- 1College of Traditional Chinese Medicine, Shandong University of Traditional Chinese Medicine, Jinan, Shandong, China
- 2Department of Breast and Thyroid Surgery, Affiliated Hospital of Shandong University of Traditional Chinese Medicine, Jinan, Shandong, China
In recent years, natural products have gradually become an important source for new drug development due to their advantages of multi-components, multi-targets, and good safety profiles. Psoralen, a furanocoumarin compound extracted from the traditional Chinese medicine psoralea corylifolia, is widely distributed among various plants. It has attracted widespread attention in the research community due to its pharmacological activities, including antitumor, anti-inflammatory, antioxidant, and neuroprotective effects. Studies have shown that psoralen has broad spectrum anti-tumor activities, offering resistance to malignant tumors such as breast cancer, liver cancer, glioma, and osteosarcoma, making it a natural, novel potential antitumor drug. Psoralen mainly exerts its antitumor effects by inhibiting tumor cell proliferation, inducing apoptosis, inhibiting tumor cell migration, and reversing multidrug resistance, presenting a wide application prospect in the field of antitumor therapy. With the deepening research on psoralea corylifolia, its safety has attracted attention, and reports on the hepatotoxicity of psoralen have gradually increased. Therefore, this article reviews recent studies on the mechanism of antitumor effects of psoralen and focuses on the molecular mechanisms of its hepatotoxicity, providing insights for the clinical development of low-toxicity, high-efficiency antitumor drugs and the safety of clinical medication.
1 Introduction
Cancer, characterized by its high recurrence rate and mortality, is one of the major diseases threatening human health and life. According to the latest data published in the official journal of the American Cancer Society, there are approximately 18.1 million new cancer cases and 9.6 million cancer-related deaths globally (Sung et al., 2021). Surgery, radiotherapy, and chemotherapy are currently the main clinical treatments for malignant tumors. However, they often cause severe adverse reactions in the body and tumor cell drug resistance, leading to unsatisfactory prognosis and therapeutic effects. In recent years, the effectiveness and safety of natural products in cancer treatment have attracted widespread attention from scholars around the world. Natural products can not only intervene in the process of tumor cells but also improve the adverse reactions caused by chemotherapy, enhancing the quality of life of cancer patients. Current research on cancer treatment mainly focuses on molecular targeting, immunotherapy, and the field of natural products monomers. Studies have shown that natural products has advantages in enhancing efficacy, reducing toxicity, improving quality of life, and extending survival periods in the prevention and treatment of tumors. Many effective anti-cancer drugs have been discovered and developed from natural products (Chopra and Dhingra, 2021).
Psoralen (PSO) is a furanocoumarin compound derived from the traditional Chinese herb psoralea corylifolia L., known for its strong pharmacological activity, low toxicity, good bioavailability, and therapeutic effectiveness (Thakur et al., 2020). It is the main active ingredient of Psoralea corylifolia L., with the molecular structure of 7H-Furo[3,2-g]chromen-7-one (molecular weight: 186.16; molecular formula: C3H6O3) (Sui et al., 2020), as shown in Figure 1. PSO is widely distributed in various plants and has shown great medicinal potential, attracting widespread attention in the research community. In recent years, natural products or patent medicines containing coumarins have been widely used in clinical treatments, and studies have found they possess multiple pharmacological activities, including antitumor (Wang et al., 2018), neuroprotective (Somani et al., 2015), anti-inflammatory (Du et al., 2020), and antioxidant effects (Seo et al., 2014), suitable for treating tumors, rheumatoid arthritis, leukemia, Alzheimer’s disease, and other conditions. This suggests a broad application prospect for PSO. With the increasing attention to the safety of PSO, the studies on liver toxicity of PSO have gradually increased in recent years, and there have been reports on clinical cases of liver toxicity. Long-term or excessive use of PSO or its compound will cause damage mainly caused by abnormal liver function, and its adverse reactions limit further clinical use. Therefore, the mechanism of hepatotoxicity of PSO was discussed and studied in order to provide some reference for the attenuation compatibility of PSO and the improvement of drug safety. Meanwhile through literature review, we found that several studies have focused on its pharmacological effects, yet a comprehensive and systematic review on the molecular mechanisms of PSO’s antitumor effects has not been conducted. Therefore, this article reviews recent studies on the antitumor mechanisms of PSO and summarizes its mechanisms of hepatotoxicity, aiming to provide references for the clinical development of low-toxicity, high-efficiency antitumor drugs and the safety of clinical medication.
2 Antitumor molecular mechanisms of PSO
2.1 Induction of tumor cell apoptosis
Apoptosis is a form of programmed cell death, serving as a critical physiological mechanism to limit the expansion of cell populations, thereby maintaining tissue homeostasis or eliminating potentially harmful cells (Morana et al., 2022). Promoting apoptosis has become one of the important strategies in antitumor therapy (Carneiro and El-Deiry, 2020). Apoptosis can be regulated through multiple pathways, including the endoplasmic reticulum stress-mediated apoptotic signaling pathway (Oakes and Papa, 2015). Under stress conditions, the endoplasmic reticulum environment and protein maturation are disrupted, leading to the accumulation of misfolded proteins and the formation of a typical stress response, known as the unfolded protein response (UPR) (Pandey et al., 2019). UPR protects cells from stress and helps them re-establish homeostasis, while prolonged endoplasmic reticulum stress activates UPR and induces apoptosis (Adams et al., 2019; Al-Hetty et al., 2023). Li et al. through in vitro studies, found that PSO treatment of MG-63 and U2OS osteosarcoma cells induced cell cycle arrest at the G0/G1 phase, promoting apoptosis in MG-63 and U2OS cells (Li and Tu, 2022). Further research revealed that PSO treatment resulted in elevated levels of ATF-6 and CHOP proteins, with a decrease in Bcl-2 protein levels, suggesting that PSO-induced apoptosis is related to endoplasmic reticulum stress. In summary, PSO activated endoplasmic reticulum stress in osteosarcoma cells, further inducing apoptosis. Wang et al. further explored the mechanism of action of PSO in inhibiting liver cancer cells through in vitro experiments (Wang X. et al., 2019). They confirmed that PSO could induce the production of unfolded proteins and cause endoplasmic reticulum stress in SMMC7721 liver cancer cells. The results showed that PSO significantly promoted the expression of GRP78 and GRP94, inducing the unfolded protein response and leading to liver cancer cell apoptosis. Further findings indicated that PSO could cause cell cycle arrest at the G1 phase, significantly induce endoplasmic reticulum stress in a dose-dependent and time-dependent manner, leading to liver cancer cell apoptosis and inhibiting liver cancer progression. This suggests that PSO may be a new therapeutic option for the prevention and treatment of hepatocellular carcinoma in the future. caspases are a class of cysteine proteases that have long been considered a key component of the apoptosis pathway (Song T. H. et al., 2019). the tumor suppressor p53 plays a crucial role in inhibiting tumor growth and inducing apoptosis, controlling cell death through interactions with other important apoptotic molecules, including members of the bcl-2 family (Vidal and Koff, 2000; Zehir et al., 2017; Tan et al., 2019). jiang et al. found that in psoralea corylifolia-treated smmc-7721 cells, caspase-3 activity increased in a dose-dependent manner, levels of p53 and bax proteins were elevated, while the expression of bcl-2 showed a dose-dependent decrease (Jiang and Xiong, 2014). These results indicate that PSO suppresses the growth of SMMC-7721 human liver cancer cells through a mechanism that induces apoptosis by regulating the activity of caspase-3 and the expression of p53 and Bcl-2/Bax proteins. Lu et al. (2014) discovered that PSO and isopsoralen have inhibitory effects on the growth of osteosarcoma xenografts in nude mice, inducing tumor cell apoptosis or necrosis without significant toxic side effects within the therapeutic dose range. This suggests that PSO, by regulating the activity of proteins such as caspase-3 to induce cancer cell apoptosis, represents a promising anticancer drug. Studies have discovered that apoptosis in CTCL (Cutaneous T-Cell Lymphoma) cells can be induced through a model combining treatment with PSO and long-wave ultraviolet light (Photochemotherapy with PSO and ultraviolet A, PUVA). PUVA treatment leads to G2/M cycle arrest and apoptosis in MyLa and HuT-78 cells, with an increase in the expression of pro-apoptotic mitochondrial genes Bax, BAK, PUMA, and a decrease in Bcl-2 expression. This suggests that interferon-α (IFN-α) enhances PUVA-induced apoptosis in skin lymphoma cell lines through the JAK1 pathway, demonstrating the synergistic effect of IFN-α (Liszewski et al., 2017). The study indicates that mitochondrial damage may occur in lymphoblastoid cell lines following PUVA treatment, leading to apoptosis (Canton et al., 2002). Viola et al. (2007) found that two PSO derivatives, 8-methoxyPSO (8-MOP) and the coumarin derivative angelicin, significantly induce apoptosis 24 h after UVA irradiation. Under UVA exposure, these compounds induce a significant decline in mitochondrial function and activate caspase-3, caspase-8, and caspase-9 (Viola et al., 2007). Sun et al. (2013) explored the regulatory role of PUVA on apoptosis and the apoptotic signaling pathway in human leukemia NB4 cells. It was found that the apoptosis rate of NB4 cells increased in a dose- and time-dependent manner with different concentrations of PSO under the influence of ultraviolet A (UVA) for 0 and 5 min, with the highest apoptosis rate at a concentration of 40 mg/mL PSO after 5 min of UVA exposure. This indicates that PUVA can induce apoptosis in NB4 cells and activate the Caspase-3 and Caspase-8 genes in vitro. Glioma is the most common primary brain tumor in adults. The World Health Organization classifies it into grades I, II, III, and IV (Miller et al., 2019), with higher grades indicating greater malignancy. Approximately 100,000 people are diagnosed with diffuse gliomas worldwide each year, and the mortality rate is extremely high (Bray et al., 2018; Molinaro et al., 2019). Unfortunately, almost all high-grade gliomas will recur, and currently, there are no reported treatment methods that are both safe and low in toxicity (Cloughesy et al., 2020). Wu et al. through network pharmacology methods, discovered that the genes PIK3CA, PIK3CB, and PIK3CG are highly related to the treatment of gliomas with PSO. Further in vitro studies found that PSO significantly promoted the early apoptosis of glioma U87 and U251 cells, and the apoptotic capability increased with the concentration of PSO, showing a significant concentration dependence (Wu et al., 2022). This suggests that PSO has a good in vitro anti-glioma effect. The mechanism of PSO inducing apoptosis is shown in Figure 2.
