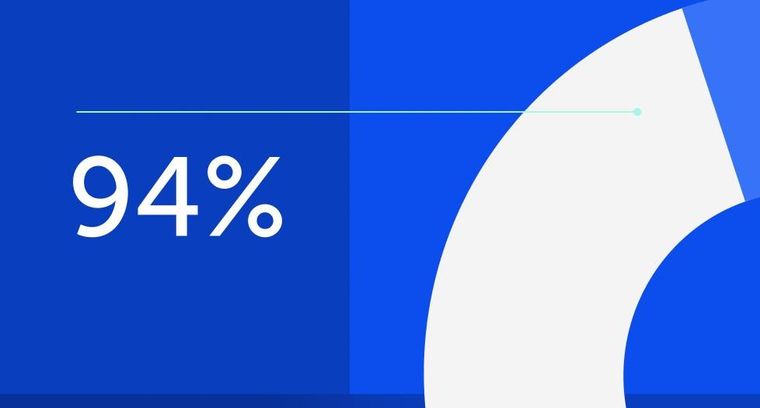
94% of researchers rate our articles as excellent or good
Learn more about the work of our research integrity team to safeguard the quality of each article we publish.
Find out more
REVIEW article
Front. Pharmacol., 31 July 2024
Sec. Integrative and Regenerative Pharmacology
Volume 15 - 2024 | https://doi.org/10.3389/fphar.2024.1437457
This article is part of the Research TopicIntegrative Pharmacological Approaches for Regenerating Cartilage and Bone TissueView all 11 articles
Bone defects caused by tumors, osteoarthritis, and osteoporosis attract great attention. Because of outstanding biocompatibility, osteogenesis promotion, and less secondary infection incidence ratio, stimuli-responsive biomaterials are increasingly used to manage this issue. These biomaterials respond to certain stimuli, changing their mechanical properties, shape, or drug release rate accordingly. Thereafter, the activated materials exert instructive or triggering effects on cells and tissues, match the properties of the original bone tissues, establish tight connection with ambient hard tissue, and provide suitable mechanical strength. In this review, basic definitions of different categories of stimuli-responsive biomaterials are presented. Moreover, possible mechanisms, advanced studies, and pros and cons of each classification are discussed and analyzed. This review aims to provide an outlook on the future developments in stimuli-responsive biomaterials.
Worldwide incidences of bone defects and other bone-related complications, including bone infection, bone tumor, and bone loss, have increased owing to the growing life expectancy and the aging population (Bose et al., 2023). Among all clinical available bone grafts, autogenous ones are the gold standard (Wang and Yeung, 2017). However, deficient blood supply and morbidity of donor site complications are the main limitations. Though allografts take up the second higher option, they also have several drawbacks, including secondary injury, limited resources, risk of infectious disease, and immunological rejection (De Long et al., 2007; Wei et al., 2022a). Moreover, various new bone restorative strategies have emerged, which comprise tissue-engineered periosteum and platelet-rich plasma (Fang et al., 2020; Zhang et al. (2022b). Nevertheless, because of the inadequate clinical application, their capacity for hard-tissue regeneration and drug delivery precision should be further verified (Bose et al., 2019; Fan et al., 2021; Losic, 2021). Hence, investigations on more intelligent bone grafting materials for treating bone disorders and reconstructing bone defects have radically become the focus in this field.
Bone tissue engineering (BTE) is a novel tactic for promoting bone regeneration by combining biomaterials and bioactive factors to encourage cells to migrate, attach and proliferate (Shang et al., 2021). Consequently, ideal restorative biomaterials should perform good mechanical strength, biocompatibility and steadily controllable drug release ability (Handa et al., 2022; Ni et al., 2023). Stimuli-responsive biomaterials are newly developed alternative materials for BTE, opening new frontiers in the development of scaffolds and implants. These biomaterials can mimic the dynamic nature of the native extracellular matrix, providing a more conducive environment for cell growth, differentiation, and bone regeneration. Therefore, stimuli-responsive biomaterials occupy a critical position in the development of restorative bone grafts.
Stimuli-responsive biomaterials for BTE include scaffolds, nanoparticles, biopolymers, hydrogel and so on. When these materials are exposed to certain stimuli, their mechanical properties, shape, form or drug-releasing rate may change accordingly to match the properties of the original bone tissues, establish tight connection with ambient hard tissue, and provide adequate mechanical strength (Lin et al., 2013; Islam et al., 2020; Sadowska and Ginebra, 2020; Wang et al., 2020; Shang et al., 2021). These materials are promising in clinical application. For instance, stimuli-responsive biomaterials like pH-responsive chitosan (CS) have been widely utilized in bone regeneration (Arora et al., 2023).
Stimuli-responsive biomaterials can be categorized into two main types: 1) external stimuli-responsive biomaterials; 2) internal stimuli-responsive biomaterials (Figure 1) (Wei et al., 2022a). External stimuli-responsive biomaterials include implants that can change their properties after being activated by external stimuli. The activated materials can affect the cell fate and enhance bone tissue regeneration by altering the mechanical characteristics, breaking chemical bonds, or causing other transformations (Lui et al., 2019). On the contrary, internal stimuli-responsive biomaterials are the ones that can respond to specific microenvironmental changes surrounding the lesion, such as decrease in pH, alteration of temperature, increase in reactive oxygen species (ROS), and fluctuation in enzyme levels (Du et al., 2019). Recently, stimuli-responsive biomaterials have been widely studied in controlling the release of loaded drugs (Yadav et al., 2023). By specific stimuli activating, changes are triggered in the stimuli-responsive biomaterials, which include self-assembly of peptides, breakage of chemical bonds, and alteration in release behaviors (Li et al., 2024; Mu et al., 2024). Thus, the activated materials can modulate certain cellular pathways related to the induction of bone regeneration.
In this review, recent studies on the development and mechanism of stimuli-responsive biomaterials in bone regeneration are summarized. An outlook on future advancements in stimuli-responsive biomaterials has also been provided.
External or out-body stimuli are signals or irradiations applied outside the body (Fang et al., 2021; Montoya et al., 2021). External stimuli include electricity, light, ultrasound, and magnetic field. External stimuli-responsive biomaterials undergoes conformational changes, reversible microphase separation, or self-assembly according to the stimulus (Figure 2) (Montoya et al., 2021). Subsequently, the activated materials can affect cell attachment, migration, and proliferation to enhance osteogenesis (Wei et al., 2022a).
Electrical stimulation (EStim) can improve bone regeneration by promoting bone-forming stem cells activities like migration, proliferation, differentiation, mineralization, extracellular matrix deposition, and attachment to scaffold materials (George et al., 2017; Leppik et al., 2020). Generally, there are two kinds of EStim, direct (external electric field) and indirect ones (piezoelectrical field) (Zhu et al., 2023).
When exposed to the certain external electric stimulator, electro-responsive composites can deliver localized electrical signals (Wei et al., 2022b). Followingly, these signals can stimulate the calcium–calmodulin pathway or induce the transformation of other cytokines, which form the basis of osteogenesis (Wei et al., 2022b). Under exogenous electrical stimulation, the delivered electrical signals can contribute to upregulating level of transforming growth factor-β (TGF-β), bone morphogenetic proteins-2 (BMP-2) and the expression of Runt-related transcription factor 2 (Runx2), Osteocalcin (OCN), and Osteopontin (OPN) (Fu et al., 2019; Khare et al., 2020; Wei et al., 2022b).
Zhang et al. prepared polypyrrole (PPY) nanoparticles dispersed in a chitosan matrix with BMP-2 covalently immobilized on the surface (PPY/chitosan films) (Zhang et al., 2013). When osteoblasts cultured on the electrical stimulated PPY/chitosan film, greater increasing in cellular metabolic activity, ALP activity and mineralization over control group were observed (Zhang et al., 2013). Moreover, osteogenic gene expression analysis showed that releasing of BMP-2 and exogenous electrical stimulation did have synergistic effects on osteoblast differentiation and maturation (Zhang et al., 2013).
Piezoelectric scaffolds are biomaterials with a uniquely porous morphology (Sultana et al., 2017; Chorsi et al., 2019; Liu et al., 2023). Under mechanical stress, transient deformation of piezoelectric scaffold occurs, leading to an atomic position shift (Mao et al., 2022). This phenomenon results in the loss of the center of symmetry and induces the accumulation of charge through an ordered dipole distribution (Mao et al., 2022). Therefore, piezoelectric scaffolds can generate electrical charges in response to applied mechanical stress or deformation (Turner et al., 2001; Halperin et al., 2004; Minary-Jolandan and Yu, 2010). The stress-generated electrical signal can activate voltage-gated Ca2+ channels, promote displacement of C=O bonds, and favor the M2 phenotype of macrophages, leading to charge alteration on the cell membrane (Balint et al., 2014; Ud-Din and Bayat, 2014; More and Kapusetti, 2017; Murillo et al., 2017). Charge transfer promotes regenerative activities by modulating cellular behavior through molecular pathways, such as phosphatidylinositol 3-kinase (PI3K) and phosphatase and tensin homolog (Balint et al., 2014; Ud-Din and Bayat, 2014; More and Kapusetti, 2017; Murillo et al., 2017).
Representative piezoelectric scaffolds for efficient osteogenesis, including piezo-bioceramics (e.g., barium titanate (BaTiO3), magnesium silicate, zinc oxide) and some piezo-biopolymers (e.g., polyvinylidene fluoride, polyhydroxybutyrate, collagen, etc.), have been extensively studied (Jacob et al., 2018; Khare et al., 2020). For example, piezoelectric BaTiO3 was integrated into bioactive nano-titania ceramics as TiO2/BaTiO3 composite ceramics scaffolds (Li et al., 2009). BaTiO3 could adjust the elastic modulus of the scaffolds analogous to that of human bone (Li et al., 2009). Meanwhile, the piezoelectric properties of BaTiO3 also showed the enhancing effects on the bioactivity, which made the osteoblasts proliferate faster on the scaffolds in vitro (Li et al., 2009). Moreover, Wang’s team developed a piezoelectric poly (vinylidene fluoride-trifluoroethylene) (PVDF-TrFE) scaffold (Wang et al., 2018). Under physiological loads, the scaffold in the defect area can generate electrical potential (Wang et al., 2018). Once the osteoblasts were stimulated by the scaffold surface charges, osteoblastic proliferation would be enhanced, which promoted bone regeneration in the defect area (Wang et al., 2018). Furthermore, via electrospinning, Barbosa et al. filled PVDF-TrFE scaffolds with hydroxyapatite (HAp) (PVDF-TrFE/HAp) (Barbosa et al., 2023). Besides cell proliferation enhancement, PVDF-TrFE/HAp can boost bone tissue mineralization process and enhance the osteogenic differentiation (Barbosa et al., 2023). Also, the collagen-based composite scaffolds have been reported for efficient hard tissue engineering (Zhang et al., 2023). The compressive force on collagen triggered the re-organization of dipole moment and generated negative charges, which prompted the electrical stimulation to the cells and leads to the opening of voltage-gated Ca2+ channels (Zhang et al., 2023). This activity can subsequently activate the expression of osteogenesis related genes like TGFβ, BMP-2 (Zhang et al., 2023).
