- 1Department of Gastroenterology, The Fourth Affiliated Hospital of China Medical University, Shenyang, China
- 2Endoscopy Center, The Fourth Affiliated Hospital of China Medical University, Shenyang, China
- 3First Digestive Endoscopy Department, The Fourth Affiliated Hospital of China Medical University, Shenyang, China
Background: Hepatocellular carcinoma accounts for 80% of primary liver cancers, is the most common primary liver malignancy. Hepatocellular carcinoma is the third leading cause of tumor-related deaths worldwide, with a 5-year survival rate of approximately 18%. Chemotherapy, although commonly used for hepatocellular carcinoma treatment, is limited by systemic toxicity and drug resistance. Improving targeted delivery of chemotherapy drugs to tumor cells without causing systemic side effects is a current research focus. Chitosan, a biopolymer derived from chitin, possesses good biocompatibility and biodegradability, making it suitable for drug delivery. Enhanced chitosan formulations retain the anti-tumor properties while improving stability. Chitosan-based biomaterials promote hepatocellular carcinoma apoptosis, exhibit antioxidant and anti-inflammatory effects, inhibit tumor angiogenesis, and improve extracellular matrix remodeling for enhanced anti-tumor therapy.
Methods: We summarized published experimental papers by querying them.
Results and Conclusions: This review discusses the physicochemical properties of chitosan, its application in hepatocellular carcinoma treatment, and the challenges faced by chitosan-based biomaterials.
1 Introduction
Hepatocellular carcinoma (HCC) is the predominant form of primary liver cancer, accounting for over 80% of liver cancer cases (Valderrama-Treviño et al., 2017). HCC is closely associated with hepatitis B, hepatitis C, alcoholic liver disease, and fatty liver disease (Kulik and El-Serag, 2019). The incidence of HCC is higher in men, predominantly due to higher rates of alcohol consumption (Villanueva, 2019). Globally, the incidence of HCC is steadily increasing, projecting over 1 million new cases per year by 2025 (Rahib et al., 2014). HCC is often asymptomatic in its early stages, leading to diagnosis at advanced stages with limited treatment options, poor prognosis, and short survival times (Fan et al., 2023). HCC ranks as the third leading cause of cancer-related deaths globally, with a 5-year survival rate of approximately 18% (Siegel et al., 2022). In the United States, the average 5-year survival rate for HCC patients is 19.6% (Chidambaranathan-Reghupaty et al., 2021).
Patients with HCC have various treatment options, including liver transplantation, surgical resection, percutaneous ablation, radiotherapy, chemotherapy, and targeted or systemic therapy (Vogel et al., 2022). Surgical resection is the preferred and most effective treatment for early-stage HCC (Sugawara and Hibi, 2021; Duong et al., 2022). However, it is unsuitable for unresectable and metastatic HCC. Chemotherapy is commonly used but often accompanied by systemic side effects (Bhatt and Wu, 2023). Advanced HCC patients who cannot undergo surgery face challenges due to tumor lesions and the development of treatment resistance (Zhao et al., 2022a; Zhao et al., 2022b). Conventional systemic chemotherapy lacks target selectivity, leading to high recurrence risks, multidrug resistance, and severe side effects. Transcatheter arterial chemoembolization (TACE) selectively blocks tumor blood supply to induce tumor cell necrosis through ischemia and hypoxia (Chang et al., 2021). Local delivery of chemotherapy drugs also enhances their anti-tumor effects (Qin et al., 2022). The combination of TACE with portal embolization or portal chemoembolization in patients with HCC improves efficacy and reduces recurrence rates (Shao et al., 2021). Although TACE is the preferred method of palliative care for HCC, the choice of a chemotherapy agent and the timing between administrations require further study (Ando et al., 2021). For HCC that cannot be cured, a liver transplant is the best way to restore liver function (Reichman et al., 2019). However, the shortage of liver transplant donors, immune rejection after liver transplantation, and prevention and treatment of liver cancer recurrence and metastasis after liver transplantation are still the direction of unremitting efforts in the future (Fan et al., 2023).
In recent years, nano-delivery strategies have shown promise in enhancing anti-tumor effects and have become a potential trend in cancer treatment. Chitosan, a biopolymer derived from chitin, is non-toxic and exhibits good histocompatibility and biodegradability (Mallakuntla et al., 2021; Abourehab et al., 2022; Saran et al., 2022; Kim et al., 2023; Matloob et al., 2023; Unal et al., 2023; El-Araby et al., 2024). It has been extensively studied as a delivery strategy for various tumors, including lung cancer, breast cancer, pancreatic cancer, and HCC (Xia Y. et al., 2022; Bashir et al., 2022; Karimi et al., 2023). Chitosan-based nanomaterials have shown significant anti-tumor effects, leveraging their ability to selectively enter cancer cells through the enhanced permeability and retention (EPR) effect (Xin et al., 2017). This article provides an overview of the physicochemical properties of chitosan. It also summarizes the anti-tumor effects of chitosan-based nanomaterials in HCC, as highlighted in Table 1; Scheme 1. Additionally, the challenges associated with the use of chitosan nanomaterials in HCC treatment are discussed.
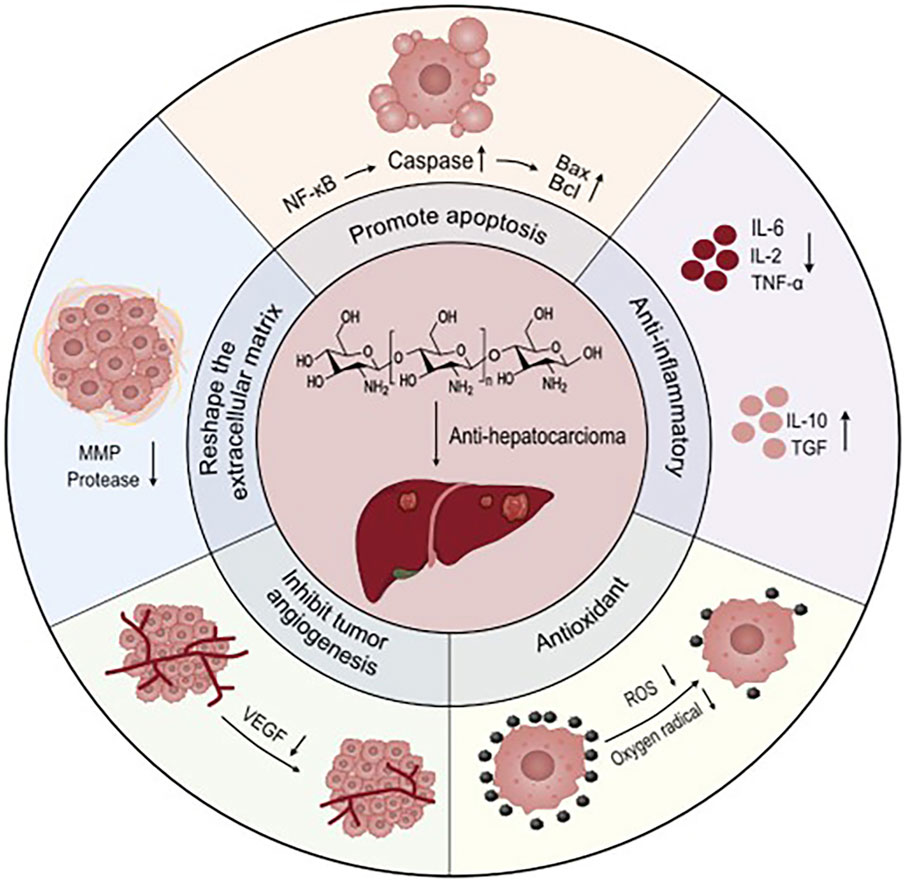
Scheme 1. Mechanism of action of chitosan in hepatocellular carcinoma: Chitosan-based materials induce apoptosis of HCC cells by promoting mitochondrial pathway, promote downregulation of expression of anti-inflammatory cytokines, and upregulation of expression of inflammatory cytokines, promote ROS degradation, scavenge oxygen radicals around HCC cells. Reducing tumor angiogenesis, reduce MMP and protease expression and remodel the tumor extracellular matrix to achieve anti-tumor effects.
2 Physical and chemical properties of chitosan
Chitosan is a natural polysaccharide obtained by deacetylation of chitin (Xia Y. L. et al., 2022). When chitinin deacetylation reaches at least 50%, it is called chitosan (Hallmann and Gerngroß, 2022). The main component of chitosan is a mixture between N-acetyl-d-glucosamine and β-(1,4)-linked-d-glucosamine (Satitsri and Muanprasat, 2020). Due to its amino groups in the backbone, chitosan often exhibits positively charged cationic copolymers. Chitosan itself has been reported to have antitumor, antioxidant, and wound-healing effects (Manna et al., 2023). Chitosan is soluble in organic acids but insoluble in neutral and alkaline solutions. The solubility of chitosan depends on the amount of free amino group and N-acetyl group (Hallmann and Gerngroß, 2022). The physicochemical properties of CS are inversely affected by its degree of deacetylation and molecular weight (Ardean et al., 2021). Chitosan has active hydroxyl, amino, and linear polyamines at positions C2, C3, and C6, and these functional groups can be modified to confer different functions on chitosan (Balagangadharan et al., 2017). For example, the conversion of primary amine groups on chitosan C2 into quaternary salts is the main mechanism for enhancing its antibacterial, antioxidant, anticoagulant, and mucus adhesion properties (Piras et al., 2019). Therefore, chitosan plays an important role in tissue engineering and regenerative medicine. In tumor treatment, chitosan can target the tumor microenvironment, increase the Enhanced permeability and retention (EPR) effect, improve the delivery ability of anti-tumor drugs, and reduce the off-target and abscopal effect of cancer nano drugs (Xia Y. et al., 2022; Zaiki et al., 2023). Chitosan can also reduce cell proliferation, eliminate tumor angiogenesis, and inhibit HCC growth (Satitsri and Muanprasat, 2020). Chitosan-based nanostructures can characterize the pharmacokinetics of natural and synthetic drugs, thereby increasing the effectiveness of HCC therapy (Karimi et al., 2023). Nanomedicine developed using functionalized chitosan becomes a potential trend in tumor treatment.