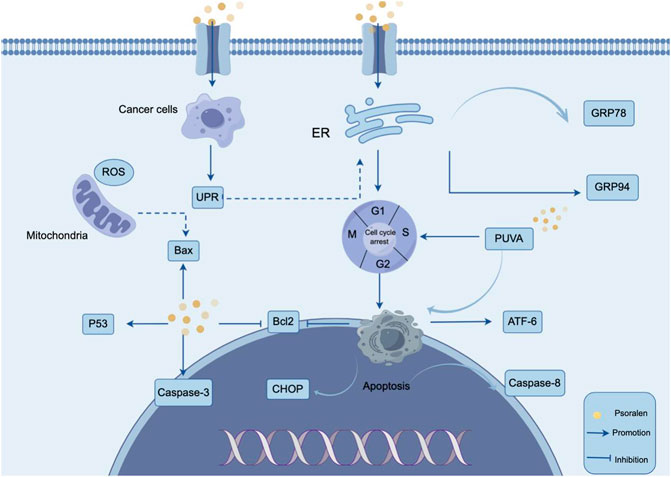
Figure 2. Mechanism of PSO inducing tumor cell apoptosis. PSO can lead to apoptosis of tumor cells by inducing cell cycle arrest and activating endoplasmic reticulum stress. PSO treatment resulted in elevated levels of ATF-6 and CHOP proteins, with a decrease in Bcl-2 protein levels.
2.2 Inhibition of tumor cell proliferation
Proliferation, as one of the fundamental cellular functions constituting life, is a process that occurs in a strictly controlled and orderly manner (Goodlad, 2017). Unrestricted proliferation is one of the three main characteristics of cancer cells, and inhibiting tumor cell proliferation is a common method in the clinical treatment of tumors. Osteosarcoma is the most common primary bone sarcoma and a leading cause of cancer death among children and adolescents. It accounts for 3%–6% of all childhood cancers, with its incidence among the most common cancers in children and adolescents second only to lymphomas and brain cancer (Zhu et al., 2016; Simpson and Brown, 2018). Li et al. through colony formation assays, showed that the number of colonies in MG-63 and U2OS cells treated with PSO was significantly reduced compared to the control group, indicating that PSO inhibits the colony formation of osteosarcoma cells (Li and Tu, 2022). This further suggests that PSO can inhibit the proliferation of osteosarcoma cells and suppress the progression of osteosarcoma, providing a molecular basis for the further development of PSO as a novel anticancer drug for the treatment of human osteosarcoma. Additionally, a team observed the inhibitory effect of PSO on the proliferation of liver cancer SMMC7721 cells through in vitro experiments and further explored its relationship with endoplasmic reticulum stress (Wang X. et al., 2019). The results suggest that PSO inhibits the proliferation of SMMC7721 cells by causing cell cycle arrest at the G1 phase. Moreover, it can cause endoplasmic reticulum expansion and dysfunction, thereby continuously inducing endoplasmic reticulum stress, leading to the apoptosis of liver cancer cells. Cell cycle dysregulation is a significant characteristic of tumor cells, especially in malignant transformation, making cell cycle proteins important targets of PSO’s anticancer effects. Overexpression of these proteins can shorten the G1 phase and advance cells into the S phase, leading to continuous proliferation and increased risk of carcinogenesis (Mills et al., 2017). Wang et al. were the first to systematically and detailedly describe the anticancer effects of PSO on human breast cancer MCF-7/ADR cells (Wang et al., 2016a). They discovered that PSO could block cells in the G1 and G2 phases, significantly reducing the proportion of cells in the S phase. However, there was no significant difference in the number of apoptotic cells after treating MCF-7/ADR cells with PSO for 48 h. This indicates that PSO inhibits the proliferation of MCF-7/ADR breast cancer cells by blocking the cell cycle. However, another study reported that PSO at 10 μM and 30 μM could promote the progression of MCF-7 cells from the G1 to the S phase, suggesting that PSO’s regulatory effects on the cell cycle of different tumor cells vary (Shen et al., 2007). The classic Wnt/β-catenin pathway plays a key role in regulating tumorigenesis by blocking the cell cycle at different stages. Wang et al. (2018) findings show that PSO inhibits cell proliferation in a dose-dependent manner by inducing G0/G1 phase block in MCF-7 cells and G2/M phase block in MDA-MB-231 cells. After treatment with PSO, the expression of Wnt/β-catenin target genes (such as CCND1 and c-Myc) in MCF-7 and MDA-MB-231 cells was regulated to varying extents. This suggests that PSO can induce cell cycle arrest in MCF-7 and MDA-MB-231 cells, possibly related to its inhibition of Wnt/β-catenin transcriptional activity. The mechanism of PSO inhibiting proliferation is shown in Figure 3.
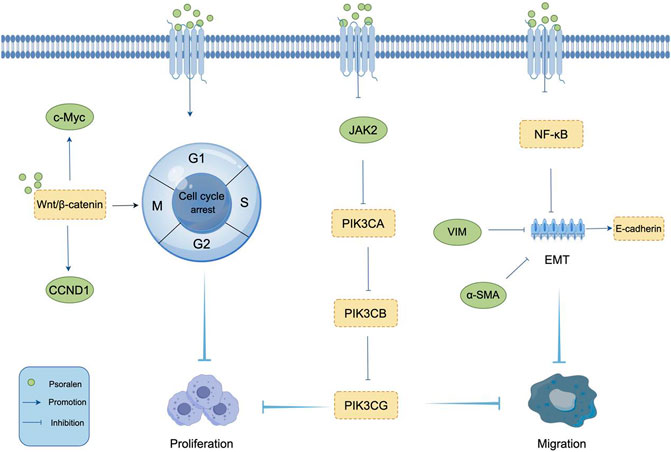
Figure 3. Mechanism of PSO inhibiting tumor cell Proliferation and Migration. PSO inhibits tumor cell proliferation by regulating cyclin and Wnt/β-catenin pathway. PSO prevents tumor cell migration by inhibiting EMT and upregulating the epithelial marker E-cadherin.
2.3 Inhibition of tumor cell migration
Migration and invasion are two major biological characteristics of malignant tumors. Epithelial-mesenchymal transition (EMT) is closely related to the invasion and metastasis of tumors, serving as a crucial mechanism facilitating cancer metastasis. During the progression of malignant tumors, EMT is activated, leading to epithelial cells losing cell-cell adhesion molecules like E-cadherin and acquiring mesenchymal markers such as N-cadherin, Vimentin, and α-SMA, related to cell polarity and the cytoskeleton (Dongre and Weinberg, 2019; Georgakopoulos-Soares et al., 2020; Brabletz et al., 2021; Ha et al., 2021). Therefore, targeting EMT could be an effective strategy for treating malignant and metastatic tumors. Wang et al. (2016a) determined the effect of PSO on the migration of MCF-7/ADR cells through wound healing assays, finding that PSO could inhibit the migration of MCF-7/ADR cells. They discovered that in MCF-7/ADR cells treated with PSO, the epithelial marker E-cadherin was significantly upregulated, while the mesenchymal markers Vimentin and α-SMA were significantly downregulated, suggesting that PSO could be a negative mediator of EMT and metastasis in MCF-7/ADR cells. PSO was also found to inhibit the activation of NF-κB necessary for EMT. Further research showed that PSO significantly reduced the migration capabilities of U87 and U251 glioma cells (Wu et al., 2022). In vitro experiments demonstrated that after 24 and 48 h of administration of 10 μM and 30 μM PSO, the migration abilities of U87 and U251 cells were significantly inhibited. It is speculated that PSO inhibits tumor cell proliferation and migration by reducing the expression of the genes JAK2, PIK3CA, PIK3CB, and PIK3CG. Breast cancer is one of the most prevalent cancers worldwide and remains the most common malignant tumor among women, leading to the highest number of cancer-related deaths among females (Nagini, 2017). Thanks to advancements in diagnostic and imaging technologies, along with early screening, the mortality rate of breast cancer has significantly decreased. However, the prognosis for breast cancer patients remains poor due to the risks of recurrence and metastasis (Chetlen et al., 2016; Li et al., 2016). Current treatments for breast cancer mainly include surgery, endocrine therapy, radiotherapy, chemotherapy, and targeted therapy (Mcdonald et al., 2016; Pan et al., 2017; Peart, 2017), but these treatments often come with severe adverse reactions (TAYLOR and KIRBY, 2015; Condorelli and Vaz-luis, 2018; Lacouture and Sibaud, 2018). In recent decades, nanoparticle-assisted combination drug therapy has made some progress in treating various types of cancer (Rawal and Patel, 2019). Polymer–lipid hybrid nanoparticles (PLNs) are a promising drug delivery system that has been widely used in the treatment of metastatic breast cancer (Zhu et al., 2015; JIang et al., 2016). Liu et al. (2021) found that PSO-loaded polymeric lipid nanoparticles (PSO-PLNs) could enhance the inhibitory effect of paclitaxel (PTX) on cell invasion and metastasis, suggesting the potential of PSO-PLNs as an adjuvant therapy against human BC cell metastasis. PTX combined with PLNs demonstrated a significant ability to inhibit cell migration and invasion activities related to the expression of IRAK1 and NF-κB in MDA-MB-231 breast cancer cells. These findings indicate that the combination of PTX and PSO-PLNs is a promising strategy for effectively treating BC metastasis. Evaluating nanoparticle formulations in animal models is also crucial for understanding their potential therapeutic impact. The mechanism of PSO inducing migration is shown in Figure 3.
