scitranslmed.3004700
Gao, R., Lu, Y., Zhang, W., and Zhang, Z. (2024). The application of berberine in fibrosis and the related diseases. Am. J. Chin. Med. 52 (3), 753–773. doi:10.1142/S0192415X24500307
Germoush, M. O., and Mahmoud, A. M. (2014). Berberine mitigates cyclophosphamide-induced hepatotoxicity by modulating antioxidant status and inflammatory cytokines. J. Cancer Res. Clin. Oncol. 140 (7), 1103–1109. doi:10.1007/s00432-014-1665-8
Godugu, C., Patel, A. R., Doddapaneni, R., Somagoni, J., and Singh, M. (2014). Approaches to improve the oral bioavailability and effects of novel anticancer drugs berberine and betulinic acid. PLoS One 9 (3), e89919. doi:10.1371/journal.pone.0089919
Gorgulu, A., Simsek, O., Cobanoglu, S., Imer, M., and Parsak, T. (2004). The effect of epidural free fat graft on the outcome of lumbar disc surgery. Neurosurg. Rev. 27 (3), 181–184. doi:10.1007/s10143-003-0310-9
Grabner, G. F., Xie, H., Schweiger, M., and Zechner, R. (2021). Lipolysis: cellular mechanisms for lipid mobilization from fat stores. Nat. Metab. 3 (11), 1445–1465. doi:10.1038/s42255-021-00493-6
Guan, C., Qiao, S., Lv, Q., Cao, N., Wang, K., Dai, Y., et al. (2018). Orally administered berberine ameliorates bleomycin-induced pulmonary fibrosis in mice through promoting activation of PPAR-gamma and subsequent expression of HGF in colons. Toxicol. Appl. Pharmacol. 343, 1–15. doi:10.1016/j.taap.2018.02.001
Gui, S. Y., Wu, L., Peng, D. Y., Liu, Q. Y., Yin, B. P., and Shen, J. Z. (2008). Preparation and evaluation of a microemulsion for oral delivery of berberine. Pharmazie 63 (7), 516–519.
Guo, Y., Wang, Q. Z., Li, F. M., Jiang, X., Zuo, Y. F., and Wang, L. (2008). Biochemical pathways in the antiatherosclerotic effect of berberine. Chin. Med. J. Engl. 121 (13), 1197–1203. doi:10.1097/00029330-200807010-00009
Habtemariam, S. (2020). Berberine pharmacology and the gut microbiota: a hidden therapeutic link. Pharmacol. Res. 155, 104722. doi:10.1016/j.phrs.2020.104722
Hardie, D. G., Ross, F. A., and Hawley, S. A. (2012). AMPK: a nutrient and energy sensor that maintains energy homeostasis. Nat. Rev. Mol. Cell Biol. 13 (4), 251–262. doi:10.1038/nrm3311
Hassanein, E. H. M., Ibrahim, I. M., Abd-Alhameed, E. K., Mohamed, N. M., and Ross, S. A. (2022). Protective effects of berberine on various kidney diseases: emphasis on the promising effects and the underlined molecular mechanisms. Life Sci. 306, 120697. doi:10.1016/j.lfs.2022.120697
Henderson, N. C., Rieder, F., and Wynn, T. A. (2020). Fibrosis: from mechanisms to medicines. Nature 587 (7835), 555–566. doi:10.1038/s41586-020-2938-9
Higashiyama, H., Yoshimoto, D., Okamoto, Y., Kikkawa, H., Asano, S., and Kinoshita, M. (2007). Receptor-activated Smad localisation in bleomycin-induced pulmonary fibrosis. J. Clin. Pathol. 60 (3), 283–289. doi:10.1136/jcp.2006.037606
Holy, E. W., Akhmedov, A., Luscher, T. F., and Tanner, F. C. (2009). Berberine, a natural lipid-lowering drug, exerts prothrombotic effects on vascular cells. J. Mol. Cell Cardiol. 46 (2), 234–240. doi:10.1016/j.yjmcc.2008.10.011
Hu, M., Wu, F., Luo, J., Gong, J., Fang, K., Yang, X., et al. (2018). The role of berberine in the prevention of HIF-1α activation to alleviate adipose tissue fibrosis in high-fat-diet-induced obese mice. Evid. Based Complement. Altern. Med. 2018, 4395137. doi:10.1155/2018/4395137
Hua, W., Ding, L., Chen, Y., Gong, B., He, J., and Xu, G. (2007). Determination of berberine in human plasma by liquid chromatography-electrospray ionization-mass spectrometry. J. Pharm. Biomed. Anal. 44 (4), 931–937. doi:10.1016/j.jpba.2007.03.022
Huang, R., Guo, F., Li, Y., Liang, Y., Li, G., Fu, P., et al. (2021). Activation of AMPK by triptolide alleviates nonalcoholic fatty liver disease by improving hepatic lipid metabolism, inflammation and fibrosis. Phytomedicine 92, 153739. doi:10.1016/j.phymed.2021.153739
Huang, Z., Li, M., Qin, Z., Ma, X., Huang, R., Liu, Y., et al. (2023). Intestines-erythrocytes-mediated bio-disposition deciphers the hypolipidemic effect of berberine from Rhizoma Coptidis: a neglected insight. J. Ethnopharmacol. 314, 116600. doi:10.1016/j.jep.2023.116600