Photo-responsive composites are stimuli-responsive materials with minimal damage toward normal tissues and easily remote-control properties (Zhao et al., 2019a; Jamnongpak et al., 2024). When exposed to certain wavelength of light, the drug delivery ratio, shape or surface charges of photo-responsive composites may be altered in response to different categories of light (Xing et al., 2023). Lights that are commonly used for photo-responsive therapy comprise 1) visible and 2) near-infrared (NIR) lights; their wavelengths fall between 400–700 nm and 700–1,300 nm, respectively, and can penetrate most tissues to reach the target area (Escudero et al., 2019; Wan et al., 2020). For example, in vivo studies verified that when an 810-nm NIR light was applied to a rat’s head, 51% of the laser could transmit through the skull and 40% through the scalp and skull in the prefrontal regions (Salehpour et al., 2019).
In Zhang’s work, an NIR light-responsive scaffold that contained shape memory polyurethane (SMPU) as an SMPU/Mg composite porous scaffold was utilized (Zhang et al., 2022b). Before being implanted into the defect area, shape memory composites were programmed to a certain size (Zhang et al., 2022b). Upon exposure to NIR light, the form-programmed shape memory composites recovered, achieving tight connection with the surrounding hard tissue (Figure 3) (Zhang et al., 2022b). Accordingly, the shape memory composites precisely repaired the defective bone tissue (Zhang et al., 2022b). Moreover, Yan et al. combined graphitic carbon nitride (g-C3N4), reduced graphene oxide (rGO) with Ti-based orthopedic implant (rGO/CN/TO) (Yan et al., 2022). Under blue LED exposure, the rGO/CN/TO ternary nanocoating exhibited higher open circuit potential and transient photocurrent density (Yan et al., 2022). This exerted greater effects on enhancing osteogenic differentiation of MC3T3-E1 cells through increasing Ca2+ influx under visible-light stimulation (Yan et al., 2022). Therefore, the implant was proved to be able to stimulate the regeneration of bone and nerves.
Figure 3. Procedure for bone regeneration using shape memory biomaterials (Reproduced with permission., Zhang et al. (2022b), Bioactive Materials).
Ultrasound-responsive composites represent a category of stimuli-responsive biomaterials with the capacity to regulate protein release, electric charges level, structure alternation, etc., by reacting to external ultrasound radiation (Brudno and Mooney, 2015). When induced by intensity-elevated ultrasound, certain chemical linkage breaks occur, such as Diels-Alder linker, fatty acid ester bonds, and phosphoester bonds (Suslick, 2013). Thereafter, the loaded bone formation-related components are released, such as cyclooxygenase 2, prostaglandin E2, short-chain fatty acid, and dopamine-functionalized hyaluronic acid ions, which can facilitate stem cells proliferation and differentiation (Veronick et al., 2018; An et al., 2021; Arrizabalaga et al., 2022; Zhou et al., 2024). Thus, combining and modulating composites with ultrasound can enhance osteoblastic response considerably and expedite the mineralization of hard issues (Moonga and Qin, 2018; Veronick et al., 2018; Fan et al., 2020).
A myriad of multifunctional biomaterials that utilize ultrasound as stimulation to enhance osteogenesis expression were fabricated recently. Among these biomaterials, the ultrasound-responsive nanofiber hydrogel (UPN@hydrogel) has provided a novel strategy for bone repair (Zhang et al., 2024). These nanofibers can be released from hydrogel in a time-dependent manner upon ultrasound stimulation (Zhang et al., 2024). Then, nanofibers could activate M2 macrophages to secrete BMP-2 and insulin-like growth factor 1, accelerating the osteogenic differentiation of BMSCs (Zhang et al., 2024). Similarly, when targeted with focused ultrasound, crosslinking chitosan also show ability in regulating BMSCs differentiation via the breakage of innate two distinct Diels-Alder linkers and the release entrapped cytokines (Pajarinen et al., 2019; Arrizabalaga et al., 2022). Moreover, combination of ultrasound irradiation and gene-activated matrix-based therapeutics also showed promising outcomes by responsively releasing osteogenesis-related peptides, such as BMP-2/7 (Nomikou et al., 2018). In addition, with assistance of ultrasound, piezoelectric nylon-11 nanoparticles (nylon-11 NPs) could promote the osteogenic differentiation of dental pulp stem cells efficiently in a noninvasive way (Ma et al., 2019). Therefore, nylon-11 NPs are promising to be used in BTE.
Lately, researchers have integrated magnetic nanoparticles, such as Co3O4, Fe3O4 or MnFe3O4 nanoparticles, into various matrices to fabricate magnetic composites, exploring the potential for application as bone scaffolds or substitutes (Xu and Gu, 2014; Lui et al., 2019). When subjected to an external magnetic field, magneto-responsive nanocomposites exhibit magnetic twisting or clustering responsiveness and can function as carriers for biologically or chemically active agents via magneto-driven delivery (Cojocaru et al., 2022). By delivering bone-forming substances, such as BMP-2 and dexamethasone acetate, magneto-responsive nanocomposites are conducive for bone regeneration (Butoescu et al., 2009; Wang et al., 2012).
Magneto-responsive nanocomposites allow several processing options. Tang et al. designed a magneto-responsive CoFe2O4/P(VDF-TrFE) nanocomposite (Tang et al., 2021). Cellular osteogenic differentiation can be enhanced when the nanocomposite is exposed to magnetic field. Moreover, this material can significantly upregulate the expression level of α2β1 integrin and p-ERK, which exhibited promising potential in bone tissue repair and regeneration. Meanwhile, Wu et al. fabricated a ceramic composite containing super-paramagnetic nanoparticles (Wu et al., 2010). In vivo experiments demonstrated that the super-paramagnetic nanoparticles integrated in the composites accelerated new bone-like tissue formation (Wu et al., 2010).
Magnetic Resonance Imaging (MRI) is widely used for clinical examinations. It is a common intermittent pulsing electromagnetic field. When magneto-responsive nanocomposites are exposed to MRI, the stiffness of magneto-responsive nanocomposites increases due to the rearrangement of magnetic particles (Li et al., 2020). Then, integrins on the stem cell membrane transferred information about the mechanical state of the extracellular matrix into cells (Li et al., 2020). Activating osteogenic differentiation signal pathways, such as the PI3K/Akt pathway, enhances stiffness, increases the number of mitochondria, and changes cell morphology (Yan et al., 1998; Lambertini et al., 2015; Androjna et al., 2021). These results suggest that stem cell osteogenic differentiation can be regulated; MRI appears to positively affect magneto-responsive nanocomposites when used for patient examinations.
External stimuli-responsive biomaterials permit noninvasive activation and remote control (Yang et al., 2018; Lin et al., 2020; Wan et al., 2020; Zhao et al., 2020). In addition, targeted drug delivery to specific sites within the body can be achieved via out-body stimulation such as magnetic guidance (Lu et al., 2018; Lui et al., 2019). However, it is challenging to achieve optimal performance and responses. Moreover, external stimuli, such as NIR light and ultrasound, may generate heat in the tissue, leading to thermal damage (Sun D. et al., 2020; Arrizabalaga et al., 2022). Therefore, further studies are needed to improve this type of biomaterials. More details on the advantages and disadvantages of stimuli-responsive biomaterials are listed in Table 1.
Internal or in-body stimulus refers to signals in the microenvironment inside the body around the biomaterial (Lee et al., 2018a; Nguyen et al., 2018; Yao et al., 2019; Ding et al., 2022). These stimuli comprise of pH, temperature, ROS, enzyme ion concentration and etc. Drug carriers of internal stimulus-responsive biomaterials can be designed to respond to specific triggers, such as pH changes, ROS fluctuations, or the presence of specific biomolecules. Thus, internal stimulus-responsive biomaterials are able to deliver the loaded medicine to the target area or release certain ions when the concentration of the chemical substance changes (Figure 4) (Hein et al., 2008; Lallana et al., 2012; McKay and Finn, 2014; Gregoritza and Brandl, 2015). Furthermore, the released medicine can exert an impact on the metabolism of a series of osteocytes. This level of precision minimizes the side effects and enhances the overall efficacy of the treatment. To develop a direct approach for bone regeneration, various studies focusing on internal-responsive biomaterials have been performed.
The pathological circumstance, such as tumor or inflammation, is mildly acidic, whereas the physiological pH is neutral (7.0-7.4) (Swietach et al., 2014). pH-responsive composites have the capacity of reacting to specific pH levels in physiological and pathological environments (Li et al., 2023; Xu et al., 2023; Zheng et al., 2023). The composites have pH-sensitive coordination bonds, such as catechins, ferric irons, citraconic amide bonds, and Schiff base bonds. These bonds can change with the fluctuation in pH levels (Liang et al., 2021; Jo et al., 2023). Therefore, the state of composites can alter accordingly (Kocak et al., 2020; Zhu et al., 2021). Drugs related to BTE can be delivered by alteration between different states (Zhu et al., 2018; Constantin et al., 2020; Ding et al., 2022). When sensing the change in pH levels, these composites can set up targeted and localized drug delivery (Liu et al., 2017). Finally, certain drugs are able to upregulate the expression of Runx2, osterix, OCN, and OPN and support mesenchymal stem cells proliferation, adhesion, osteoinduction, and biocompatibility, which are essential to regenerate bone tissues (Lavanya et al., 2020).