2.4 Reversing multidrug resistance
Drug resistance refers to the tolerance developed by tumor cells to anticancer drugs, which significantly diminishes the therapeutic effects of these medications. It is primarily categorized into primary resistance, secondary resistance, and multidrug resistance (MDR). Cancer poses a significant threat to global human health, and chemotherapy is one of the most common and effective strategies for treating cancer (Siegel et al., 2021). However, drug resistance and adverse reactions are widespread, constituting major obstacles and challenges in current cancer treatment (Nurgali et al., 2018). In recent years, the feasibility of using traditional Chinese medicine to combat MDR has garnered considerable attention and has begun to be explored preliminarily (Chai et al., 2010). ABCB1, a member of the ATP-binding cassette family, has been found to be associated with drug resistance and transport (Calatozzolo et al., 2005). Studies have shown that the addition of PSO made A549/D16 cells dose-dependently resensitize to the toxicity of docetaxel (DOC); further in vitro experiments found that PSO significantly reduced the ABCB1 mRNA levels in A549/D16 lung cancer cells (Hsieh et al., 2014). When used in combination with DOC, the levels of ABCB1 mRNA were further decreased. This suggests that PSO can inhibit the activity of the ABCB1 promoter, downregulate the expression of the ABCB1 gene, inhibit the function of ABCB1, and ultimately sensitize resistant cells to chemotherapy-induced death, thereby effectively reversing multidrug resistance. This capability positions PSO as a potential remedial measure to overcome MDR involving ABCB1. Identifying the resistance mechanisms of different chemotherapeutic drugs in various tumor cells and implementing comprehensive targeted regulation could facilitate the reversal of chemotherapy resistance. Wang et al. (2016a) explored the effect of PSO on MDR in breast cancer cells through in vitro experiments. They discovered that PSO at a concentration of 8 μg/mL had a reversal fold of 3.39 in MCF-7/ADR cells, indicating that PSO could significantly reverse MDR, increasing the toxicity of adriamycin (ADR) to MCF-7/ADR cells. The release of exosomes has been proven to play a key role in resistance. Targeting the transfer of exosomes from resistant cells to sensitive cells might be a method to overcome certain resistances. The PPAR signaling pathway regulates the level of ceramide synthesis, an important regulatory molecule for exosome secretion (Trajkovic et al., 2008; Wang G. et al., 2012). Research has found that PSO can reduce the spread of resistance via exosomes through the PPAR and P53 signaling pathways, thus overcoming resistance (Wang et al., 2016b). The human ABCB1 gene, also known as multidrug resistance 1 (MDR1), encodes P-glycoprotein (P-gp). The MDR1 phenotype is commonly observed in breast cancer, producing chemotherapeutic resistance (Leonard et al., 2003). Jiang et al. used an adriamycin (ADR)-resistant human breast cancer cell line, MCF-7/ADR, to investigate whether PSO could reverse MDR by regulating the function of P-gp (Jiang et al., 2016). They further discovered that at certain concentrations, PSO could significantly reduce P-gp-mediated MDR in human breast cancer MCF-7/ADR cells by inhibiting the function of P-gp, affecting the reversal of MDR. This could provide new strategies for overcoming drug resistance in breast cancer in the future. The mechanism of PSO reversing MDR is shown in Figure 4. The mechanism of antitumor action of PSO is shown in Table 1.
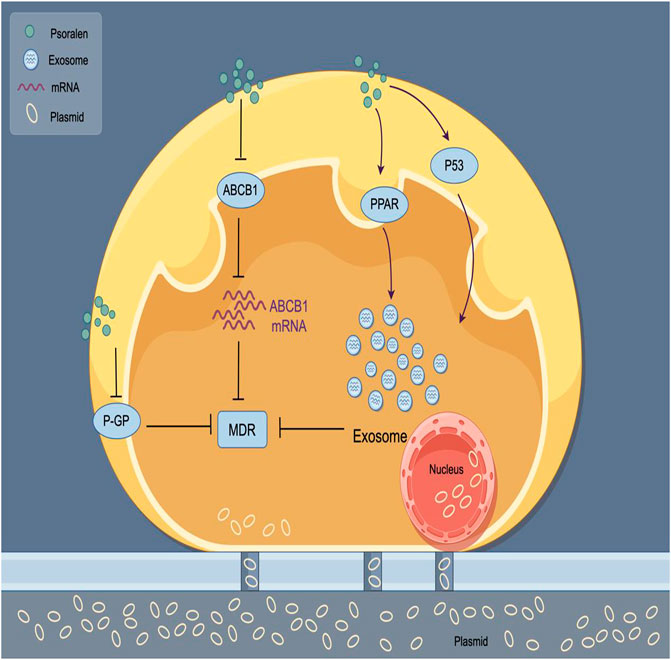
Figure 4. Mechanism of PSO reversing tumor cell MDR. PSO regulates the spread of exosome drug resistance through the PPAR and P53 signaling pathways, thereby inhibiting tumor cell drug resistance.
3 Mechanisms of hepatotoxicity
The liver, being the primary organ involved in drug metabolism and a crucial detoxification organ, is susceptible to drug toxicity (Hou et al., 2016). Drugs absorbed into the bloodstream are metabolized by the liver, where their metabolites or the drugs themselves can cause direct or indirect damage to this vital organ. Despite the widespread use of traditional Chinese medicine in clinical treatment due to its natural ingredients and perceived safety, reports of liver damage related to these herbal medicines have gradually increased in recent years. As research into Psoralea corylifolia and its main active component, PSO, deepens and its range of application expands, PSO has been identified as the primary cause of FP-induced hepatotoxicity (Cheung et al., 2009; Smith and Macdonald, 2014). Toxicological studies have shown that PSO can lead to multisystem damage, including to the reproductive, immune, and nervous systems, as well as to substantial organs such as the liver and kidneys, with the liver being particularly severely affected (Wang X. et al., 2012; Xia et al., 2018). Increasing evidence suggests that the mechanisms behind PSO-induced hepatotoxicity may involve a variety of factors, including bile stasis (Wang Y. et al., 2019; Huang et al., 2021), interference with liver regeneration (Zhou et al., 2018), oxidative stress responses and mitochondrial dysfunction (Xia et al., 2018), endoplasmic reticulum stress responses (Yu et al., 2020), and abnormalities in amino acid metabolism (Zhang et al., 2018). The mechanisms of PSO induced hepatotoxicity are shown in Table 2.
3.1 Bile stasis
Bile acids (BAs), derived from cholesterol in the liver, play a crucial role in liver function, physiology, and metabolic regulation. Bile acids have multiple functions, including preventing the formation of gallstones, promoting the excretion of cholesterol, and facilitating the absorption of lipids and nutrients in the intestines (Li and Chiang, 2009). Intrahepatic bile stasis can further lead to liver fibrosis, cirrhosis, and even liver failure. Studies have shown that approximately half of all cases of drug-induced liver injury (DILI) are associated with cholestatic dysfunction, and disruption in bile acid homeostasis is one of the mechanisms behind DILI (Tajiri and Shimizu, 2008; Mosedale and Watkins, 2017). Huang et al. explored the hepatotoxic effects of PSO on rats through in vitro experiments (Huang et al., 2021). They found that after administering PSO for 3 days, there was a significant upward trend in the levels of serum alanine aminotransferase (ALT), aspartate aminotransferase (AST), and total cholesterol (TC) in rats, suggesting that PSO could cause liver damage in rats after 3 days of administration. Hepatic bile acid transporters, responsible for the transport of bile acids and drugs, are crucial for preventing various cholestatic liver diseases. A decrease in or absence of the expression of hepatic bile acid transporters is a significant cause of such diseases. Further findings revealed significant changes in the mRNA and protein levels of rat hepatic bile acid transporters, with downregulation of MRP4, ABCG5, and ABCG8 proteins, and an increase in NTCP protein levels. This suggests that PSO may cause liver damage by affecting bile acid transporters, leading to disrupted transport and accumulation of bile acids within hepatocytes, which could be a potential mechanism behind PSO-induced liver injury. The transport of bile acids is coordinated by transport proteins located on the basolateral side of hepatocytes and in the bile ducts, such as the bile salt export pump (BSEP) and organic solute transporter α (OSTα). Chen et al. discovered that after administering PSO at a dose of 80 mg/kg for seven consecutive days, liver damage was induced in C57BL/6J mice (Chen et al., 2023). To further explore PSO-induced cholestatic liver injury, the bile acid content in the liver of mice was measured after 14 days of administration. It was found that PSO inhibited the expression of BSEP and OSTα, leading to impaired bile acid secretion and a significant increase in the hydrophobic bile acids CA and ALCA. This suggests that PSO may disrupt the balance of bile acid metabolism by inhibiting the expression of efflux transport proteins, thereby causing liver injury. The mechanism of PSO inducing Bile Stasis is shown in Figure 5.
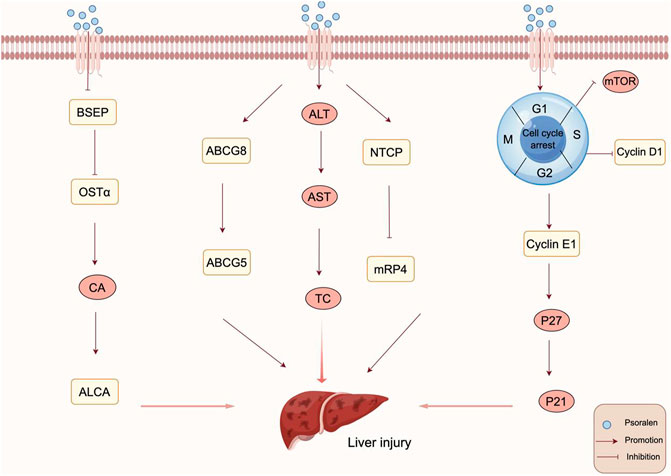
Figure 5. Mechanism of PSO inducing Bile Stasis and Delaying Liver Regeneration. PSO disrupt the balance of bile acid metabolism by inhibiting the expression of efflux transport proteins, thereby causing liver injury. PSO delay liver regeneration by regulating the expression of cyclin and inhibiting the mTOR signaling pathway.
3.2 Delaying liver regeneration
The liver is a vital organ for maintaining metabolic balance and detoxification within the body, possessing remarkable regenerative and compensatory abilities that allow it to repair and replace lost or damaged liver tissue and restore its physiological functions after injury. Liver regeneration is a complex process involving multiple cells and factors. The mammalian target of rapamycin (mTOR) acts as a sensor for various intracellular and extracellular signals, appropriately regulating biological processes including cell proliferation, metabolism, and cell cycle progression (Laplante and Sabatini, 2012). Liang et al. (2020) through studies utilizing a carbon tetrachloride (CCl4)-induced liver injury model in mice, found that PSO could induce G1/S phase arrest in hepatocytes, affecting the liver’s regenerative and repair capabilities and delaying recovery after partial hepatectomy. The preliminary mechanism may be related to the inhibition of PCNA and regulation of some cell cycle-associated protein by PSO, in which the significant upregulation of p27, p53 and p21 may play important roles. A 2/3 partial hepatectomy (PHx; also known as 2/3 PH or 70% PH) is a common in vivo model used to study liver regeneration. Zhou et al. (2018) through a 2/3 partial hepatectomy mouse model and in vitro experiments, discovered that PSO could induce mild liver injury in mice and cytotoxicity in L02 cells. The cell cycle process plays an indispensable role in tissue growth and regeneration in multicellular organisms. Further investigation into the effects of PSO on liver regeneration and cell cycle arrest in vivo revealed that PSO could upregulate the protein expression of cyclin E1 and p27 and downregulate cyclin D1, inducing G1/S phase arrest. This suggests that the reduced capacity for liver regeneration caused by hepatocyte cell cycle arrest may be related to the regulation of cell cycle protein expression and inhibition of the mTOR signaling pathway. The mechanism of PSO Delaying Liver Regeneration is shown in Figure 5.
