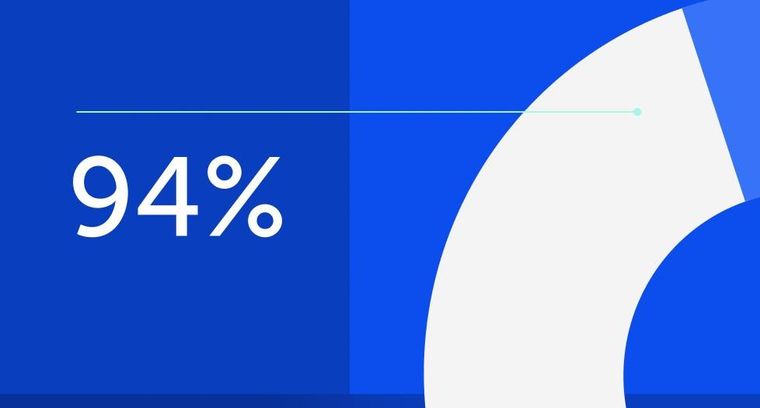
94% of researchers rate our articles as excellent or good
Learn more about the work of our research integrity team to safeguard the quality of each article we publish.
Find out more
ORIGINAL RESEARCH article
Front. Pharmacol., 13 August 2024
Sec. Ethnopharmacology
Volume 15 - 2024 | https://doi.org/10.3389/fphar.2024.1428341
This article is part of the Research TopicPlant and Fungal Metabolites: Innovations in Special Dietary Foods and Disease PreventionView all 5 articles
Introduction: Colocasia affinis Schott (Family: Araceae), found in the Asian region, is a traditional root vegetable consumed by the locals and well-known as Dwarf Elephant Ear.
Methods: For the pharmacological exploration of this root vegetable, four kupchan fractions (i.e. HSF, DCMSF, EASF, and AQSF) from ethanolic extract of C. affinis were employed to in vitro i.e. antioxidant, cytotoxicity, and antimicrobial and in vivo i.e. antidiarrheal and analgesic assays, followed by phytochemical screening and GC-MS protocol.
Result and Discussion: In the antioxidant assay, the AQSF showed promising potential with an IC50 value of 29.4 μg/mL and additionally, it exhibited the greatest overall phenolic content, measuring 57.23 mg GAE/gm. of extract among other fractions. The AQSF also revealed promising cytotoxic activity in brine shrimp lethality assay with an LC50 value of 1.36 μg/mL. Both AQSF and EASF exhibited substantial antimicrobial efficacy against both gram-positive and gram-negative bacteria as well as various fungus species with a remarkable zone of inhibitions compared to standards. Whereas, during both the castor oil-induced antidiarrheal and acetic acid-induced writhing assay, the DCMSF at 400 mg/kg dose exhibited the highest 51.16% reduction of diarrhea and 52.33% reduction of writhing. Phytochemical screening revealed several chemical groups while GC-MS study of different fractions of dwarf elephant ear ethanolic extract revealed 48 different bioactive phytochemicals in total. Several targets such as KAS, DHFR for anti-microbial activities, GLR, URO for antioxidant activities, EGFR, BCL-2 for cytotoxicity, KOR, DOR for antidiarrheal activities and COX-2, TNF-α for analgesic activities are considered for molecular docking against identified phytocompounds and standards along with ADME/T studies to ascertain their safety, efficacy and drug likeliness profiles.
Conclusion: To recapitulate, our study revealed that vegetables such as dwarf elephant ear can be considered as a prospective source of therapeutics and drug development besides their nutritive food values.
Vegetables are essential to the human diet, providing vital vitamins, minerals, nutrients, and bioactive secondary metabolites that support health and enhance food’s color and flavor (Naczk and Shahidi, 2006). Vegetables have been utilized for medical purposes and illness prevention since ancient times. Foods high in antioxidants are in greater demand due to growing health consciousness. Including fresh veggies in the diet is a cheap method to receive a variety of nutrients (Webb and Villamor, 2007). Many vegetables contain immune-boosting macromolecules, carotenoids, flavonoids, and vitamin C. They are also rich in antioxidants like tannins, phenolic acids, flavonoids, and various metabolites (Bhat and Al-Daihan, 2014; Thakur, 2018). Studies have revealed that various antioxidant compounds in vegetables demonstrate diverse activities, including but not limited to anti-inflammatory, antitumor, anticarcinogenic, antimutagenic, anti-atherosclerotic, antibacterial, and antiviral properties (Alonso et al., 2004; Hamzah et al., 2014). Moreover, almost 80% of all commercially available drug substances either directly come from natural metabolites or represent a modified version of the natural analogs (Alam et al., 2021a). Synthetic drugs face safety, efficacy, multi-drug resistance, availability, and cost-effectiveness challenges. Therefore, extracts from vegetable leaves, roots, or whole plants could become valuable sources for natural drug discovery (Maridass and De Britto, 2008; Sudhakar et al., 2020).
Oxidative stress, an imbalance between pro-oxidants and antioxidants, is a major concern linked to chronic illnesses such as diabetes, obesity, metabolic syndrome, cancer, neurological and cardiovascular disorders, as well as inflammatory diseases, with elevated reactive oxygen species (ROS) promoting tumor formation and cancer cell proliferation (Hayes et al., 2020; Morvaridzadeh et al., 2020). With more than 14 million new cases diagnosed every year, cancer ranks high among the world’s leading causes of mortality. By 2030, experts predict that this figure will have almost doubled (Klaunig, 2019). Pain from inflammation arises when the body’s response to injury, infection, or irritation releases signaling molecules like prostaglandins and cytokines, leading to discomfort and adverse effects such as degeneration, necrosis, and exudations (Omoigui, 2007). While NSAIDs, opioids, and non-opioids are effective for managing inflammation and pain (Alam et al., 2020), prolonged use can lead to serious side effects, such as respiratory depression, cardiovascular issues, GI ulceration, liver toxicity, and renal impairment (Carter et al., 2014). Consequently, it is needed to explore and analyze emerging secondary metabolites for better alternatives (Liu, 2007). Diarrhea, marked by frequent, loose bowel movements, is the fifth leading cause of death in developing countries and the eighth worldwide. Despite progress in reducing early childhood deaths, diarrheal morbidity and mortality remain high in older children and adults, with 5.7 billion cases and 1.1 million deaths in 2019. Although most cases are benign, about 285 million annually (5%) result in moderate to severe disease requiring medical treatment (Abbafati et al., 2020; Levine et al., 2023). The underlying causes of diarrhea include unhygienic lifestyles and exposure to pathogenic microbes like bacteria, fungi, viruses, and parasites. Candida albicans, Escherichia coli, Salmonella typhi, Shigella flexneri, and Staphylococcus aureus are common among the many entero-pathogens that can cause diarrhea (Alam et al., 2021a). Antibiotics and other antimicrobial therapy are no less prevalent in diarrheal management than in other infectious diseases, giving rise to global health emergencies like ‘antibiotic/antimicrobial resistance,’ ‘antibiotic-associated diarrhea,’ ‘multi-drug resistance,’ etc. (Mekonnen et al., 2020; Darby et al., 2023). Therefore, clinical microbiologists are focusing on plant-derived antimicrobials to natural plant extract antimicrobials over conventional antibiotics (Sher, 2009; Tiwari et al., 2023).
Colocasia affinis Schott, belonging to the family Araceae, is one of the most prominent species with medicinal food values among the 9 species of the genus Colocasia cultivated throughout the northern tropical area of Bangladesh (Alam et al., 2021a). Indigenously, this 46–91 cm tall perennial herb is known as ‘Kochu’ (Bengali) or ‘Dwarf Elephant’s Ear’ (English) and can be found all over Bangladesh, especially, in Chittagong hill tracts and rural areas of the country. It is also available in Thailand, India, Myanmar, and other Asian countries (Mondal et al., 2019). The leaves, leaf stalk, and tuber of this perennial food crop are used as vegetables in curries and stews (Mondal et al., 2019; Dev et al., 2021). The green leafy vegetables of Colocasia botanical drugs were also found to be used as savory and a rich source of calcium, iron, vitamin C, and other secondary metabolites, having remarkable potential in diabetes management, hypertension control, immune support, neuroprotection, and anticarcinogenic activities (Gupta et al., 2019). In different traditional medicine systems, fever, wound healing, infection, phlegm, constipation, atrophy, emaciation, drowsiness, tuberculosis, arresting arterial hemorrhage, fading melasma and ameliorating stomach issues were among the conditions for which Colocasia botanical drugs have been utilized (Pornprasertpol et al., 2015; Sudhakar et al., 2020). Specifically, C. affinis was used by the locals to treat cataracts (Mondal et al., 2019). A research study reported that this edible botanical drug contains different alkaloids, flavonoids, glycosides, saponins, tannins, steroids, triterpenoids, fatty acids derivatives, carbohydrates, proteins, and amino acids (Lalremruati et al., 2018)
Prior studies on C. affinis have predominantly concentrated on its nutritional worth and customary application. Nevertheless, evidence is scarce regarding the extensive pharmacological capabilities and chemical characterization of the substance, particularly when employing modern techniques such as GC-MS. Research has indicated that Colocasia species possess bioactive substances that offer diverse health advantages, while there is a lack of comprehensive pharmacological assessments. This study will combine in vitro and in vivo pharmacological assays with rigorous GC-MS analysis and molecular docking to thoroughly examine the bioactive metabolites of C. affinis Schott. This research will be groundbreaking in finding precise molecular targets and pharmacological potentials of this traditional vegetable, which has not been thoroughly investigated previously. This comprehensive approach will emphasize the medicinal potential of the subject, going beyond its nutritional benefits.