To fabricate composites with pH-responsive drug release property, Tang et al. designed a hydrogel with a alendronate-modified oxidized alginate network (GelMA-OSA@ALNDN hydrogel) in which Schiff base bonds were distributed uniformly (Tang et al., 2022). Alendronate (ALN) is a type of bisphosphonate with promising hard tissue repair functions (Wang et al., 2021). Therefore, by reacting to changing pH level, GelMA-OSA@ALNDN hydrogel can maintain a stable drug concentration to activate the repair process in the defective bone area (Tang et al., 2022). Besides, an asymmetric microfluidic/chitosan hydrogel, poly [2-(dimethylamino) ethyl methacrylate] (PDMAEMA) hydrogel, was successfully fabricated (Chen et al., 2023). The hydrogel was demonstrated with the ability of achieving pH-responsive drug release to promote osteoblast proliferation and combating with bacterial infection simultaneously (Figure 5) (Chen et al., 2023).
Figure 5. (A) CCK-8 assay of osteoblasts seeded into wells (Control) and on the surface of the chitosan membrane. *p < 0.05. (B) LSCM images of osteoblasts in wells and attached to the chitosan surface. Scale bar in LSCM images is 50 µm. (C) SEM images of the surface of chitosan membrane in 200 µm scale (left), and osteoblasts attached on the chitosan surface after 5 days culturing in 5 µm scale (right). Reproduced with permission., Chen et al. (2023), MDPI.
Currently, researchers tend to synthetize CS-based biomaterials, which are natural polymers with enzymatic biodegradability, pH sensitivity, polycationic nature, extensive application, etc. (Almajidi et al., 2023). For example, pH-responsive carboxymethyl chitosan/amorphous calcium phosphate (CMC-ACP) hydrogel system was designed (Zhao et al., 2019b). This hydrogel system was found to significantly upregulate the expression of bone markers, such as Runx2, osterix, OCN, and OPN (Zhao et al., 2019b). In vivo findings also showed that the injectable hydrogel strongly enhances the efficiency and maturity of the bone regeneration while suppressing the bone resorption (Zhao et al., 2019b). Furthermore, Ressler et al. synthesized a pH-responsive chitosan-hydroxyapatite hydrogel (CS/HAp/NaHCO3) (Ressler et al., 2018). Activating by acidic microenvironment, CS/HAp/NaHCO3 can improve stability, homogenous dispersion of mesenchymal stem cell (MSCs) and promote calcium phosphate deposits and extracellular matrix (ECM) mineralization (Ressler et al., 2018).
Phase transition temperature refers to the temperature required to induce a change between phases, such as solid and liquid, sol and gel (Scott, 1974). Thermo-responsive composites exhibit reversible phase transition effect in response to change in temperature (Kim and Matsunaga, 2017). The rapid shift from a sol to a gel state at physiologic temperature can optimize the release of loaded TGF-β, drugs or ions, which has positive effect in boosting osteoblasts adherence, differentiation and proliferation (Duan et al., 2020; Khan et al., 2022).
To address the issue of the efficient local delivery of BMP-2 to complex bone fracture sites, BMP-2-functionalized MgFe-layered double hydroxide nanosheets were integrated into chitosan/silk fibroin hydrogels (CSP-LB hydrogel) (Lv et al., 2023). This thermo-responsive hydrogel solution can be injected to fit the defects precisely and then solidify quickly under 37°C condition (Lv et al., 2023). The solidified hydrogel showed 4.5-fold bone volume and 3.6-fold bone mineral density increment compared with that of the control group (Lv et al., 2023). Simultaneously, CS thermogels, supported with demineralized bone matrix, could retain more cells and provide better strength for efficient chondrogenesis in both in vitro and in vivo (Huang et al., 2014). Wu et al. constructed a thermo-sensitive N-isopropylacrylamide-chitosan hydrogel (Wu et al., 2018). This hydrogel ensured osteoinduction and biocompatibility (Wu et al., 2018). And these properties were proved by in vitro tests with infrapatellar fat pad-derived mesenchymal stem cells (IFP-MSCs), fibroblasts (NIH-3T3), and osteoblasts (MC3T3-E1) (Wu et al., 2018).
ROS are a family of reactive chemical species that contain oxygen (Zorov et al., 2014). ROS have been considered pivotal signaling molecules in many physiological processes and are usually overproduced in various inflammatory tissues (Yao et al., 2019). These molecules can influence the differentiation of osteoclasts (Badila et al., 2022). ROS-responsive composites are a series of biomaterials that can target the high-level ROS in bone-related diseases (Ren et al., 2022). These scaffolds possess unique features under oxidative conditions (Figure 6) and can fit in different bone defects to achieve bone regeneration (Lee et al., 2013; Xu et al., 2016; Ren et al., 2022). Moreover, these materials can support osteocyte adhesion and growth owing to their porous structures to boost the BTE (Yao et al., 2019).
In order to renovate the adverse effect of ROS stimulation to bone regeneration, Martin et al. synthesized a layer-by-layer-compatible polycation (Yamaguchi et al., 1991). This polycation presented an ROS dose-dependent delivery of the preloaded BMP-2 and promoted the cellular proliferation of progenitor cells and spurred stem cell differentiation into bone-forming osteoblasts (Yamaguchi et al., 1991; Tian et al., 2022). Moreover, a 3D-printed ROS-responsive molybdenum (Mo)-containing bioactive glass ceramic scaffold was developed recently (Lee et al., 2018b). The scaffold decreased mitochondrial ROS produced by osteoclasts and increased the expressions of certain genes related to osteogenesis, such as matrix metalloprotein (MMP), NFATc1, and RANKL (Lee et al., 2018b). In the study by Lee et al., 3-dimension polycaprolactone (PCL) scaffold with tannic acid (TA) and BMP-2 (BMP-2/TA/PCL) was fabricated (Yang et al., 2020). In the ROS-overproduction environment, the BMP-2/TA/PCL scaffold maintained the sustained and controlled release of BMP-2, which stimulated the osteogenic differentiation of MC3T3-E1 cells by increasing ALP activity and calcium deposition (Figure 7) (Yang et al., 2020).
Figure 7. (A) Alkaline phosphatase activity and (B) calcium deposition of MC3T3-E1 cells cultured on PCL, TA/PCL, BMP-2/PCL, and BMP-2/TA/PCL scaffolds. Error bars represent mean ± SD. Reproduced with permission., Yang et al. (2020), MDPI.
Enzymes play pivotal roles in growth, blood coagulation, wound healing, respiration, digestion, and various other physiological processes (Yang et al., 2020). Disruptions in the expressions or activities of enzymes lead to severe pathological conditions, including but not limited to cancer, cardiovascular disorders, inflammation, and degenerative arthritis (Hu et al., 2014). Composites responsive to enzymes are activated by the selective catalytic activities of specific enzymes (Zhang et al., 2016). For example, type II collagen forms the base for the formation of cartilage and bone (Anjum et al., 2016; Ding et al., 2020; Fischer et al., 2021). Local gene expression and the secretion of type II collagen can be regulated by the overexpression of enzymes such as polygalacturonase, BMPs, and matrix metalloprotein (Anjum et al., 2016; Ding et al., 2020; Fischer et al., 2021).
Blood coagulation factor XIII (FXIIIa) is closely related to bone tissue repair. This enzyme can regulate the RANKL/RANK system in MSCs, augmenting osteoblast differentiation and mineralization (Ikebuchi et al., 2018; Dang et al., 2023). Therefore, Anjum et al. built an enzymatically formed chondroitin sulfate and poly (ethylene glycol) (PEG)-based hybrid hydrogel scaffold system (Anjum et al., 2016). Induced by FXIIIa-catalyzed glutaminating reaction, the degraded hydrogel released BMP-2, facilitating the integration of the newly formed bone tissue (Anjum et al., 2016).
Inbody ion level may shift in some physiological and pathological environment (Mészáros et al., 2022; Ye et al., 2022; Adella and de Baaij, 2023). Therefore, the fluctuation of ions concentration like Na+, K+, Ca2+ can be used as the internal stimulation to activate the ion-responsive scaffolds (Zhou et al., 2013; Xu et al., 2015). Certain ions loaded on the porous surface of the ion-responsive scaffolds would be displaced when the materials were activated. The released ions could automatically combine with hydroxy phosphates (Wang et al., 2023). The self-combination results in large hydroxyapatite-like crystals in vivo, accelerating bone remineralization and regeneration (Zhou et al., 2013; Gao et al., 2021; Wang et al., 2023).
Sun et al. used melt electrowritten printing technology to design a mineralized zeolitic imidazolate framework-8/polycaprolactone (ZIF-8/PCL) scaffold (Wang et al., 2023). In a simulated body fluid solution, Zn-N bonds and hydrogen bonds in ZIF-8 slightly decomposed because of the competitive binding of Ca ions, forming excess insoluble zinc hydroxy phosphates (Wang et al., 2023). Apatite and zinc positively promote bone regeneration by rising the biocompatibility of biomaterials and promoting tissue integration (Su et al., 2019; Gao et al., 2021). Accordingly, ZIF-8 showed a favorable impact on promoting osteogenesis. Moreover, by mixing ZIF-8 and PCL, the scaffold presented low inflammatory responses and increased biocompatibility (Wang et al., 2023).