3 Chitosan-based materials enhance antitumor therapy
3.1 Promote apoptosis of tumor cells
Apoptosis is triggered by a series of mitochondria dysfunction, including the collapse of the intimal potential, swelling of the mitochondria, and increased permeability (Chipuk et al., 2006). Mitochondria control a variety of cellular physiological processes, including cell respiration, metabolism, signaling, differentiation, apoptosis, and intracellular calcium levels (Smith et al., 2011). Chitosan can induce apoptosis. The specific mechanism is that chitosan competitively blocks the integral proteins on tumor cells, so that the tumor loses the ability to adhere to normal tissues, thereby inhibiting tumor metastasis, and it can also directly enter the cell and activate the caspase-3 at the end of the apoptosis pathway, thereby causing the degradation of structural proteins and functional proteins, and finally disintegrating the cell (Atmaca et al., 2024). Mitochondrial function is associated with anabolic, unlimited multiplication and decreased apoptosis autophagy in cancer cells (Wang et al., 2017). Doxorubicin (DOX) is a mitotically active cyto-toxic agent that binds specifically to phospholipid cardiolipin and could accumulate mitochondria. Studies have shown that DOX-mediated membrane perturbation can inhibit mitochondrial membrane potential disruption of complex I and II disordered electron transport chains, thereby affecting cellular energy transfer (Gorini et al., 2018). Lactobionic (LA) has HCC cell targeting by binding to Asiatica protein (ASGP) receptors that are overexpressed on the HCC cell surface (Zhang et al., 2011). The grafting of LA and chitosan can enhance the HCC cell targeting ability of chitosan. Hefnawy et al. used carboxymethyl chitosan to complexe poly-acrylate, and glycyrrhetinic acid (GA) and LA grafted onto the complex to make a two-ligand nanoshell structure for the delivery of DOX (Figure 1) (Hefnawy et al., 2020). GA and LA double ligands enhance the HCC cell targeting ability of core-shell nanoparticles, and precisely release DOX into tumor cells to achieve their anti-tumor effects (Li et al., 2019). Studies have shown that nanoparticles larger than 150 nm are able to maximize the benefit of EPR effects in HCC cells (Torchilin, 2011). The core-shell nanoparticles have a diameter of 274 nm and are capable of loading DOX for more than 10 days. The ability of HCC cells to phagocytose DOX is greatly improved, and the significantly increased apoptotic genes are caspase 3, p53, and Bax. Triphenylphosphine (TPP) is one of the polymers commonly used to target mitochondria, and TPP has a unique structure composed of lipophilic phenyl groups and phosphine cations, which allows it to be deposited in mitochondria (Mossalam et al., 2010). Arisifa et al. grafted TPP with chitosan to deliver DOX to make DOX-TPP-CS NPS for the treatment of HCC (Arafa et al., 2022). The particle size of DOX-TPP-CS NPS is 70–110 nm, and the spherical and positive surface charge structure of DOX-TPP-CS NPTs enhances mitochondrial uptake. DOX-TPP-CS NPS continuously releases DOX at 168 h and reduces systemic toxicity. The co-incubation of DOX-TPP-CS NPS with HCC cells for 48 h compared with the blank control group promoted the programmed death of HCC cells by 7.86 times higher than that of the control group.
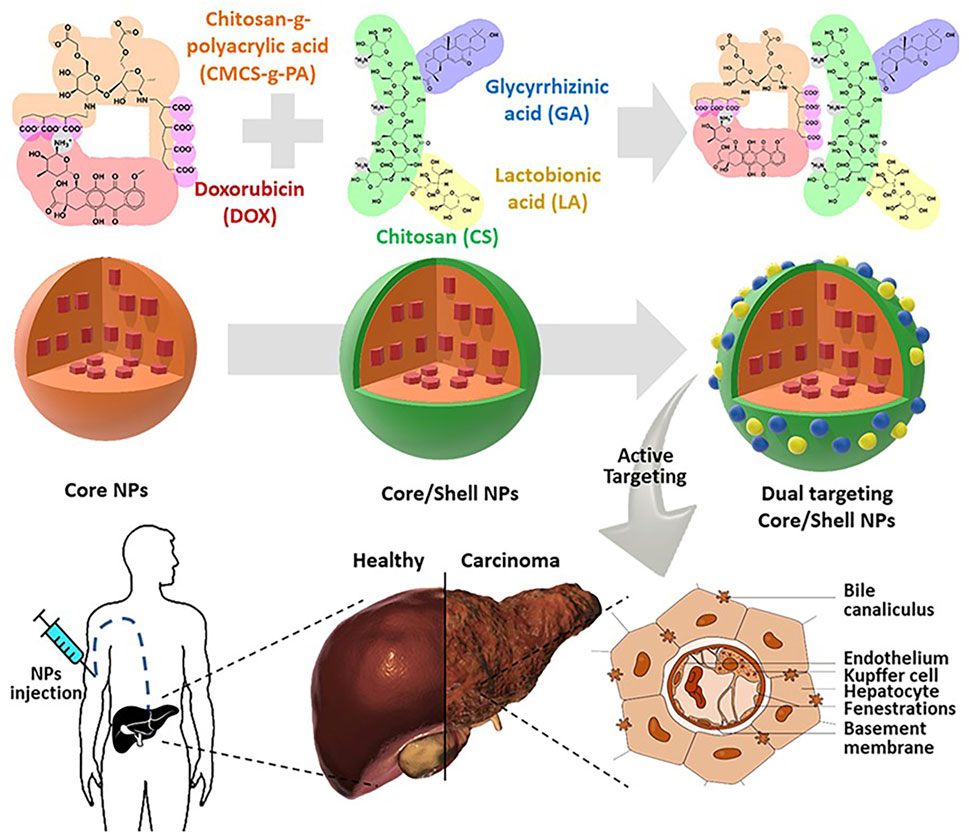
Figure 1. Carboxymethyl chitosan to complexe polyacrylate, and glycyrrhetinic acid (GA) and LA grafted onto the complex to make a two-ligand nanoshell structure for the delivery of DOX. After intravenous injection of nanoparticles, it can actively target liver cancer cells to achieve anti-tumor effects. Reproduced with permission from (Hefnawy et al., 2020).
Coated magnetic Fe3O4 can absorb more drug molecules, increasing the dispersion and stability of chitosan nanoparticles (He et al., 2010). Coated chitosan nanoparticles can enhance the drug loading rate of chitosan nanoparticles, making chitosan nanoparticles a good choice for nano drug loading (Jędrzak et al., 2020). Chen L. et al. (2022) used Fe3O4-coated chitosan, DOX was loaded into CS by the aldehyde group, and GQD was prepared into DOX-Fe3O4@CGA on the surface of magnetic CS by amide bonds. The encapsulation efficiency of DOX is about 85%, and the loading efficiency is about 12% on average. The DOX-Fe3O4@CGA is injected into tumor-bearing mice and collected at the tumor site for 6 h to treat the tumor more effectively. TwHFOLIDE (TP) is the main component of Chinese herbal medicine and has anti-inflammatory and antitumor effects (Gali-Muhtasib et al., 2015). TP has been shown to have anti-tumor effects in hematologic tumors, lung cancer, liver cancer (Zhao et al., 2020; Gao et al., 2021). TP limits its clinical application due to its high toxicity and low water solubility. Galactosylated chitosan also has the ability to target HCC cells, binding to TP to deliver TP to tumor cells (Yu et al., 2014). Zhang Y-Q. et al. (2019) combined galactosylated chitosan with TP to make GC-TP-NPTs to enhance the targeting of HCC cells. The particle size of GC-TP-NPs was 204.2 ± 1.2 nm, GC-TP-NPs released about 70% TP within 2 h, and GC-TP-NPs released nearly 80.0% TP after 24 h of incubation. The precise targeting of GC-TP-NPs not only reduced hepatic and renal toxicity but also induced apoptosis of HCC cells through TNF/NF-κB/BCL2 signaling. Niacin can increase the NAD+/NADH ratio in the body and promote autophagy in tumor cells by inducing NAD+/NADH balance (Song et al., 2013). Hanafy et al. (2023) developed chitosan liposomes loaded with niacin and curcumin to achieve autophagy in HCC cells. The diameter of the nanoliposomes is 96 ± 1.2 μm. In vivo, chitosan liposomes can induce autophagy by activating the GPR109A/AMPK/NRF-2 signaling pathway. Sorafenib (SF) is an oral multi-kinase inhibitor with significant anticancer effects through antiproliferative, antiangiogenic and pro-apoptotic mechanisms (Juaid et al., 2021). Fahad Albalawi et al. fabricated chitosan nanoparticles (SF-CS NPs) for SF delivery using CS and sodium tripolyphosphate (TPP) (Albalawi et al., 2023). At the lowest concentration of TPP of 2.5 mg/mL, SF-CS NPs showed the largest spherical particles with an average diameter of 212.4 ± 59.7 nm. In vitro experiments confirmed that SF-CS NPs could continuously release SF for 169 h. Gemcitabine is a pyrimidine nucleoside antimetabolite that is effective in the treatment of HCC in combination with other anticancer drugs, including sorafenib, oxaliplatin, carboplatin, and bevacizumab (Fan et al., 2023). However, adverse effects associated with gemcitabine and myelosuppression and pulmonary toxicity remain a problem (Chi et al., 2012). Nair et al. (2019) designed galactosylated chitosan nanoparticles for delivery of gemcitabine to reduce its toxic side effects. The zeta potential values (19–22 mV) of galactosylated chitosan nanoparticles increase modest rejection and electrostatic stabilization between galactosylated chitosan nanoparticles to provide stability. In vivo experiments have proved that galactosylated chitosan nanoparticles can release about 85% of gemcitabine within 24 h, and the drug distribution in vivo has proved that the accumulation of galactosylated chitosan nanoparticles in the liver is significantly higher than that of other organs, reducing the adverse reactions in the whole body. The study of chitosan-based nanomaterials to promote apoptosis in HCC cells has been widely confirmed (Priya et al., 2020).
3.2 Antioxidant
After mitochondrial dysfunction, tumor cells are in a hypoxic state. Long-term hypoxia leads to enhanced glycolytic pathways, enhanced hypoxia-inducible factor (HIF-1α), and the production of Reactive oxygen species (ROS) (Zhang W. et al., 2019). The imbalance between ROS and antioxidants in the body is one of the factors that promote the proliferation of tumor cells (Snezhkina et al., 2019). Mitochondria are the main organs for ROS production, and most anti-cancer drugs alter excess ROS production in cancer cells, thereby activating mitochondrial intrinsic pathways, releasing pro-apoptotic factors, and leading to apoptosis (Perillo et al., 2020). Cerium oxide (CeO2) exerts antioxidant activity by removing ROS from the body (Siposova et al., 2022). Sathiyaseelan et al. (2022) used CeO2 modified 5-fluorouracil (5FU)-loaded chitosan nanoparticles to make CS-5FU-CeO2NPs for HCC cell therapy. 5FU binds to chitosan through hydrogen bonding and intermolecular force, which greatly increases the drug loading rate of CS-5FU-CeO2NPs (16.17% ± 0.55%). In vitro experiments, CS-5FU-CeO2NPs released 21.88% of 5-FU within 8 h and sustained release within 6 months. Compared with CeO2NPs, CS-5FU-CeO2NPs greatly improved the scavenging capacity of free radicals and promoted HCC apoptosis (apoptotic cell mortality was 26.04%). Although mitochondria are the main site of ROS production, CS-5FU-CeO2NPs clearance of ROS does not cause damage to cellular structures (nuclear and mitochondrial membrane potentials). This also makes CS-5FU-CeO2NPs have good biocompatibility and low toxicity. Scavenging of oxidative free radicals in the body can promote apoptosis of tumor cells. Targeted induction of ROS production also induces apoptosis in tumor cells. Chitosan nanoparticles can induce increased ROS production, leading to ROS-induced activation of mitochondrial disease and endoplasmic reticulum stress (Jiang et al., 2019). Anushree et al. developed phosphorylated galactosylated chitosan (PGC) for antioxidant use in HCC cells (U et al., 2022). The average particle size of PGC is 197 nm, and PGC has a high affinity with ASGPR on the surface of HCC cells, which can enhance the HCC cell targeting ability of PGC (Zhu et al., 2022). In vivo experiments have confirmed that PGC can inhibit lipid peroxidation and superoxide scavenging ability and enhance glutathione levels compared with chitosan. PGC exhibits stronger anti-tumor effects than chitosan.