Whole plants of C. affinis were picked from the hill areas of Bandarban, Chittagong, Bangladesh, in September 2022 (Figure 1). In Mirpur, Dhaka, the specialists at the Bangladesh National Herbarium confirmed the authenticity of the plant samples. The plant’s voucher specimen, labeled with the accession number 57065, has been stored in the herbarium for forthcoming reference.
Analytical-grade medicines and substances were employed in this investigation. Ethanol and Tween-80 were bought from Merk (Darmstadt, Germany). Folin-Ciocalteau reagent (FCR) and 1,1-diphenyl -2-picryl-hydroxyl radical (DPPH) were procured from Sigma Chemicals Co. (St. Louis, Missouri, United States). Diclofenac sodium, Azithromycin, Amoxicillin, Ciprofloxacin, and Fluconazole were purchased from the following suppliers, respectively (Square Pharmaceuticals Ltd., Bangladesh, and Incepta Pharmaceuticals Ltd., Bangladesh).
For the antimicrobial assay, gram-positive bacteria (Sarcina lutea, Bacillus megaterium, S. aureus, Bacillus cereus, and Bacillus subtilis), gram-negative bacteria (Vibrio mimicus, Pseudomonas aeruginosa, S. typhi, Salmonella paratyphi, E. coli, Shigella dysenteriae, and Vibrio parahemolyticus) and fungi strains (Aspergillus niger, Sacharomyces cerevacae, and C. albicans) were utilized, provided from University of Dhaka, Bangladesh.
The whole plant of C. affinis was thoroughly washed and then sun-dried. After drying the moisture content was around 10%. Afterward, a grinding apparatus was used to process the dried plant into a coarse powder. Then 800 gm of dried leaf power was placed into a 5L round-bottomed clean flask. This 2.5L of ethanol (analytical grade, with ≥96% purity) was added and kept for 21 days with periodic shaking and stirring. After that, the entire mixture (32% w/v) was filtered using Whatman No.1 filter paper, followed by a new cotton plug. The method was repeated thrice with analytical-grade ethanol. Buchi Rotavapor was then used to decrease the filtrate content at low temperatures and pressures. The crude extract weighed 60.82 g (7.57% yield value).
The Kupchan fractionation method was employed to fractionate the crude ethanolic extract of C. affinis. This method, originally detailed by VanWagenen et al. (1993), involves solvent-solvent partitioning using a series of solvents with increasing polarity (VanWagenen et al., 1993). In this study, the crude extract was sequentially fractionated with n-hexane, dichloromethane (DCM), ethyl acetate (EA), and distilled water. Each solvent targets compounds based on their polarity, with n-hexane extracting non-polar compounds, DCM extracting slightly polar compounds, EA extracting moderately polar compounds, and water-extracting highly polar compounds. After fractionation, rotary evaporation was employed to concentrate each fraction, resulting in the following yields: hexane soluble fraction (HSF, 12.7 g), DCM soluble fraction (DCMSF, 10.35 g), EA soluble fraction (EASF, 15.1 g), and water-soluble fraction (AQSF, 9.8 g). This process exemplifies the systematic and efficient isolation of different compound classes, which is characteristic of the Kupchan method.
The whole plant extract of C. affinis was subjected to electron impact ionization (EI) to extract beneficial substances. This analysis has been performed utilizing a SHIMADZU GC-MS QP-2020 instrument with an AOC-20s auto-sampler and an AOC-20i auto-injector. The analysis utilised an SH Rxi 5M Sill column with dimensions of 30 m × 0.25 mm and a particle size of 0.25 μm. The carrier gas used was helium, having 1.72 mL/min flow pressure. The oven temperature adhered to a pre-established pattern, beginning at 80 °C (maintained for 2.00 min, increased at a rate of 5 °C/min) and achieving 150 °C (maintained for 5.00 min), ultimately attaining an ultimate temperature of 280 °C (maintained for 5.00 min). In splitless injection mode, a 5.0 μL injection volume was used with a split proportion of 50:1, while the injector was heated to 220 °C and the ion source to 280 °C. A mass spectrometric ionization analysis was carried out at an energy of 70 electron volts (eV), encompassing a mass range from 45 mass-to-charge ratio (m/z) to 350 m/z, during 50.0 min. A solvent cutting time of 5.0 min and a total running duration of 55.0 min were used. Bioactive substances were recognized by calculating their proportion from the entire peak area, using their retention time and MS fragment ions. The secondary metabolites were ascertained by comparing respective mass spectra with the records in the NIST08, NIST08s and NIST14 libraries. This method assisted in the establishment of structures, compound names and molecular weights of metabolites (Kim et al., 2016; Obaidullah et al., 2021).
The primary phytochemical analysis of crude ethanolic extract of C. affinis was carried out following the protocols that were established by Harborne (1998) and Edeoga et al. (2005). The existence of a wide range of secondary metabolites was investigated in this article. These metabolites included tannins, sterols, carbohydrates, quinones, alkaloids, phenolic resin, fatty oils, proteins and amino acids, glycosides, terpenoids, hormones, saponins, phlobatannins, and flavonoids (Harborne, 1998; Edeoga et al., 2005).
The Folin-Ciocalteu reagent served as the oxidizing agent, and gallic acid was used as the standard for the measurement of the total phenolic content of different fractionated extractives of C. affinis as per established methodology (Škerget, 2005). After adding 0.5 mL of each of the four fractionated solutions (HSF, DCMSF, EASF and AQSF) with a concentration of 2 mg/mL, 2.5 mL of a Folin-Ciocalteu reagent that had been diluted 10 times with water and 2.0 mL of a Na2CO3 solution with a concentration of 7.5% (w/v) were subsequently added. The mixtures were allowed to ferment at room temperature for 20 min. After the 20 min of incubation, an absorbance reading was taken at 760 nm using a UV spectrophotometer. The total phenols content of each sample was determined by comparing this reading to a standard curve constructed from a gallic acid solution of different concentrations. The phenolic insides of the extracts were measured in milligrams of gallic acid equivalent (mg of GAE) per gram of extract.
The antioxidant potential is generally assessed using 1,1-diphenyl-2-picrylhydrazyl (DPPH) free radical method (Brand-Williams et al., 1995). In this study, 3.0 mL of a DPPH ethanol solution with a concentration of 20 μg/mL was combined with 2.0 mL of each sample of the four fractionated extractives of C. affinis (HSF, DCMSF, EASF and AQSF) and control sample at various serially diluted to some concentrations (spanning from 500 μg/mL to 0.977 μg/mL). During serial dilution, the concentrations were reduced to half each of the times. Then, the mixtures were stored in a place with complete darkness at room temperature for 30 min. Following the reaction period, a UV spectrophotometer was employed to measure the absorbance at 517 nm relative to ethanol used as a blank. As a positive control, tert-butyl-1-hydroxytoluene (BHT) was employed and observed with DPPH. The succeeding equation was utilized to estimate the free radical DPPH inhibition percentage:
Then % inhibitions of the test samples were plotted against the different concentrations utilized, and the IC50 was estimated from the graph during this phytochemical analysis.
To conduct the in vivo experiment, 4–5 weeks of swiss-albino mice of either sex were acquired from the Animal Resource Branch of the International Centre for Diarrhoeal Diseases and Research, Bangladesh (ICDDR,B). Standard polypropylene cages with a 12-h light-dark cycle were used to house the mice. Additionally, the experiment maintained other ideal circumstances such as a controlled room temperature of 24°C ± 2 °C with a relative humidity of 60%–70%. The subjects were provided with ICDDR,B formulated rodent chow and water (ad libitum). During the experiments, the guidelines regarding the use and care of laboratory animals were correctly followed. Due to the high sensitivity of mice to environmental changes, they were acclimated in the experimental environment for a minimum of 3–4 days before the experiment. All the ethical rules and regulations were also implemented while designing the research and experiments. An intraperitoneal anesthesia overdose of ketamine HCl (100 mg/kg) and xylazine (7.5 mg/kg) was administered to the mice models at the end of the experiment, followed by euthanasia. The institutional ethics committee gave its stamp of approval to all experiments that involved the use of laboratory animals (Zimmermann, 1983). The “Animal Ethics Number” for the test animal models of this work is 2023–01-04/SUB/A-ERC/002 approved by the Animal Ethics Committee, State University of Bangladesh.
The mice were given high oral doses of 2000 mg/kg ethanol soluble C. affinis crude extract under normal conditions of laboratories following the “Organization for Environmental Control Development” guidelines (OECD: Guidelines 420) fixed-dose method (Rudra et al., 2020)(Van den Heuvel M., 1984). After the oral administration, several parameters were measured within 72 h. No allergic reaction, behavioral change (sedation/excitability), or lethality was found. Therefore, considering the oral acute toxicity perspective, the chosen safe doses for the antidiarrheal activity study were adjusted at 200 and 400 (mg/kg, b.w; p.o).