Diabetes mellitus (DM) is an intricate disorder of glucose metabolism. The incidence of DM is projected to reach 10.2% by 2030 and 10.9% by 2045 (Saeedi et al., 2019). The irregular hyperglycemic environment makes the cells and tissues in dysfunction and hinder the osseointegration process (Khosla et al., 2021). Hence, biomaterials can effectively utilize superfluous glucose in blood to renovate the oppressed bone remodeling are fairly in need. And series glucose-responsive biomaterials have emerged. For instance, a functional glucose-responsive immunomodulation-assisted periosteal regeneration composite material, Polylactic Acid/Collagen I/Liposome-APY29 (PCLA), was constructed (Qiao et al., 2023). In the DM microenvironment, the high glucose can promote the combination of hydroxyl groups grafted in the glucose-responsive composites (Mohanty et al., 2022; Qiao et al., 2023). This makes the composite surface changes from hydrophobic to hydrophilic, which is beneficial to promote cell adhesion and proliferation (Mohanty et al., 2022; Qiao et al., 2023). Meanwhile, by blocking the IREα/NOD-like/NF-κB signaling pathway, PCLA can remodel the pathologic diabetic microenvironment into a regenerative microenvironment (Qiao et al., 2023). Moreover, He et al. devised a glucose-primed orthopedic polyetheretherketone (PEEK) implant (sP-P@C-G) composed of Cu-chelated metal-polyphenol network (hauberk coating) and glucose oxidase (GOx) (He et al., 2023). In hyperglycemic microenvironment, glucose-responsive enzymatic cascade can be activated by GOx to produce endogenous H2O2 (He et al., 2023). Then, dopamine (DA) anchor onto PEEK implant surfaces in the alkaline environment via self-polymerization (He et al., 2023). DA shows promising ability on facilitating cell attachment, which endows sP-P@C-G with appealing biocompatibility and outstanding osteogenicity (Chen et al., 2017; He et al., 2023).
When compared with external stimuli-responsive biomaterials, the primary benefit of internal stimuli-responsive biomaterials lies in their capacity for activation without external stimuli (Berillo et al., 2021). These materials may lower the cost of purchasing certain external machines and alleviate the workload of treatment (Boskey and Coleman, 2010). However, changes in the specific stimuli may happen under various conditions and may lead to the unintended activation of the materials (Chen et al., 2019; Zhang et al., 2020; Montoya et al., 2021). In addition, achieving a balance between sufficient stability of the materials and appropriate biodegradability can be challenging (Boskey and Coleman, 2010; Nguyen et al., 2018; Berillo et al., 2021).
Stimuli-responsive biomaterials have been considerably explored and have garnered substantial attention. As the interdisciplinary collaboration among materials science, biology, and medicine continues to flourish, the future holds exciting prospects for stimuli-responsive biomaterials. Nonetheless, despite the promising bone regeneration effect, stimuli-responsive biomaterials are still in infancy with challenges need to be settled:
1) Due to insufficient sample size, inadequate simulated conditions and deficient number of experiments, the clinical application evidence for most stimuli-responsive biomaterials is still limited. Therefore, more high-quality studies and preclinical studies are needed.
2) More and more studies tend to design multiple stimulation-responsive biomaterials. However, it remains to be discussed that whether applying two or more stimulus would obtain better bone regeneration effect than single one.
3) Proper application conditions for valid osteogenesis need to be verified. For example, feasible pH stimulation range for optimal bone regeneration effect, suitable magnetic field strength for BTE, etc.
4) Current synthetic processes of stimuli-responsive biomaterials are generally time-consuming and complicated. Thus, the exploration of safer and more efficient synthetic processes is necessary.
In a nutshell, although certain challenges persist and clinical translation remains a formidable task, it is conceivable that the integration of intelligent stimuli-responsive materials holds considerable promise for transformative biomedical applications in the future. Consequently, there is still a long way to go to reach the optimum of ideal stimuli-responsive biomaterials for bone regeneration.
KY: Investigation, Methodology, Writing–original draft, Writing–review and editing. ZW: Writing–original draft. KZ: Writing–original draft, Writing–review and editing. MW: Conceptualization, Writing–review and editing. HX: Writing–original draft, Writing–review and editing. LC: Writing–original draft, Writing–review and editing. XH: Conceptualization, Funding acquisition, Project administration, Writing–review and editing, Writing–original draft. WZ: Conceptualization, Funding acquisition, Project administration, Writing–original draft, Writing–review and editing.
The author(s) declare that financial support was received for the research, authorship, and/or publication of this article. This work was supported by the Joint Funds for the innovation of science and Technology, Fujian province (Grant number: 2021Y9026), Introduced Talents Grant of School and Hospital of Stomatology Fujian Medical University (Grant number: 2023KQYJ01), and Joint Funds for the innovation of science and Technology, Fujian province (Grant number: 2023Y9223).
The authors declare that the research was conducted in the absence of any commercial or financial relationships that could be construed as a potential conflict of interest.
The handling editor JF declared a shared parent affiliation with the author LC at the time of review.
All claims expressed in this article are solely those of the authors and do not necessarily represent those of their affiliated organizations, or those of the publisher, the editors and the reviewers. Any product that may be evaluated in this article, or claim that may be made by its manufacturer, is not guaranteed or endorsed by the publisher.
Adella, A., and de Baaij, J. H. F. (2023). Mtor signaling in renal ion transport. Acta Physiol. (Oxf) 238, e13960. doi:10.1111/apha.13960
Almajidi, Y. Q., Gupta, J., Sheri, F. S., Zabibah, R. S., Faisal, A., Ruzibayev, A., et al. (2023). Advances in chitosan-based hydrogels for pharmaceutical and biomedical applications: a comprehensive review. Int. J. Biol. Macromol. 253, 127278. doi:10.1016/j.ijbiomac.2023.127278
An, J. Y., Um, W., You, D. G., Song, Y., Lee, J., Van Quy, N., et al. (2021). Gold-installed hyaluronic acid hydrogel for ultrasound-triggered thermal elevation and on-demand cargo release. Int. J. Biol. Macromol. 193, 553–561. doi:10.1016/j.ijbiomac.2021.10.071
Androjna, C., Yee, C. S., White, C. R., Waldorff, E. I., Ryaby, J. T., Zborowski, M., et al. (2021). A comparison of alendronate to varying magnitude pemf in mitigating bone loss and altering bone remodeling in skeletally mature osteoporotic rats. Bone 143, 115761. doi:10.1016/j.bone.2020.115761
Anjum, F., Lienemann, P. S., Metzger, S., Biernaskie, J., Kallos, M. S., and Ehrbar, M. (2016). Enzyme responsive gag-based natural-synthetic hybrid hydrogel for tunable growth factor delivery and stem cell differentiation. Biomaterials 87, 104–117. doi:10.1016/j.biomaterials.2016.01.050
Arora, S., Das, G., Alqarni, M., Grover, V., Manzoor Baba, S., Saluja, P., et al. (2023). Role of chitosan hydrogels in clinical dentistry. Gels 9, 698. doi:10.3390/gels9090698
Arrizabalaga, J. H., Smallcomb, M., Abu-Laban, M., Liu, Y., Yeingst, T. J., Dhawan, A., et al. (2022). Ultrasound-responsive hydrogels for on-demand protein release. ACS Appl. Bio Mater 5, 3212–3218. doi:10.1021/acsabm.2c00192
Badila, A. E., Radulescu, D. M., Ilie, A., Niculescu, A. G., Grumezescu, A. M., and Radulescu, A. R. (2022). Bone regeneration and oxidative stress: an updated overview. Antioxidants (Basel) 11, 318. doi:10.3390/antiox11020318
Balint, R., Cassidy, N. J., and Cartmell, S. H. (2014). Conductive polymers: towards a smart biomaterial for tissue engineering. Acta Biomater. 10, 2341–2353. doi:10.1016/j.actbio.2014.02.015
Barbosa, F., Garrudo, F. F. F., Alberte, P. S., Resina, L., Carvalho, M. S., Jain, A., et al. (2023). Hydroxyapatite-filled osteoinductive and piezoelectric nanofibers for bone tissue engineering. Sci. Technol. Adv. Mater 24, 2242242. doi:10.1080/14686996.2023.2242242
Berillo, D., Yeskendir, A., Zharkinbekov, Z., Raziyeva, K., and Saparov, A. (2021). Peptide-based drug delivery systems. Med. Kaunas. 57, 1209. doi:10.3390/medicina57111209
Bose, S., Sarkar, N., and Jo, Y. (2023). Natural medicine delivery from 3d printed bone substitutes. J. Control Release 365, 848–875. doi:10.1016/j.jconrel.2023.09.025