Curcumin is a polyphenolic compound whose clinical effects have been widely proven, including anti-inflammatory, antioxidant, antitumor, antiviral, antibacterial and analgesic effects (Hewlings and Kalman, 2017). Studies have shown that curcumin’s anti-inflammatory properties are achieved by blocking IκBα phosphorylation and degradation (Wilken et al., 2011). In addition to this, curcumin scavenges superoxide, nitric oxide, and hydrogen peroxide free radicals and reduces inflammation by lowering histamine levels (Alok et al., 2015). Due to low water solubility, low bioavailability, chemical instability, rapid metabolism in the gastrointestinal tract, and other factors, the clinical application of curcumin is limited. Kong et al. (2019) used silica encapsulation curcumin nanoparticles (SCNP) and chitosan co-encapsulation to make cur-cumin nanoparticles (CSCNP). The average size of CSCNP is 75.0 ± 14.62 nm and capable of loading 28.9% curcumin. In vitro experiments have confirmed that CSCNP does not affect the physiological activity of normal cells. Compared to curcumin, CSCNP has a stronger oxidant free radical scavenging effect. Celastrol is a natural proteasome inhibitor extracted from Chinese herbal medicine, according to a wide range of antitumor effects (Wang et al., 2019). However, the low solubility, low bioavailability and systemic toxicity of Celastrol hinder its clinical application (Chen et al., 2022). Zhang et al. (2023) used glycyrrhetinic acid (GA) and carboxymethylchitosan (CMCS) to make polymer micelles Cela/GCTR PMs for the delivery of Celastrol. HCC cells showed high expression of GA receptors, and GC-made Cela/GCTR PMs have good HCC cell targeting, thereby increasing HCC cell accumulation in Celastrol. The particle size of Cela/GCTR PMs is 220.17 ± 5.50 nm. Cela/GCTR PMs release Celastrol continuously, with a cumulative release rate of >12% at 70 h. Studies have shown that Cela/GCTR PMs can target ROS in HCC cells to achieve antioxidant effects in vivo. Compared with Celastrol, Cela/GCTR PMs showed good proliferation inhibition in hepatoma cells. Not only that, Cela/GCTR PMs have a stronger tumor suppression rate. This shows that Cela/GCTR PMs have great potential as an anti-liver cancer drug delivery system.
3.3 Anti-inflammatory
Inflammation is one of the important features of tumor-contributing markers and plays an important role in tumorigenesis (Hanahan, 2022). Inflammation can regulate and induce cell polarization in the tumor microenvironment and induce the development of tumor cell drug resistance (Denk and Greten, 2022; Kennel et al., 2023). Factors released by inflammatory cells (transforming growth factor (TGF)-β, tumor necrosis factor (TNF)-α, and interleukin (IL)-6) stimulate tumor cell survival and proliferation through nuclear factor (NF)-κB and signal transductors and transcriptional activators (STAT) 3 (Greten and Grivennikov, 2019; Kruse et al., 2023). M2-macrophages, fibroblasts, and myeloid-derived suppressor cells can induce immunosuppressive blocker antitumor effects of T cells (Kuo et al., 2022). Extended activation of the IL-6/IL-6-R signaling pathway is critical in the occurrence and progression of HCC (Hatting et al., 2015). Therefore, reducing the inflammatory response in the tumor microenvironment has a degree of antitumor effect. Butyric acid (BA) reduces the production of cytokines (IL-6, IL-8, TNF-α, and TGF-β) to achieve anti-inflammatory effects in HCC (Meijer et al., 2010). In addition, BA shows anticancer properties against HCC cells mainly based on its histone deacetylase (HDAC) inhibitory activity (Coradini and Speranza, 2005). However, the low bioavailability of BA and poor intestinal absorption after oral administration limit its clinical use (Clemente et al., 2012). Quagliariello et al. (2019) prepared chitosan liposomes, which were legally loaded with BA by membrane water for BA delivery. The chitosan liposomes that encapsulate BA have an average size of 126 nm and can continuously release BA around tumor cells. Chitosan liposomes that encapsulate BA have good cytocompatibility and do not cause toxicity to normal cells. In vitro, experimental results showed that chitosan liposomes encapsulated BA could reduce the production of IL-8, IL-6, TGF-β, and TNF-α to achieve anti-inflammatory effects.
Aspirin is a commonly used nonsteroidal anti-inflammatory drug in clinical practice, and the most well-known biological target of aspirin is cyclooxygenase 2 (COX-2) (Menter and Bresalier, 2023). COX-2 is highly expressed in HCC to convert arachidonic acid to prostaglandins, thereby helping to promote HCC cell proliferation and inhibit apoptosis (Kern et al., 2004). Aspirin inhibits cell migration and induces apoptosis in human HCC cells by inhibiting the activation of the NF-κB pathway, downregulating COX-2 levels (Dong et al., 2014). A meta-analysis by Zeng et al. (2023) has confirmed that aspirin use is associated with a reduced risk of hepatocellular carcinoma. Wang et al. (2020) developed a chitosan nanoparticle for the delivery of aspirin and 5-fluororacil (5-FACN). The average particle size of 5-FACN is 109.2 ± 5.2 nm. The encapsulation efficiency of 5-FACN for 5-Fu and aspirin was 88.6% and 91.0%, respectively. 5-FACN can continuously release 5-Fu and aspirin around tumor cells. Studies have shown that 5-FACN is able to reduce COX-2 and prostaglandin expression around tumors. Macrophages are involved in all stages of tumor progression and are associated with poor prognosis and chemotherapy resistance (Bohn et al., 2018). Tumor-associated macrophages (TAMs) are mainly divided into anti-tumor M1-TAM and protumor M2-TAM (Sica et al., 2008). In tumor progression, the pro-tumor function of TAM is caused by NF-κB activation (Li et al., 2017). It has been reported that NF-κB can promote the polarization of TAM from M1 to M2, and inhibiting NF-κB activation can enhance the ratio of M1/M2 (Taniguchi and Karin, 2018). Fan et al. (2021) designed chitosan nanopole capsules for the delivery of cisplatin, named PC-CP. PC-CP can cause less fibroblast response and less macrophage response in the tumor microenvironment.
Wang T. et al. (2019) prepared cationic lipid-based nanoparticles (SF-CLN) loaded with SF, and coated CMCS in SF-CLN to prepare CMCS/SF-CLN for targeting HCC cells. The load ratio of CMCS/SF-CLN to SF is 7.43% ± 0.51%. CMCS with a negative charge can make CMCS/SF-CLN repel from normal cell membranes and reduce the cytotoxicity of CMCS/SF-CLN. In HCC cells, CMCS/SF-CLN has charge-inversion properties and can adapt to the acidic environment of tumor cells and aggregate in large quantities. In vivo, experiments confirmed that CMCS/SF-CLN has a good ability to target HCC and reduce TGF-β1 and IL-10 secreted by M2-TAM and M2-TAM. Compared with SF, CMCS/SF-CLN has better anti-inflammatory and anti-tumor effects.
3.4 Inhibits tumor angiogenesis
Tumor angiogenesis plays an important role in the growth and metastasis of tumor cells (Park et al., 2007). Tumor blood vessels provide nutrients and oxygen to tumor cells and carry away metabolic waste products produced by tumor cell metabolism (Miura et al., 2010). The formation of new blood vessels is the result of the intermodulation of proangiogenic compounds such as vascular endothelial growth factor (VEGF), transforming growth factor-β (TGF-β), basic fibroblast growth factor (bFGF), matrix metalloproteinases (MMP), platelet-derived growth factor (PDGF), and antiangiogenic factors such as tissue inhibitors of angiostatin, endostatin, and metalloproteinases (Zhou et al., 2022). Inhibition of tumor angiogenesis inhibits tumor cell proliferation. CMCS has been shown to inhibit tumor angiogenesis and downregulate levels of VEGF and TIMP-1, inhibitors of MMP (Jiang et al., 2015). Rutin has antioxidant, anti-inflammatory, antithrombotic and cytoprotective activities (Ma et al., 2018). However, Rutin’s poor water solubility and low bioavailability limit its clinical use. Radwan and Ali (2021) used chitosan and poly (acrylic) to make nano gels (CANs) for the delivery of Rutin. CAN has a diameter of 116.7 nm and is connected to Rutin by hydrogen bonding. CAN release Rutin around HCC cells, upsetting the balance of angiogenesis and disruption. Compared to Rutin, CAN reduced the expression of VEGF and inhibited tumor vascular formation. Not only that, CAN also reduces the proliferation of HCC cells and promotes HCC apoptosis.