The cytotoxicity of different extractives of C. affinis was examined on brine shrimp nauplii (Artemia salina), and as a reference standard anticancer substance, vincristine sulfate, was used (Meyer et al., 1982). To create a simulated saltwater solution for this experiment, 38 g of NaCl salt were dissolved in 1000 mL of distilled water. NaOH was then added to maintain a pH of 8.0. The each of the four fractionated extractives was dissolved in DMSO (dimethyl sulfoxide) at 50 µL/5 mL concentration to formulate the test model with simulated seawater. Each of the fractionated extractives and vincristine standard solution were serially diluted, starting from the concentration of 400 μg/mL to 0.78125 μg/mL and from the concentration of 20 μg/mL to 0.039 μg/mL, respectively. During serial dilution, the concentrations were reduced to half each time in both cases. Subsequently, ten fully developed live shrimps were placed in individual test tubes at room temperature (25°C ± 1 °C), and the quantity of deceased nauplii was assessed after a 24-h period.
where, N0 = Number of nauplii taken; N1 = Number of nauplii death.
The antimicrobial activity of four fractions of C. affinis ethanolic extract was assessed using the disc diffusion technique (Huys et al., 2002). Dried and sterilized filter paper discs (6 mm diameter) containing 100 µg of each test sample (HSF, DCMSF, EASF, AQSF) were placed on nutrient agar medium previously inoculated with test bacteria and fungi strains. Commercial antibiotic discs of Azithromycin, Amoxicillin, and Ciprofloxacin (30 µg/disc) and an antifungal disc of Fluconazole (30 µg/disc) were used as positive controls, while blank discs served as negative controls. To ensure even distribution, the plates were inverted and kept at 4°C for 24 h, then incubated at 37°C for another 24 h. Zones of inhibition, indicating antibacterial activity, were measured in millimeters (Alam et al., 2021a). Antibacterial activity was evaluated on the clinically isolated strains of gram-positive bacteria (S. lutea, B. megaterium, S. aureus, B. subtilis, B. cereus), gram-negative bacteria (V. mimicus, P. aeruginosa, S. typhi, S. paratyphi, E. coli, S. dysenteriae, and V. parahemolyticus) and fungi strains (A. niger, Sacharomyces cerevacae and C. albicans)
Following the protocol outlined by previous research, the anti-diarrheal activity of different fractions of C. affinis was tested on castor oil-induced diarrheal mice (Shoba and Thomas, 2001; Rudra et al., 2020). Each mouse was administered 1 mL of highly pure analytical grade castor oil to induce diarrhea, and the total number of feces excreted was recorded. Mice were divided into four groups: control, positive control, and two test groups, each containing five mice. The control group received 10 mL/kg of 1% Tween 80 in water orally, while the positive control group received 5 mg/kg of loperamide orally. The test groups were given C. affinis extract fractions at 200 mg/kg and 400 mg/kg, respectively. After treatment, diarrhea was induced with castor oil, and each mouse was housed in a separate cage with hourly floor lining changes. The anti-diarrheal effects were assessed by comparing the test groups to the control group, with fecal stool counts recorded over a 4-h observation period. The percent inhibition of diarrhea was calculated using the following equation.
The pain-relieving effectiveness of various fractions from C. affinis was examined using the acetic acid-induced writhing test methodology (Ahmad et al., 2010). The procedure is the injection of acetic acid into mice, resulting in a distinct writhing reaction caused by pain. The decrease in the number of writhes serves as an indicator of the analgesic agent’s efficacy. Groups of five mice each were designated as control, positive control, and test groups for four different fractions. All mice received 0.1 mL of acetic acid intraperitoneally to induce writhing. The positive control group was then orally given 5 mg/kg of diclofenac sodium. The test groups received C. affinis extract fractions at doses of 200 mg/kg and 400 mg/kg orally. Five minutes after acetic acid injection, the number of writhing movements was recorded over 25 min. The percentage of writhing inhibition was calculated using the following equation.
The statistical analysis was performed using GraphPad Prism 5.2 (GraphPad Software, Inc., La Jolla, CA, United States), and the results were expressed as mean ± standard error (SEM). One-way variance analysis (ANOVA) and Dunnett’s test were used to determine statistical significance; *p < 0.5, **p < 0.01, and ***p < 0.001 were deemed statistically significant.
The metabolites identified from different extracts of the whole plant extract of C. affinis underwent computational docking study utilizing well-known software tools, including PyRx, PyMoL 2.3, Discovery Studio 4.5, and Swiss PDB viewer (Hasnat et al., 2023).
The phytochemicals listed in Table 1 were queried in the PubChem database (https://pubchem.ncbi.nlm.nih.gov/) and their 3D structures were downloaded in SDF format. Additionally, the 3D SDF structures of loperamide (PubChem CID- 3955), amoxicillin (PubChem CID- 33613), and ascorbic acid (PubChem CID- 54670067) were obtained as standard references. These ligands, alongside their particular PubChem CIDs, were successively stacked into Discovery Studio 4.5. Strikingly, the Pm6 semi-empirical method was utilized to optimize all phytochemicals, improving docking precision (Taher et al., 2023).
A computational docking analysis was performed to find the antidiarrheal, antimicrobial, and anti-inflammatory potentiality of 48 identified metabolites from C. affinis in the whole plant. The 3D crystalized structures of target receptors utilized in this study were sourced from the Protein Data Bank RCSBPDB (https://www.rcsb.org) (Prlić et al., 2010) These structures include the kappa-opioid receptor (KOR) [PDB: 6VI4] (Che et al., 2020) and human delta-opioid receptor (DOR) [PDB: 4RWD] (Fenalti et al., 2015) for antidiarrheal docking research. Additionally, the Beta-ketoaryl-ACP synthase 3 receptor (KAS) [PDB: 1HNJ] (Qiu et al., 2001) and Dihydrofolate reductase (DHFR) [PDB: 4M6J] (Khatun et al., 2021) were used for antimicrobial docking analysis. In contrast, Glutathione reductase (GLR) [PDB: 3GRS] (Qiu et al., 2001) and Urate oxidase (URO) [PDB: 1R4U] (Retailleau et al., 2004) were employed for antioxidant docking investigations. Again, Epidermal growth factor receptor (EGFR) [PDB: 1XKK] (El Azab et al., 2021) and B-cell lymphoma 2 (BCL-2) [PDB:4LXD] (Nurdiansyah et al., 2023) were picked for investigation of cytotoxic activity. Last but not least, in the case of analgesic activity, Cyclooxygenase- 2 (COX-2) [PDB ID: 1CX2] (Muhammad et al., 2015) and Tumor necrosis factor alpha (TNF-α) [PDB ID: 2AZ5] (Kumar et al., 2021) were selected for site-specific docking. These proteins or receptors were stored in the PDB format. These proteins were processed using PyMOL 2.3 to remove water molecules and ligands/residues. Subsequently, non-polar hydrogen atoms were added to the biomolecules and optimized to their lowest energy state using the energy minimization tool in the Swiss PDB viewer (Hasnat et al., 2023).
Computer-based ligand-protein interaction analysis was conducted to predict probable binding interactions between phytochemicals and their target proteins. This process involved employing the advanced PyRxAutodock Vina for molecular docking and utilizing semiflexible modeling techniques. Initially, the protein was laden and organized as the intended macromolecule. Amino acids derived from literature sources and their matching identification codes were meticulously chosen to guarantee the accurate attachment of ligands only to the intended macromolecule.
In the case of the KOR [PDB: 6VI4], specific amino acids from the A chain, including Leu 103, Leu 107, Ser 136, Ile 137, Try 140, Ile 180, Trp 183, Leu 184, Ser 187, Ile 191, Leu 192, Ile 194, and Val 195, were chosen for docking (Shompa et al., 2024). Ala9, Ile16, Lys54, Lys55, Thr56, Leu75, Ser76, Arg77, Glu78, Arg91, Ser92, Leu93, Gly117, Ser118, Ser119, and Val120 were picked for DHFR [PDB: 4M6J] (Khatun et al., 2021) However, the site specific amino acids for EGFR [PDB: 1XKK] were Leu 718, Val 726, Ala 743, Lys 745, Met 766, Lys 775, Arg 776, Leu 777, Leu 788, Thr 790, Gln 791, Leu 792, Met 793, Gly 796, Cys 797, Leu 799, Asp 800, Arg 803, Leu 844, Thr 854, Asp 855, and Phe 856 (Taher et al., 2023). Also, in the case of COX-2 [PDB ID: 1CX2] amino acids, including His 90, Gln 192, Val 349, Leu 352, Ser 353, Tyr 355, Tyr 385, Ala 516, Phe 518, Val 523, Ala 527, and Ser 530 were selected for docking. Furthermore, Tyr59, Tyr119, Leu 120, Gly 121, and Tyr 151 amino acids within the A chain, along with Tyr 59, Ser 60, Gln 61, Tyr 119, Leu 120, and Gly 121 amino acids in the B chain of TNF- α, were selected for site-specific docking, as detailed by (Shahriar et al., 2024).