Bose, S., Traxel, K. D., Vu, A. A., and Bandyopadhyay, A. (2019). Clinical significance of three-dimensional printed biomaterials and biomedical devices. MRS Bull. 44, 494–504. doi:10.1557/mrs.2019.121
Boskey, A. L., and Coleman, R. (2010). Aging and bone. J. Dent. Res. 89, 1333–1348. doi:10.1177/0022034510377791
Brudno, Y., and Mooney, D. J. (2015). On-demand drug delivery from local depots. J. Control Release 219, 8–17. doi:10.1016/j.jconrel.2015.09.011
Butoescu, N., Seemayer, C. A., Foti, M., Jordan, O., and Doelker, E. (2009). Dexamethasone-containing plga superparamagnetic microparticles as carriers for the local treatment of arthritis. Biomaterials 30, 1772–1780. doi:10.1016/j.biomaterials.2008.12.017
Chen, H., Qin, Z., Zhao, J., He, Y., Ren, E., Zhu, Y., et al. (2019). Cartilage-targeting and dual mmp-13/ph responsive theranostic nanoprobes for osteoarthritis imaging and precision therapy. Biomaterials 225, 119520. doi:10.1016/j.biomaterials.2019.119520
Chen, H., Tan, W., Tong, T., Shi, X., Ma, S., and Zhu, G. (2023). A ph-responsive asymmetric microfluidic/chitosan device for drug release in infective bone defect treatment. Int. J. Mol. Sci. 24, 4616. doi:10.3390/ijms24054616
Chen, X., Cortez-Jugo, C., Choi, G. H., Björnmalm, M., Dai, Y., Yoo, P. J., et al. (2017). Patterned poly(dopamine) films for enhanced cell adhesion. Bioconjug Chem. 28, 75–80. doi:10.1021/acs.bioconjchem.6b00544
Chorsi, M. T., Curry, E. J., Chorsi, H. T., Das, R., Baroody, J., Purohit, P. K., et al. (2019). Piezoelectric biomaterials for sensors and actuators. Adv. Mater 31, e1802084. doi:10.1002/adma.201802084
Cojocaru, F.-D., Balan, V., and Verestiuc, L. (2022). Advanced 3d magnetic scaffolds for tumor-related bone defects. Int. J. Mol. Sci. 23, 16190. doi:10.3390/ijms232416190
Constantin, M., Bucatariu, S., Ascenzi, P., Butnaru, M., and Fundueanu, G. (2020). Smart drug delivery system activated by specific biomolecules. Mater Sci. Eng. C Mater Biol. Appl. 108, 110466. doi:10.1016/j.msec.2019.110466
Dang, Y., Zhang, Y., Jian, M., Luo, P., Anwar, N., Ma, Y., et al. (2023). Advances of blood coagulation factor xiii in bone healing. Tissue Eng. Part B Rev. 29, 591–604. doi:10.1089/ten.TEB.2023.0016
De Long, W. G., Einhorn, T. A., Koval, K., McKee, M., Smith, W., Sanders, R., et al. (2007). Bone grafts and bone graft substitutes in orthopaedic trauma surgery. A critical analysis. J. Bone Jt. Surg. Am. 89, 649–658. doi:10.2106/jbjs.F.00465
Ding, H., Tan, P., Fu, S., Tian, X., Zhang, H., Ma, X., et al. (2022). Preparation and application of ph-responsive drug delivery systems. J. Control Release 348, 206–238. doi:10.1016/j.jconrel.2022.05.056
Ding, Y., Hao, Y., Yuan, Z., Tao, B., Chen, M., Lin, C., et al. (2020). A dual-functional implant with an enzyme-responsive effect for bacterial infection therapy and tissue regeneration. Biomater. Sci. 8, 1840–1854. doi:10.1039/c9bm01924c
Du, Y., Guo, J. L., Wang, J., Mikos, A. G., and Zhang, S. (2019). Hierarchically designed bone scaffolds: from internal cues to external stimuli. Biomaterials 218, 119334. doi:10.1016/j.biomaterials.2019.119334
Duan, J., Huang, Y., Zong, S., and Jiang, J. (2020). Preparation and drug release properties of a thermo sensitive Ga hydrogel. Polym. (Basel) 13, 119. doi:10.3390/polym13010119
Escudero, J. S. B., Perez, M. G. B., de Oliveira Rosso, M. P., Buchaim, D. V., Pomini, K. T., Campos, L. M. G., et al. (2019). Photobiomodulation therapy (pbmt) in bone repair: a systematic review. Injury 50, 1853–1867. doi:10.1016/j.injury.2019.09.031
Fan, B., Guo, Z., Li, X., Li, S., Gao, P., Xiao, X., et al. (2020). Electroactive barium titanate coated titanium scaffold improves osteogenesis and osseointegration with low-intensity pulsed ultrasound for large segmental bone defects. Bioact. Mater 5, 1087–1101. doi:10.1016/j.bioactmat.2020.07.001
Fan, L., Guan, P., Xiao, C., Wen, H., Wang, Q., Liu, C., et al. (2021). Exosome-functionalized polyetheretherketone-based implant with immunomodulatory property for enhancing osseointegration. Bioact. Mater 6, 2754–2766. doi:10.1016/j.bioactmat.2021.02.005
Fang, J., Wang, X., Jiang, W., Zhu, Y., Hu, Y., Zhao, Y., et al. (2020). Platelet-rich plasma therapy in the treatment of diseases associated with orthopedic injuries. Tissue Eng. Part B Rev. 26, 571–585. doi:10.1089/ten.TEB.2019.0292
Fang, R., Pi, J., Wei, T., Ali, A., and Guo, L. (2021). Stimulus-responsive polymers based on polypeptoid skeletons. Polymers 13, 2089. doi:10.3390/polym13132089
Fischer, N. G., Chen, X., Astleford-Hopper, K., He, J., Mullikin, A. F., Mansky, K. C., et al. (2021). Antimicrobial and enzyme-responsive multi-peptide surfaces for bone-anchored devices. Mater Sci. Eng. C Mater Biol. Appl. 125, 112108. doi:10.1016/j.msec.2021.112108
Fu, J., Liu, X., Tan, L., Cui, Z., Zheng, Y., Liang, Y., et al. (2019). Photoelectric-responsive extracellular matrix for bone engineering. ACS Nano 13, 13581–13594. doi:10.1021/acsnano.9b08115
Gao, L., Chen, Q., Gong, T., Liu, J., and Li, C. (2019). Recent advancement of imidazolate framework (Zif-8) based nanoformulations for synergistic tumor therapy. Nanoscale. 11, 21030–21045. doi:10.1039/c9nr06558j
Gao, X., Xue, Y., Zhu, Z., Chen, J., Liu, Y., Cheng, X., et al. (2021). Nanoscale zeolitic imidazolate framework-8 activator of canonical mapk signaling for bone repair. ACS Appl. Mater Interfaces 13, 97–111. doi:10.1021/acsami.0c15945
George, P. M., Bliss, T. M., Hua, T., Lee, A., Oh, B., Levinson, A., et al. (2017). Electrical preconditioning of stem cells with a conductive polymer scaffold enhances stroke recovery. Biomaterials 142, 31–40. doi:10.1016/j.biomaterials.2017.07.020
Ghosh, S., Qiao, W., Yang, Z., Orrego, S., and Neelakantan, P. (2022). Engineering dental tissues using biomaterials with piezoelectric effect: current progress and future perspectives. J. Funct. Biomater. 14, 8. doi:10.3390/jfb14010008
Gregoritza, M., and Brandl, F. P. (2015). The diels-alder reaction: a powerful tool for the design of drug delivery systems and biomaterials. Eur. J. Pharm. Biopharm. 97, 438–453. doi:10.1016/j.ejpb.2015.06.007
Halperin, C., Mutchnik, S., Agronin, A., Molotskii, M., Urenski, P., Salai, M., et al. (2004). Piezoelectric effect in human bones studied in nanometer scale. Nano Lett. 4 (7), 1253–1256. doi:10.1021/nl049453i
Handa, M., Singh, A., Flora, S. J. S., and Shukla, R. (2022). Stimuli-responsive polymeric nanosystems for therapeutic applications. Curr. Pharm. Des. 28, 910–921. doi:10.2174/1381612827666211208150210
Hayyan, M., Hashim, M. A., and AlNashef, I. M. (2016). Superoxide ion: generation and chemical implications. Chem. Rev. 116, 3029–3085. doi:10.1021/acs.chemrev.5b00407
He, M., Wang, H., Han, Q., Shi, X., He, S., Sun, J., et al. (2023). Glucose-primed peek orthopedic implants for antibacterial therapy and safeguarding diabetic osseointegration. Biomaterials 303, 122355. doi:10.1016/j.biomaterials.2023.122355
Hein, C. D., Liu, X. M., and Wang, D. (2008). Click chemistry, a powerful tool for pharmaceutical sciences. Pharm. Res. 25, 2216–2230. doi:10.1007/s11095-008-9616-1
Hu, Q., Katti, P. S., and Gu, Z. (2014). Enzyme-responsive nanomaterials for controlled drug delivery. Nanoscale 6, 12273–12286. doi:10.1039/c4nr04249b
Huang, H., Zhang, X., Hu, X., Shao, Z., Zhu, J., Dai, L., et al. (2014). A functional biphasic biomaterial homing mesenchymal stem cells for in vivo cartilage regeneration. Biomaterials 35, 9608–9619. doi:10.1016/j.biomaterials.2014.08.020
Ikebuchi, Y., Aoki, S., Honma, M., Hayashi, M., Sugamori, Y., Khan, M., et al. (2018). Coupling of bone resorption and formation by rankl reverse signalling. Nature 561, 195–200. doi:10.1038/s41586-018-0482-7
Islam, M. M., Shahruzzaman, M., Biswas, S., Nurus Sakib, M., and Rashid, T. U. (2020). Chitosan based bioactive materials in tissue engineering applications-a review. Bioact. Mater 5, 164–183. doi:10.1016/j.bioactmat.2020.01.012
Jacob, J., More, N., Kalia, K., and Kapusetti, G. (2018). Piezoelectric smart biomaterials for bone and cartilage tissue engineering. Inflamm. Regen. 38, 2. doi:10.1186/s41232-018-0059-8
Jamnongpak, W., Tiptipakorn, S., Arumugam, H., Charoensuk, K., Karagiannidis, P., and Rimdusit, S. (2024). Development of nir light-responsive shape memory composites based on bio-benzoxazine/bio-urethane copolymers reinforced with graphene. Nanoscale Adv. 6, 499–510. doi:10.1039/d3na00647f
Jo, M. J., Shin, H. J., Yoon, M. S., Kim, S. Y., Jin, C. E., Park, C. W., et al. (2023). Physicochemical, pharmacokinetic, and toxicity evaluation of Soluplus® polymeric micelles encapsulating fenbendazole. Pharmaceutics 12, 1000. doi:10.3390/pharmaceutics12101000