Telmisartan (TLM) alleviates malignant cell proliferation by activating peroxisome proliferator-activated receptor γ (Li et al., 2014). Nasr et al. (2023) designed lactose-modified chitosan nanoparticles to deliver TLM (TLM-LCH NPTs) to enhance the uptake of TLM by HCC cells (Figure 2 (1, 2)). TLM-LCH NPs have a diameter of 145.46 ± 0.7 nm. Studies have confirmed that lactose-modified chitosan nanoparticles actively target ASGPR and enhance the up-take of nanoparticles by HCC cells. TLM-LCH NPs released TLM aggregates in HCC cells at a content 232.92 times higher than that of TLM alone. Compared with the common TLM group, the TLM-LCH NPTs group significantly reduced the expression levels of VEGF and MMP-2. Not only that, TLM-LCH NPs also reduce inflammation around tumor cells and reduce the expression level of alpha-fetoprotein. T cell immunoglobulin mucin-3 (Tim-3) is a promising immune checkpoint molecule for HCC therapy (Romero, 2016). Inhibition of Tim-3 expression may be a novel therapeutic strategy for HCC. Song et al. (2022) grafted Tim-3 siRNA and SF to CMCS nanoparticles by a single emulsification method. Tim-3 siRNA can target and inhibit Tim-3 expression in HCC cells. The diameter of the nanoparticles is 50.49 ± 5.34 nm, and 90% of SF can be released in vivo for 40 h. In vivo experiments have shown that CMCS nanoparticles induce a 95% reduction in tumor vascular density and enhance the recruitment of cytotoxic T cells to kill tumor cells. In another study, Yao et al. (2019) used CMCS nanoparticles to deliver VEGF-siRNA and SF. VEGF-siRNA can target lower VEGF around HCC cells, reduce tumor vascular production, and induce early apoptosis. Xu et al. (2018) grafted nonaarginine (9R) onto chitosan (CS) and constructed a positively charged kernel (CS-SS-9R) for delivery of VEGF-siRNA. In vivo experiments confirmed that siVEGF was rapidly released into the cytoplasm, resulting in a 78.9% decrease in VEGF expression and 81.2% inhibition of tumor cell proliferation.
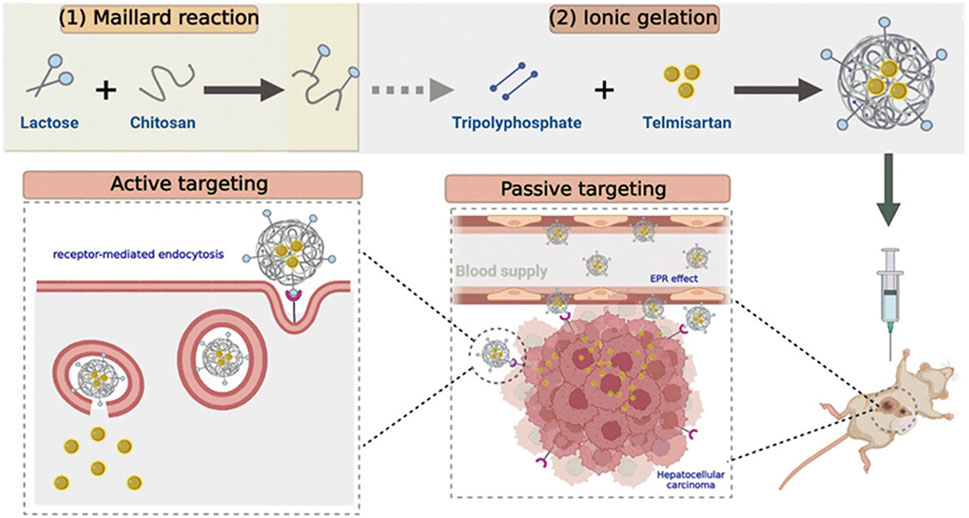
Figure 2. Lactose-modified chitosan nanoparticles (LCH NPs) were used as a delivery system for the delivery of telmisartan. After intravenous injection of nanoparticles, the nanoparticles can enhance the targeting effect on the liver, release telmisartan, and enhance its anti-tumor effect. Reproduced with permission from (Nasr et al., 2023).
3.5 Extracellular matrix remodeling
The extracellular matrix (ECM) is composed of proteins, glycoproteins, and polysaccharides and is an important component of the tumor microenvironment (TME) (Lu et al., 2012). The ECM is a dynamically changing system that provides nutrients to the tumor cell parenchyma and regulates tumor cell growth and metabolism (Bissell and Hines, 2011). ECM is an important factor for tumor cells to escape attack by the immune system (Roy et al., 2023). Chronic inflammation and dysregulation of ECM remodeling work together to contribute to an immunosuppressive environment, which in turn promotes HCC proliferation, invasion, and metastasis (Chen et al., 2023). In addition, ECM, which also has a large number of immunosuppressive cells (regulatory T cells, myeloid-derived suppressor cells, tumor-associated fibroblasts, etc.) and cytokines (TGF-β, VEGF, or IL-10), promotes tumor cells to escape attack by the immune system (Chiang et al., 2008). Studies have shown that HCC cells are able to cause abnormal ECM deposition through the WNT/TGFB signaling pathway, leading to intratumor fibrosis (Desert et al., 2023). Liu et al. (2023) confirmed that HCC cells participate in the regulation of ECM through the PI3K/AKT signaling pathway, which leads to tumor cell proliferation, migration, and invasion. Xu et al. (2022) confirmed that MMP in ECM is associated with poor prognosis of HCC. Therefore, regulatory ECM remodeling is a potential clinical mechanism for the treatment of HCC.
Delaying the degradation of host proteins in ECM has advantages in inhibiting tumor cell proliferation (Lu et al., 2012). Nitrotrixine (NIT) is a potent inhibitor of cathepsin B that impairs tumor progression by reducing extracellular matrix degradation (Spottiswoode et al., 2023). Varshosaz et al. (2020) loaded 5-FU and NIT in chitosan-chondroitin nanoparticles (Figure 3 (1–4)). Chondroitin binds to hyaluronic acid in ECM to enhance the targeting of HCC cells by nanoparticles. The particle size of chitosan-chondroitin nanoparticles is 244.7 ± 16.3 nm, the loading rate of 5-FU is 3.5% ± 0.5%, and the loading rate of NIT is 75.1% ± 0.9%. Chitosan-chondroitin nanoparticles can continuously release the carrier, releasing about 6.0% ± 9.5% of 5-FU and 62.9% ± 0.7% of NIT at 8 h. In vivo experiments have shown that chitosan-chondroitin nanoparticles can delay the degradation of ECM and reduce the proliferation and migration of HCC cells compared with NIT. MMP9 plays an important role in proteolysis, membrane peptide degradation, and extracellular protein denaturation of extracellular matrices. This protein denaturation promotes cancer cell proliferation, which promotes metastasis (Deryugina and Quigley, 2006). Zayed et al. developed chitosan nanoparticles for the delivery of Apigenin (Mabrouk Zayed et al., 2022). Apigenin has powerful anticancer, anti-inflammatory and antioxidant activities (Yan et al., 2017). Chitosan nanoparticles have a particle size of 189 nm and are capable of continuous release of Apigenin in vitro, with a 40-h drug release rate of 24%. The Apigenin released by chitosan nanoparticles can downregulate the expression level of MMP-9 and delay HCC cell transfer.
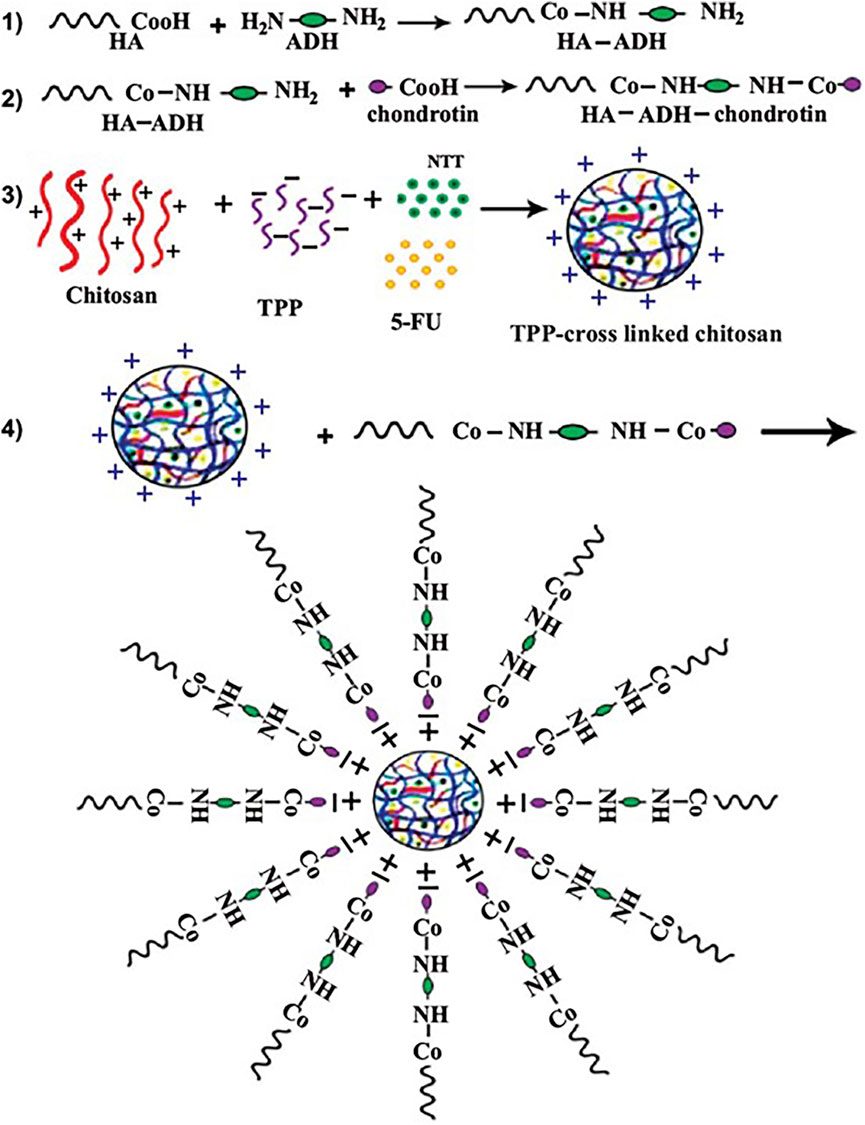
Figure 3. 5-FU and Nitroxoline (NIT) were loaded in chitosan-chondroitin nanoparticles. To target the CD44 receptors of HepG2 cells, Hyaluronic Acid (HA) was conjugated to the chondroitin by adipic acid dihydrazide and the conjugation was confirmed by FTIR and 1HNMR. Target nanoparticles co-delivery of 5-FU and NIT to enhance the 5-FU cytotoxic effects and reduce the metastatic properties of HepG2 cells. Reproduced with permission from (Varshosaz et al., 2020).