3.3 Endoplasmic reticulum stress
The endoplasmic reticulum (ER) maintains cellular homeostasis by regulating the synthesis, folding, and modification of proteins, as well as the transport of Ca2+. Disruptions in ER homeostasis can affect proper protein folding, leading to endoplasmic reticulum stress (ER stress) and dysfunction. The unfolded protein response (UPR) is an evolutionary mechanism designed to restore ER homeostasis, but if ER stress is severe and unresolved, it can induce apoptosis. The UPR pathway consists of three sensor proteins: protein kinase R (PKR)-like ER kinase (PERK), inositol-requiring protein 1α (IRE1α), and activating transcription factor 6 (ATF6) (Urra et al., 2013). Numerous clinical and experimental studies have demonstrated that abnormalities in ER stress can activate apoptosis, leading to severe liver damage in clinical settings (Iracheta-Vellve et al., 2016; Ren et al., 2017). Yu et al. conducted in vitro experiments to explore the toxic effects of PSO on HepG2 cells. They found that PSO significantly induced liver cell death and apoptosis in a time- and dose-dependent manner (Yu et al., 2020). Moreover, PSO significantly increased the expression and transcription levels of ER stress-related markers, including Grp78, PERK, eIF2α, ATF4, and ATF6. The ER stress inhibitor 4-phenylbutyrate (4-PBA) effectively inhibited PSO-induced cell death and apoptosis, as well as the ER stress response. This suggests that PSO induces ER stress-mediated apoptosis through the PERK-eIF2α-ATF4-CHOP and ATF6-CHOP related pathways, thereby causing liver injury. Targeting ER stress presents a potential therapeutic and preventive strategy for addressing liver toxicity induced by PSO.
3.4 Oxidative stress and mitochondrial dysfunction
Oxidative stress is a condition characterized by an imbalance between the production of reactive oxygen species (ROS) and the body’s ability to clear them through its antioxidant defense system. It is recognized as a mechanism of chemically induced toxicity. Elevated levels of ROS can react with complex cellular molecules such as lipids, proteins, or DNA, leading to homeostatic imbalance, oxidative stress, and damage to cellular components (Cederbaum et al., 2009; Rahal et al., 2014). Studies have shown that oxidative damage can lead to liver injury when the production of ROS exceeds the antioxidant capacity of the cell (Guo et al., 2021).
The transcription factor nuclear factor erythroid 2-related factor 2 (Nrf2) plays a pivotal role in the cellular oxidative stress response, maintaining redox balance and signaling, and regulating the expression of various antioxidants (Kozieł et al., 2021). The activation of the PI3K-Akt pathway mediates positive regulatory signals of Nrf2 within the cell, participating in the antioxidative process (Kim et al., 2004). Sun et al. (2022) using proteomics and network pharmacology techniques, identified ABL1 as a direct potential target for PSO-induced hepatotoxicity and subsequently verified its toxic mechanism through in vitro experiments. They found that the combination of PSO and ABL1 reduced the expression of downstream Nrf2 and mTOR, leading to elevated levels of ROS and resulting in cellular damage. CYP1A2 is a major Phase I metabolic enzyme abundantly expressed in the liver (Zanger and Schwab, 2013). Research has linked PSO-induced hepatotoxicity with upregulated CYP1A2 expression (SONG L. et al., 2019). The formation of reactive metabolites catalyzed by Cytochrome P450(CYP450) and subsequent oxidative stress is a common pathway for metabolism-mediated exogenous toxicity (Jee et al., 2021; Wang et al., 2021). Zhang et al. revealed the role of CYP1A2 in PSO-induced metabolic activation and hepatotoxicity through transcriptomics and metabolomics (Zhang et al., 2023). In vitro and in vivo studies have shown that PSO-induced oxidative stress is associated with CYP1A2, where the induction of CYP1A2 leads to increased levels of MDA, and reduced activity of SOD and levels of GSH. This suggests that PSO is metabolically activated by CYP1A2, forming reactive intermediates, thereby depleting glutathione (GSH), inducing cellular oxidative stress, and hepatotoxicity. Cell survival is maintained through a balance between the levels of ROS and the cell’s antioxidative capacity (Lee et al., 2017). Xia et al. (2018) evaluated the developmental toxicity of PSO on zebrafish embryos/larvae through experiments. They discovered an increase in ROS production in the PSO treatment group; however, the activity of total superoxide dismutase (T-SOD) significantly decreased, and the levels of malondialdehyde (MDA) significantly increased. This indicates that PSO treatment induced oxidative stress during the development of zebrafish embryos, suggesting that the molecular mechanism behind PSO-induced liver damage in zebrafish might involve the generation of excessive ROS or inhibition of the organism’s antioxidative system’s clearance capacity. This triggers oxidative stress, producing a large number of free radicals and lipid peroxides, thereby causing liver damage. Moreover, mitochondrial dysfunction is also associated with the occurrence and development of hepatotoxicity (Behrends et al., 2019). Mitochondria are the main sites of energy metabolism within the cell, playing a crucial role in processes such as ATP synthesis and apoptosis. In zebrafish larvae treated with PSO, the expression levels of pro-apoptotic protein-encoding genes (p53, puma, apaf-1, caspase-9, caspase-3) were elevated, and the expression level of Bcl-2 was decreased, indicating that PSO induces apoptosis in zebrafish larvae through a mitochondria-dependent pathway (Xia et al., 2018). Additionally, gene expression analysis results suggest that PSO induces liver developmental toxicity through oxidative stress, apoptosis, and abnormalities in energy metabolism. The mechanism of PSO inducing Oxidative Stress and Mitochondrial Dysfunction is shown in Figure 6.
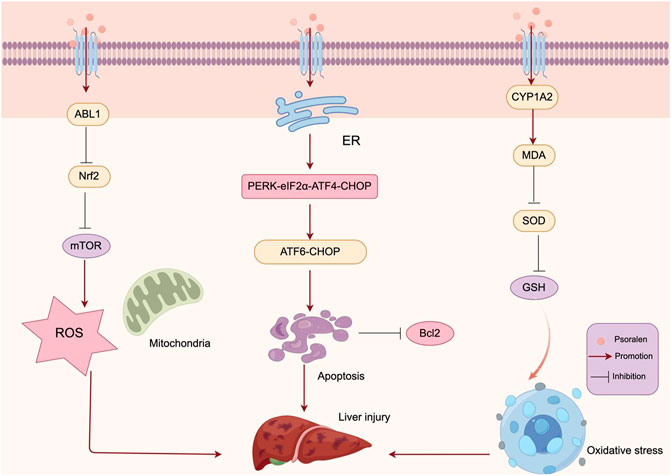
Figure 6. Mechanism of PSO inducing Oxidative Stress and Mitochondrial Dysfunction. PSO is metabolically activated by CYP1A2, forming reactive intermediates, thereby depleting GSH, inducing cellular oxidative stress, and liver injury. The combination of PSO and ABL1 reduced the expression of Nrf2 and mTOR, leading to elevated levels of ROS and resulting in liver injury.
3.5 Other mechanisms
In addition to bile stasis, interference with liver regeneration, oxidative stress responses with mitochondrial dysfunction, and endoplasmic reticulum stress responses, the hepatotoxicity of PSO involves other related pathways such as drug metabolism and amino acid metabolism. The liver is the primary organ for drug metabolism, which includes phases of oxidation, reduction, hydrolysis, and conjugation. CYP450 enzymes, a class of heme-containing monooxygenases, are the main liver enzymes involved in Phase I drug metabolism through oxidation or reduction (Zanger and Schwab, 2013). Research by Jiang et al. found that the accumulation of PSO in the liver is a major cause of liver injury (Jiang et al., 2022). Further investigation revealed that PSO can directly bind to CYP2D6, CYP3A4, GST-α, and GST-μ, inhibiting their activity, leading to the depletion of GSH in the body, causing liver damage and resulting in disorders of drug metabolism. The combined use of PSO with glutathione can alleviate the elevation of transaminase levels caused by PSO and improve liver pathological changes, suggesting that the combination of GSH and PSO is an effective method to avoid liver damage in clinical practice. The aryl hydrocarbon receptor (AhR) is a ligand-dependent transcription factor that initiates the transcription of target genes by forming heterodimers with AhR nuclear translocator, regulating the expression of drug-metabolizing enzymes such as CYP1A and 1B (Vogel et al., 2020; Xu et al., 2021; Zhang W. et al., 2022). PSO-induced hepatotoxicity has been reported to be associated with the exogenous metabolism of cytochrome P450 (CYPs), with CYP1A2 being a significant metabolic enzyme involved in PSO-induced hepatotoxicity (Song L. et al., 2019). CYP1A2 accounts for approximately 4%–16% of the total CYP pool in the liver and is involved in the metabolism of the main components in PF (Zhou et al., 2019; Kwon et al., 2021). Zhang C. et al. (2022) discovered through in vitro and in vivo experiments that the hepatotoxicity induced by PSO in HepG2 390 cells and mice is related to the induction of CYP1A2 expression. The potential mechanism may involve the activation of AhR by these molecules, initiating the transcription of the target gene CYP1A2, leading to increased levels of CYP1A2. This is accompanied by elevated levels of CYP1A2 mRNA and protein, and significant inhibition of CYP1A2 activity in vitro. Metabolomics, a relatively new research technique, has become a viable tool for studying the biochemical actions of many toxic substances (Han et al., 2016). With the continuous development of metabolomics, untargeted metabolomics has been widely applied in drug safety evaluation and toxicity prediction, providing valuable information for drug-induced cardiotoxicity, hepatotoxicity, and nephrotoxicity. Research teams have observed that long-term exposure to low levels of PSO and isoPSO induces hepatotoxicity in female rats and changes in the serum metabolome. These changes include disruptions in the metabolic pathways of alanine metabolism, glutamate metabolism, the urea cycle, the glucose-alanine cycle, the ammonia cycle, and the metabolism of glycine and serine. This suggests that PSO may inhibit the synthesis of proteins from free amino acids while also preventing their use as substrates for gluconeogenesis in rats (Yu et al., 2019). Zhang et al. (2018) utilizing 1H-NMR metabolomics technology combined with conventional serum biochemistry, investigated the mechanism of liver injury induced by PSO and found that the liver is a direct target of PSO toxicity. Multivariate analysis identified seven metabolites in serum samples and fifteen metabolites in liver samples as potential biomarkers for liver injury caused by PSO. Further findings suggested that PSO might cause liver damage by disrupting the biosynthesis of valine, leucine, and isoleucine in the serum and liver. These findings provide a reference for the medicinal safety and potential risks of PSO.