Prankweb was utilized to determine the probable active site of the rest of the target proteins (Jendele et al., 2019). Val 62, Leu 65, Gly 66, Leu 69, Val 70, Phe 72, Gly 73, Tyr 77, Pro 315, Val 316, Ala 319, Phe 325, Cys 328, Phe 329, Gln 331, and Leu 332 of were picked for A chain of DOR [PDB: 4RWD]. Consequently, prankweb was used to select amino acids including Trp 32, Arg 36, Thr 37, Thr 81, Ala 109, Ala 110, Ala 111, Cys 112, Leu 142, Gly 152, Ile 155, Ile 156, Phe 157, Leu 189, Thr 190, Leu 191, Leu 205, Met 207, Gly 209, Asn 210, Val 212, Phe 213, Ala 216, Leu 220, His 244, Ala 246, Asn 247, Ile 250, Asn 274, Glu 302, Ala 303, Phe 304, Gly 306, and Gly307 for KAS [PDB: 1HNJ], as well as Ile 26, Gly 27, Gly 29, Ser 30, Gly 31, Val 49, Glu 50, Ser 51, Lys 52, Gly 56, Thr 57, Cys 58, Val 61, Gly 62, Cys 63, Lys 66, Lys 67, Gly 128, His 129, Ala 130, Ala 155, Thr 156, Gly 157, Gly 158, Met 159, Ser 177, Phe 181, Tyr 197, Ile 198, Glu 201, Met 202, Arg 291, Asn 294, Leu 298, Asp 331, Leu 337, Leu 338, Thr 339, Pro 340, Ala 342, Val 370, and Phe 372 for GLR [PDB: 3GRS]. Additionally, amino acids Phe 159, Phe 162, Thr 168, Leu 170, Lys 171, Thr 173, Arg 176, Ile 177, Val 227, Gln 228, Asn 254, His 256, Tyr 257, Phe 258, Glu 259, Phe 278, Pro 284, Gly 286, Leu 287, Ile 288 for URO [PDB: 1R4U] (Jendele et al., 2019) were selected for site-specific docking. Lastly, Phe 101, Asp 108, Phe 109, Met 112, Val 130, Glut 133, Leu 134, Arg 143, Ala 146, Glu 149, Phe 150, and Val 153 were selected for BCL-2 [PDB: 4LXD].
As for the standard, loperamide was used against KOR and DOR, while amoxicillin was chosen for KAS and DHFR. Ascorbic acid was utilized against GLR and URO, while lapatinib was utilized as the standard in the case of EGFR and BCL-2. Finally, diclofenac was employed for COX-2 and TNF-α. Furthermore, the ligands’ PDB files were uploaded and converted to pdbqt format using the Open Babel feature in PyRxAutoDock Vina software. This allowed us to find the best binding interactions while docking with the chosen macromolecules.
The grid box was generated by ensuring that the active binding sites of the protein were enclosed within the designated box, as determined through grid mapping. The KOR grid box is positioned with a center at X = 41.2113828749, Y = −54.1291662632, and Z = −22.5997323179, with dimensions X = 16.7196551518, Y = 27.2876737202, and Z = 16.5467118974. For DOR, the center was at X = −57.2878363954, Y = 2.11011895459, and Z = 53.1963612028, with dimensions x = 22.8756741846, y = 19.0839303805, and z = 20.669369331. KAS maintained a center at X = 28.9339098379, Y = 17.5191106637, Z = 31.6525120583, with dimensions X = 26.0697735011, Y = 34.8553116374, and Z = 22.3970043137. DHFR had a center at X = 2.99808311921, Y = −3.54674634211, and Z = −18.5871843936, with dimensions X = 19.9283113118, Y = 27.7380574575, and Z = 27.1400591135. GLR was positioned at X = 60.8957977373, Y = 50.7392192752, Z = 15.8962732414, with dimensions X = 35.4645585801, Y = 24.3261287651, and Z = 26.4858683614. Again, URO was fixed at a center of X = 31.325851641, Y = 25.0311624292, and Z = 44.6854822753, with dimensions X = 19.2797025429, Y = 27.2857277055, and Z = 27.0044877641. For EGFR, the grid box peaked at a center of X = 15.9440369259, Y = 34.4198880619, and Z = 35.8044407692, with dimensions X = 24.7750487506, Y = 19.7793076754, and Z = 32.2557047924. Conversely, BCL-2 was centered at X = 27.0730446356, Y = 28.4708961863, and Z = 5.29432825771, with dimensions X = 16.1526029895, Y = 20.5518919333, and Z = 22.8016796153. Also, center X = 23.011633329, Y = 20.9981639946, and Z = 15.518303992 and dimension X = 21.4798597234, Y = 18.5617032509, and Z = 23.8823675559 were maintained for COX-2. For TNF-α, the center was positioned at X = −19.7381611157, Y = 74.2118779135, and Z = 37.7182960594, with dimensions X = 19.5448302136, Y = 22.3686683962, and Z = 15.1249201064.
The leftover settings were set to their default configurations during the docking process. After that, AutoDock Vina (version 1.1.2) guaranteed a uniform set of circumstances for computer-dependent molecular docking of the phytochemicals. In the end, BIOVIA Discovery Studio version 4.5 was used to carefully examine all docking tests, helping to identify the best models through a thorough analysis of both 2D and 3D configurations.
In contemporary drug design, computational methods focusing on pharmacokinetics (absorption, distribution, metabolism, excretion, and toxicology) and assessing bioavailability through drug-like properties have gained substantial traction. In drug discovery, ADMET analyses are pivotal tools for unraveling the pharmacological landscape (http://biosig.unimelb.edu.au/pkcsm/prediction). Furthermore, Swiss ADME (http://www.sib.swiss), an online platform, was employed to forecast drug likeliness based on Lipinski rules and pharmacokinetic parameters for various compounds. According to Lipinski’s criteria, a compound is considered orally viable if it satisfies the following conditions: the compound should have a molecular weight of less than 500 atomic mass units (amu), no more than 5 hydrogen bond donor sites, no more than 10 hydrogen bond acceptor sites, and a lipophilicity value (LogP) of 5 or less (Taher et al., 2024).
During the gas chromatography-mass spectrometry (GC-MS) analysis, various portions of the plant being examined exhibited a combined total of 67 peaks, each representing a bioactive compound. The identity of these molecules was determined by comparing their molecular mass, chemical formula, and peak retention time with the properties of compounds recognized in the NIST library. This investigation seeks to provide insight into the various bioactive metabolitess found in several extracts of C. affinis, which make a major contribution to the existing body of knowledge.
A DCM-based extract of C. affinis revealed the presence of 20 chemicals, as detailed in Table 1 and depicted in Figure 2. The relative concentration of each compound was expressed as a peak area percentage. Noteworthy compounds with substantial prevalence included Phenol, 3,5-bis(1,1-dimethylethyl)- (48.952%), 9-Octadecenoic acid (Z)-, methyl ester (8.161%), Estragole (5.673%), 15-Tetracosenoic acid, methyl ester, (Z)- (4.806%), Undecanoic acid, 10-methyl-, methyl ester (4.366%), 9-Methoxybicyclo[6.1.0]nona-2,4,6-triene (3.249%), and Di-n-octyl phthalate (3.059%). The rest of the compounds are found within a concentration of less than 3%. The retention time, mass/charge ratio and the peak area are also predicted in Table 1.
Figure 2. GC-MS chromatogram of different fractions of ethanolic extract of Colocasia affinis whole plant, here (A, B,C) and (D) represent n-hexane, DCM, ethyl acetate and aqueous fractions, respectively.
Six compounds were identified in the case of ethyl acetate extract of the plant (Table 1, Figure 2). Here Phenol, 2,6-bis (1,1-dimethyl ethyl)- presented with highest 63.907% concentration, followed by Diisooctyl phthalate with 14.403%, Estragole with 8.399%, Tetracosane with 5.621%, Heptadecane, 2,6,10,15-tetramethyl- with 4.555%, and Phenol, 3,5-bis (1,1-dimethyl ethyl)- with 3.116% concentration.
Seven peaks were detected in the plant hexane extract. The compound with the highest concentration is Phenol, 3,5-bis(1,1-dimethylethyl), constituting 83.898% of the total. Additionally, Epoxy-linalool oxide, 9-methoxybicyclo [6.1.0] nona-2,4,6-triene, and 1-tetradecene are present at concentrations of 3.325%, 3.254%, and 3.127%, respectively.
The highest 34 compounds have been identified from the aqueous fraction of the C. affinis. The most prominent peak area with a concentration of 10.746% was observed for Phenol, 3,5-bis(1,1-dimethylethyl)-. Other notable compounds included Undecane, 5,7-dimethyl- (6.911%), 2,3-Dimethyldodecane (6.761%), Dodecane, 2,6,10-trimethyl- (6.617%), Decane, 2,3,5,8-tetramethyl- (5.236%), Tridecane (4.886%), Tetracosane (3.738%), Dodecane (3.467%), Undecane, 2,4-dimethyl- (3.378%), Heptadecane, 2,6,10,15-tetramethyl- (3.320%), and Heneicosane (3.237%). Details are outlined in both Table 1 and Figure 2.
Several extracts of C. affinis were subjected to preliminary phytochemical analysis, which identified tannins, sterols, carbohydrates, quinones, saponins, alkaloids, phenolic compounds, flavonoids, glycosides, terpenoids, and steroids (Table 2).
The extent of total phenolic content of different fractions of C. affinis varied from 7.98 to 57.23 mg of GAE/gm of extractives (Table 3). The plant’s aqueous fraction (AQSF) had the highest concentration of phenolic content, whereas the dichloromethane soluble fraction (DCMSF) displayed a significant proportion. Figure 3 shows the standard curve of gallic acid used to determine the total phenolic content of various extracts.
Table 3. Total phenolic content, free radical scavenging and cytotoxic activities of different extracts of C. affinis with respective standards.
Figure 3. Analysis of Cytotoxic and Antioxidant Attributes of Colocasia affinis Fractions: (A) Standard curve of gallic acid for determining total phenolic content in various fractions. (B) Linear regression equations (IC50) for butylated hydroxytoluene (BHT) and different fractions observed with DPPH assay. (C) Percentage of radical scavenging activities of BHT and different fractions. (D) Linear regression equations (LC50) for vincristine sulfate (VS) and different fractions.