4 Challenges for chitosan-based materials
Although chitosan-based biomaterials have achieved excellent results in tumor delivery, the clinical application of chitosan-based materials also presents certain challenges (Patel and Goyal, 2017). Chitosan and chitin are almost inseparable, and chitin, when the deacetylation of chitin reaches at least 50%, is called chitosan (Hallmann and Gerngroß, 2022). Chitin has more protein components than chitosan and therefore has a stronger ability to activate the immune system (Thambiliyagodage et al., 2023). However, too much protein makes chitosan enhance anti-tumor by inducing activation of the immune system, but also causes allergic symptoms in some patients (Taokaew and Kriangkrai, 2023). The purification of chitosan mainly includes strong acid, strong base and enzymatic degradation (Harish Prashanth and Tharanathan, 2007; Islam et al., 2022). Enzymatic degradation is extremely expensive, and large-scale production of high-purity chitosan is still a problem to be solved (Herdiana et al., 2023). Secondly, the biggest challenge of chitosan is its low solubility and poor mechanical properties (Szymańska and Winnicka, 2015). Chitosan is incompatible with hydrophobic chemotherapy drugs and, therefore, has limitations in chemotherapy drug delivery (Herdiana et al., 2023). This necessitates the modification of chitosan materials (Huang et al., 2023). Stability is another challenge affecting chitosan applications. There are significant differences in the effects of molecular weight, degree of acetylation, and purity level of chitosan materials on the stability of chitosan-based materials (Szymańska and Winnicka, 2015). Negatively charged components (gelatin, hyaluronic acid, alginate, etc.) are often crosslinked with chitosan to improve their stability (Hamman, 2010). Therefore, more preclinical studies are needed to improve the stability of chitosan-based materials.
5 Conclusion and outlook
Biomaterials drug delivery strategies to improve anti-tumor therapy have become a research hotspot in recent years. As a type of natural polysaccharide, chitosan has been shown to have antitumor activity due to its biological histocompatibility, low toxicity and positive charge. However, the anti-tumor properties of chitosan are not as significant as one might expect. Due to unfavorable factors such as low solubility, poor mechanical properties, low yield, and poor stability of chitosan, its clinical application is limited. There is an urgent clinical need for a bioactive material with low toxicity, target specificity and excellent drug delivery properties. To this end, a variety of improved chitosan-based biomaterials have been designed for anti-tumor therapy in preclinical studies. Studies have confirmed that chitosan-based biomaterials can promote mitochondria-induced apoptosis, promote tumor cell antioxidants, and reduce the production of IL-8, IL-6, TGF-β, and TNF-α to achieve anti-inflammatory effects. In addition, chitosan-based materials can enhance anti-tumor therapy by inhibiting the expression of VEGF to reduce tumor angiogenesis and promote extracellular matrix remodeling. However, the treatment of HCC by chitosan-based biomaterials is still only in preclinical studies and has not been reported clinically. Therefore, future research should focus on addressing the above difficulties to realize the full potential of chitosan-based biomaterials.
Author contributions
XW: Conceptualization, Data curation, Writing–original draft, Writing–review and editing. YY: Investigation, Software, Writing–original draft, Writing–review and editing. SZ: Methodology, Project administration, Writing–original draft, Writing–review and editing. DW: Investigation, Methodology, Writing–review and editing. LL: Methodology, Software, Writing–original draft. ZZ: Conceptualization, Project administration, Validation, Writing–original draft, Writing–review and editing.
Funding
The author(s) declare that no financial support was received for the research, authorship, and/or publication of this article.
Acknowledgments
We would like to express our appreciation to everyone who was involved in the drafting and preparation of the manuscript.
Conflict of interest
The authors declare that the research was conducted in the absence of any commercial or financial relationships that could be construed as a potential conflict of interest.
Publisher’s note
All claims expressed in this article are solely those of the authors and do not necessarily represent those of their affiliated organizations, or those of the publisher, the editors and the reviewers. Any product that may be evaluated in this article, or claim that may be made by its manufacturer, is not guaranteed or endorsed by the publisher.
References
Abourehab, M. A. S., Pramanik, S., Abdelgawad, M. A., Abualsoud, B. M., Kadi, A., Ansari, M. J., et al. (2022). Recent advances of chitosan formulations in biomedical applications. Int. J. Mol. Sci. 23, 10975. doi:10.3390/ijms231810975
Albalawi, F., Hussein, M. Z., Fakurazi, S., and Masarudin, M. J. (2023). Fabrication and characterization of nanodelivery platform based on chitosan to improve the anticancer outcome of sorafenib in hepatocellular carcinoma. Sci. Rep. 13, 12180. doi:10.1038/s41598-023-38054-4
Alok, A., Singh, I. D., Singh, S., Kishore, M., and Jha, P. C. (2015). Curcumin - pharmacological actions and its role in oral submucous fibrosis: a review. J. Clin. diagnostic Res. JCDR 9, Ze01–3. doi:10.7860/JCDR/2015/13857.6552
Ando, Y., Kawaoka, T., Amioka, K., Naruto, K., Ogawa, Y., Yoshikawa, Y., et al. (2021). Efficacy and safety of lenvatinib-transcatheter arterial chemoembolization sequential therapy for patients with intermediate-stage hepatocellular carcinoma. Oncology 99, 507–517. doi:10.1159/000515865
Arafa, K. K., Hamzawy, M. A., Mousa, S. A., and El-Sherbiny, I. M. (2022). Mitochondria-targeted alginate/triphenylphosphonium-grafted-chitosan for treatment of hepatocellular carcinoma. RSC Adv. 12, 21690–21703. doi:10.1039/d2ra03240f
Ardean, C., Davidescu, C. M., Nemeş, N. S., Negrea, A., Ciopec, M., Duteanu, N., et al. (2021). Factors influencing the antibacterial activity of chitosan and chitosan modified by functionalization. Int. J. Mol. Sci. 22, 7449. doi:10.3390/ijms22147449
Atmaca, H., Oguz, F., and Ilhan, S. (2024). Chitosan in cancer therapy: a dual role as a therapeutic agent and drug delivery system. Z Naturforsch C J. Biosci. 79, 95–105. doi:10.1515/znc-2023-0148
Balagangadharan, K., Dhivya, S., and Selvamurugan, N. (2017). Chitosan based nanofibers in bone tissue engineering. Int. J. Biol. Macromol. 104, 1372–1382. doi:10.1016/j.ijbiomac.2016.12.046
Bashir, S. M., Ahmed Rather, G., Patrício, A., Haq, Z., Sheikh, A. A., Shah, M., et al. (2022). Chitosan nanoparticles: a versatile platform for biomedical applications. Mater. Basel, Switz. 15, 6521. doi:10.3390/ma15196521
Bhatt, A., and Wu, J. (2023). Immunotherapy for recurrent hepatocellular carcinoma. World J. gastroenterology 29, 2261–2271. doi:10.3748/wjg.v29.i15.2261
Bissell, M. J., and Hines, W. C. (2011). Why don’t we get more cancer? A proposed role of the microenvironment in restraining cancer progression. Nat. Med. 17, 320–329. doi:10.1038/nm.2328
Bohn, T., Rapp, S., Luther, N., Klein, M., Bruehl, T.-J., Kojima, N., et al. (2018). Tumor immunoevasion via acidosis-dependent induction of regulatory tumor-associated macrophages. Nat. Immunol. 19, 1319–1329. doi:10.1038/s41590-018-0226-8
Chang, W. C., Hsu, H. H., Chiu, S. H., Huang, W. Y., Lo, C. H., Lin, H. H., et al. (2021). Transcatheter arterial chemoembolization with drug-eluting beads for the treatment of hepatocellular carcinoma: recommended selection for small-caliber. J. Hepatocell. carcinoma 8, 937–949. doi:10.2147/jhc.s319920
Chen, C., Wang, Z., Ding, Y., and Qin, Y. (2023). Tumor microenvironment-mediated immune evasion in hepatocellular carcinoma. Front. Immunol. 14, 1133308. doi:10.3389/fimmu.2023.1133308
Chen, G., Liu, Y., Shi, G., Luo, Y., Fu, S., Yang, A., et al. (2022b). Preparation of polydopamine-modified celastrol nanosuspension and its anti-liver cancer activity in vitro. J. Drug Deliv. Sci. Technol. 75, 103630. doi:10.1016/j.jddst.2022.103630
Chen, L., Hong, W., Duan, S., Li, Y., Wang, J., and Zhu, J. (2022a). Graphene quantum dots mediated magnetic chitosan drug delivery nanosystems for targeting synergistic photothermal-chemotherapy of hepatocellular carcinoma. Cancer Biol. Ther. 23, 281–293. doi:10.1080/15384047.2022.2054249
Chi, D. C., Brogan, F., Turenne, I., Zelonis, S., Schwartz, L., and Saif, M. W. (2012). Gemcitabine-induced pulmonary toxicity. Anticancer Res. 32, 4147–4149.
Chiang, D. Y., Villanueva, A., Hoshida, Y., Peix, J., Newell, P., Minguez, B., et al. (2008). Focal gains of VEGFA and molecular classification of hepatocellular carcinoma. Cancer Res. 68, 6779–6788. doi:10.1158/0008-5472.CAN-08-0742
Chidambaranathan-Reghupaty, S., Fisher, P. B., and Sarkar, D. (2021). Hepatocellular carcinoma (HCC): epidemiology, etiology and molecular classification. Adv. cancer Res. 149, 1–61. doi:10.1016/bs.acr.2020.10.001
Chipuk, J. E., Bouchier-Hayes, L., and Green, D. R. (2006). Mitochondrial outer membrane permeabilization during apoptosis: the innocent bystander scenario. Cell Death Differ. 13, 1396–1402. doi:10.1038/sj.cdd.4401963
Clemente, J. C., Ursell, L. K., Parfrey, L. W., and Knight, R. (2012). The impact of the gut microbiota on human health: an integrative view. Cell 148, 1258–1270. doi:10.1016/j.cell.2012.01.035
Coradini, D., and Speranza, A. (2005). Histone deacetylase inhibitors for treatment of hepatocellular carcinoma. Acta Pharmacol. Sin. 26, 1025–1033. doi:10.1111/j.1745-7254.2005.00195.x
Denk, D., and Greten, F. R. (2022). Inflammation: the incubator of the tumor microenvironment. Trends Cancer 8, 901–914. doi:10.1016/j.trecan.2022.07.002
Deryugina, E. I., and Quigley, J. P. (2006). Matrix metalloproteinases and tumor metastasis. Cancer Metastasis Rev. 25, 9–34. doi:10.1007/s10555-006-7886-9
Desert, R., Chen, W., Ge, X., Viel, R., Han, H., Athavale, D., et al. (2023). Hepatocellular carcinomas, exhibiting intratumor fibrosis, express cancer-specific extracellular matrix remodeling and WNT/TGFB signatures, associated with poor outcome. Hepatol. Baltim. Md 78, 741–757. doi:10.1097/HEP.0000000000000362
Dong, X., Li, R., Xiu, P., Dong, X., Xu, Z., Zhai, B., et al. (2014). Meloxicam executes its antitumor effects against hepatocellular carcinoma in COX-2- dependent and -independent pathways. PloS one 9, e92864. doi:10.1371/journal.pone.0092864
Duong, L. M., Cai, H., Shrubsole, M. J., Bailey, C. E., Idrees, K., and Shu, X. O. (2022). Outcomes of robotic-assisted liver surgery versus laparoscopic liver surgery for treatment of stage I hepatocellular carcinoma. Cancer 128, 762–769. doi:10.1002/cncr.33979
El-Araby, A., Janati, W., Ullah, R., Ercisli, S., and Errachidi, F. (2024). Chitosan, chitosan derivatives, and chitosan-based nanocomposites: eco-friendly materials for advanced applications (a review). Front. Chem. 11, 1327426–1333389. doi:10.3389/fchem.2023.1327426
Fan, X., Wu, H., Zhao, L., and Guo, X. (2021). A poly-chitosan and cis-platinum conjugated composite nanoparticle system for liver cancer therapy. J. Biomed. Nanotechnol. 17, 1726–1734. doi:10.1166/jbn.2021.3157
Fan, Z., Zhou, P., Jin, B., Li, G., Feng, L., Zhuang, C., et al. (2023). Recent therapeutics in hepatocellular carcinoma. Am. J. cancer Res. 13, 261–275.