4 Discussion
The global incidence and mortality rates of cancer continue to climb, posing a significant impact on human health worldwide. The mechanisms underlying malignant tumors are incredibly complex, making the exploration of their pathogenesis and the search for safe and effective anti-tumor drugs especially important. With the rapid advancements in medical science and the continuous refinement of bioinformatics, effective targets and pathways for natural drugs to regulate tumors are gradually being identified, and an increasing number of active monomers from traditional Chinese medicines are being discovered. PSO, a major active component of the traditional Chinese medicine Psoralea corylifolia, can inhibit tumor growth through multiple targets and has relatively good safety. Therefore, researching and developing the anti-tumor activity and mechanisms of PSO hold significant importance and have broad prospects in treating malignant tumors. PSO is widely distributed in various natural plants and has attracted widespread attention from the research community due to its good medicinal potential. It exhibits multiple pharmacological activities, including anti-tumor, neuroprotective, anti-inflammatory, and antioxidant effects, playing an important role in the treatment of various diseases. PSO’s anti-tumor effect is extensive; it not only inhibits the growth of solid tumors and primary tumor cells but also controls the invasive activity of tumor cells to prevent metastasis. PSO exerts its anti-tumor effects mainly by inducing apoptosis, inhibiting cell proliferation and migration, and reversing multi-drug resistance of tumor cells, making it a promising anti-tumor drug. With the continuous development and improvement of experimental techniques and bioinformatics, the anti-tumor effect of PSO has been fully confirmed in experiments, and its pharmacological mechanism has been elucidated at multiple levels. However, current research is mostly limited to in vitro experiments, lacking necessary clinical studies. Further research on its precise mechanisms, target actions, and clinical applications is needed. Besides the most common anti-tumor active components of Psoralea corylifolia, PSO and isoPSO, research on the anti-tumor effects of other chemical components is scarce. It would be beneficial to increase the research on the synthesis of derivatives of PSO and isoPSO and their anti-tumor activities to discover compounds with less toxicity and resistance. Additionally, comparing and analyzing the chemical structures of anti-tumor active components in Psoralea corylifolia, modifying and optimizing the compounds, and focusing on in vivo anti-tumor activity and drug processes in the body will help develop more clinically meaningful anti-tumor drugs.
The percentage of a medicinal product absorbed unmodified in the systemic circulation represent the bioavailability of that product (Pandareesh et al., 2015; Salehi et al., 2020). The bioavailability represents the actual effective concentration of these compounds at the site of drug action following their absorption from the gastrointestinal tract after oral administration of a dosage form (solution, suspension, tablet, etc.) (Chow, 2014; Salehi et al., 2018). PSO is a natural compound that is orally bioavailable. New drug forms such as nanoencapsulation have been developed to improve the bioavailability of PSO (Chen et al., 2017; Zhang et al., 2019). Yen et al. found that nanoencapsulation of PSO (viachitosan and Eudragit S100) improved the efficiency of oral drug delivery. The pharmacokinetic analysis of PSO and PSO-nanoencapsulate revealed that the bioavailability of nano-microencapsulated microspheres was 339.02% higher compared to simple microsphere suspension, indicating that nano-microcapsules exhibited superior potential in enhancing the oral absorption of PSO when compared to traditional suspensions (Yin et al., 2016). These results suggest that PSO pharmaceutical nanoformulations containing chitosan and EudragitS100 compounds have great potential for improving PSO bioavailability.
To address psoralen-induced hepatotoxicity, the dosage of psoralen can be reduced to mitigate toxicity, especially for patients with impaired liver function. Additionally, developing psoralen analogs with lower toxicity but retained efficacy is another approach. Combining psoralen with other drugs that enhance its therapeutic effects can also reduce its toxicity. Structurally modifying psoralen to enhance its therapeutic properties and reduce toxicity has been achieved by synthesizing several derivatives and analogs, such as 8-methoxypsoralen (8-MOP) and 5-methoxypsoralen (5-MOP). These modifications aim to improve psoralen’s photoreactivity and reduce side effects. Psoralen targeted delivery systems utilize nanoparticle-based systems to direct psoralen to tumor cells, minimizing exposure to healthy tissues. Researchers have developed various targeted delivery systems to improve psoralen’s therapeutic index. Encapsulating psoralen in nanoparticles can enhance its delivery to tumor cells while reducing systemic toxicity. Nanoparticles can be designed to release psoralen in response to specific stimuli in the tumor microenvironment, such as pH or temperature changes. Regarding the clinical application of psoralen, a review of relevant literature indicates that psoralen combined with UVA light (PUVA therapy) has been clinically used to treat various skin diseases, including psoriasis, vitiligo, and cutaneous T-cell lymphoma. The use of traditional Chinese medicine can lead to certain liver injuries (Herb-induced liver injury, HILI). The development of HILI is insidious, closely related to the drug’s toxicity, dosage, duration of use, and individual differences. As reports of liver toxicity caused by Psoralea corylifolia and its compound preparations increase, the material basis of Psoralea corylifolia liver toxicity has received widespread attention from scholars. PSO, as the main active component of Psoralea corylifolia, has been proven to have hepatotoxicity, and long-term use can lead to liver damage. PSO can cause liver toxicity through various pathways, such as inhibiting liver regeneration, bile stasis, oxidative stress, and mitochondrial dysfunction, causing damage to the liver. Further analysis reveals the complexity of the mechanisms behind herbal medicine-induced liver toxicity due to the diversity of toxic substances, the complex chemical components of natural drugs, and their multi-level, multi-target, and multi-pathway effects on the body, further hindering the systematic characterization and elucidation of the mechanisms of herbal liver toxicity. On one hand, the emergence of multi-omics technologies provides a powerful tool for the study of traditional Chinese medicine toxicology, describing cellular life activities at multiple levels. Its advantages lie in the specific changes in biological pathways during the onset and treatment of diseases, aligning with the characteristics of natural drugs’ multi-pathway, multi-component, and multi-target effects, to some extent solving issues of unclear action targets and undefined toxicity mechanisms in natural drug toxicity research. Therefore, it is necessary to further apply metabolomics technology to the study of traditional Chinese medicine toxicology, providing key support for rational and safe medication. On the other hand, as a natural drug with potential toxicity, a rational evaluation of the hepatotoxicity of PSO can help improve the safety and rationality of clinical medication. Establishing scientific and rational traditional Chinese medicine safety evaluation methods, further exploring the mechanisms of PSO’s toxicity reduction and the “toxicity-effect” transformation relationship, will aid in rationally evaluating the benefits and risks of using PSO, providing references for its clinical safe use, new drug development, and drug evaluation containing Psoralea corylifolia. In summary, the advantages of natural drugs in terms of safety and efficacy have attracted widespread attention from scholars. The development and utilization of traditional Chinese medicine have become a hot topic. An increasing number of natural drugs are being developed, and in the future, traditional Chinese medicine monomers are expected to be developed into new, safe, and effective anti-tumor drugs. Meanwhile, we should also pay attention to the potential liver damage caused by natural drugs, rationally evaluate their hepatotoxicity while developing and utilizing them, and maximize the advantages of natural drugs for clinical safe use, new drug research and development, and drug evaluation.
Author contributions
DM: Conceptualization, Supervision, Validation, Writing–original draft, Writing–review and editing. YD: Conceptualization, Investigation, Validation, Writing–original draft, Writing–review and editing. QS: Conceptualization, Investigation, Methodology, Writing–original draft, Writing–review and editing. ZS: Conceptualization, Investigation, Methodology, Project administration, Writing–original draft, Writing–review and editing.
Funding
The author(s) declare that no financial support was received for the research, authorship, and/or publication of this article.
Acknowledgments
All authors are thanked for their contributions to this study. We would like to thank the Figdraw platform for supporting this study.
Conflict of interest
The authors declare that the research was conducted in the absence of any commercial or financial relationships that could be construed as a potential conflict of interest.
Publisher’s note
All claims expressed in this article are solely those of the authors and do not necessarily represent those of their affiliated organizations, or those of the publisher, the editors and the reviewers. Any product that may be evaluated in this article, or claim that may be made by its manufacturer, is not guaranteed or endorsed by the publisher.