Ibrahim Fouad, G., and Ahmed, K. A. (2021). The protective impact of berberine against doxorubicin-induced nephrotoxicity in rats. Tissue Cell 73, 101612. doi:10.1016/j.tice.2021.101612
Imenshahidi, M., and Hosseinzadeh, H. (2016). Berberis vulgaris and berberine: an update review. Phytother. Res. 30 (11), 1745–1764. doi:10.1002/ptr.5693
Inagaki, Y., and Okazaki, I. (2007). Emerging insights into Transforming growth factor beta Smad signal in hepatic fibrogenesis. Gut 56 (2), 284–292. doi:10.1136/gut.2005.088690
Jiang, X. P., Ai, W. B., Wan, L. Y., Zhang, Y. Q., and Wu, J. F. (2017). The roles of microRNA families in hepatic fibrosis. Cell Biosci. 7, 34. doi:10.1186/s13578-017-0161-7
Keskin, E., Tonge, C., Kaya, M., and Isik, E. (2022). Evaluation of the effects of berberine in the prevention of epidural fibrosis in rats: an experimental research. Saudi Med. J. 43 (4), 370–377. doi:10.15537/smj.2022.43.4.20210918
Kobayashi, E. H., Suzuki, T., Funayama, R., Nagashima, T., Hayashi, M., Sekine, H., et al. (2016). Nrf2 suppresses macrophage inflammatory response by blocking proinflammatory cytokine transcription. Nat. Commun. 7, 11624. doi:10.1038/ncomms11624
Koenen, M., Hill, M. A., Cohen, P., and Sowers, J. R. (2021). Obesity, adipose tissue and vascular dysfunction. Circ. Res. 128 (7), 951–968. doi:10.1161/CIRCRESAHA.121.318093
Kong, W., Wei, J., Abidi, P., Lin, M., Inaba, S., Li, C., et al. (2004). Berberine is a novel cholesterol-lowering drug working through a unique mechanism distinct from statins. Nat. Med. 10 (12), 1344–1351. doi:10.1038/nm1135
Kong, W. J., Vernieri, C., Foiani, M., and Jiang, J. D. (2020). Berberine in the treatment of metabolism-related chronic diseases: a drug cloud (dCloud) effect to target multifactorial disorders. Pharmacol. Ther. 209, 107496. doi:10.1016/j.pharmthera.2020.107496
Kong, X., Horiguchi, N., Mori, M., and Gao, B. (2012). Cytokines and STATs in liver fibrosis. Front. Physiol. 3, 69. doi:10.3389/fphys.2012.00069
Koo, J. H., Lee, H. J., Kim, W., and Kim, S. G. (2016). Endoplasmic reticulum stress in hepatic stellate cells promotes liver fibrosis via PERK-mediated degradation of HNRNPA1 and up-regulation of SMAD2. Gastroenterology 150 (1), 181–193. doi:10.1053/j.gastro.2015.09.039
Kumar, A., Chopra, K., Mukherjee, M., Pottabathini, R., and Dhull, D. K. (2015). Current knowledge and pharmacological profile of berberine: an update. Eur. J. Pharmacol. 761, 288–297. doi:10.1016/j.ejphar.2015.05.068
Li, D., Yang, C., Zhu, J. Z., Lopez, E., Zhang, T., Tong, Q., et al. (2022). Berberine remodels adipose tissue to attenuate metabolic disorders by activating sirtuin 3. Acta Pharmacol. Sin. 43 (5), 1285–1298. doi:10.1038/s41401-021-00736-y
Li, J., Pan, Y., Kan, M., Xiao, X., Wang, Y., Guan, F., et al. (2014). Hepatoprotective effects of berberine on liver fibrosis via activation of AMP-activated protein kinase. Life Sci. 98 (1), 24–30. doi:10.1016/j.lfs.2013.12.211
Li, L., Li, Q., Wei, L., Wang, Z., Ma, W., Liu, F., et al. (2019). Dexamethasone combined with berberine is an effective therapy for bleomycin-induced pulmonary fibrosis in rats. Exp. Ther. Med. 18 (4), 2385–2392. doi:10.3892/etm.2019.7861
Li, L., Zeng, H., Shan, L., Yuan, X., Li, Y., Liu, R., et al. (2012). The different inhibitory effects of Huang-Lian-Jie-Du-Tang on cyclooxygenase 2 and 5-lipoxygenase. J. Ethnopharmacol. 143 (2), 732–739. doi:10.1016/j.jep.2012.07.037
Li, N., Yamamoto, G., Fuji, H., and Kisseleva, T. (2021a). Interleukin-17 in liver disease pathogenesis. Semin. Liver Dis. 41 (4), 507–515. doi:10.1055/s-0041-1730926
Li, Q. P., Dou, Y. X., Huang, Z. W., Chen, H. B., Li, Y. C., Chen, J. N., et al. (2021b). Therapeutic effect of oxyberberine on obese non-alcoholic fatty liver disease rats. Phytomedicine 85, 153550. doi:10.1016/j.phymed.2021.153550
Li, X. X., Li, N., Ban, C. J., Zhu, M., Xiao, B., and Dai, H. P. (2011). Idiopathic pulmonary fibrosis in relation to gene polymorphisms of transforming growth factor-β1 and plasminogen activator inhibitor 1. Chin. Med. J. Engl. 124 (13), 1923–1927.