Khan, M. U. A., Al-Arjan, W. S., Ashammakhi, N., Haider, S., Amin, R., and Hasan, A. (2022). Multifunctional bioactive scaffolds from arx-G-(Zn@Rgo)-Hap for bone tissue engineering: in vitro antibacterial, antitumor, and biocompatibility evaluations. ACS Appl. Bio Mater 5, 5445–5456. doi:10.1021/acsabm.2c00777
Khare, D., Basu, B., and Dubey, A. K. (2020). Electrical stimulation and piezoelectric biomaterials for bone tissue engineering applications. Biomaterials 258, 120280. doi:10.1016/j.biomaterials.2020.120280
Khosla, S., Samakkarnthai, P., Monroe, D. G., and Farr, J. N. (2021). Update on the pathogenesis and treatment of skeletal fragility in type 2 diabetes mellitus. Nat. Rev. Endocrinol. 17, 685–697. doi:10.1038/s41574-021-00555-5
Kim, J., Choi, H. S., Kim, Y. M., and Song, S. C. (2023). Thermo-responsive nanocomposite bioink with growth-factor holding and its application to bone regeneration. Small 19, e2203464. doi:10.1002/smll.202203464
Kim, Y.-J., and Matsunaga, Y. T. (2017). Thermo-responsive polymers and their application as smart biomaterials. J. Mater. Chem. B 5, 4307–4321. doi:10.1039/c7tb00157f
Kocak, F. Z., Talari, A. C. S., Yar, M., and Rehman, I. U. (2020). In-situ forming ph and thermosensitive injectable hydrogels to stimulate angiogenesis: potential candidates for fast bone regeneration applications. Int. J. Mol. Sci. 21, 1633. doi:10.3390/ijms21051633
Kumar, A., Sharma, A., Chen, Y., Jones, M. M., Vanyo, S. T., Li, C., et al. (2021). Copper@Zif-8 core-shell nanowires for reusable antimicrobial face masks. Adv. Funct. Mater 31, 2008054. doi:10.1002/adfm.202008054
Lallana, E., Fernandez-Trillo, F., Sousa-Herves, A., Riguera, R., and Fernandez-Megia, E. (2012). Click chemistry with polymers, dendrimers, and hydrogels for drug delivery. Pharm. Res. 29, 902–921. doi:10.1007/s11095-012-0683-y
Lambertini, E., Penolazzi, L., Morganti, C., Lisignoli, G., Zini, N., Angelozzi, M., et al. (2015). Osteogenic differentiation of human mscs: specific occupancy of the mitochondrial DNA by Nfatc1 transcription factor. Int. J. Biochem. Cell. Biol. 64, 212–219. doi:10.1016/j.biocel.2015.04.011
Lavanya, K., Chandran, S. V., Balagangadharan, K., and Selvamurugan, N. (2020). Temperature- and ph-responsive chitosan-based injectable hydrogels for bone tissue engineering. Mater Sci. Eng. C Mater Biol. Appl. 111, 110862. doi:10.1016/j.msec.2020.110862
Lee, J., Byun, H., Madhurakkat Perikamana, S. K., Lee, S., and Shin, H. (2018a). Current advances in immunomodulatory biomaterials for bone regeneration. Adv. Healthc. Mater. 8, e1801106. doi:10.1002/adhm.201801106
Lee, J. Y., Lim, H., Ahn, J. W., Jang, D., Lee, S. H., Park, K., et al. (2018b). Design of a 3d bmp-2-delivering tannylated pcl scaffold and its anti-oxidant, anti-inflammatory, and osteogenic effects in vitro. Int. J. Mol. Sci. 19, 3602. doi:10.3390/ijms19113602
Lee, S. H., Gupta, M. K., Bang, J. B., Bae, H., and Sung, H. J. (2013). Current progress in reactive oxygen species (Ros)-Responsive materials for biomedical applications. Adv. Healthc. Mater 2, 908–915. doi:10.1002/adhm.201200423
Leppik, L., Oliveira, K. M. C., Bhavsar, M. B., and Barker, J. H. (2020). Electrical stimulation in bone tissue engineering treatments. Eur. J. Trauma Emerg. Surg. 46, 231–244. doi:10.1007/s00068-020-01324-1
Li, P., Li, Y., Fu, R., Duan, Z., Zhu, C., and Fan, D. (2023). Nir- and ph-responsive injectable nanocomposite alginate-graft-dopamine hydrogel for melanoma suppression and wound repair. Carbohydr. Polym. 314, 120899. doi:10.1016/j.carbpol.2023.120899
Li, S., Wei, C., and Lv, Y. (2020). Preparation and application of magnetic responsive materials in bone tissue engineering. Curr. Stem Cell. Res. Ther. 15, 428–440. doi:10.2174/1574888X15666200101122505
Li, X., Shen, M., Yang, J., Liu, L., and Yang, Y. W. (2024). Pillararene-based stimuli-responsive supramolecular delivery systems for cancer therapy. Adv. Mater 36, e2313317. doi:10.1002/adma.202313317
Li, Z., Qu, Y., Zhang, X., and Yang, B. (2009). Bioactive nano-titania ceramics with biomechanical compatibility prepared by doping with piezoelectric batio(3). Acta Biomater. 5, 2189–2195. doi:10.1016/j.actbio.2009.02.013
Liang, Y., Li, Z., Huang, Y., Yu, R., and Guo, B. (2021). Dual-dynamic-bond cross-linked antibacterial adhesive hydrogel sealants with on-demand removability for post-wound-closure and infected wound healing. ACS Nano 15, 7078–7093. doi:10.1021/acsnano.1c00204
Lin, C., Hao, H., Mei, L., and Wu, M. (2020). Metal-free two-dimensional nanomaterial-mediated photothermal tumor therapy. Smart Mater. Med. 1, 150–167. doi:10.1016/j.smaim.2020.09.001
Lin, K., Xia, L., Li, H., Jiang, X., Pan, H., Xu, Y., et al. (2013). Enhanced osteoporotic bone regeneration by strontium-substituted calcium silicate bioactive ceramics. Biomaterials 34, 10028–10042. doi:10.1016/j.biomaterials.2013.09.056
Liu, H., Shi, Y., Zhu, Y., Wu, P., Deng, Z., Dong, Q., et al. (2023). Bioinspired piezoelectric periosteum to augment bone regeneration via synergistic immunomodulation and osteogenesis. ACS Appl. Mater Interfaces 15, 12273–12293. doi:10.1021/acsami.2c19767
Liu, M., Du, H., Zhang, W., and Zhai, G. (2017). Internal stimuli-responsive nanocarriers for drug delivery: design strategies and applications. Mater Sci. Eng. C Mater Biol. Appl. 71, 1267–1280. doi:10.1016/j.msec.2016.11.030
Losic, D. (2021). Advancing of titanium medical implants by surface engineering: recent progress and challenges. Expert Opin. Drug Deliv. 18, 1355–1378. doi:10.1080/17425247.2021.1928071
Lu, J. W., Yang, F., Ke, Q. F., Xie, X. T., and Guo, Y. P. (2018). Magnetic nanoparticles modified-porous scaffolds for bone regeneration and photothermal therapy against tumors. Nanomedicine. 14, 811–822. doi:10.1016/j.nano.2017.12.025
Lui, Y. S., Sow, W. T., Tan, L. P., Wu, Y., Lai, Y., and Li, H. (2019). 4d printing and stimuli-responsive materials in biomedical aspects. Acta Biomater. 92, 19–36. doi:10.1016/j.actbio.2019.05.005
Lv, Z., Hu, T., Bian, Y., Wang, G., Wu, Z., Li, H., et al. (2023). A mgfe-ldh nanosheet-incorporated smart thermo-responsive hydrogel with controllable growth factor releasing capability for bone regeneration. Adv. Mater 35, e2206545. doi:10.1002/adma.202206545
Ma, B., Liu, F., Li, Z., Duan, J., Kong, Y., Hao, M., et al. (2019). Piezoelectric nylon-11 nanoparticles with ultrasound assistance for high-efficiency promotion of stem cell osteogenic differentiation. J. Mater Chem. B 7, 1847–1854. doi:10.1039/c8tb03321h
Mao, L., Bai, L., Wang, X., Chen, X., Zhang, D., Chen, F., et al. (2022). Enhanced cell osteogenesis and osteoimmunology regulated by piezoelectric biomaterials with controllable surface potential and charges. ACS Appl. Mater Interfaces 14, 44111–44124. doi:10.1021/acsami.2c11131
McKay, C. S., and Finn, M. G. (2014). Click chemistry in complex mixtures: bioorthogonal bioconjugation. Chem. Biol. 21, 1075–1101. doi:10.1016/j.chembiol.2014.09.002
Mészáros, B., Csoti, A., Szanto, T. G., Telek, A., Kovács, K., Toth, A., et al. (2022). The heag1 K(+) channel inhibitor astemizole stimulates Ca(2+) deposition in saos-2 and Mg-63 osteosarcoma cultures. Int. J. Mol. Sci. 23, 10533. doi:10.3390/ijms231810533
Minary-Jolandan, M., and Yu, M.-F. (2010). Shear piezoelectricity in bone at the nanoscale. Appl. Phys. Lett. 97, 97. doi:10.1063/1.3503965
Mohanty, A. R., Ravikumar, A., and Peppas, N. A. (2022). Recent advances in glucose-responsive insulin delivery systems: novel hydrogels and future applications. Regen. Biomater. 9, rbac056. doi:10.1093/rb/rbac056
Montoya, C., Du, Y., Gianforcaro, A. L., Orrego, S., Yang, M., and Lelkes, P. I. (2021). On the road to smart biomaterials for bone research: definitions, concepts, advances, and outlook. Bone Res. 9, 12. doi:10.1038/s41413-020-00131-z
Moonga, S. S., and Qin, Y. X. (2018). Mc3t3 infiltration and proliferation in bovine trabecular scaffold regulated by dynamic flow bioreactor and augmented by low-intensity pulsed ultrasound. J. Orthop. Transl. 14, 16–22. doi:10.1016/j.jot.2018.02.002
More, N., and Kapusetti, G. (2017). Piezoelectric material - a promising approach for bone and cartilage regeneration. Med. Hypotheses 108, 10–16. doi:10.1016/j.mehy.2017.07.021
Mu, R., Zhu, D., Abdulmalik, S., Wijekoon, S., Wei, G., and Kumbar, S. G. (2024). Stimuli-responsive peptide assemblies: design, self-assembly, modulation, and biomedical applications. Bioact. Mater 35, 181–207. doi:10.1016/j.bioactmat.2024.01.023
Murillo, G., Blanquer, A., Vargas-Estevez, C., Barrios, L., Ibanez, E., Nogues, C., et al. (2017). Electromechanical nanogenerator-cell interaction modulates cell activity. Adv. Mater 29, 29. doi:10.1002/adma.201605048