The DPPH free radical scavenging investigation showed that several fractionated extracts of C. affinis exhibited promising free radical scavenging properties in phytochemical analysis. AQSF manifested the most pronounced scavenging attributes, with an IC50 value of 29.40 μg/mL, compared to the standard BHT, with an IC50 value of 22.13 μg/mL. The IC50 values, calculated by linear regression equation, and the respective percent (%) scavenging attribute of BHT and the fractions are summarized in Table 3 and Figure 3.
The LC50 values of different fractions of C. affinis and the standard were 14.51 μg/mL (HSF), 7.02 μg/mL (EASF), 3.21 μg/mL (DCMSF), 1.36 μg/mL (AQSF), and 0.451 μg/mL (VS), respectively. The respective data are presented in Figure 3 and Table 3. Among all the fractions, AQSF showed the most promising cytotoxicity activity.
The antibacterial activity of all the partitions was tested against seven strains of gram-positive bacteria, seven strains of gram-negative bacteria, and three strains of fungi. As a reference, standard Azithromycin, Amoxicillin, Ciprofloxacin, and Fluconazole were taken to test the respective antimicrobial activity. The zone of inhibition (ZOI) of the test samples ranged from 5 mm to 20 mm, summarized in Table 4. The AQSF and DCMSF showed considerable antibacterial activity, whereas the EASF and AQSF exhibited promising antifungal attributes. As per ZOI, the fractionated extracts exerted notable antimicrobial activities against B. cereus, B. megaterium, S. aureus, S. lutea, E. coli, P. aeruginosa, S. paratyphi, S. dysenteriae, A. niger and relatively lower ZOI against B. subtilis, S. typhi, V. mimicus, V. parahemolyticus, C. albicans and Sacharomyces cerevacae.
Table 4. The antimicrobial activity of Colocasia affinis extracts and standard against Gram-positive bacterial, Gram-negative bacterial and fungal strains.
DCMSF, AQSF, HSF, and EASF fractions at 200 and 400 mg/kg doses exhibited significant (p < 0.05, p < 0.01, p < 0.001) reduction in the number of feces (Table 5). In terms of wet feces number, DCMSF, AQSF, HSF and EASF demonstrated percentages of diarrhea inhibition respectively by 39.53%, 18.6%, 11.63% and 6.98% at 200 mg/kg dose; while at 400 mg/kg dose the values were 51.16%, 27.91%, 20.93% and 16.28% respectively. Whereas, the value of the standard loperamide was 67.44%.
Table 5. The antidiarrheal and analgesic effect of different extracts of Colocasia affinis respectively on castor oil-induced and acetic acid-induced test in mice.
DCMSF, AQSF, HSF, and EASF fractions at 200 and 400 mg/kg doses exhibited significant (p < 0.05, p < 0.01, p < 0.001) analgesia with a considerable percent reduction of acetic acid-induced writhing compared to the standard diclofenac sodium (Table 5). Though the sample extracts did not exhibit dose-dependent percent reduction except the EASF, the DCMSF showed a 52.33% reduction compared to the standard of 77.91%.
The whole plant extract of C. affinis revealed the presence of 48 identified compounds, which underwent computational docking tests against various receptors. Table 6 displays the binding strengths of multiple substances. Notably, for target KOR, compound C19 displayed a significant binding affection with a value of −6.9 kcal/mol, followed by C9 (−5.8 kcal/mol). C8 and C14 exhibited the same binding affinity (−5.7 kcal/mol), comparable to the standard Loperamide with a value of −7.7 kcal/mol. Regarding receptor DOR, C2, C4, C8, and C37 demonstrated the most significant binding affinity with a value of −7 kcal/mol. However, the standard Loperamide outperformed them with a binding strength of −8.9 kcal/mol. Moreover, C19 demonstrated the highest binding affinity against KAS, scoring −7.1 kcal/mol, while Compound 23 exhibited promising affinity with a value of −6.4 kcal/mol. C12 and C21 also displayed strong affinities for binding to the receptor, with values of −6.3 kcal/mol. The standard Amoxicillin had a binding affinity of −7.1 kcal/mol. Compared to the standard Amoxicillin binding score of −7.6 kcal/mol, C19 demonstrates a significant affinity for DHFR with a binding score of −7.4 kcal/mol. Additionally, C8, C9, C18, and C23 also exhibit noteworthy binding affinity to the receptor, with scores of −6.1, −6.4, −6.1, and −6.3 kcal/mol, respectively Moving to receptor GLR, C23 exhibited the highest binding affinity at −7.3 kcal/mol, and C19 also showed strong binding affinity with a score of −7 kcal/mol. Additionally, C12 and C18 displayed strong affinities for binding to 3GRS, with values of −6.2 and −6.9 kcal/mol, respectively. On the other hand, standard Ascorbic acid possessed a binding ability of −6.4 kcal/mol. Furthermore, C18 scored −5.4 kcal/mol against 3GRS, while C8 and C9 demonstrated the same prominent affinities of −5.7 kcal/mol. Interestingly, C19 exhibited very notable activity towards URO with a value of −6.8 kcal/mol, surpassing the standard Ascorbic acid, which scored −5.3 kcal/mol. Regarding EGFR, C19 exhibited the lowest affinity score at −7.8 kcal/mol, followed by C23 with a score of −7.4 kcal/mol. Additionally, C12 and C21 demonstrated noteworthy affinity towards the receptor with scores of −7.1 kcal/mol and −7 kcal/mol, respectively, compared to the Lapatinib binding affinity of −10.6 kcal/mol. Also, C19 demonstrated the highest activity against BCL-2, showing an affinity of −6.7 kcal/mol compared to the standard Lapatinib affinity of −8.4 kcal/mol. Meanwhile, C2, C4, C21, and C23 exhibited promising affinities of −6.3, −6.4, −6.3, and −6.6 kcal/mol towards the receptor. The C19 suppressed the binding affinity of standard Diclofenac against COX-2 with a binding score of −7.9 kcal/mol, whereas the Diclofenac showed −7.8 kcal/mol. Moreover, C23 manifested equal binding affinity as standard. Promising binding affinities were also observed for C9, C14, C18, and C21 with affinities of −7.3, −7.2, −7.4, and −7.2 kcal/mol, respectively. Finally, in the case of TNF-α, C19 exhibited a very potential binding effect with an affinity of −7.4 kcal/mol, which easily suppressed the affinity of Diclofenac (−7.1 kcal/mol). Also, C8, C9, C18, and C23 registered affinities of −6.5, −6.7, −6.3, and −6.5 kcal/mol, respectively. The ADME/T analysis of selected compounds with prospective value in molecular docking simulation studies has been subjected to ADME/T analysis to have a prior safety and efficacy profile. The compounds also show promising safety and efficacy backgrounds in this computer-based analysis.
Table 6. Binding affinities of the identified compounds and standards against ten receptors representing antidiarrheal, antimicrobial, antioxidant, cytotoxic, and analgesic activities.
Vegetables abound in a multitude of vitamins, minerals, nutritive phytochemicals or secondary metabolites that carry diverse phototherapeutic properties (Wolfender et al., 2011). In both modern and folk medicine systems, the secondary metabolites of vegetables, namely, alkaloids, fatty acids, flavonoids, phenolic compounds, tannins and so on, have provided ethnopharmacological actions such as, analgesic, antidiarrheal, antimicrobial, and antioxidant activities (Alam et al., 2021b; Nakaziba et al., 2021). Based on previous research, 80% of its 122 drug moieties derived from phyto sources closely aligned with their original ethnopharmacological purposes (Nakaziba et al., 2021). Consequently, vegetables deciphering therapeutic potentials can open a new well-spring for novel drug discovery or enhance existing therapeutics.
Plant extracts often consist of complex mixtures of secondary metabolites obtained from plants, animals, and microbes. These extracts usually comprised 10 to 60 metabolites with different quantities, but their biological properties are mainly attributed to two to four significant molecules (Rahman et al., 2013). Through the analysis of the chemical composition and arrangement of samples, numerous biological capabilities can be revealed inside extracts from medicinal plants. Remarkably, there is a conspicuous lack of published studies employing GC-MS/MS to characterize bioactive compounds in the C. affinis plant. To fill this gap, a well-organized inquiry was conducted, which involved the use of GC–MS/MS analysis. Phenolic compound 3,5-bis(1,1-dimethylethyl), was notably abundant in all extract fractions except for EA. Conversely, its analogous phenolic compound, 2,6-bis(1,1-dimethylethyl), demonstrated a substantial presence, accounting for 63.907%, specifically within the EA fraction (Table 1). Research showed that phenol, 3,5-bis(1,1-dimethylethyl)- also known as 3,5-di-tert-butylphenol demonstrated antifungal effects on Candida strains by inhibiting biofilm formation and affecting planktonic cell viability. It caused structural alterations in free-floating and surface-attached cells, notably impacting cell membrane integrity. Additionally, 3,5-DTB exhibited synergism with sodium dodecyl sulfate (SDS), disrupting membrane integrity further. The chemical also generated reactive oxygen species (ROS) in Candida, adding to its anti-biofilm function (Vijayakumar and MuhilVannan, 2021). However, this compound and its equivalent 2,6-ditert-butylphenol or Phenol, 2,6-bis (1,1-dimethyl ethyl)- has been documented to have several activities, including antioxidant, cytotoxic, insecticidal, and nematicidal. antibacterial, and antiviral (Zhao et al., 2020). A considerable amount of fatty acid, especially methyl esters, was observed from different plant fractions (Table-). 9-Octadecenoic acid (Z)- exhibits notable efficacy as a potent antibacterial and antiviral compound, showcasing promising capabilities in combating microbial infections (Hadi and Hussein, 2016). Estragole is commonly used in food flavoring, and while studies indicate its potential carcinogenicity, controversial mutagenicity results exist, with its key biological activity linked to the formation of hepatic DNA adducts by metabolites (De Vincenzi et al., 2000). Diisooctyl phthalate, a derivative of phthalic acid esters, belongs to a group of lipophilic chemicals known as plasticizers. These metabolites are extensively utilized to enhance mechanical extensibility and flexibility in various products. Additionally, they are recognized for exhibiting diverse biological activities, including allelopathic, antimicrobial, and insecticidal properties (Huang et al., 2021).