Li, Z., and Zhang, W. (2017). Protective effect of berberine on renal fibrosis caused by diabetic nephropathy. Mol. Med. Rep. 16 (2), 1055–1062. doi:10.3892/mmr.2017.6707
Liu, C. S., Zheng, Y. R., Zhang, Y. F., and Long, X. Y. (2016). Research progress on berberine with a special focus on its oral bioavailability. Fitoterapia 109, 274–282. doi:10.1016/j.fitote.2016.02.001
Liu, M., Lopez de Juan Abad, B., and Cheng, K. (2021). Cardiac fibrosis: myofibroblast-mediated pathological regulation and drug delivery strategies. Adv. Drug Deliv. Rev. 173, 504–519. doi:10.1016/j.addr.2021.03.021
Liu, X., Wang, L., Tan, S., Chen, Z., Wu, B., and Wu, X. (2022). Therapeutic effects of berberine on liver fibrosis are associated with lipid metabolism and intestinal flora. Front. Pharmacol. 13, 814871. doi:10.3389/fphar.2022.814871
Liu, X., Wang, Y., Shao, P., Chen, Y., Yang, C., Wang, J., et al. (2024). Sargentodoxa cuneata and Patrinia villosa extract inhibits LPS-induced inflammation by shifting macrophages polarization through FAK/PI3K/Akt pathway regulation and glucose metabolism reprogramming. J. Ethnopharmacol. 318 (Pt A), 116855. doi:10.1016/j.jep.2023.116855
Liu, Y. T., Hao, H. P., Xie, H. G., Lai, L., Wang, Q., Liu, C. X., et al. (2010). Extensive intestinal first-pass elimination and predominant hepatic distribution of berberine explain its low plasma levels in rats. Drug Metab. Dispos. 38 (10), 1779–1784. doi:10.1124/dmd.110.033936
Luo, R., Liao, Z., Song, Y., Yin, H., Zhan, S., Li, G., et al. (2019). Berberine ameliorates oxidative stress-induced apoptosis by modulating ER stress and autophagy in human nucleus pulposus cells. Life Sci. 228, 85–97. doi:10.1016/j.lfs.2019.04.064
Ma, H. Y., Yamamoto, G., Xu, J., Liu, X., Karin, D., Kim, J. Y., et al. (2020). IL-17 signaling in steatotic hepatocytes and macrophages promotes hepatocellular carcinoma in alcohol-related liver disease. J. Hepatol. 72 (5), 946–959. doi:10.1016/j.jhep.2019.12.016
Ma, Z., Chen, X., Xiong, M., Wang, H., Sun, C., Tang, W., et al. (2024). Cyberpharmacology uncover the mechanism of the total Rhizoma Coptidis extracts ameliorate chronic atrophic gastritis. J. Ethnopharmacol. 335, 118644. doi:10.1016/j.jep.2024.118644
Mahmoudi, M., Zamani Taghizadeh Rabe, S., Balali-Mood, M., Karimi, G., Memar, B., Rahnama, M., et al. (2016). Immunotoxicity induced in mice by subacute exposure to berberine. J. Immunotoxicol. 13 (2), 255–262. doi:10.3109/1547691X.2015.1058306
Maiers, J. L., Kostallari, E., Mushref, M., deAssuncao, T. M., Li, H., Jalan-Sakrikar, N., et al. (2017). The unfolded protein response mediates fibrogenesis and collagen I secretion through regulating TANGO1 in mice. Hepatology 65 (3), 983–998. doi:10.1002/hep.28921
Marcelin, G., Da Cunha, C., Gamblin, C., Suffee, N., Rouault, C., Leclerc, A., et al. (2020). Autophagy inhibition blunts PDGFRA adipose progenitors' cell-autonomous fibrogenic response to high-fat diet. Autophagy 16 (12), 2156–2166. doi:10.1080/15548627.2020.1717129
Masamune, A., Watanabe, T., Kikuta, K., and Shimosegawa, T. (2009). Roles of pancreatic stellate cells in pancreatic inflammation and fibrosis. Clin. Gastroenterol. Hepatol. 7 (11 Suppl. l), S48–S54. doi:10.1016/j.cgh.2009.07.038
Meng, F., Wang, K., Aoyama, T., Grivennikov, S. I., Paik, Y., Scholten, D., et al. (2012). Interleukin-17 signaling in inflammatory, Kupffer cells, and hepatic stellate cells exacerbates liver fibrosis in mice. Gastroenterology 143 (3), 765–776. doi:10.1053/j.gastro.2012.05.049
Meng, X. M., Nikolic-Paterson, D. J., and Lan, H. Y. (2016). TGF-β: the master regulator of fibrosis. Nat. Rev. Nephrol. 12 (6), 325–338. doi:10.1038/nrneph.2016.48
Mishra, S. P., Jain, S., Taraphder, S., and Yadav, H. (2021). New horizons in microbiota and metabolic health research. J. Clin. Endocrinol. Metab. 106 (2), e1052–e1059. doi:10.1210/clinem/dgaa769