References
Adams, C. J., Kopp, M. C., Larburu, N., Nowak, P. R., and Ali, M. M. U. (2019). Structure and molecular mechanism of ER stress signaling by the unfolded protein response signal activator IRE1. Front. Mol. Biosci. 6, 11. doi:10.3389/fmolb.2019.00011
Al-Hetty, H., Jabbar, A. D., Eremin, V. F., Jabbar, A. M., Jalil, A. T., Al-Dulimi, A. G., et al. (2023). The role of endoplasmic reticulum stress in endometriosis. Cell. Stress Chaperones 28 (2), 145–150. doi:10.1007/s12192-023-01323-2
Behrends, V., GiskeøDEGåRD, G. F., Bravo-Santano, N., Letek, M., and Keun, H. C. (2019). Acetaminophen cytotoxicity in HepG2 cells is associated with a decoupling of glycolysis from the TCA cycle, loss of NADPH production, and suppression of anabolism. Arch. Toxicol. 93 (2), 341–353. doi:10.1007/s00204-018-2371-0
Brabletz, S., Schuhwerk, H., Brabletz, T., and Stemmler, M. P. (2021). Dynamic EMT: a multi-tool for tumor progression. Embo J. 40 (18), e108647. doi:10.15252/embj.2021108647
Bray, F., Ferlay, J., Soerjomataram, I., Siegel, R. L., Torre, L. A., and Jemal, A. (2018). Global cancer statistics 2018: GLOBOCAN estimates of incidence and mortality worldwide for 36 cancers in 185 countries. CA Cancer J. Clin. 68 (6), 394–424. doi:10.3322/caac.21492
Calatozzolo, C., Gelati, M., Ciusani, E., Sciacca, F. L., Pollo, B., Cajola, L., et al. (2005). Expression of drug resistance proteins Pgp, MRP1, MRP3, MRP5 and GST-pi in human glioma. J. Neurooncol 74 (2), 113–121. doi:10.1007/s11060-004-6152-7
Canton, M., Caffieri, S., Dall’Acqua, F., and Di Lisa, F. (2002). PUVA-induced apoptosis involves mitochondrial dysfunction caused by the opening of the permeability transition pore. FEBS Lett. 522 (1-3), 168–172. doi:10.1016/s0014-5793(02)02926-5
Carneiro, B. A., and el-Deiry, W. S. (2020). Targeting apoptosis in cancer therapy. Nat. Rev. Clin. Oncol. 17 (7), 395–417. doi:10.1038/s41571-020-0341-y
Cederbaum, A. I., Lu, Y., and Wu, D. (2009). Role of oxidative stress in alcohol-induced liver injury. Arch. Toxicol. 83 (6), 519–548. doi:10.1007/s00204-009-0432-0
Chai, S., To, K. K., and Lin, G. (2010). Circumvention of multi-drug resistance of cancer cells by Chinese herbal medicines. Chin. Med. 5, 26. doi:10.1186/1749-8546-5-26
Chen, C. H., Hwang, T. L., Chen, L. C., Chang, T. H., Wei, C. S., and Chen, J. J. (2017). Isoflavones and anti-inflammatory constituents from the fruits of Psoralea corylifolia. Phytochemistry 143, 186–193. doi:10.1016/j.phytochem.2017.08.004
Chen, M. Y., Wang, Q., Meng, Z. J., Men, W. J., Huang, J. Y., Yu, B., et al. (2023). Psoralen induces liver injury and affects hepatic bile acids metabolism in female and male C57BL/6J mice. Phytother. Res. 37 (6), 2280–2289. doi:10.1002/ptr.7739
Chetlen, A., Mack, J., and Chan, T. (2016). Breast cancer screening controversies: who, when, why, and how? Clin. Imaging 40 (2), 279–282. doi:10.1016/j.clinimag.2015.05.017
Cheung, W. I., Tse, M. L., Ngan, T., Lin, J., Lee, W. K., Poon, W. T., et al. (2009). Liver injury associated with the use of Fructus Psoraleae (Bol-gol-zhee or Bu-gu-zhi) and its related proprietary medicine. Clin. Toxicol. (Phila) 47 (7), 683–685. doi:10.1080/15563650903059136
Chopra, B., and Dhingra, A. K. (2021). Natural products: a lead for drug discovery and development. Phytother. Res. 35 (9), 4660–4702. doi:10.1002/ptr.7099
Chow, S. C. (2014). Bioavailability and bioequivalence in drug development. Wiley Interdiscip. Rev. Comput. Stat. 6 (4), 304–312. doi:10.1002/wics.1310
Cloughesy, T. F., Petrecca, K., Walbert, T., Butowski, N., Salacz, M., Perry, J., et al. (2020). Effect of vocimagene amiretrorepvec in combination with flucytosine vs standard of care on survival following tumor resection in patients with recurrent high-grade glioma: a randomized clinical trial. JAMA Oncol. 6 (12), 1939–1946. doi:10.1001/jamaoncol.2020.3161
Condorelli, R., and Vaz-Luis, I. (2018). Managing side effects in adjuvant endocrine therapy for breast cancer. Expert Rev. Anticancer Ther. 18 (11), 1101–1112. doi:10.1080/14737140.2018.1520096
Dongre, A., and Weinberg, R. A. (2019). New insights into the mechanisms of epithelial-mesenchymal transition and implications for cancer. Nat. Rev. Mol. Cell. Biol. 20 (2), 69–84. doi:10.1038/s41580-018-0080-4
Du, M. Y., Duan, J. X., Zhang, C. Y., Yang, H. H., Guan, X. X., Zhong, W. J., et al. (2020). Psoralen attenuates bleomycin-induced pulmonary fibrosis in mice through inhibiting myofibroblast activation and collagen deposition. Cell. Biol. Int. 44 (1), 98–107. doi:10.1002/cbin.11205
Georgakopoulos-Soares, I., Chartoumpekis, D. V., Kyriazopoulou, V., and Zaravinos, A. (2020). EMT factors and metabolic pathways in cancer. Front. Oncol. 10, 499. doi:10.3389/fonc.2020.00499
Goodlad, R. A. (2017). Quantification of epithelial cell proliferation, cell dynamics, and cell kinetics in vivo. Wiley Interdiscip. Rev. Dev. Biol. 6 (4). doi:10.1002/wdev.274
Guo, L., Gong, H., Tang, T. L., Zhang, B. K., Zhang, L. Y., and Yan, M. (2021). Crizotinib and sunitinib induce hepatotoxicity and mitochondrial apoptosis in L02 cells via ROS and Nrf2 signaling pathway. Front. Pharmacol. 12, 620934. doi:10.3389/fphar.2021.620934
Ha, J., Lee, S., Park, J., Seo, J., Kang, E., Yoon, H., et al. (2021). Identification of a novel inhibitor of liver cancer cell invasion and proliferation through regulation of Akt and Twist1. Sci. Rep. 11 (1), 16765. doi:10.1038/s41598-021-95933-4
Han, H., Xiao, H., and Lu, Z. (2016). Short-term toxicity assessments of an antibiotic metabolite in Wistar rats and its metabonomics analysis by ultra-high performance liquid chromatography coupled to quadrupole time-of-flight mass spectrometry. Toxicol. Appl. Pharmacol. 293, 1–9. doi:10.1016/j.taap.2016.01.004
Hou, J., Sun, E., Song, J., Yang, L., Zhang, Z. H., Ning, Q., et al. (2016). Relationship between hepatic drug-metabolizing enzymes CYP450 and traditional Chinese medicine-induced hepatotoxicity. Zhongguo Zhong Yao Za Zhi 41 (15), 2774–2780. doi:10.4268/cjcmm20161505
Hsieh, M. J., Chen, M. K., Yu, Y. Y., Sheu, G. T., and Chiou, H. L. (2014). Psoralen reverses docetaxel-induced multidrug resistance in A549/D16 human lung cancer cells lines. Phytomedicine 21 (7), 970–977. doi:10.1016/j.phymed.2014.03.008
Huang, J., Wang, Q., Chen, M., Bi, Y., Shi, H., and Zhou, K. (2021). Effects of psoralen on hepatic bile acid transporters in rats. Hum. Exp. Toxicol. 40 (6), 1012–1021. doi:10.1177/0960327120979346
Iracheta-Vellve, A., Petrasek, J., Gyongyosi, B., Satishchandran, A., Lowe, P., Kodys, K., et al. (2016). Endoplasmic reticulum stress-induced hepatocellular death pathways mediate liver injury and fibrosis via stimulator of interferon genes. J. Biol. Chem. 291 (52), 26794–26805. doi:10.1074/jbc.M116.736991
Jee, A., Sernoskie, S. C., and Uetrecht, J. (2021). Idiosyncratic drug-induced liver injury: mechanistic and clinical challenges. Int. J. Mol. Sci. 22 (6), 2954. doi:10.3390/ijms22062954
Jiang, J., Wang, X., Cheng, K., Zhao, W., Hua, Y., Xu, C., et al. (2016). Psoralen reverses the P-glycoprotein-mediated multidrug resistance in human breast cancer MCF-7/ADR cells. Mol. Med. Rep. 13 (6), 4745–4750. doi:10.3892/mmr.2016.5098
Jiang, M., Wang, X., Lv, B., Lu, Y., Ma, X., Liu, W., et al. (2022). Psoralen induces hepatotoxicity by covalently binding to glutathione-S-transferases and the hepatic cytochrome P450. Phytomedicine 104, 154165. doi:10.1016/j.phymed.2022.154165
Jiang, Z., and Xiong, J. (2014). Induction of apoptosis in human hepatocarcinoma SMMC-7721 cells in vitro by psoralen from Psoralea corylifolia. Cell. Biochem. Biophys. 70 (2), 1075–1081. doi:10.1007/s12013-014-0025-2
Kim, S. K., Woodcroft, K. J., Khodadadeh, S. S., and Novak, R. F. (2004). Insulin signaling regulates gamma-glutamylcysteine ligase catalytic subunit expression in primary cultured rat hepatocytes. J. Pharmacol. Exp. Ther. 311 (1), 99–108. doi:10.1124/jpet.104.070375
Kozieł, M. J., Kowalska, K., and Piastowska-Ciesielska, A. W. (2021). Nrf2: a main responsive element in cells to mycotoxin-induced toxicity. Arch. Toxicol. 95 (5), 1521–1533. doi:10.1007/s00204-021-02995-4
Kwon, Y. J., Shin, S., and Chun, Y. J. (2021). Biological roles of cytochrome P450 1A1, 1A2, and 1B1 enzymes. Arch. Pharm. Res. 44 (1), 63–83. doi:10.1007/s12272-021-01306-w