Flavonoids are a class of organic chemicals with a wide range of phenolic structures. They are commonly found in many natural sources, such as fruits, vegetables, grains, bark, roots, stems, flowers, tea, and wine. These appeared to have several bioactivities, including anti-diabetic, anti-inflammatory, antibacterial, antioxidant, antiviral, cytotoxic, and lipid-lowering activities (Hasnat et al., 2024). The apparent reason for the antioxidant, anti-inflammatory, antibacterial, and cytotoxic activities in leaf extract of C. affinis is accountable owing to the presence of flavonoids found throughout the botanical herb (Table 2).
Antioxidants play a vital role in neutralizing reactive oxygen and nitrogen species generated by the human immune system, thereby mitigating oxidative stress. This stress, if left unaddressed, has the potential to harm cells and tissues by causing damage to DNA, lipids, and proteins (Rajlic et al., 2023). Several studies reported eicosane, 15-tetracosenoic acid derivatives, and phenol derivatives metabolites to exhibit antioxidant activity (Wojdyło et al., 2007; Medaowe et al., 2020; Balachandran et al., 2023). Interestingly, GC-MS data from C. affinis fractions also demonstrated the presence of these metabolites. Quantitative analysis of C. affinis AQSF showed a maximum total phenolic content of 57.23 mg GAE/g, among other things. Furthermore, AQSF demonstrated remarkable antioxidant activity in the DPPH scavenging assessment, with an IC50 value of 29.40 μg/mL. The different fractions also deciphered promising antioxidant attributes, which may validate the presence of antioxidant metabolites in GC-MS data.
The analgesics alleviate pain sensation via several mechanisms, including inhibition of cyclooxygenase enzymes (COX-1 and COX-2), inhibition of activated transcription factors, and binding with receptors which increase transcription of anti-inflammatory proteins. Anti-inflammatory agents also relieve pain associated with inflammatory conditions by interfering with the biosynthesis of inflammatory mediators (Alam et al., 2020; Phull et al., 2022). Azulene, eicosane, heneicosane, and some phenolic compounds found in C. affinis extractives have been reported to exhibit substantial analgesic and anti-inflammatory activities (Ambriz-Pérez et al., 2016; Okechukwu, 2020; Mousa et al., 2022). Probably for this reason, during the analgesic assay of C. affinis fractionated extracts, notable analgesic actions were observed ranging from 16.28% to 52.33% compared to standard diclofenac sodium with 77.91% inhibition of writhing. Furthermore, cancer is a widespread health condition characterized by unregulated cell proliferation and the capacity to infiltrate or inflame surrounding tissues. Cytotoxic compounds can halt the expansion of cancer cells (Guzow-Krzemińska et al., 2019). Estragole and Tetracosane are the metabolites found in C. affinis as per GC-MS data and possess cytotoxic activities (Uddin et al., 2012; Andrade et al., 2015). In quantitative cytotoxicity analysis of different C. affinis fractions, DCMSF (LC50 3.21) and AQSF (LC50 1.36) exerted promising cytotoxic properties, among others, followed by EASF and HSF when compared to vincristine sulfate (LC50 0.451). The cytotoxic activities of these fractions may occur due to the presence of above-mentioned metabolites. An antidiarrheal compound is a substance or medication that exerts its effects through mechanisms to mitigate or prevent diarrhea. These metabolites typically target various mechanisms, such as slowing down bowel movements, reducing intestinal inflammation, augmenting water absorption or addressing the underlying cause of diarrhea to restore normal bowel function (Beserra et al., 2016). The DCMSF of C. affinis exerted significant antidiarrheal activity with 51.16% diarrheal inhibition followed by AQSF, HSF, and EASF, compared to 67.44% diarrheal inhibition by standard loperamide. The presence of azulene in DCM soluble fraction can be the underlying cause of such activity, as azulene has been reported to have a promising antidiarrheal and anti-inflammatory potential in previous (Aziz et al., 2011; Bakun et al., 2021) Bacteria inherently possess a genetic inclination to evolve resistance, underscoring the need for the continual development of novel antimicrobial drugs to combat a wide range of microbes (Nascimento et al., 2000). The antimicrobials target diverse mechanisms of action such as disruption of microbial cell wall synthesis, the intervention of key enzymatic pathways, and interference with the production of genetic materials and proteins (Epand and Vogel, 1999; Ghannoum and Rice, 1999). The GC-MS analysis of C. affinis fractionated extracts has exerted the presence of eicosane, heneicosane, estragole, and some other phenolic compounds, which show significant antifungal and antimicrobial activities according to previous studies (Park et al., 2001; Donati et al., 2015; Vanitha et al., 2020; Octarya et al., 2021)AQSF and DCMSF showed relatively higher ZOI, followed by DCMSF against both gram-positive and gram-negative bacteria. The presence of the antimicrobial estragole and phenolic compounds in these fractions is the reason for such attributes. Besides, the antifungal compounds eicosane and heneicosane in AQSF and EASF made them exhibit prominent antifungal properties.
The modulation of gastrointestinal (GI) signaling in the human body involves opioid receptors, namely, µ, ƙ, and δ receptors. These receptors exercise their impact by limiting the activity of enteric nerves, reducing the discharge of neurotransmitters, and reducing the strength of excitatory and inhibitory motor pathways. Consequently, this cascade of effects leads to delayed colonic transit, diminished excitability of enteric nerves, and modifications in secretion and fluid transport. Ultimately, these alterations culminate in changes to motility and stool consistency (Pannemans and Corsetti, 2018). Sevaral compounds from C. affinis have displayed noteworthy potential against KOR, with C19 exhibiting the highest affinity (−6.9 kcal/mol). It forms bonds with five amino acids through alkyl interactions. In contrast, C9 establishes pi-alkyl bonds, resulting in a binding score of −5.8 kcal/mol. This is compared to the standard Loperamide, which forms a single pi-alkyl bond and two alkyl bonds, achieving a binding score of −7.7 kcal/mol (Table 7; Figure 4). Meanwhile, C2, C4, C8, and C37 demonstrated significant activity against DOR, showing binding affinities of −7 kcal/mol. They connect to the receptor through four pi-alkyl, eight alkyl, six alkyl, and eight alkyl bonds, respectively. The standard Loperamide binds to this receptor through four alkyls, three pi-sigma, and a single carbon-hydrogen bond, forming a more favorable binding score of −8.9 kcal/mol (Table 7; Figure 4). Beta-ketoacyl-ACP synthase 3 is an essential enzyme that plays a key part in antimicrobial activities in microbial species. It contributes actively to mycolic acid synthesis, an essential component of the microbial cell wall, by participating in the last steps of FAS-II condensation and extension. Mycolic acid biosynthesis may be hampered by blocking the activity of this enzyme, making it a viable target for antimicrobial therapies against such pathogenic diseases (Singh et al., 2011). According to the computational docking analysis, C19 demonstrated a binding affinity of −6.8 kcal/mol against KAS, nearly matching the −7.1 kcal/mol binding score of the standard Amoxicillin. C19 established connections through two alkyl bonds and a conventional hydrogen bond. Conversely, the standard Amoxicillin interacted with KAS through two conventional hydrogen bonds, a single alkyl bond, pi-sigma interaction, and unfavorable donor-donor interactions. In addition, C12, C21, and C23 also exhibited potential affinity towards the receptor (Table 7; Figure 5). The bacterial dihydrofolate reductase (DHFR) is essential in producing thymidylate, making it a highly potential candidate for the treatment of infections. Inhibitors of DHFR can lead to bacterial death, providing a potential avenue for addressing microbial infections (He et al., 2020). Furthermore, disturbances in the folate pathway, facilitated by DHFR, might lead to unregulated cell proliferation, affecting cellular proliferation and development in different cancers (Kodidela et al., 2016). Ciprofloxacin formed two conventional hydrogen bonds, two alkyl bonds, and a single carbon-hydrogen and pi-sigma bond when interacting with DHFR. This interaction resulted in a binding score of −7.6 kcal/mol. In contrast, C19 demonstrated a highly promising affinity with a score of −7.4 kcal/mol, forming a single pi-sigma and alkyl bonds. Conversely, C8, C9, C18, and C23 displayed affinities lower than −6 kcal/mol, establishing 2, 3, 4, and 2 different types of interactions, respectively (Table 7; Figure 5). Glutathione reductase plays a crucial role in maintaining the antioxidant activity of the tripeptide glutathione. Catalyzing the regeneration of its reduced form from the oxidized state ensures the balance of the cellular redox state. This enzyme, featuring flavin adenine dinucleotide as a redox-active group, utilizes nicotinamide adenine dinucleotide phosphate (NADPH) as a specific reductant to convert glutathione disulfide (GSSG) back to its reduced state. The specificity of glutathione reductase for NADPH and its selective activity with certain substrates highlights its significance in preserving the cellular antioxidant defense system (Mannervik, 1999). Regarding GLR, C23, C19, and C18 exhibited inhibitory effects on the standard Ascorbic acid binding affinity, which was measured at −6.4 kcal/mol. Specifically, C23 formed a binding score of −7.3 kcal/mol by engaging a conventional hydrogen bond, two pi-pi bonds, and six alkyl bonds. In contrast, C19 demonstrated a binding score of −7 kcal/mol by establishing three pi-alkyl bonds and single van der Waals and aide pi-stacked interactions. Additionally, C18 bound with a score of −6.9 kcal/mol, utilizing five alkyl bonds, two conventional hydrogen bonds, and an amide pi-stacked bond. In comparison, Ascorbic acid displayed binding through four conventional hydrogen bonds and an unfavorable donor-donor interaction (Table 7; Figure 6). The uric acid degrading URO, also known as uricase, converts uric acid into 5-hydroxy isourate and H2O2, amplifying oxidant stress and its associated disorders (Cleveland et al., 2009). By inhibiting URO, certain identified metabolites demonstrate potential antioxidant activity. Notably, C19, C8, C9, and C18 exhibited a surprising enhancement in receptor affinity, with binding scores of −6.8, −5.7, −6.7, and −5.4 kcal/mol, respectively, surpassing the standard affinity of −5.3 kcal/mol. These improved affinities resulted from specific interactions between the ligands and receptors. For instance, C19 engaged in single Pi-alkyl, Pi-sigma, and Pi-Donor Hydrogen interactions, while C8 formed three alkyl bonds. C9 demonstrated two Alkyl bonds and a conventional hydrogen bond, and C18 interacted through three alkyl bonds, along with single pi-sigma, pi-donor hydrogen, and conventional hydrogen bonds. Notably, C18’s binding pattern surpassed that of Ascorbic acid, which formed four conventional hydrogen bonds (Table 7; Figure 6).