Nicholson, J. K., Holmes, E., Kinross, J., Burcelin, R., Gibson, G., Jia, W., et al. (2012). Host-gut microbiota metabolic interactions. Science 336 (6086), 1262–1267. doi:10.1126/science.1223813
Palanivel, C., Gowrikumar, S., Ramar, M., and Ganapasam, S. (2015). Berberine inhibits Smad and non-Smad signaling cascades and enhances autophagy against pulmonary fibrosis. J. Mol. Med. Berl. 93 (9), 1015–1031. doi:10.1007/s00109-015-1283-1
Passino, M. A., Adams, R. A., Sikorski, S. L., and Akassoglou, K. (2007). Regulation of hepatic stellate cell differentiation by the neurotrophin receptor p75NTR. Science 315 (5820), 1853–1856. doi:10.1126/science.1137603
Perez-Carreon, J. I., Martinez-Perez, L., Loredo, M. L., Yanez-Maldonado, L., Velasco-Loyden, G., Vidrio-Gomez, S., et al. (2010). An adenosine derivative compound, IFC305, reverses fibrosis and alters gene expression in a pre-established CCl(4)-induced rat cirrhosis. Int. J. Biochem. Cell Biol. 42 (2), 287–296. doi:10.1016/j.biocel.2009.11.005
Pietra, D., Borghini, A., and Bianucci, A. M. (2015). In vitro studies of antifibrotic and cytoprotective effects elicited by proto-berberine alkaloids in human dermal fibroblasts. Pharmacol. Rep. 67 (6), 1081–1089. doi:10.1016/j.pharep.2015.04.001
Rafiei, H., Yeung, M., Kowalski, S., Krystal, G., and Elisia, I. (2023). Development of a novel human triculture model of non-alcoholic fatty liver disease and identification of berberine as ameliorating steatosis, oxidative stress and fibrosis. Front. Pharmacol. 14, 1234300. doi:10.3389/fphar.2023.1234300
Roy, S. K., Chen, Q., Fu, J., Shankar, S., and Srivastava, R. K. (2011). Resveratrol inhibits growth of orthotopic pancreatic tumors through activation of FOXO transcription factors. PLoS One 6 (9), e25166. doi:10.1371/journal.pone.0025166
Sanchez-Valle, V., Chavez-Tapia, N. C., Uribe, M., and Mendez-Sanchez, N. (2012). Role of oxidative stress and molecular changes in liver fibrosis: a review. Curr. Med. Chem. 19 (28), 4850–4860. doi:10.2174/092986712803341520
Sanyal, A. J., Chalasani, N., Kowdley, K. V., McCullough, A., Diehl, A. M., Bass, N. M., et al. (2010). Pioglitazone, vitamin E, or placebo for nonalcoholic steatohepatitis. N. Engl. J. Med. 362 (18), 1675–1685. doi:10.1056/NEJMoa0907929
Saxena, N. K., and Anania, F. A. (2015). Adipocytokines and hepatic fibrosis. Trends Endocrinol. Metab. 26 (3), 153–161. doi:10.1016/j.tem.2015.01.002
Schmiedel, J. M., Klemm, S. L., Zheng, Y., Sahay, A., Bluthgen, N., Marks, D. S., et al. (2015). Gene expression. MicroRNA control of protein expression noise. Science 348 (6230), 128–132. doi:10.1126/science.aaa1738
Scorletti, E., and Carr, R. M. (2022). A new perspective on NAFLD: focusing on lipid droplets. J. Hepatol. 76 (4), 934–945. doi:10.1016/j.jhep.2021.11.009
Seo, W., Eun, H. S., Kim, S. Y., Yi, H. S., Lee, Y. S., Park, S. H., et al. (2016). Exosome-mediated activation of toll-like receptor 3 in stellate cells stimulates interleukin-17 production by γδ T cells in liver fibrosis. Hepatology 64 (2), 616–631. doi:10.1002/hep.28644
Shang, G. K., Han, L., Wang, Z. H., Liu, Y. P., Yan, S. B., Sai, W. W., et al. (2020). Sarcopenia is attenuated by TRB3 knockout in aging mice via the alleviation of atrophy and fibrosis of skeletal muscles. J. Cachexia Sarcopenia Muscle 11 (4), 1104–1120. doi:10.1002/jcsm.12560
Shao, M., Ye, C., Bayliss, G., and Zhuang, S. (2021). New insights into the effects of individual Chinese herbal medicines on chronic kidney disease. Front. Pharmacol. 12, 774414. doi:10.3389/fphar.2021.774414
Singh, I. P., and Mahajan, S. (2013). Berberine and its derivatives: a patent review (2009 - 2012). Expert Opin. Ther. Pat. 23 (2), 215–231. doi:10.1517/13543776.2013.746314