Lacouture, M., and Sibaud, V. (2018). Toxic side effects of targeted therapies and immunotherapies affecting the skin, oral mucosa, hair, and nails. Am. J. Clin. Dermatol 19 (Suppl. 1), 31–39. doi:10.1007/s40257-018-0384-3
Laplante, M., and Sabatini, D. M. (2012). mTOR signaling in growth control and disease. Cell. 149 (2), 274–293. doi:10.1016/j.cell.2012.03.017
Lee, M. T., Lin, W. C., Yu, B., and Lee, T. T. (2017). Antioxidant capacity of phytochemicals and their potential effects on oxidative status in animals - a review. Asian-Australas J. Anim. Sci. 30 (3), 299–308. doi:10.5713/ajas.16.0438
Leonard, G. D., Fojo, T., and Bates, S. E. (2003). The role of ABC transporters in clinical practice. Oncologist 8 (5), 411–424. doi:10.1634/theoncologist.8-5-411
Liang, P. S., Zhou, W., Jiang, Z. Z., and Zhang, L. Y. (2020). Mechanism of psoralen in aggravating hepatotoxicity induced by CCl_4 by delaying liver regeneration. Zhongguo Zhong Yao Za Zhi 45 (12), 2916–2923. doi:10.19540/j.cnki.cjcmm.20200115.401
Li, S., and Tu, H. (2022). Psoralen inhibits the proliferation and promotes apoptosis through endoplasmic reticulum stress in human osteosarcoma cells. Folia Histochem Cytobiol. 60 (1), 101–109. doi:10.5603/FHC.a2022.0010
Liszewski, W., Naym, D. G., Biskup, E., and Gniadecki, R. (2017). Psoralen with ultraviolet A-induced apoptosis of cutaneous lymphoma cell lines is augmented by type I interferons via the JAK1-STAT1 pathway. Photodermatol. Photoimmunol. Photomed. 33 (3), 164–171. doi:10.1111/phpp.12302
Li, T., and Chiang, J. Y. (2009). Regulation of bile acid and cholesterol metabolism by PPARs. PPAR Res. 2009, 501739. doi:10.1155/2009/501739
Li, T., Mello-Thoms, C., and Brennan, P. C. (2016). Descriptive epidemiology of breast cancer in China: incidence, mortality, survival and prevalence. Breast Cancer Res. Treat. 159 (3), 395–406. doi:10.1007/s10549-016-3947-0
Liu, F., Li, L., Lan, M., Zou, T., Kong, Z., Cai, T., et al. (2021). Psoralen-loaded polymeric lipid nanoparticles combined with paclitaxel for the treatment of triple-negative breast cancer. Nanomedicine (Lond) 16 (27), 2411–2430. doi:10.2217/nnm-2021-0241
Lu, H., Zhang, L., Liu, D., Tang, P., and Song, F. (2014). Isolation and purification of psoralen and isopsoralen and their efficacy and safety in the treatment of osteosarcoma in nude rats. Afr. Health Sci. 14 (3), 641–647. doi:10.4314/ahs.v14i3.20
Mcdonald, E. S., Clark, A. S., Tchou, J., Zhang, P., and Freedman, G. M. (2016). Clinical diagnosis and management of breast cancer. J. Nucl. Med. 57 (Suppl. 1), 9s–16s. doi:10.2967/jnumed.115.157834
Miller, A. M., Shah, R. H., Pentsova, E. I., Pourmaleki, M., Briggs, S., Distefano, N., et al. (2019). Tracking tumour evolution in glioma through liquid biopsies of cerebrospinal fluid. Nature 565 (7741), 654–658. doi:10.1038/s41586-019-0882-3
Mills, C. C., Kolb, E. A., and Sampson, V. B. (2017). Recent advances of cell-cycle inhibitor therapies for pediatric cancer. Cancer Res. 77 (23), 6489–6498. doi:10.1158/0008-5472.CAN-17-2066
Molinaro, A. M., Taylor, J. W., Wiencke, J. K., and Wrensch, M. R. (2019). Genetic and molecular epidemiology of adult diffuse glioma. Nat. Rev. Neurol. 15 (7), 405–417. doi:10.1038/s41582-019-0220-2
Morana, O., Wood, W., and Gregory, C. D. (2022). The apoptosis paradox in cancer. Int. J. Mol. Sci. 23 (3), 1328. doi:10.3390/ijms23031328
Mosedale, M., and Watkins, P. B. (2017). Drug-induced liver injury: advances in mechanistic understanding that will inform risk management. Clin. Pharmacol. Ther. 101 (4), 469–480. doi:10.1002/cpt.564
Nagini, S. (2017). Breast cancer: current molecular therapeutic targets and new players. Anticancer Agents Med. Chem. 17 (2), 152–163. doi:10.2174/1871520616666160502122724
Nurgali, K., Jagoe, R. T., and Abalo, R. (2018). Editorial: adverse effects of cancer chemotherapy: anything new to improve tolerance and reduce sequelae? Front. Pharmacol. 9, 245. doi:10.3389/fphar.2018.00245
Oakes, S. A., and Papa, F. R. (2015). The role of endoplasmic reticulum stress in human pathology. Annu. Rev. Pathol. 10, 173–194. doi:10.1146/annurev-pathol-012513-104649
Pandareesh, M. D., Mythri, R. B., and Srinivas Bharath, M. M. (2015). Bioavailability of dietary polyphenols: factors contributing to their clinical application in CNS diseases. Neurochem. Int. 89, 198–208. doi:10.1016/j.neuint.2015.07.003
Pandey, V. K., Mathur, A., and Kakkar, P. (2019). Emerging role of Unfolded Protein Response (UPR) mediated proteotoxic apoptosis in diabetes. Life Sci. 216, 246–258. doi:10.1016/j.lfs.2018.11.041
Pan, H., Gray, R., Braybrooke, J., Davies, C., Taylor, C., McGale, P., et al. (2017). 20-Year risks of breast-cancer recurrence after stopping endocrine therapy at 5 years. N. Engl. J. Med. 377 (19), 1836–1846. doi:10.1056/NEJMoa1701830
Rahal, A., Kumar, A., Singh, V., Yadav, B., Tiwari, R., Chakraborty, S., et al. (2014). Oxidative stress, prooxidants, and antioxidants: the interplay. Biomed. Res. Int. 2014, 761264. doi:10.1155/2014/761264
Rawal, S., and Patel, M. M. (2019). Threatening cancer with nanoparticle aided combination oncotherapy. J. Control Release 301, 76–109. doi:10.1016/j.jconrel.2019.03.015
Ren, Z., Chen, S., Qing, T., Xuan, J., Couch, L., Yu, D., et al. (2017). Endoplasmic reticulum stress and MAPK signaling pathway activation underlie leflunomide-induced toxicity in HepG2 Cells. Toxicology 392, 11–21. doi:10.1016/j.tox.2017.10.002
Salehi, B., Calina, D., Docea, A. O., Koirala, N., Aryal, S., Lombardo, D., et al. (2020). Curcumin’s nanomedicine formulations for therapeutic application in neurological diseases. J. Clin. Med. 9 (2), 430. doi:10.3390/jcm9020430
Salehi, B., Sestito, S., Rapposelli, S., Peron, G., Calina, D., Sharifi-Rad, M., et al. (2018). Epibatidine: a promising natural alkaloid in health. Biomolecules 9 (1), 6. doi:10.3390/biom9010006
Seo, E., Lee, E. K., Lee, C. S., Chun, K. H., Lee, M. Y., and Jun, H. S. (2014). Psoralea corylifolia L. seed extract ameliorates streptozotocin-induced diabetes in mice by inhibition of oxidative stress. Oxid. Med. Cell. Longev. 2014, 897296. doi:10.1155/2014/897296
Shen, L., Zhao, P., and Niu, J. (2007). Effect of psoralen on proliferation of human breast carcinoma cells. Chin. Pharmacol. Bull. (11), 1448–1451.
Siegel, R. L., Miller, K. D., Fuchs, H. E., and Jemal, A. (2021). Cancer statistics, 2021. CA Cancer J. Clin. 71 (1), 7–33. doi:10.3322/caac.21654
Simpson, E., and Brown, H. L. (2018). Understanding osteosarcomas. Jaapa 31 (8), 15–19. doi:10.1097/01.JAA.0000541477.24116.8d
Smith, D. A., and Macdonald, S. (2014). A rare case of acute hepatitis induced by use of Babchi seeds as an Ayurvedic remedy for vitiligo. BMJ Case Rep. 2014, bcr2013200958. doi:10.1136/bcr-2013-200958
Somani, G., Kulkarni, C., Shinde, P., Shelke, R., Laddha, K., and Sathaye, S. (2015). In vitro acetylcholinesterase inhibition by psoralen using molecular docking and enzymatic studies. J. Pharm. Bioallied Sci. 7 (1), 32–36. doi:10.4103/0975-7406.148775
Song, T. H., Chen, X. X., Lee, C. K., Sze, S. C. W., Feng, Y. B., Yang, Z. J., et al. (2019a). Dendrobine targeting JNK stress signaling to sensitize chemotoxicity of cisplatin against non-small cell lung cancer cells in vitro and in vivo. Phytomedicine 53, 18–27. doi:10.1016/j.phymed.2018.06.018
Song, L., Yu, B., Yang, L., Wang, Z. X., Zhang, Y., Yu, Y. L., et al. (2019b). The mechanism of Psoralen and Isopsoralen hepatotoxicity as revealed by hepatic gene expression profiling in SD rats. Basic Clin. Pharmacol. Toxicol. 125 (6), 527–535. doi:10.1111/bcpt.13287
Sui, X., Liu, T., Liu, J., Zhang, J., Zhang, H., Wang, H., et al. (2020). Ultrasonic-enhanced surface-active ionic liquid-based extraction and defoaming for the extraction of psoralen and isopsoralen from Psoralea corylifolia seeds. Ultrason. Sonochem 69, 105263. doi:10.1016/j.ultsonch.2020.105263
Sun, S. J., Zhao, W. J., Xiang, Y., Chen, N. N., Sun, F., Chang, X. H., et al. (2013). Photochemotherapy with psoralen and ultraviolet A induced apoptosis of NB4 cells and its effects on caspase-8 and caspase-8 protein expressions. Zhongguo Zhong Xi Yi Jie He Za Zhi 33 (4), 502–505.