Gali-Muhtasib, H., Hmadi, R., Kareh, M., Tohme, R., and Darwiche, N. (2015). Cell death mechanisms of plant-derived anticancer drugs: beyond apoptosis. Apoptosis 20, 1531–1562. doi:10.1007/s10495-015-1169-2
Gao, J., Zhang, Y., Liu, X., Wu, X., Huang, L., and Gao, W. (2021). Triptolide: pharmacological spectrum, biosynthesis, chemical synthesis and derivatives. Theranostics 11, 7199–7221. doi:10.7150/thno.57745
Gorini, S., De Angelis, A., Berrino, L., Malara, N., Rosano, G., and Ferraro, E. (2018). Chemotherapeutic drugs and mitochondrial dysfunction: focus on doxorubicin, trastuzumab, and sunitinib. Oxidative Med. Cell. Longev. 2018, 7582730. doi:10.1155/2018/7582730
Greten, F. R., and Grivennikov, S. I. (2019). Inflammation and cancer: triggers, mechanisms, and consequences. Immunity 51, 27–41. doi:10.1016/j.immuni.2019.06.025
Hallmann, L., and Gerngroß, M.-D. (2022). Chitosan and its application in dental implantology. J. Stomatology, Oral Maxillofac. Surg. 123, e701–e707. doi:10.1016/j.jormas.2022.02.006
Hamman, J. H. (2010). Chitosan based polyelectrolyte complexes as potential carrier materials in drug delivery systems. Mar. drugs 8, 1305–1322. doi:10.3390/md8041305
Hanafy, N. A. N., Sheashaa, R. F., Moussa, E. A., and Mahfouz, M. E. (2023). Potential of curcumin and niacin-loaded targeted chitosan coated liposomes to activate autophagy in hepatocellular carcinoma cells: an in vitro evaluation in HePG2 cell line. Int. J. Biol. Macromol. 245, 125572. doi:10.1016/j.ijbiomac.2023.125572
Hanahan, D. (2022). Hallmarks of cancer: new dimensions. Cancer Discov. 12, 31–46. doi:10.1158/2159-8290.CD-21-1059
Harish Prashanth, K. V., and Tharanathan, R. N. (2007). Chitin/chitosan: modifications and their unlimited application potential—an overview. Trends Food Sci. Technol. 18, 117–131. doi:10.1016/j.tifs.2006.10.022
Hatting, M., Spannbauer, M., Peng, J., Al Masaoudi, M., Sellge, G., Nevzorova, Y. A., et al. (2015). Lack of gp130 expression in hepatocytes attenuates tumor progression in the DEN model. Cell death Dis. 6, e1667. doi:10.1038/cddis.2014.590
He, L., Yao, L., Liu, F., Qin, B., Song, R., and Huang, W. (2010). Magnetic Fe3O4@chitosan nanoparticle: synthesis, characterization and application as catalyst carrier. J. Nanosci. Nanotechnol. 10, 6348–6355. doi:10.1166/jnn.2010.2549
Hefnawy, A., Khalil, I. H., Arafa, K., Emara, M., and El-Sherbiny, I. M. (2020). Dual-ligand functionalized core-shell chitosan-based nanocarrier for hepatocellular carcinoma-targeted drug delivery. Int. J. nanomedicine 15, 821–837. doi:10.2147/IJN.S240359
Herdiana, Y., Wathoni, N., Gozali, D., Shamsuddin, S., and Muchtaridi, M. (2023). Chitosan-based nano-smart drug delivery system in breast cancer therapy. Pharmaceutics 15, 879. doi:10.3390/pharmaceutics15030879
Hewlings, S. J., and Kalman, D. S. (2017). Curcumin: a review of its effects on human health. Foods 6, 92. doi:10.3390/foods6100092
Huang, K. X., Zhou, L. Y., Chen, J. Q., Peng, N., Chen, H. X., Gu, H. Z., et al. (2023). Applications and perspectives of quaternized cellulose, chitin and chitosan: a review. Int. J. Biol. Macromol. 242, 124990. doi:10.1016/j.ijbiomac.2023.124990
Islam, N., Hoque, M., and Taharat, S. F. (2022). Recent advances in extraction of chitin and chitosan. World J. Microbiol. Biotechnol. 39, 28. doi:10.1007/s11274-022-03468-1
Jędrzak, A., Grześkowiak, B. F., Golba, K., Coy, E., Synoradzki, K., Jurga, S., et al. (2020). Magnetite nanoparticles and spheres for chemo- and photothermal therapy of hepatocellular carcinoma in vitro. Int. J. nanomedicine 15, 7923–7936. doi:10.2147/IJN.S257142
Jiang, Y., Yu, X., Su, C., Zhao, L., and Shi, Y. (2019). Chitosan nanoparticles induced the antitumor effect in hepatocellular carcinoma cells by regulating ROS-mediated mitochondrial damage and endoplasmic reticulum stress. Artif. Cells, Nanomedicine, Biotechnol. 47, 747–756. doi:10.1080/21691401.2019.1577876
Jiang, Z., Han, B., Li, H., Yang, Y., and Liu, W. (2015). Carboxymethyl chitosan represses tumor angiogenesis in vitro and in vivo. Carbohydr. Polym. 129, 1–8. doi:10.1016/j.carbpol.2015.04.040
Juaid, N., Amin, A., Abdalla, A., Reese, K., Alamri, Z., Moulay, M., et al. (2021). Anti-hepatocellular carcinoma biomolecules: molecular targets insights. Int. J. Mol. Sci. 22, 10774. doi:10.3390/ijms221910774
Karimi, K., Mojtabavi, S., Tehrany, P. M., Nejad, M. M., Rezaee, A., Mohtashamian, S., et al. (2023). Chitosan-based nanoscale delivery systems in hepatocellular carcinoma: versatile bio-platform with theranostic application. Int. J. Biol. Macromol. 242, 124935. doi:10.1016/j.ijbiomac.2023.124935
Kennel, K. B., Bozlar, M., De Valk, A. F., and Greten, F. R. (2023). Cancer-associated fibroblasts in inflammation and antitumor immunity. Clin. cancer Res. official J. Am. Assoc. Cancer Res. 29, 1009–1016. doi:10.1158/1078-0432.CCR-22-1031
Kern, M. A., Schöneweiss, M. M., Sahi, D., Bahlo, M., Haugg, A. M., Kasper, H. U., et al. (2004). Cyclooxygenase-2 inhibitors suppress the growth of human hepatocellular carcinoma implants in nude mice. Carcinogenesis 25, 1193–1199. doi:10.1093/carcin/bgh110
Kim, Y., Zharkinbekov, Z., Raziyeva, K., Tabyldiyeva, L., Berikova, K., Zhumagul, D., et al. (2023). Chitosan-based biomaterials for tissue regeneration. Pharmaceutics 15, 807. doi:10.3390/pharmaceutics15030807
Kong, Z. L., Kuo, H. P., Johnson, A., Wu, L. C., and Chang, K. L. B. (2019). Curcumin-loaded mesoporous silica nanoparticles markedly enhanced cytotoxicity in hepatocellular carcinoma cells. Int. J. Mol. Sci. 20, 2918. doi:10.3390/ijms20122918
Kruse, B., Buzzai, A. C., Shridhar, N., Braun, A. D., Gellert, S., Knauth, K., et al. (2023). CD4(+) T cell-induced inflammatory cell death controls immune-evasive tumours. Nature 618, 1033–1040. doi:10.1038/s41586-023-06199-x
Kulik, L., and El-Serag, H. B. (2019). Epidemiology and management of hepatocellular carcinoma. Gastroenterology 156, 477–491. doi:10.1053/j.gastro.2018.08.065
Kuo, C. L., Ponneri Babuharisankar, A., Lin, Y. C., Lien, H. W., Lo, Y. K., Chou, H. Y., et al. (2022). Mitochondrial oxidative stress in the tumor microenvironment and cancer immunoescape: foe or friend? J. Biomed. Sci. 29, 74. doi:10.1186/s12929-022-00859-2
Li, J., Chen, L., Yu, P., Liu, B., Zhu, J., and Yang, Y. (2014). Telmisartan exerts anti-tumor effects by activating peroxisome proliferator-activated receptor-γ in human lung adenocarcinoma A549 cells. Mol. Basel, Switz. 19, 2862–2876. doi:10.3390/molecules19032862
Li, M., Wang, Y., Jiang, S., Gao, Y., Zhang, W., Hu, S., et al. (2019). Biodistribution and biocompatibility of glycyrrhetinic acid and galactose-modified chitosan nanoparticles as a novel targeting vehicle for hepatocellular carcinoma. Nanomedicine Nanotechnol. Biol. Med. 15, 145–161. doi:10.2217/nnm-2018-0455
Li, Y.-R., Lin, C.-C., Huang, C.-Y., Wong, Y.-H., Hsieh, C.-H., Wu, H.-W., et al. (2017). Study of the inhibitory effects on TNF-α-induced NF-κB activation of IMD0354 analogs. Chem. Biol. Drug Des. 90, 1307–1311. doi:10.1111/cbdd.13032
Liu, X., Hu, Y., Li, C., Chen, J., Liu, X., Shen, Y., et al. (2023). Overexpression of YEATS2 remodels the extracellular matrix to promote hepatocellular carcinoma progression via the PI3K/AKT pathway. Cancers 15, 1850. doi:10.3390/cancers15061850
Lu, P., Weaver, V. M., and Werb, Z. (2012). The extracellular matrix: a dynamic niche in cancer progression. J. Cell Biol. 196, 395–406. doi:10.1083/jcb.201102147
Ma, J. Q., Liu, C. M., and Yang, W. (2018). Protective effect of rutin against carbon tetrachloride-induced oxidative stress, inflammation and apoptosis in mouse kidney associated with the ceramide, MAPKs, p53 and calpain activities. Chemico-biological Interact. 286, 26–33. doi:10.1016/j.cbi.2018.03.003
Mabrouk Zayed, M. M., Sahyon, H. A., Hanafy, N. A. N., and El-Kemary, M. A. (2022). The effect of encapsulated Apigenin nanoparticles on HePG-2 cells through regulation of P53. Pharmaceutics 14, 1160. doi:10.3390/pharmaceutics14061160
Mallakuntla, M. K., Penugurti, V., Manavathi, B., and Podile, A. R. (2021). Chitooligosaccharides induce apoptosis in human breast cancer cells. Carbohydr. Polym. Technol. Appl. 2, 100077. doi:10.1016/j.carpta.2021.100077
Manna, S., Seth, A., Gupta, P., Nandi, G., Dutta, R., Jana, S., et al. (2023). Chitosan derivatives as carriers for drug delivery and biomedical applications. ACS biomaterials Sci. Eng. 9, 2181–2202. doi:10.1021/acsbiomaterials.2c01297