Song, L., Zhang, W., Tang, S. Y., Luo, S. M., Xiong, P. Y., Liu, J. Y., et al. (2024). Natural products in traditional Chinese medicine: molecular mechanisms and therapeutic targets of renal fibrosis and state-of-the-art drug delivery systems. Biomed. Pharmacother. 170, 116039. doi:10.1016/j.biopha.2023.116039
Sun, K., Kusminski, C. M., and Scherer, P. E. (2011). Adipose tissue remodeling and obesity. J. Clin. Invest 121 (6), 2094–2101. doi:10.1172/JCI45887
Sun, X., Zhang, X., Hu, H., Lu, Y., Chen, J., Yasuda, K., et al. (2009). Berberine inhibits hepatic stellate cell proliferation and prevents experimental liver fibrosis. Biol. Pharm. Bull. 32 (9), 1533–1537. doi:10.1248/bpb.32.1533
Sun, Y., Huang, H., Zhan, Z., Gao, H., Zhang, C., Lai, J., et al. (2022). Berberine inhibits glioma cell migration and invasion by suppressing TGF-β1/COL11A1 pathway. Biochem. Biophys. Res. Commun. 625, 38–45. doi:10.1016/j.bbrc.2022.07.101
Sun, Y., Zhou, Q., Chen, F., Gao, X., Yang, L., Jin, X., et al. (2023). Berberine inhibits breast carcinoma proliferation and metastasis under hypoxic microenvironment involving gut microbiota and endogenous metabolites. Pharmacol. Res. 193, 106817. doi:10.1016/j.phrs.2023.106817
Tacke, F., and Weiskirchen, R. (2012). Update on hepatic stellate cells: pathogenic role in liver fibrosis and novel isolation techniques. Expert Rev. Gastroenterol. Hepatol. 6 (1), 67–80. doi:10.1586/egh.11.92
Tan, E., Gao, Z., Wang, Q., Han, B., Shi, H., Wang, L., et al. (2023a). Berberine ameliorates renal interstitial inflammation and fibrosis in mice with unilateral ureteral obstruction. Basic Clin. Pharmacol. Toxicol. 133 (6), 757–769. doi:10.1111/bcpt.13947
Tan, H. L., Chan, K. G., Pusparajah, P., Duangjai, A., Saokaew, S., Mehmood Khan, T., et al. (2016). Rhizoma coptidis: a potential cardiovascular protective agent. Front. Pharmacol. 7, 362. doi:10.3389/fphar.2016.00362
Tan, X. S., Ma, J. Y., Feng, R., Ma, C., Chen, W. J., Sun, Y. P., et al. (2013). Tissue distribution of berberine and its metabolites after oral administration in rats. PLoS One 8 (10), e77969. doi:10.1371/journal.pone.0077969
Tan, Y., Li, C., Zhou, J., Deng, F., and Liu, Y. (2023b). Berberine attenuates liver fibrosis by autophagy inhibition triggering apoptosis via the miR-30a-5p/ATG5 axis. Exp. Cell Res. 427 (2), 113600. doi:10.1016/j.yexcr.2023.113600
Tew, X. N., Xin Lau, N. J., Chellappan, D. K., Madheswaran, T., Zeeshan, F., Tambuwala, M. M., et al. (2020). Immunological axis of berberine in managing inflammation underlying chronic respiratory inflammatory diseases. Chem. Biol. Interact. 317, 108947. doi:10.1016/j.cbi.2020.108947
Thoen, L. F., Guimaraes, E. L., Dolle, L., Mannaerts, I., Najimi, M., Sokal, E., et al. (2011). A role for autophagy during hepatic stellate cell activation. J. Hepatol. 55 (6), 1353–1360. doi:10.1016/j.jhep.2011.07.010
Thursby, E., and Juge, N. (2017). Introduction to the human gut microbiota. Biochem. J. 474 (11), 1823–1836. doi:10.1042/BCJ20160510
Wang, B., Wang, L., Wang, H., Dai, H., Lu, X., Lee, Y. K., et al. (2021). Targeting the gut microbiota for remediating obesity and related metabolic disorders. J. Nutr. 151 (7), 1703–1716. doi:10.1093/jn/nxab103
Wang, B., Yang, H., Fan, Y., Yang, Y., Cao, W., Jia, Y., et al. (2017a). 3-Methyladenine ameliorates liver fibrosis through autophagy regulated by the NF-κB signaling pathways on hepatic stellate cell. Oncotarget 8 (64), 107603–107611. doi:10.18632/oncotarget.22539
Wang, F. M., Yang, Y. J., Ma, L. L., Tian, X. J., and He, Y. Q. (2014). Berberine ameliorates renal interstitial fibrosis induced by unilateral ureteral obstruction in rats. Nephrol. Carlt. 19 (9), 542–551. doi:10.1111/nep.12271
Wang, L., Ye, X., Hua, Y., and Song, Y. (2018). Berberine alleviates adipose tissue fibrosis by inducing AMP-activated kinase signaling in high-fat diet-induced obese mice. Biomed. Pharmacother. 105, 121–129. doi:10.1016/j.biopha.2018.05.110