Sung, H., Ferlay, J., Siegel, R. L., Laversanne, M., Soerjomataram, I., Jemal, A., et al. (2021). Global cancer statistics 2020: GLOBOCAN estimates of incidence and mortality worldwide for 36 cancers in 185 countries. CA Cancer J. Clin. 71 (3), 209–249. doi:10.3322/caac.21660
Sun, S., Wang, M., Yuan, Y., Ding, H., Liang, C., Li, X., et al. (2022). A new strategy for the rapid identification and validation of direct toxicity targets of psoralen-induced hepatotoxicity. Toxicol. Lett. 363, 11–26. doi:10.1016/j.toxlet.2022.05.002
Tajiri, K., and Shimizu, Y. (2008). Practical guidelines for diagnosis and early management of drug-induced liver injury. World J. Gastroenterol. 14 (44), 6774–6785. doi:10.3748/wjg.14.6774
Tan, Y. S., Mhoumadi, Y., and Verma, C. S. (2019). Roles of computational modelling in understanding p53 structure, biology, and its therapeutic targeting. J. Mol. Cell. Biol. 11 (4), 306–316. doi:10.1093/jmcb/mjz009
Taylor, C. W., and Kirby, A. M. (2015). Cardiac side-effects from breast cancer radiotherapy. Clin. Oncol. R. Coll. Radiol. 27 (11), 621–629. doi:10.1016/j.clon.2015.06.007
Thakur, A., Sharma, R., Jaswal, V. S., Nepovimova, E., Chaudhary, A., and Kuca, K. (2020). Psoralen: a biologically important coumarin with emerging applications. Mini Rev. Med. Chem. 20 (18), 1838–1845. doi:10.2174/1389557520666200429101053
Trajkovic, K., Hsu, C., Chiantia, S., Rajendran, L., Wenzel, D., Wieland, F., et al. (2008). Ceramide triggers budding of exosome vesicles into multivesicular endosomes. Science 319 (5867), 1244–1247. doi:10.1126/science.1153124
Urra, H., Dufey, E., Lisbona, F., Rojas-Rivera, D., and Hetz, C. (2013). When ER stress reaches a dead end. Biochim. Biophys. Acta 1833 (12), 3507–3517. doi:10.1016/j.bbamcr.2013.07.024
Vidal, A., and Koff, A. (2000). Cell-cycle inhibitors: three families united by a common cause. Gene 247 (1-2), 1–15. doi:10.1016/s0378-1119(00)00092-5
Viola, G., Fortunato, E., Cecconet, L., Disarò, S., and Basso, G. (2007). Induction of apoptosis in Jurkat cells by photoexcited psoralen derivatives: implication of mitochondrial dysfunctions and caspases activation. Toxicol Vitro 21 (2), 211–216. doi:10.1016/j.tiv.2006.09.016
Vogel, C. F. A., van Winkle, L. S., Esser, C., and Haarmann-Stemmann, T. (2020). The aryl hydrocarbon receptor as a target of environmental stressors - implications for pollution mediated stress and inflammatory responses. Redox Biol. 34, 101530. doi:10.1016/j.redox.2020.101530
Wang, Y. K., Li, W. Q., Xia, S., Guo, L., Miao, Y., and Zhang, B. K. (2021). Metabolic activation of the toxic natural products from herbal and dietary supplements leading to toxicities. Front. Pharmacol. 12, 758468. doi:10.3389/fphar.2021.758468
Wang, G., Dinkins, M., He, Q., Zhu, G., Poirier, C., Campbell, A., et al. (2012a). Astrocytes secrete exosomes enriched with proapoptotic ceramide and prostate apoptosis response 4 (PAR-4): potential mechanism of apoptosis induction in Alzheimer disease (AD). J. Biol. Chem. 287 (25), 21384–21395. doi:10.1074/jbc.M112.340513
Wang, X., Cheng, K., Han, Y., Zhang, G., Dong, J., Cui, Y., et al. (2016a). Effects of psoralen as an anti-tumor agent in human breast cancer MCF-7/ADR cells. Biol. Pharm. Bull. 39 (5), 815–822. doi:10.1248/bpb.b15-00957
Wang, X., Lou, Y. J., Wang, M. X., Shi, Y. W., Xu, H. X., and Kong, L. D. (2012b). Furocoumarins affect hepatic cytochrome P450 and renal organic ion transporters in mice. Toxicol. Lett. 209 (1), 67–77. doi:10.1016/j.toxlet.2011.11.030
Wang, X., Peng, P., Pan, Z., Fang, Z., Lu, W., and Liu, X. (2019a). Psoralen inhibits malignant proliferation and induces apoptosis through triggering endoplasmic reticulum stress in human SMMC7721 hepatoma cells. Biol. Res. 52 (1), 34. doi:10.1186/s40659-019-0241-8
Wang, X., Xu, C., Hua, Y., Cheng, K., Zhang, Y., Liu, J., et al. (2018). Psoralen induced cell cycle arrest by modulating Wnt/β-catenin pathway in breast cancer cells. Sci. Rep. 8 (1), 14001. doi:10.1038/s41598-018-32438-7
Wang, X., Xu, C., Hua, Y., Sun, L., Cheng, K., Jia, Z., et al. (2016b). Exosomes play an important role in the process of psoralen reverse multidrug resistance of breast cancer. J. Exp. Clin. Cancer Res. 35 (1), 186. doi:10.1186/s13046-016-0468-y
Wang, Y., Zhang, H., Jiang, J. M., Zheng, D., Chen, Y. Y., Wan, S. J., et al. (2019b). Hepatotoxicity induced by psoralen and isopsoralen from Fructus Psoraleae: wistar rats are more vulnerable than ICR mice. Food Chem. Toxicol. 125, 133–140. doi:10.1016/j.fct.2018.12.047
Wu, Y., Zhang, Y. Z., Li, M. J., Yang, W. Q., and Cheng, L. F. (2022). The in vitro effect of psoralen on glioma based on network pharmacology and potential target research. Evid. Based Complement. Altern. Med. 2022, 1952891. doi:10.1155/2022/1952891
Xia, Q., Wei, L., Zhang, Y., Kong, H., Shi, Y., Wang, X., et al. (2018). Psoralen induces developmental toxicity in zebrafish embryos/larvae through oxidative stress, apoptosis, and energy metabolism disorder. Front. Pharmacol. 9, 1457. doi:10.3389/fphar.2018.01457
Xu, X., Zhang, X., Yuan, Y., Zhao, Y., Fares, H. M., Yang, M., et al. (2021). Species-specific differences in aryl hydrocarbon receptor responses: how and why? Int. J. Mol. Sci. 22 (24), 13293. doi:10.3390/ijms222413293
Yin, J., Xiang, C., and Song, X. (2016). Nanoencapsulation of psoralidin via chitosan and Eudragit S100 for enhancement of oral bioavailability. Int. J. Pharm. 510 (1), 203–209. doi:10.1016/j.ijpharm.2016.05.007
Yu, Y., Wang, P., Yu, R., Lu, J., Jiang, M., and Zhou, K. (2019). Long-term exposure of psoralen and isopsoralen induced hepatotoxicity and serum metabolites profiles changes in female rats. Metabolites 9 (11), 263. doi:10.3390/metabo9110263
Yu, Y., Yu, R., Men, W., Zhang, P., Zhang, Y., Song, L., et al. (2020). Psoralen induces hepatic toxicity through PERK and ATF6 related ER stress pathways in HepG2 cells. Toxicol. Mech. Methods 30 (1), 39–47. doi:10.1080/15376516.2019.1650150
Zanger, U. M., and Schwab, M. (2013). Cytochrome P450 enzymes in drug metabolism: regulation of gene expression, enzyme activities, and impact of genetic variation. Pharmacol. Ther. 138 (1), 103–141. doi:10.1016/j.pharmthera.2012.12.007
Zehir, A., Benayed, R., Shah, R. H., Syed, A., Middha, S., Kim, H. R., et al. (2017). Mutational landscape of metastatic cancer revealed from prospective clinical sequencing of 10,000 patients. Nat. Med. 23 (6), 703–713. doi:10.1038/nm.4333
Zhang, C., Fan, S., Zhao, J. Q., Jiang, Y., Sun, J., and Li, H. (2023). Transcriptomics and metabolomics reveal the role of CYP1A2 in psoralen/isopsoralen-induced metabolic activation and hepatotoxicity. Phytother. Res. 37 (1), 163–180. doi:10.1002/ptr.7604
Zhang, C., Zhao, J. Q., Sun, J. X., and Li, H. J. (2022b). Psoralen and isopsoralen from Psoraleae Fructus aroused hepatotoxicity via induction of aryl hydrocarbon receptor-mediated CYP1A2 expression. J. Ethnopharmacol. 297, 115577. doi:10.1016/j.jep.2022.115577
Zhang, R., Shi, W., Li, L., Huang, X., Xu, D., and Wu, L. (2019). Biological activity and health promoting effects of psoralidin. Pharmazie 74 (2), 67–72. doi:10.1691/ph.2019.8619
Zhang, W., Xie, H. Q., Li, Y., Zhou, M., Zhou, Z., Wang, R., et al. (2022a). The aryl hydrocarbon receptor: a predominant mediator for the toxicity of emerging dioxin-like compounds. J. Hazard Mater 426, 128084. doi:10.1016/j.jhazmat.2021.128084
Zhang, Y., Wang, Q., Wang, Z. X., Bi, Y. N., Yuan, X. M., Song, L., et al. (2018). A study of NMR-based hepatic and serum metabolomics in a liver injury sprague-dawley rat model induced by psoralen. Chem. Res. Toxicol. 31 (9), 852–860. doi:10.1021/acs.chemrestox.8b00082
Zhou, Q. H., Zhu, Y. D., Zhang, F., Song, Y. Q., Jia, S. N., Zhu, L., et al. (2019). Interactions of drug-metabolizing enzymes with the Chinese herb Psoraleae Fructus. Chin. J. Nat. Med. 17 (11), 858–870. doi:10.1016/S1875-5364(19)30103-7
Zhou, W., Chen, X., Zhao, G., Xu, D., Jiang, Z., Zhang, L., et al. (2018). Psoralen induced liver injury by attenuating liver regenerative capability. Front. Pharmacol. 9, 1179. doi:10.3389/fphar.2018.01179
Zhu, K., Liu, L., Zhang, J., Wang, Y., Liang, H., Fan, G., et al. (2016). MiR-29b suppresses the proliferation and migration of osteosarcoma cells by targeting CDK6. Protein Cell. 7 (6), 434–444. doi:10.1007/s13238-016-0277-2
Keywords: psoralen, tumor, apoptosis, hepatotoxicity, molecular mechanism, natural products
Citation: Meng D, Dong Y, Shang Q and Sun Z (2024) Anti-tumor effect and hepatotoxicity mechanisms of psoralen. Front. Pharmacol. 15:1442700. doi: 10.3389/fphar.2024.1442700
Received: 02 June 2024; Accepted: 18 July 2024;
Published: 05 August 2024.
Edited by:
Lekshmi R. Nath, Amrita Vishwa Vidyapeetham, IndiaReviewed by:
Agnieszka Gunia-Krzyżak, Jagiellonian University Medical College, PolandVinod B. S., Sree Narayana College, Kollam, India
Alex Boye, University of Cape Coast, Ghana
Copyright © 2024 Meng, Dong, Shang and Sun. This is an open-access article distributed under the terms of the Creative Commons Attribution License (CC BY). The use, distribution or reproduction in other forums is permitted, provided the original author(s) and the copyright owner(s) are credited and that the original publication in this journal is cited, in accordance with accepted academic practice. No use, distribution or reproduction is permitted which does not comply with these terms.
*Correspondence: Qingxin Shang, c2hhbmdxeDA1MzFAMTYzLmNvbQ==; Ziyuan Sun, eml5dWFuc3p5QDE2My5jb20=
†These authors have contributed equally to this work and share first authorship