Matloob, A., Ayub, H., Mohsin, M., Ambreen, S., Khan, F. A., Oranab, S., et al. (2023). A review on edible coatings and films: advances, composition, production methods, and safety concerns. ACS Omega 8, 28932–28944. doi:10.1021/acsomega.3c03459
Meijer, K., de Vos, P., and Priebe, M. G. (2010). Butyrate and other short-chain fatty acids as modulators of immunity: what relevance for health? Curr. Opin. Clin. Nutr. Metabolic Care 13, 715–721. doi:10.1097/MCO.0b013e32833eebe5
Menter, D. G., and Bresalier, R. S. (2023). An aspirin a day: new pharmacological developments and cancer chemoprevention. Annu. Rev. Pharmacol. Toxicol. 63, 165–186. doi:10.1146/annurev-pharmtox-052020-023107
Miura, S., Mitsui, K., Heishi, T., Shukunami, C., Sekiguchi, K., Kondo, J., et al. (2010). Impairment of VEGF-A-stimulated lamellipodial extensions and motility of vascular endothelial cells by chondromodulin-I, a cartilage-derived angiogenesis inhibitor. Exp. Cell Res. 316, 775–788. doi:10.1016/j.yexcr.2009.12.009
Mossalam, M., Dixon, A. S., and Lim, C. S. (2010). Controlling subcellular delivery to optimize therapeutic effect. Ther. Deliv. 1, 169–193. doi:10.4155/tde.10.8
Nair, A. B., Shah, J., Al-Dhubiab, B. E., Patel, S. S., Morsy, M. A., Patel, V., et al. (2019). Development of asialoglycoprotein receptor-targeted nanoparticles for selective delivery of gemcitabine to hepatocellular carcinoma. Mol. Basel, Switz. 24, 4566. doi:10.3390/molecules24244566
Nasr, M., Kira, A. Y., Saber, S., Essa, E. A., and El-Gizawy, S. A. (2023). Lactosylated chitosan nanoparticles potentiate the anticancer effects of telmisartan in vitro and in a N-Nitrosodiethylamine-Induced mice model of hepatocellular carcinoma. Mol. Pharm. 20, 4758–4769. doi:10.1021/acs.molpharmaceut.3c00542
Park, H. J., Kim, M. N., Kim, J. G., Bae, Y. H., Bae, M. K., Wee, H. J., et al. (2007). Up-regulation of VEGF expression by NGF that enhances reparative angiogenesis during thymic regeneration in adult rat. Biochimica biophysica acta 1773, 1462–1472. doi:10.1016/j.bbamcr.2007.05.006
Patel, S., and Goyal, A. (2017). Chitin and chitinase: role in pathogenicity, allergenicity and health. Int. J. Biol. Macromol. 97, 331–338. doi:10.1016/j.ijbiomac.2017.01.042
Perillo, B., Di Donato, M., Pezone, A., Di Zazzo, E., Giovannelli, P., Galasso, G., et al. (2020). ROS in cancer therapy: the bright side of the moon. Exp. Mol. Med. 52, 192–203. doi:10.1038/s12276-020-0384-2
Piras, A. M., Esin, S., Benedetti, A., Maisetta, G., Fabiano, A., Zambito, Y., et al. (2019). Antibacterial, antibiofilm, and antiadhesive properties of different quaternized chitosan derivatives. Int. J. Mol. Sci. 20, 6297. doi:10.3390/ijms20246297
Priya, K., Vijayakumar, M., and Janani, B. (2020). Chitosan-mediated synthesis of biogenic silver nanoparticles (AgNPs), nanoparticle characterisation and in vitro assessment of anticancer activity in human hepatocellular carcinoma HepG2 cells. Int. J. Biol. Macromol. 149, 844–852. doi:10.1016/j.ijbiomac.2020.02.007
Qin, J., Zhang, J., Fan, G., Wang, X., Zhang, Y., Wang, L., et al. (2022). Cold atmospheric plasma activates selective photothermal therapy of cancer. Mol. Basel, Switz. 27, 5941. doi:10.3390/molecules27185941
Quagliariello, V., Masarone, M., Armenia, E., Giudice, A., Barbarisi, M., Caraglia, M., et al. (2019). Chitosan-coated liposomes loaded with butyric acid demonstrate anticancer and anti-inflammatory activity in human hepatoma HepG2 cells. Oncol. Rep. 41, 1476–1486. doi:10.3892/or.2018.6932
Radwan, R. R., and Ali, H. E. (2021). Radiation-synthesis of chitosan/poly (acrylic acid) nanogel for improving the antitumor potential of rutin in hepatocellular carcinoma. Drug Deliv. Transl. Res. 11, 261–278. doi:10.1007/s13346-020-00792-7
Rahib, L., Smith, B. D., Aizenberg, R., Rosenzweig, A. B., Fleshman, J. M., and Matrisian, L. M. (2014). Projecting cancer incidence and deaths to 2030: the unexpected burden of thyroid, liver, and pancreas cancers in the United States. Cancer Res. 74, 2913–2921. doi:10.1158/0008-5472.CAN-14-0155
Reichman, T. W., Bhati, C. S., and Battula, N. R. (2019). Obtaining optimal long-term outcomes from liver transplantation for hepatocellular cancer. Dig. Dis. Sci. 64, 976–984. doi:10.1007/s10620-019-05550-5
Romero, D. (2016). Immunotherapy: PD-1 says goodbye, TIM-3 says hello. Nat. Rev. Clin. Oncol. 13, 202–203. doi:10.1038/nrclinonc.2016.40
Roy, A. M., Iyer, R., and Chakraborty, S. (2023). The extracellular matrix in hepatocellular carcinoma: mechanisms and therapeutic vulnerability. Cell Rep. Med. 4, 101170. doi:10.1016/j.xcrm.2023.101170
Saran, E. Y., Cavusoglu, S., Alpaslan, D., Eren, E., Yilmaz, N., and Uzun, Y. (2022). Effect of egg white protein and agar-agar on quality of button mushrooms (Agaricus bisporus) during cold storage. Turkish J. Agric. For. 46 (2), 173–181. doi:10.55730/1300-011X.2969
Sathiyaseelan, A., Saravanakumar, K., and Wang, M.-H. (2022). Cerium oxide decorated 5-fluorouracil loaded chitosan nanoparticles for treatment of hepatocellular carcinoma. Int. J. Biol. Macromol. 216, 52–64. doi:10.1016/j.ijbiomac.2022.06.112
Satitsri, S., and Muanprasat, C. (2020). Chitin and chitosan derivatives as biomaterial resources for biological and biomedical applications. Mol. Basel, Switz. 25, 5961. doi:10.3390/molecules25245961
Shao, Z., Liu, X., Peng, C., Wang, L., and Xu, D. (2021). Combination of transcatheter arterial chemoembolization and portal vein embolization for patients with hepatocellular carcinoma: a review. World J. Surg. Oncol. 19, 293. doi:10.1186/s12957-021-02401-4
Sica, A., Larghi, P., Mancino, A., Rubino, L., Porta, C., Totaro, M. G., et al. (2008). Macrophage polarization in tumour progression. Seminars Cancer Biol. 18, 349–355. doi:10.1016/j.semcancer.2008.03.004
Siegel, R. L., Miller, K. D., Fuchs, H. E., and Jemal, A. (2022). Cancer statistics. CA A Cancer J. Clin. 72, 7–30. doi:10.3322/caac.21332
Siposova, K., Huntosova, V., Garcarova, I., Shlapa, Y., Timashkov, I., Belous, A., et al. (2022). Dual-functional antioxidant and antiamyloid cerium oxide nanoparticles fabricated by controlled synthesis in water-alcohol solutions. Biomedicines 10, 942. doi:10.3390/biomedicines10050942
Smith, R. A. J., Hartley, R. C., and Murphy, M. P. (2011). Mitochondria-targeted small molecule therapeutics and probes. Antioxidants Redox Signal. 15, 3021–3038. doi:10.1089/ars.2011.3969
Snezhkina, A. V., Kudryavtseva, A. V., Kardymon, O. L., Savvateeva, M. V., Melnikova, N. V., Krasnov, G. S., et al. (2019). ROS generation and antioxidant defense systems in normal and malignant cells. Oxidative Med. Cell. Longev. 2019, 6175804. doi:10.1155/2019/6175804
Song, C., Zhang, J., Wen, R., Li, Q., Zhou, J., Xiaoli, L., et al. (2022). Improved anti-hepatocellular carcinoma effect by enhanced Co-delivery of Tim-3 siRNA and sorafenib via multiple pH triggered drug-eluting nanoparticles. Bio 16, 100350. doi:10.1016/j.mtbio.2022.100350
Song, H., Su, C., Cui, W., Zhu, B., Liu, L., Chen, Z., et al. (2013). Folic acid-chitosan conjugated nanoparticles for improving tumor-targeted drug delivery. BioMed Res. Int. 2013, 723158. doi:10.1155/2013/723158
Spottiswoode, N., Pet, D., Kim, A., Gruenberg, K., Shah, M., Ramachandran, A., et al. (2023). Successful treatment of balamuthia mandrillaris granulomatous amebic encephalitis with nitroxoline. Emerg. Infect. Dis. 29, 197–201. doi:10.3201/eid2901.221531
Sugawara, Y., and Hibi, T. (2021). Surgical treatment of hepatocellular carcinoma. Biosci. trends 15, 138–141. doi:10.5582/bst.2021.01094
Szymańska, E., and Winnicka, K. (2015). Stability of chitosan-a challenge for pharmaceutical and biomedical applications. Mar. drugs 13, 1819–1846. doi:10.3390/md13041819
Taniguchi, K., and Karin, M. (2018). NF-κB, inflammation, immunity and cancer: coming of age. Nat. Rev. Immunol. 18, 309–324. doi:10.1038/nri.2017.142
Taokaew, S., and Kriangkrai, W. (2023). Chitinase-Assisted bioconversion of chitinous waste for development of value-added chito-oligosaccharides products. Biology 12, 87. doi:10.3390/biology12010087
Thambiliyagodage, C., Jayanetti, M., Mendis, A., Ekanayake, G., Liyanaarachchi, H., and Vigneswaran, S. (2023). Recent advances in chitosan-based applications-A review. Mater. Basel, Switz. 16, 2073. doi:10.3390/ma16052073