Wang, N., Xu, Q., Tan, H. Y., Hong, M., Li, S., Yuen, M. F., et al. (2016). Berberine inhibition of fibrogenesis in a rat model of liver fibrosis and in hepatic stellate cells. Evid. Based Complement. Altern. Med. 2016, 8762345. doi:10.1155/2016/8762345
Wang, T., Wang, N., Song, H., Xi, X., Wang, J., Hao, A., et al. (2011). Preparation of an anhydrous reverse micelle delivery system to enhance oral bioavailability and anti-diabetic efficacy of berberine. Eur. J. Pharm. Sci. 44 (1-2), 127–135. doi:10.1016/j.ejps.2011.06.015
Wang, X., Wang, R., Xing, D., Su, H., Ma, C., Ding, Y., et al. (2005). Kinetic difference of berberine between hippocampus and plasma in rat after intravenous administration of Coptidis rhizoma extract. Life Sci. 77 (24), 3058–3067. doi:10.1016/j.lfs.2005.02.033
Wang, Y., Han, Y., Shang, K., Xiao, J., Tao, L., Peng, Z., et al. (2024a). Kokusaginine attenuates renal fibrosis by inhibiting the PI3K/AKT signaling pathway. Biomed. Pharmacother. 175, 116695. doi:10.1016/j.biopha.2024.116695
Wang, Y., Liao, J., Luo, Y., Li, M., Su, X., Yu, B., et al. (2023). Berberine alleviates doxorubicin-induced myocardial injury and fibrosis by eliminating oxidative stress and mitochondrial damage via promoting nrf-2 pathway activation. Int. J. Mol. Sci. 24 (4), 3257. doi:10.3390/ijms24043257
Wang, Y., Tong, Q., Shou, J. W., Zhao, Z. X., Li, X. Y., Zhang, X. F., et al. (2017b). Gut microbiota-mediated personalized treatment of hyperlipidemia using berberine. Theranostics 7 (9), 2443–2451. doi:10.7150/thno.18290
Wang, Y., Wu, G. R., Yue, H., Zhou, Q., Zhang, L., He, L., et al. (2024b). Kynurenine acts as a signaling molecule to attenuate pulmonary fibrosis by enhancing the AHR-PTEN axis. J. Adv. Res. doi:10.1016/j.jare.2024.06.017
Wang, Y., and Zidichouski, J. A. (2018). Update on the benefits and mechanisms of action of the bioactive vegetal alkaloid berberine on lipid metabolism and homeostasis. Cholesterol 2018, 7173920. doi:10.1155/2018/7173920
Wei, X., Wang, C., Hao, S., Song, H., and Yang, L. (2016). The therapeutic effect of berberine in the treatment of nonalcoholic fatty liver disease: a meta-analysis. Evid. Based Complement. Altern. Med. 2016, 3593951. doi:10.1155/2016/3593951
Wynn, T. A. (2011). Integrating mechanisms of pulmonary fibrosis. J. Exp. Med. 208 (7), 1339–1350. doi:10.1084/jem.20110551
Wynn, T. A., and Ramalingam, T. R. (2012). Mechanisms of fibrosis: therapeutic translation for fibrotic disease. Nat. Med. 18 (7), 1028–1040. doi:10.1038/nm.2807
Xia, Z. N., Lin, Y. X., Guo, L. X., Yang, F. Q., Xu, P., Zhang, Y. L., et al. (2013). Development of a cell-based high-throughput peroxisome proliferator-activated receptors (PPARs) screening model and its application for evaluation of the extracts from Rhizoma Coptis. J. Asian Nat. Prod. Res. 15 (3), 225–234. doi:10.1080/10286020.2012.761977
Xiao, Y., Liu, Y., Lai, Z., Huang, J., Li, C., Zhang, Y., et al. (2021). An integrated network pharmacology and transcriptomic method to explore the mechanism of the total Rhizoma Coptidis alkaloids in improving diabetic nephropathy. J. Ethnopharmacol. 270, 113806. doi:10.1016/j.jep.2021.113806
Xie, Z., Zhou, Y., Lin, M., and Huang, C. (2023). Binding of berberine to PEBP1 synergizes with sorafenib to induce the ferroptosis of hepatic stellate cells. Amino Acids 55 (12), 1867–1878. doi:10.1007/s00726-023-03345-7
Xu, L., Lin, G., Yu, Q., Li, Q., Mai, L., Cheng, J., et al. (2021a). Anti-hyperuricemic and nephroprotective effects of dihydroberberine in potassium oxonate- and hypoxanthine-induced hyperuricemic mice. Front. Pharmacol. 12, 645879. doi:10.3389/fphar.2021.645879
Xu, X., Yi, H., Wu, J., Kuang, T., Zhang, J., Li, Q., et al. (2021b). Therapeutic effect of berberine on metabolic diseases: both pharmacological data and clinical evidence. Biomed. Pharmacother. 133, 110984. doi:10.1016/j.biopha.2020.110984