Table 7. Bond and binding site of highly active compounds against different targets including KOR, DOR, KAS, DHFR, GLR, URO, BCL-2, EGFR, COX-2, and TNF-α.
Figure 4. Molecular Interactions of Phytochemicals with KOR and DOR Enzymes: (I) Graphical representation of the molecular interactions of the most prominent phytocompounds with the KOR enzyme in 3D visualization (Compound 8 = A, Compound 9 = B, Compound 14 = C, Compound 19 = D, and Standard Loperamide = E). (II) Graphical representation of the molecular interactions of the most prominent phytocompounds with the DOR enzyme in 3D visualization (Compound 2 = A, Compound 4 = B, Compound 8 = C, Compound 37 = D, and Standard Loperamide = E).
Figure 5. Molecular Interactions of Phytochemicals with KAS and DHFR Enzymes: (I) Graphical representation of the molecular interactions of the most prominent phytocompounds with the KAS enzyme with 3D visualization (Compound 12 = A, Compound 19 = B, Compound 21 = C, Compound 23 = D, and Standard Amoxicillin = E). (II) Graphical representation of the molecular interactions of the most prominent phytocompounds with the DHFR enzyme with 3D visualization (Compound 9 = A, Compound 18 = B, Compound 19 = C, Compound 23 = D, and Standard Amoxicillin = E).
Figure 6. Molecular Interactions of Phytochemicals with GLR and URO Enzymes: (I) Graphical representation of the molecular interactions of the most prominent phytocompounds with the GLR enzyme with 3D visualization (Compound 12 = A, Compound 18 = B, Compound 19 = C, Compound 23 = D, and Standard Ascorbic acid = E). (II) Graphical representation of the molecular interactions of the most prominent phytocompounds with the URO enzyme with 3D visualization (Compound 8 = A, Compound 9 = B, Compound 18 = C, Compound 19 = D, and Standard Ascorbic acid = E).
EGFR, a pivotal regulator of cellular processes, undergoes conformational changes upon ligand binding, activating downstream pathways that promote cancer-related activities (Ongko et al., 2022). Elevated EGFR levels in gastric and breast cancers correlate with poor overall survival, advanced clinical stage, and resistance to therapy, emphasizing its prognostic relevance across various tumors. Additionally, in colorectal cancer, EGFR’s impact on tumor grade, stage, and survival underscores its significant role in cancer progression (Nicholson et al., 2001). In its interaction with EGFR, C19 formed one unfavorable acceptor-acceptor bond, one pi-sigma bond, and three alkyl bonds, yielding a binding affinity score of −7.8 kcal/mol. In contrast, C23 formed a solitary conventional hydrogen bond, a pi-sigma bond, and nine alkyl bonds, leading to a binding affinity of −7.4 kcal/mol. Additionally, C12 and C21 exhibited lower than −7 kcal/mol compared to the standard score of −10.6 kcal/mol (Table 7; Figure 7).
Figure 7. Molecular Interactions of Phytochemicals with EGFR and BCL-2 Enzymes: (I) Graphical representation of the molecular interactions of the most prominent phytocompounds with the EGFR enzyme with 3D visualization (Compound 12 = A, Compound 19 = B, Compound 21 = C, Compound 23 = D, and Standard Lapatinib = E). (II) Graphical representation of the molecular interactions of the most prominent phytocompounds with the BCL-2 enzyme with 3D visualization (Compound 2 = A, Compound 9 = B, Compound 19 = C, Compound 23 = D, and Standard Lapatinib = E).
BCL-2, initially identified as the first anti-death gene, is implicated in cancer through various mechanisms such as chromosomal translocations, gene amplification, and altered expression regulation. Dysregulation of BCL-2 and related antiapoptotic proteins often contributes to chemotherapy resistance, making them potential targets for cancer therapy. Elevated BCL-2 expression is associated with poor prognosis in various cancers, emphasizing its significance in cancer development and treatment outcomes (Yip and Reed, 2008). Compound 19 exhibited the most excellent affinity for the BCL-2 receptor, establishing two pi-alkyl bonds. On the other hand, C23, which formed two pi-sigma and three alkyl bonds, demonstrated notable affinity with a score of −6.6 kcal/mol. In contrast, Lapatinib established three distinct types of bonds with the receptor, achieving a higher score of −8.4 kcal/mol. Furthermore, nine identified metabolites displayed binding affinities below −6 kcal/mol (Table 7; Figure 7).
Implicated in inflammation, cyclooxygenase-2 (COX-2) generates prostaglandins that play a pro-inflammatory role in the initial stages of the inflammatory process. However, recent studies propose a dual role for COX-2, suggesting that the prostaglandins it produces in later stages may contribute to the resolution of inflammation. Inhibition of COX-2 has been demonstrated to reduce inflammation in specific experimental models, emphasizing the complexity of its involvement in inflammatory responses (Williams et al., 1999). In our research, C19 and C23 showed remarkable affinity towards cox-2, where C19 formed a single pi-sigma and five alkyl bonds with the receptor, showcasing a score of −7.9 kcal/mol, while C23 bound with two carbon-hydrogen, one pi-sulfur, one amide-pi, and eight alkyl bonds with a score of −7.8 kcal/mol. However, with a score of −7.8 kcal/mol, Diclofenac interacted through one conventional hydrogen, two pi-sigma, one amide-pi, and two alkyl bonds. Considering the binding score, other metabolites, especially C9, C14, C18, and C21, scored lower than −7 kcal/mol by forming multiple interactions with the receptor (Table 7; Figure 8).
Figure 8. Molecular Interactions of Phytochemicals with COX-2 and TNF-α Enzymes: (I) Graphical representation of the molecular interactions of the most prominent phytocompounds with the COX-2 enzyme with 3D visualization (Compound 9 = A, Compound 18 = B, Compound 19 = C, Compound 23 = D, and Standard Diclofenac = E). (II) Graphical representation of the molecular interactions of the most prominent phytocompounds with the TNF-α enzyme with 3D visualization (Compound 8 = A, Compound 9 = B, Compound 29 = C, Compound 23 = D, and Standard Diclofenac = E).
Infection or injury triggers inflammation as a physiological response, marked by acute inflammation characterized by cytokines and neutrophils. Chronic inflammation, which involves additional immune cells, is linked to various diseases, including cancer. In the context of inflammation, TNF-α plays a pivotal role by activating nuclear factor kappa B (NF-kB), leading to the expression of various inflammatory genes (Sethi et al., 2008). With remarkable binding affinity. In our study, C19 exhibited the highest efficacy against TNF- α, interacting with the receptor through one pi-pi and three alkyl bonds. Diclofenac (−7.1 kcal/mol) is bound with one conventional hydrogen bond and two pi-pi bonds. Notably, C8, C9, C18, and C23 significantly decreased docking scores compared to the standard diclofenac (Table 7; Figure 8). This suggests the potential for a potent anti-inflammatory effect from the crude extract of the plant.