Torchilin, V. (2011). Tumor delivery of macromolecular drugs based on the EPR effect. Adv. Drug Deliv. Rev. 63, 131–135. doi:10.1016/j.addr.2010.03.011
U, A., Shetty, S., Kulkarni, S. D., B, H. K., Pai, K. S. R., A, J. M., et al. (2022). Anticancer therapeutic potential of phosphorylated galactosylated chitosan against N-nitrosodiethyl amine-induced hepatocarcinogenesis. Archives Biochem. Biophysics 728, 109375. doi:10.1016/j.abb.2022.109375
Unal, S., Sabir Kucukbasmaci, F., and Sabir, A. (2023). Some physicochemical and bioactive features of organically grown blackberry fruits(Rubus fructicosus L.) as influenced by postharvest UV-C and chitosan treatments. Turkish J. Agric. For. 47 (6), 907–917. doi:10.55730/1300-011X.3136
Valderrama-Treviño, A. I., Barrera-Mera, B., Ceballos-Villalva, J. C., and Montalvo-Javé, E. E. (2017). Hepatic metastasis from colorectal cancer. Euroasian J. hepato-gastroenterology 7, 166–175. doi:10.5005/jp-journals-10018-1241
Varshosaz, J., Fard, M. M., Mirian, M., and Hassanzadeh, F. (2020). Targeted nanoparticles for Co-delivery of 5-FU and nitroxoline, a cathepsin B inhibitor, in HepG2 cells of hepatocellular carcinoma. Anti-cancer agents Med. Chem. 20, 346–358. doi:10.2174/1871520619666190930124746
Villanueva, A. (2019). Hepatocellular carcinoma. N. Engl. J. Med. 380, 1450–1462. doi:10.1056/NEJMra1713263
Vogel, A., Meyer, T., Sapisochin, G., Salem, R., and Saborowski, A. (2022). Hepatocellular carcinoma. Lancet London, Engl. 400, 1345–1362. doi:10.1016/S0140-6736(22)01200-4
Wang, G., Xiao, Q., Wu, Y., Wei, Y.-j., Jing, Y., Cao, X.-r., et al. (2019a). Design and synthesis of novel celastrol derivative and its antitumor activity in hepatoma cells and antiangiogenic activity in zebrafish. J. Cell. Physiology 234, 16431–16446. doi:10.1002/jcp.28312
Wang, P., Shen, Y., and Zhao, L. (2020). Chitosan nanoparticles loaded with aspirin and 5-fluororacil enable synergistic antitumour activity through the modulation of NF-κB/COX-2 signalling pathway. IET nanobiotechnology 14, 479–484. doi:10.1049/iet-nbt.2020.0002
Wang, T., Zhang, J., Hou, T., Yin, X., and Zhang, N. (2019b). Selective targeting of tumor cells and tumor associated macrophages separately by twin-like core-shell nanoparticles for enhanced tumor-localized chemoimmunotherapy. Nanoscale 11, 13934–13946. doi:10.1039/c9nr03374b
Wang, Z., Guo, W., Kuang, X., Hou, S., and Liu, H. (2017). Nanopreparations for mitochondria targeting drug delivery system: current strategies and future prospective. Asian J. Pharm. Sci. 12, 498–508. doi:10.1016/j.ajps.2017.05.006
Wilken, R., Veena, M. S., Wang, M. B., and Srivatsan, E. S. (2011). Curcumin: a review of anti-cancer properties and therapeutic activity in head and neck squamous cell carcinoma. Mol. Cancer 10, 12. doi:10.1186/1476-4598-10-12
Xia, Y., Yang, R., Zhu, J., Wang, H., Li, Y., Fan, J., et al. (2022a). Engineered nanomaterials trigger abscopal effect in immunotherapy of metastatic cancers. Front. Bioeng. Biotechnol. 10, 890257. doi:10.3389/fbioe.2022.890257
Xia, Y. L., Yang, R. H., Wang, H. Y., Li, Y. H., and Fu, C. F. (2022b). Application of chitosan-based materials in surgical or postoperative hemostasis. Front. Mater. 9. doi:10.3389/fmats.2022.994265
Xin, Y., Yin, M., Zhao, L., Meng, F., and Luo, L. (2017). Recent progress on nanoparticle-based drug delivery systems for cancer therapy. Cancer Biol. Med. 14, 228–241. doi:10.20892/j.issn.2095-3941.2017.0052
Xu, B., Xu, Y., Su, G., Zhu, H., and Zong, L. (2018). A multifunctional nanoparticle constructed with a detachable albumin outer shell and a redox-sensitive inner core for efficient siRNA delivery to hepatocellular carcinoma cells. J. drug Target. 26, 941–954. doi:10.1080/1061186X.2018.1455840
Xu, L., Yang, H., Yan, M., and Li, W. (2022). Matrix metalloproteinase 1 is a poor prognostic biomarker for patients with hepatocellular carcinoma. Clin. Exp. Med. 23, 2065–2083. doi:10.1007/s10238-022-00897-y
Yan, X., Qi, M., Li, P., Zhan, Y., and Shao, H. (2017). Apigenin in cancer therapy: anti-cancer effects and mechanisms of action. Cell and Biosci. 7, 50. doi:10.1186/s13578-017-0179-x
Yao, Y., Wang, T., Liu, Y., and Zhang, N. (2019). Co-delivery of sorafenib and VEGF-siRNA via pH-sensitive liposomes for the synergistic treatment of hepatocellular carcinoma. Artif. Cells Nanomed Biotechnol. 47, 1374–1383. doi:10.1080/21691401.2019.1596943
Yu, J.-M., Li, W.-D., Lu, L., Zhou, X.-Y., Wang, D.-Y., Li, H.-M., et al. (2014). Preparation and characterization of galactosylated glycol chitosan micelles and its potential use for hepatoma-targeting delivery of doxorubicin. J. Mater. Sci. Mater. Med. 25, 691–701. doi:10.1007/s10856-013-5109-9
Zaiki, Y., Iskandar, A., and Wong, T. W. (2023). Functionalized chitosan for cancer nano drug delivery. Biotechnol. Adv. 67, 108200. doi:10.1016/j.biotechadv.2023.108200
Zeng, R. W., Yong, J. N., Tan, D. J. H., Fu, C. E., Lim, W. H., Xiao, J., et al. (2023). Meta-analysis: chemoprevention of hepatocellular carcinoma with statins, aspirin and metformin. Alimentary Pharmacol. Ther. 57, 600–609. doi:10.1111/apt.17371
Zhang, J., Li, C., Xue, Z.-Y., Cheng, H.-W., Huang, F.-W., Zhuo, R.-X., et al. (2011). Fabrication of lactobionic-loaded chitosan microcapsules as potential drug carriers targeting the liver. Acta biomater. 7, 1665–1673. doi:10.1016/j.actbio.2010.11.042
Zhang, W., Hu, X., Shen, Q., and Xing, D. (2019b). Mitochondria-specific drug release and reactive oxygen species burst induced by polyprodrug nanoreactors can enhance chemotherapy. Nat. Commun. 10, 1704. doi:10.1038/s41467-019-09566-3
Zhang, X., Xu, X., Wang, X., Lin, Y., Zheng, Y., Xu, W., et al. (2023). Hepatoma-targeting and reactive oxygen species-responsive chitosan-based polymeric micelles for delivery of celastrol. Carbohydr. Polym. 303, 120439. doi:10.1016/j.carbpol.2022.120439
Zhang, Y.-Q., Shen, Y., Liao, M.-M., Mao, X., Mi, G.-J., You, C., et al. (2019a). Galactosylated chitosan triptolide nanoparticles for overcoming hepatocellular carcinoma: enhanced therapeutic efficacy, low toxicity, and validated network regulatory mechanisms. Nanomedicine Nanotechnol. Biol. Med. 15, 86–97. doi:10.1016/j.nano.2018.09.002
Zhao, J., Xie, C., Wang, K., Takahashi, S., Krausz, K. W., Lu, D., et al. (2020). Comprehensive analysis of transcriptomics and metabolomics to understand triptolide-induced liver injury in mice. Toxicol. Lett. 333, 290–302. doi:10.1016/j.toxlet.2020.08.007
Zhao, L., Jiang, M., Xu, Z., Sun, F., Wu, X., Zhang, M., et al. (2022b). Selective thermotherapy of tumor by self-regulating photothermal conversion system. J. Colloid Interface Sci. 605, 752–765. doi:10.1016/j.jcis.2021.07.134
Zhao, L., Wang, X., Lou, H., Jiang, M., Wu, X., Qin, J., et al. (2022a). Buffet-style Cu(II) for enhance disulfiram-based cancer therapy. J. Colloid Interface Sci. 624, 734–746. doi:10.1016/j.jcis.2022.06.009
Zhou, W., Liu, K., Zeng, L., He, J., Gao, X., Gu, X., et al. (2022). Targeting VEGF-A/VEGFR2 Y949 signaling-mediated vascular permeability alleviates hypoxic pulmonary hypertension. Circulation 146, 1855–1881. doi:10.1161/CIRCULATIONAHA.122.061900
Keywords: biomaterials, chitosan, hepatocellular carcinoma, apoptosis, antioxidant, antiinflammatory, angiogenesis inhibition, extracellular matrix remodeling
Citation: Wang X, Yang Y, Zhao S, Wu D, Li L and Zhao Z (2024) Chitosan-based biomaterial delivery strategies for hepatocellular carcinoma. Front. Pharmacol. 15:1446030. doi: 10.3389/fphar.2024.1446030
Received: 08 June 2024; Accepted: 23 July 2024;
Published: 05 August 2024.
Edited by:
Awanish Mishra, National Institute of Pharmaceutical Education and Research, IndiaReviewed by:
Changfeng Fu, The First Hospital of Jilin University, ChinaVasudevarao Penugurti, Duke University, United States
Copyright © 2024 Wang, Yang, Zhao, Wu, Li and Zhao. This is an open-access article distributed under the terms of the Creative Commons Attribution License (CC BY). The use, distribution or reproduction in other forums is permitted, provided the original author(s) and the copyright owner(s) are credited and that the original publication in this journal is cited, in accordance with accepted academic practice. No use, distribution or reproduction is permitted which does not comply with these terms.
*Correspondence: Zhifeng Zhao, 77706841@cmu.edu.cn
†These authors have contributed equally to this work