Xu, Y., Chen, J., Jiang, W., Zhao, Y., Yang, C., Wu, Y., et al. (2022). Multiplexing nanodrug ameliorates liver fibrosis via ROS elimination and inflammation suppression. Small 18 (3), e2102848. doi:10.1002/smll.202102848
Xuan, Y., Chen, S., Ding, X., Wang, L., Li, S., Yang, G., et al. (2021). Tetrahydropalmatine attenuates liver fibrosis by suppressing endoplasmic reticulum stress in hepatic stellate cells. Chin. Med. J. Engl. 135 (5), 628–630. doi:10.1097/CM9.0000000000001883
Yang, G., Zhao, Z., Zhang, X., Wu, A., Huang, Y., Miao, Y., et al. (2017). Effect of berberine on the renal tubular epithelial-to-mesenchymal transition by inhibition of the Notch/snail pathway in diabetic nephropathy model KKAy mice. Drug Des. Devel Ther. 11, 1065–1079. doi:10.2147/DDDT.S124971
Yang, J., Huang, Y., Cui, Z., Liu, C., and Xie, G. (2024). Rhizoma coptidis can inhibit the excessive proliferation, inflammation, and transformation of lung fibroblasts into myofibroblasts. Allergol. Immunopathol. Madr. 52 (4), 15–20. doi:10.15586/aei.v52i4.1111
Yang, L., Yu, S., Yang, Y., Wu, H., Zhang, X., Lei, Y., et al. (2022). Berberine improves liver injury induced glucose and lipid metabolic disorders via alleviating ER stress of hepatocytes and modulating gut microbiota in mice. Bioorg Med. Chem. 55, 116598. doi:10.1016/j.bmc.2021.116598
Yang, Z., Bian, M., Ma, J., Dong, Y., Yang, D., Qiu, M., et al. (2023). Berberine regulates pulmonary inflammatory microenvironment and decreases collagen deposition in response to bleomycin-induced pulmonary fibrosis in mice. Basic Clin. Pharmacol. Toxicol. 132 (2), 154–170. doi:10.1111/bcpt.13818
Yi, J., Wu, S., Tan, S., Qin, Y., Wang, X., Jiang, J., et al. (2021). Berberine alleviates liver fibrosis through inducing ferrous redox to activate ROS-mediated hepatic stellate cells ferroptosis. Cell Death Discov. 7 (1), 374. doi:10.1038/s41420-021-00768-7
Yu, H., Li, C., Yang, J., Zhang, T., and Zhou, Q. (2016). Berberine is a potent agonist of peroxisome proliferator activated receptor alpha. Front. Biosci. 21 (5), 1052–1060. doi:10.2741/4440
Yuan, G. J., Zhang, M. L., and Gong, Z. J. (2004). Effects of PPARg agonist pioglitazone on rat hepatic fibrosis. World J. Gastroenterol. 10 (7), 1047–1051. doi:10.3748/wjg.v10.i7.1047
Zeng, H., Dou, S., Zhao, J., Fan, S., Yuan, X., Zhu, S., et al. (2011). The inhibitory activities of the components of Huang-Lian-Jie-Du-Tang (HLJDT) on eicosanoid generation via lipoxygenase pathway. J. Ethnopharmacol. 135 (2), 561–568. doi:10.1016/j.jep.2011.03.055
Zhang, B. J., Xu, D., Guo, Y., Ping, J., Chen, L. B., and Wang, H. (2008). Protection by and anti-oxidant mechanism of berberine against rat liver fibrosis induced by multiple hepatotoxic factors. Clin. Exp. Pharmacol. Physiol. 35 (3), 303–309. doi:10.1111/j.1440-1681.2007.04819.x
Zhang, H., Wei, J., Xue, R., Wu, J. D., Zhao, W., Wang, Z. Z., et al. (2010). Berberine lowers blood glucose in type 2 diabetes mellitus patients through increasing insulin receptor expression. Metabolism 59 (2), 285–292. doi:10.1016/j.metabol.2009.07.029
Zhang, Q., Xiao, X., Li, M., Li, W., Yu, M., Zhang, H., et al. (2014a). Berberine moderates glucose metabolism through the GnRH-GLP-1 and MAPK pathways in the intestine. BMC Complement. Altern. Med. 14, 188. doi:10.1186/1472-6882-14-188
Zhang, X., He, H., Liang, D., Jiang, Y., Liang, W., Chi, Z. H., et al. (2016a). Protective effects of berberine on renal injury in streptozotocin (STZ)-Induced diabetic mice. Int. J. Mol. Sci. 17 (8), 1327. doi:10.3390/ijms17081327
Zhang, X., Zhao, Y., Zhang, M., Pang, X., Xu, J., Kang, C., et al. (2012). Structural changes of gut microbiota during berberine-mediated prevention of obesity and insulin resistance in high-fat diet-fed rats. PLoS One 7 (8), e42529. doi:10.1371/journal.pone.0042529