Nguyen, H. T., Ono, M., Oida, Y., Hara, E. S., Komori, T., Akiyama, K., et al. (2018). Bone marrow cells inhibit bmp-2-induced osteoblast activity in the marrow environment. J. Bone Mineral Res. 34, 327–332. doi:10.1002/jbmr.3598
Ni, X., Xing, X., Deng, Y., and Li, Z. (2023). Applications of stimuli-responsive hydrogels in bone and cartilage regeneration. Pharmaceutics 15, 982. doi:10.3390/pharmaceutics15030982
Nomikou, N., Feichtinger, G. A., Saha, S., Nuernberger, S., Heimel, P., Redl, H., et al. (2018). Ultrasound-responsive gene-activated matrices for osteogenic gene therapy using matrix-assisted sonoporation. J. Tissue Eng. Regen. Med. 12, e250–e260. doi:10.1002/term.2406
Pajarinen, J., Lin, T., Gibon, E., Kohno, Y., Maruyama, M., Nathan, K., et al. (2019). Mesenchymal stem cell-macrophage crosstalk and bone healing. Biomaterials 196, 80–89. doi:10.1016/j.biomaterials.2017.12.025
Qiao, Y., Yu, L., Yang, P., Chen, M., Sun, H., Wang, L., et al. (2023). Spatiotemporal immunomodulation and biphasic osteo-vascular aligned electrospun membrane for diabetic periosteum regeneration. Adv. Sci. (Weinh) 10, e2302874. doi:10.1002/advs.202302874
Ren, X., Liu, H., Wu, X., Weng, W., Wang, X., and Su, J. (2022). Reactive oxygen species (Ros)-Responsive biomaterials for the treatment of bone-related diseases. Front. Bioeng. Biotechnol. 9, 820468. doi:10.3389/fbioe.2021.820468
Ressler, A., Ródenas-Rochina, J., Ivanković, M., Ivanković, H., Rogina, A., and Gallego Ferrer, G. (2018). Injectable chitosan-hydroxyapatite hydrogels promote the osteogenic differentiation of mesenchymal stem cells. Carbohydr. Polym. 197, 469–477. doi:10.1016/j.carbpol.2018.06.029
Sadowska, J. M., and Ginebra, M. P. (2020). Inflammation and biomaterials: role of the immune response in bone regeneration by inorganic scaffolds. J. Mater Chem. B 8, 9404–9427. doi:10.1039/d0tb01379j
Saeedi, P., Petersohn, I., Salpea, P., Malanda, B., Karuranga, S., Unwin, N., et al. (2019). Global and regional diabetes prevalence estimates for 2019 and projections for 2030 and 2045: results from the international diabetes federation diabetes atlas, 9(Th) edition. Diabetes Res. Clin. Pract. 157, 107843. doi:10.1016/j.diabres.2019.107843
Salehpour, F., Cassano, P., Rouhi, N., Hamblin, M. R., De Taboada, L., Farajdokht, F., et al. (2019). Penetration profiles of visible and near-infrared lasers and light-emitting diode light through the head tissues in animal and human species: a review of literature. Photobiomodul Photomed. Laser Surg. 37, 581–595. doi:10.1089/photob.2019.4676
Scott, J. F. (1974). Soft-mode spectroscopy: experimental studies of structural phase transitions. Rev. Mod. Phys. 46, 83–128. doi:10.1103/RevModPhys.46.83
Shang, F., Yu, Y., Liu, S., Ming, L., Zhang, Y., Zhou, Z., et al. (2021). Advancing application of mesenchymal stem cell-based bone tissue regeneration. Bioact. Mater 6, 666–683. doi:10.1016/j.bioactmat.2020.08.014
Simon-Yarza, T., Mielcarek, A., Couvreur, P., and Serre, C. (2018). Nanoparticles of metal-organic frameworks: on the road to in vivo efficacy in biomedicine. Adv. Mat. 30, e1707365. doi:10.1002/adma.201707365
Su, Y., Yang, H., Gao, J., Qin, Y. X., Zheng, Y., and Zhu, D. (2019). Interfacial zinc phosphate is the key to controlling biocompatibility of metallic zinc implants. Adv. Sci. (Weinh) 6, 1900112. doi:10.1002/advs.201900112
Sultana, A., Ghosh, S. K., Sencadas, V., Zheng, T., Higgins, M. J., Middya, T. R., et al. (2017). Human skin interactive self-powered wearable piezoelectric bio-E-skin by electrospun poly-L-lactic acid nanofibers for non-invasive physiological signal monitoring. J. Mater Chem. B 5, 7352–7359. doi:10.1039/c7tb01439b
Sun, C., Wang, Z., Yue, L., Huang, Q., Lu, S., and Wang, R. (2020b). Ros-initiated chemiluminescence-driven payload release from macrocycle-based azo-containing polymer nanocapsules. J. Mater Chem. B 8, 8878–8883. doi:10.1039/d0tb01475c
Sun, D., Zhang, Z., Chen, M., Zhang, Y., Amagat, J., Kang, S., et al. (2020a). Co-immobilization of Ce6 sono/photosensitizer and protonated graphitic carbon nitride on pcl/gelation fibrous scaffolds for combined sono-photodynamic cancer therapy. ACS Appl. Mater Interfaces 12, 40728–40739. doi:10.1021/acsami.0c08446
Suslick, K. S. (2013). Applications of ultrasound to materials chemistry. MRS Bull. 20, 29–34. doi:10.1557/s088376940004464x
Swietach, P., Vaughan-Jones, R. D., Harris, A. L., and Hulikova, A. (2014). The chemistry, physiology and pathology of ph in cancer. Philos. Trans. R. Soc. Lond B Biol. Sci. 369, 20130099. doi:10.1098/rstb.2013.0099
Tandon, B., Blaker, J. J., and Cartmell, S. H. (2018). Piezoelectric materials as stimulatory biomedical materials and scaffolds for bone repair. Acta Biomater. 73, 1–20. doi:10.1016/j.actbio.2018.04.026
Tang, B., Shen, X., Yang, Y., Xu, Z., Yi, J., Yao, Y., et al. (2021). Enhanced cellular osteogenic differentiation on cofe(2)O(4)/P(Vdf-Trfe) nanocomposite coatings under static magnetic field. Colloids Surf. B Biointerfaces 198, 111473. doi:10.1016/j.colsurfb.2020.111473
Tang, G., Zhu, L., Wang, W., Zuo, D., Shi, C., Yu, X., et al. (2022). Alendronate-functionalized double network hydrogel scaffolds for effective osteogenesis. Front. Chem. 10, 977419. doi:10.3389/fchem.2022.977419
Tian, B., Li, X., Zhang, J., Zhang, M., Gan, D., Deng, D., et al. (2022). A 3d-printed molybdenum-containing scaffold exerts dual pro-osteogenic and anti-osteoclastogenic effects to facilitate alveolar bone repair. Int. J. Oral Sci. 14, 45. doi:10.1038/s41368-022-00195-z
Turner, C. H., Wang, T., and Burr, D. B. (2001). Shear strength and fatigue properties of human cortical bone determined from pure shear tests. Calcif. Tissue Int. 69, 373–378. doi:10.1007/s00223-001-1006-1
Ud-Din, S., and Bayat, A. (2014). Electrical stimulation and cutaneous wound healing: a review of clinical evidence. Healthc. (Basel) 2 (2), 445–467. doi:10.3390/healthcare2040445
Veronick, J. A., Assanah, F., Piscopo, N., Kutes, Y., Vyas, V., Nair, L. S., et al. (2018). Mechanically loading cell/hydrogel constructs with low-intensity pulsed ultrasound for bone repair. Tissue Eng. Part A 24, 254–263. doi:10.1089/ten.TEA.2016.0547
Wan, Z., Zhang, P., Lv, L., and Zhou, Y. (2020). Nir light-assisted phototherapies for bone-related diseases and bone tissue regeneration: a systematic review. Theranostics 10, 11837–11861. doi:10.7150/thno.49784
Wang, A., Liu, Z., Hu, M., Wang, C., Zhang, X., Shi, B., et al. (2018). Piezoelectric nanofibrous scaffolds as in vivo energy harvesters for modifying fibroblast alignment and proliferation in wound healing. Nano Energy 43, 63–71. doi:10.1016/j.nanoen.2017.11.023
Wang, B., Zeng, Y., Liu, S., Zhou, M., Fang, H., Wang, Z., et al. (2023). Zif-8 induced hydroxyapatite-like crystals enabled superior osteogenic ability of mew printing pcl scaffolds. J. Nanobiotechnology 21, 264. doi:10.1186/s12951-023-02007-w
Wang, C., Huang, W., Zhou, Y., He, L., He, Z., Chen, Z., et al. (2020). 3d printing of bone tissue engineering scaffolds. Bioact. Mater 5, 82–91. doi:10.1016/j.bioactmat.2020.01.004
Wang, H., Leeuwenburgh, S. C., Li, Y., and Jansen, J. A. (2012). The use of micro- and nanospheres as functional components for bone tissue regeneration. Tissue Eng. Part B Rev. 18, 24–39. doi:10.1089/ten.TEB.2011.0184
Wang, L., Mi, B., Zhang, Y., Yan, H., and Zhu, H. (2021). Alendronate promotes the gene expression of extracellular matrix mediated by sp-1/sox-9. Hum. Exp. Toxicol. 40, 1173–1182. doi:10.1177/0960327120988875
Wang, W., and Yeung, K. W. K. (2017). Bone grafts and biomaterials substitutes for bone defect repair: a review. Bioact. Mater 2, 224–247. doi:10.1016/j.bioactmat.2017.05.007
Wei, H., Cui, J., Lin, K., Xie, J., and Wang, X. (2022a). Recent advances in smart stimuli-responsive biomaterials for bone therapeutics and regeneration. Bone Res. 10, 17. doi:10.1038/s41413-021-00180-y
Wei, H., Cui, J., Lin, K., Xie, J., and Wang, X. (2022b). Recent advances in smart stimuli-responsive biomaterials for bone therapeutics and regeneration. Bone Res. 10, 17. doi:10.1038/s41413-021-00180-y
Wu, S. W., Liu, X., Miller, A. L., Cheng, Y. S., Yeh, M. L., and Lu, L. (2018). Strengthening injectable thermo-sensitive nipaam-G-chitosan hydrogels using chemical cross-linking of disulfide bonds as scaffolds for tissue engineering. Carbohydr. Polym. 192, 308–316. doi:10.1016/j.carbpol.2018.03.047
Wu, Y., Jiang, W., Wen, X., He, B., Zeng, X., Wang, G., et al. (2010). A novel calcium phosphate ceramic-magnetic nanoparticle composite as a potential bone substitute. Biomed. Mater 5, 15001. doi:10.1088/1748-6041/5/1/015001
Xing, Y., Qiu, L., Liu, D., Dai, S., and Sheu, C. L. (2023). The role of smart polymeric biomaterials in bone regeneration: a review. Front. Bioeng. Biotechnol. 11, 1240861. doi:10.3389/fbioe.2023.1240861