Zhang, Y., Li, X., Zhang, Q., Li, J., Ju, J., Du, N., et al. (2014b). Berberine hydrochloride prevents postsurgery intestinal adhesion and inflammation in rats. J. Pharmacol. Exp. Ther. 349 (3), 417–426. doi:10.1124/jpet.114.212795
Zhang, Y. P., Deng, Y. J., Tang, K. R., Chen, R. S., Liang, S., Liang, Y. J., et al. (2019). Berberine ameliorates high-fat diet-induced non-alcoholic fatty liver disease in rats via activation of SIRT3/AMPK/ACC pathway. Curr. Med. Sci. 39 (1), 37–43. doi:10.1007/s11596-019-1997-3
Zhang, Z., Guo, M., Li, Y., Shen, M., Kong, D., Shao, J., et al. (2020). RNA-binding protein ZFP36/TTP protects against ferroptosis by regulating autophagy signaling pathway in hepatic stellate cells. Autophagy 16 (8), 1482–1505. doi:10.1080/15548627.2019.1687985
Zhang, Z., Li, B., Meng, X., Yao, S., Jin, L., Yang, J., et al. (2016b). Berberine prevents progression from hepatic steatosis to steatohepatitis and fibrosis by reducing endoplasmic reticulum stress. Sci. Rep. 6, 20848. doi:10.1038/srep20848
Zhang, Z., Zhao, S., Yao, Z., Wang, L., Shao, J., Chen, A., et al. (2017). Autophagy regulates turnover of lipid droplets via ROS-dependent Rab25 activation in hepatic stellate cell. Redox Biol. 11, 322–334. doi:10.1016/j.redox.2016.12.021
Zhao, J., Ma, X., Li, S., Liu, C., Liu, Y., Tan, J., et al. (2023). Berberine hydrochloride ameliorates PM2.5-induced pulmonary fibrosis in mice through inhibiting oxidative stress and inflammatory. Chem. Biol. Interact. 386, 110731. doi:10.1016/j.cbi.2023.110731
Zhao, P., Sun, X., Chaggan, C., Liao, Z., Wong, K., He, F., et al. (2020a). An AMPK-caspase-6 axis controls liver damage in nonalcoholic steatohepatitis. Science 367 (6478), 652–660. doi:10.1126/science.aay0542
Zhao, X., Kwan, J. Y. Y., Yip, K., Liu, P. P., and Liu, F. F. (2020b). Targeting metabolic dysregulation for fibrosis therapy. Nat. Rev. Drug Discov. 19 (1), 57–75. doi:10.1038/s41573-019-0040-5
Zhi, D., Feng, P. F., Sun, J. L., Guo, F., Zhang, R., Zhao, X., et al. (2015). The enhancement of cardiac toxicity by concomitant administration of Berberine and macrolides. Eur. J. Pharm. Sci. 76, 149–155. doi:10.1016/j.ejps.2015.05.009
Zhou, J. Y., Zhou, S. W., Zhang, K. B., Tang, J. L., Guang, L. X., Ying, Y., et al. (2008). Chronic effects of berberine on blood, liver glucolipid metabolism and liver PPARs expression in diabetic hyperlipidemic rats. Biol. Pharm. Bull. 31 (6), 1169–1176. doi:10.1248/bpb.31.1169
Zimmermann, H. W., and Tacke, F. (2011). Modification of chemokine pathways and immune cell infiltration as a novel therapeutic approach in liver inflammation and fibrosis. Inflamm. Allergy Drug Targets 10 (6), 509–536. doi:10.2174/187152811798104890
Glossary
Keywords: berberine, fibrosis, natural product, pharmacological mechanisms, treatment
Citation: Liu X, Liang Q, Wang Y, Xiong S and Yue R (2024) Advances in the pharmacological mechanisms of berberine in the treatment of fibrosis. Front. Pharmacol. 15:1455058. doi: 10.3389/fphar.2024.1455058
Received: 26 June 2024; Accepted: 09 September 2024;
Published: 20 September 2024.
Edited by:
Ligia Salgueiro, University of Coimbra, PortugalReviewed by:
Olga Silva, Universidade de Lisboa, PortugalArtur Figueirinha, University of Coimbra, Portugal
Copyright © 2024 Liu, Liang, Wang, Xiong and Yue. This is an open-access article distributed under the terms of the Creative Commons Attribution License (CC BY). The use, distribution or reproduction in other forums is permitted, provided the original author(s) and the copyright owner(s) are credited and that the original publication in this journal is cited, in accordance with accepted academic practice. No use, distribution or reproduction is permitted which does not comply with these terms.
*Correspondence: Rensong Yue, c29uZ3Jlbnl1ZUBjZHV0Y20uZWR1LmNu
†These authors have contributed equally to this work and share first authorship