Xu, B., Li, Y., Gao, F., Zhai, X., Sun, M., Lu, W., et al. (2015). High strength multifunctional multiwalled hydrogel tubes: ion-triggered shape memory, antibacterial, and anti-inflammatory efficacies. ACS Appl. Mater Interfaces 7, 16865–16872. doi:10.1021/acsami.5b05074
Xu, H.-Y., and Gu, N. (2014). Magnetic responsive scaffolds and magnetic fields in bone repair and regeneration. Front. Mater. Sci. 8, 20–31. doi:10.1007/s11706-014-0232-1
Xu, L., Bai, E., Zhu, Y., Qin, J., Du, X., and Huang, H. (2023). Ph-responsive hydrogel as a potential oral delivery system of baicalin for prolonging gastroprotective activity. Pharmaceutics 15, 257. doi:10.3390/pharmaceutics15010257
Xu, Q., He, C., Xiao, C., and Chen, X. (2016). Reactive oxygen species (ros) responsive polymers for biomedical applications. Macromol. Biosci. 16, 635–646. doi:10.1002/mabi.201500440
Yadav, D., Sharma, P. K., Malviya, R., Mishra, P. S., Surendra, A. V., Rao, G. K., et al. (2023). Stimuli-responsive biomaterials for tissue engineering applications. Curr. Pharm. Biotechnol. 25, 981–999. doi:10.2174/1389201024666230818121821
Yamaguchi, A., Katagiri, T., Ikeda, T., Wozney, J. M., Rosen, V., Wang, E. A., et al. (1991). Recombinant human bone morphogenetic protein-2 stimulates osteoblastic maturation and inhibits myogenic differentiation in vitro. J. Cell. Biol. 113 (3), 681–687. doi:10.1083/jcb.113.3.681
Yan, Q. C., Tomita, N., and Ikada, Y. (1998). Effects of static magnetic field on bone formation of rat femurs. Med. Eng. Phys. 20, 397–402. doi:10.1016/s1350-4533(98)00051-4
Yan, Z., Li, K., Shao, D., Shen, Q., Ding, Y., Huang, S., et al. (2022). Visible-light-responsive reduced graphene oxide/G-C(3)N(4)/tio(2) composite nanocoating for photoelectric stimulation of neuronal and osteoblastic differentiation. RSC Adv. 12, 8878–8888. doi:10.1039/d2ra00282e
Yang, B., Yin, J., Chen, Y., Pan, S., Yao, H., Gao, Y., et al. (2018). 2d-Black-Phosphorus-Reinforced 3d-printed scaffolds:A stepwise countermeasure for osteosarcoma. Adv. Mater 30, 30. doi:10.1002/adma.201705611
Yang, G., Miton, C. M., and Tokuriki, N. (2020). A mechanistic view of enzyme evolution. Protein Sci. 29, 1724–1747. doi:10.1002/pro.3901
Yao, Y., Zhang, H., Wang, Z., Ding, J., Wang, S., Huang, B., et al. (2019). Reactive oxygen species (Ros)-Responsive biomaterials mediate tissue microenvironments and tissue regeneration. J. Mater. Chem. B 7, 5019–5037. doi:10.1039/c9tb00847k
Ye, J., Zhuang, X., Li, X., Gong, X., Sun, Y., Wang, W., et al. (2022). Novel metabolic classification for extrahepatic complication of metabolic associated fatty liver disease: a data-driven cluster analysis with international validation. Metabolism 136, 155294. doi:10.1016/j.metabol.2022.155294
Yu, Y., Yu, X., Tian, D., Yu, A., and Wan, Y. (2021). Thermo-responsive chitosan/silk fibroin/amino-functionalized mesoporous silica hydrogels with strong and elastic characteristics for bone tissue engineering. Int. J. Biol. Macromol. 182, 1746–1758. doi:10.1016/j.ijbiomac.2021.05.166
Zhang, F., Lv, M., Wang, S., Li, M., Wang, Y., Hu, C., et al. (2024). Ultrasound-triggered biomimetic ultrashort peptide nanofiber hydrogels promote bone regeneration by modulating macrophage and the osteogenic immune microenvironment. Bioact. Mater 31, 231–246. doi:10.1016/j.bioactmat.2023.08.008
Zhang, J., Liu, L., Wang, L., Zhu, W., Wang, H., and Ph, (2020). pH responsive zwitterionic-to-cationic transition for safe self-defensive antibacterial application. J. Mater Chem. B 8, 8908–8913. doi:10.1039/d0tb01717e
Zhang, J., Neoh, K. G., Hu, X., Kang, E. T., and Wang, W. (2013). Combined effects of direct current stimulation and immobilized bmp-2 for enhancement of osteogenesis. Biotechnol. Bioeng. 110, 1466–1475. doi:10.1002/bit.24796
Zhang, W., Wang, N., Yang, M., Sun, T., Zhang, J., Zhao, Y., et al. (2022a). Periosteum and development of the tissue-engineered periosteum for guided bone regeneration. J. Orthop. Transl. 33, 41–54. doi:10.1016/j.jot.2022.01.002
Zhang, X., Li, Y., Chen, Y. E., Chen, J., and Ma, P. X. (2016). Cell-free 3d scaffold with two-stage delivery of mirna-26a to regenerate critical-sized bone defects. Nat. Commun. 7, 10376. doi:10.1038/ncomms10376
Zhang, Y., Li, C., Zhang, W., Deng, J., Nie, Y., Du, X., et al. (2022b). 3d-Printed nir-responsive shape memory polyurethane/magnesium scaffolds with tight-contact for robust bone regeneration. Bioact. Mater 16, 218–231. doi:10.1016/j.bioactmat.2021.12.032
Zhang, Z. M., Yu, P., Zhou, K., Yu, F. Y., Bao, R. Y., Yang, M. B., et al. (2023). Hierarchically porous implants orchestrating a physiological viscoelastic and piezoelectric microenvironment for bone regeneration. Adv. Healthc. Mater 12, e2300713. doi:10.1002/adhm.202300713
Zhao, C., Qazvini, N. T., Sadati, M., Zeng, Z., Huang, S., De La Lastra, A. L., et al. (2019b). A ph-triggered, self-assembled, and bioprintable hybrid hydrogel scaffold for mesenchymal stem cell based bone tissue engineering. ACS Appl. Mater Interfaces 11, 8749–8762. doi:10.1021/acsami.8b19094
Zhao, P.-P., Ge, Y.-W., Liu, X.-L., Ke, Q.-F., Zhang, J.-W., Zhu, Z.-A., et al. (2020). Ordered arrangement of hydrated Gdpo4 nanorods in magnetic chitosan matrix promotes tumor photothermal therapy and bone regeneration against breast cancer bone metastases. Chem. Eng. J. 381, 122694. doi:10.1016/j.cej.2019.122694
Zhao, W., Zhao, Y., Wang, Q., Liu, T., Sun, J., and Zhang, R. (2019a). Remote light-responsive nanocarriers for controlled drug delivery: advances and perspectives. Small 15, e1903060. doi:10.1002/smll.201903060
Zheng, Z., Yang, X., Zhang, Y., Zu, W., Wen, M., Liu, T., et al. (2023). An injectable and ph-responsive hyaluronic acid hydrogel as metformin carrier for prevention of breast cancer recurrence. Carbohydr. Polym. 304, 120493. doi:10.1016/j.carbpol.2022.120493
Zhou, S., Bismarck, A., and Steinke, J. H. G. (2013). Ion-responsive alginate based macroporous injectable hydrogel scaffolds prepared by emulsion templating. J. Mater Chem. B 1, 4736–4745. doi:10.1039/c3tb20888e
Zhou, S., Xiao, C., Fan, L., Yang, J., Ge, R., Cai, M., et al. (2024). Injectable ultrasound-powered bone-adhesive nanocomposite hydrogel for electrically accelerated irregular bone defect healing. J. Nanobiotechnology 22, 54. doi:10.1186/s12951-024-02320-y
Zhu, C., Tang, N., Gan, J., Zhang, X., Li, Y., Jia, X., et al. (2021). A ph-sensitive semi-interpenetrating polymer network hydrogels constructed by konjac glucomannan and poly (Gamma-Glutamic acid): synthesis, characterization and swelling behavior. Int. J. Biol. Macromol. 185, 229–239. doi:10.1016/j.ijbiomac.2021.06.046
Zhu, F., Liu, W., Li, P., Zhao, H., Deng, X., and Wang, H. L. (2023). Electric/magnetic intervention for bone regeneration: a systematic review and network meta-analysis. Tissue Eng. Part B Rev. 29, 217–231. doi:10.1089/ten.TEB.2022.0127
Zhu, J., Han, H., Ye, T. T., Li, F. X., Wang, X. L., Yu, J. Y., et al. (2018). Biodegradable and ph sensitive peptide based hydrogel as controlled release system for antibacterial wound dressing application. Molecules 23, 3383. doi:10.3390/molecules23123383
Keywords: bone regeneration, stimuli-responsive biomaterials, scaffolds, implants, composites, hydrogels
Citation: Yang K, Wu Z, Zhang K, Weir MD, Xu HHK, Cheng L, Huang X and Zhou W (2024) Unlocking the potential of stimuli-responsive biomaterials for bone regeneration. Front. Pharmacol. 15:1437457. doi: 10.3389/fphar.2024.1437457
Received: 23 May 2024; Accepted: 18 July 2024;
Published: 31 July 2024.
Edited by:
Jie Fang, Sichuan University, ChinaReviewed by:
Wei Huang, Huazhong University of Science and Technology, ChinaCopyright © 2024 Yang, Wu, Zhang, Weir, Xu, Cheng, Huang and Zhou. This is an open-access article distributed under the terms of the Creative Commons Attribution License (CC BY). The use, distribution or reproduction in other forums is permitted, provided the original author(s) and the copyright owner(s) are credited and that the original publication in this journal is cited, in accordance with accepted academic practice. No use, distribution or reproduction is permitted which does not comply with these terms.
*Correspondence: Xiaojing Huang, aHhpYW9qQDE2My5jb20=; Wen Zhou, emhvdXdlbmRlbnRpc3RAMTM5LmNvbQ==
Disclaimer: All claims expressed in this article are solely those of the authors and do not necessarily represent those of their affiliated organizations, or those of the publisher, the editors and the reviewers. Any product that may be evaluated in this article or claim that may be made by its manufacturer is not guaranteed or endorsed by the publisher.
Research integrity at Frontiers
Learn more about the work of our research integrity team to safeguard the quality of each article we publish.