Given the observed affinities towards various receptors, it is plausible to propose that the mentioned metabolites play a significant role by modulating the many biological impacts of the leaf extract. This highlights the possible significance of these chemicals in the overall pharmacological activity of the extract. Moreover, it is advisable to investigate these substances in future studies better to understand their distinct contributions and prospective therapeutic uses. Interestingly, C12, C18, and C23 exhibited significant binding efficacy with multiple receptors associated with particular disease conditions, highlighting their potential versatility across various disease states. However, computational ADME/T analysis of most active compounds towards those receptors is represented in Tables 8 and Table 9, where C2, C4, and C19 stand out for their excellent oral bioavailability as they only violate a single Rule of Five. Conversely, C18 and C23 present challenges by contravening three rules. However, most of the metabolites in Table 9, while violating two rules, still maintain a commendable level of oral bioavailability. Notably, C19 is the sole compound showing a potential for AMES toxicity, suggesting a need for caution due to potential carcinogenicity. On a positive note, all metabolites, except C4, exhibit negative hepatotoxicity, increasing their attractiveness as potential drug candidates.
Table 8. Absorption, Distribution, and Metabolism profile of the selected compounds that showed the best interaction against these receptors.
Table 9. Excretion, Toxicology and Drug-likeliness profile of the selected compounds that showed the best interaction against these receptors.
Upon phytochemical analysis and biological investigations of different fractionated extracts of C. affinis, the AQSF showed the most potent antioxidant, cytotoxic, and antimicrobial attributes among the other three. In contrast, the DCMSF exhibited the most potent antidiarrheal and analgesic activities among the others. The EASF also demonstrated notable activities in antioxidant, cytotoxicity, and antimicrobial tests. Upon GC-MS analysis of all four fractions, a total of 34 metabolites were found in AQSF, 20 metabolites in DCM, 7 metabolites in HSF, and 6 metabolites in EASF. At the same time, phytochemical screening showed the presence of different chemical classes. The obtained metabolites possess drug-like properties, which can account for the documented pharmacological actions. Computer-aided techniques also corroborate the role of these substances in producing these effects. Based on the in-vitro, in-vivo, GC-MS, and in silico observations of this study, it can be concluded that, the fractionated extracts, especially the aqueous soluble fraction (AQSF) of the vegetable C. affinis demand further extensive scientific research for the isolation of its phytochemicals and determination of their mode of action to employ medicinal actions along with their safety profiles.
The raw data supporting the conclusions of this article will be made available by the authors, without undue reservation.
The animal study was approved by Animal Ethics Committee, State University of Bangladesh. The study was conducted in accordance with the local legislation and institutional requirements.
SA: Conceptualization, Data curation, Investigation, Methodology, Software, Writing–original draft, Writing–review and editing. FR: Data curation, Formal Analysis, Investigation, Methodology, Software, Validation, Writing–original draft, Writing–review and editing. HH: Data curation, Investigation, Project administration, Visualization, Writing–review and editing, Writing–original draft. FA: Formal Analysis, Visualization, Writing–review and editing. NE: Data curation, Software, Visualization, Writing–review and editing. MU: Data curation, Formal Analysis, Investigation, Writing–review and editing. GR: Data curation, Formal Analysis, Investigation, Writing–review and editing. SW: Formal Analysis, Funding acquisition, Writing–review and editing. MY: Formal Analysis, Writing–review and editing. NA: Formal Analysis, Visualization, Writing–review and editing. MK: Software, Visualization, Writing–review and editing, AA: Conceptualization, Formal Analysis, Funding acquisition, Supervision, Validation, Visualization, Writing - original draft, Writing–review and editing.
The author(s) declare that financial support was received for the research, authorship, and/or publication of this article. This work was funded by the Bangladesh Council of Scientific and Industrial Research (BCSIR) as an R&D project work of the 2022-23 fiscal year, Reference No: 39.02.0000.011.14.157.2022/172 (Date: 10.11.2022) and partially supported by the Zhejiang Provincial Traditional Chinese Medicine Science and Technology funds (2024ZL1302).
The authors declare that the research was conducted in the absence of any commercial or financial relationships that could be construed as a potential conflict of interest.
All claims expressed in this article are solely those of the authors and do not necessarily represent those of their affiliated organizations, or those of the publisher, the editors and the reviewers. Any product that may be evaluated in this article, or claim that may be made by its manufacturer, is not guaranteed or endorsed by the publisher.
HSF, hexane soluble fraction; DCMSF, dichloromethane soluble fraction; EASF, ethyl acetate soluble fraction; AQSF, aqueous soluble fraction; BHT, butylated hydroxytoluene; VS, vincristine; ROS, reactive oxygen species; COX 1, cyclooxygenase-1; COX 2, cyclooxygenase-2; IC50, half maximal inhibitory concentration; LC50, lethal concentration 50; DPPH, 2,2-diphenyl-1-picryl-hydrazyl hydrate; GC-MS, Gas Chromatography-Mass Spectrometry; BCL-2, B-cell lymphoma 2; DHFR, Dihydrofolate reductase; DOR, Delta opioid receptors; EGFR, Epidermal growth factor receptor; GLR, Glutamate Receptors; KAS, ketoaryl-ACP synthase; KOR, kappa-opioid receptor; TNF-α, Tumor necrosis factor-alpha; URO, Urate oxidase.
Abbafati, C., Abbas, K. M., Abbasi, M., Abbasifard, M., Abbasi-Kangevari, M., Abbastabar, H., et al. (2020). Global burden of 369 diseases and injuries in 204 countries and territories, 1990–2019: a systematic analysis for the Global Burden of Disease Study 2019. Lancet. 396 (10258), 1204–1222. doi:10.1016/S0140-6736(20)30925-9
Ahmad, N. S., Waheed, A., Farman, M., and Qayyum, A. (2010). Analgesic and anti-inflammatory effects of Pistacia integerrima extracts in mice. J. Ethnopharmacol. 129 (2), 250–253. doi:10.1016/j.jep.2010.03.017
Alam, S., Emon, N. U., Shahriar, S., Richi, F. T., Haque, M. R., Islam, M. N., et al. (2020). Pharmacological and computer-aided studies provide new insights into Millettia peguensis Ali (Fabaceae). Saudi Pharm. J. 28 (12), 1777–1790. doi:10.1016/j.jsps.2020.11.004
Alam, S., Rashid, M. A., Sarker, M. M. R., Emon, N. U., Arman, M., Mohamed, I. N., et al. (2021a). Antidiarrheal, antimicrobial and antioxidant potentials of methanol extract of Colocasia gigantea Hook. f. leaves: evidenced from in vivo and in vitro studies along with computer-aided approaches. BMC Complement. Med. Ther. 21 (1), 119. doi:10.1186/s12906-021-03290-6
Alam, S., Sarker, M. M. R., Afrin, S., Richi, F. T., Zhao, C., Zhou, J. R., et al. (2021b). Traditional herbal medicines, bioactive metabolites, and plant products against COVID-19: update on clinical trials and mechanism of actions. Front. Pharmacol. 12 (May), 671498. doi:10.3389/fphar.2021.671498
Ambriz-Pérez, D. L., Leyva-López, N., Gutierrez-Grijalva, E. P., and Heredia, J. B. (2016). Phenolic compounds: natural alternative in inflammation treatment. A Review. Cogent Food Agric. 2 (1). doi:10.1080/23311932.2015.1131412
Andrade, T. C. B., Lima, S. G. D., Freitas, R. M., Rocha, M. S., Islam, T., Silva, T. G. D., et al. (2015). Isolation, characterization and evaluation of antimicrobial and cytotoxic activity of estragole, obtained from the essential oil of croton zehntneri (euphorbiaceae). Acad Bras Cienc 87 (1), 173–182. doi:10.1590/0001-3765201520140111
Alonso, A. M., Castro, R., Rodrıguez, M. C., Guillén, D. A., and Barroso, C. G. (2004). Study of the antioxidant power of brandies and vinegars derived from Sherry wines and correlation with their content in polyphenols. Int. Food Res. 37 (7), 715–721. doi:10.1016/j.foodres.2004.03.007
El Azab, I. H., El-Sheshtawy, H. S., Bakr, R. B., and Elkanzi, N. A. (2021). New 1, 2, 3-triazole-containing hybrids as antitumor candidates: Design, click reaction synthesis, DFT calculations, and molecular docking study. Molecules 26 (3), 708. doi:10.3390/molecules26030708
Aziz, M., Karim, A., Tab, N., Mekhfi, H., Bnouham, M., Ziyyat, A., et al. (2011). Antidiarrhoeal activity of Cistus ladaniferus aqueous extract. Spat. DD - Peer Rev. J. Complement. Med. Drug Discov. 1 (3), 175. doi:10.5455/spatula.20110908034958
Bakun, P., Czarczynska-Goslinska, B., Goslinski, T., and Lijewski, S. (2021). In vitro and in vivo biological activities of azulene derivatives with potential applications in medicine. Med. Chem. Res. 30 (4), 834–846. doi:10.1007/s00044-021-02701-0
Balachandran, A., Choi, S. B., Beata, M. M., Małgorzata, J., Froemming, G. R. A., Lavilla, C. A., et al. (2023). Antioxidant, wound healing potential and in silico assessment of naringin, eicosane and octacosane. Molecules 28 (3), 1043. doi:10.3390/molecules28031043
Beserra, F. P., de Cássia Santos, R., Périco, L. L., Rodrigues, V. P., de Almeida Kiguti, L. R., Saldanha, L. L., et al. (2016). Cissus sicyoides: pharmacological mechanisms involved in the anti-inflammatory and antidiarrheal activities. Int. J. Mol. Sci. 17 (2), 149. doi:10.3390/ijms17020149
Bhat, R. S., and Al-Daihan, S. (2014). Phytochemical constituents and antibacterial activity of some green leafy vegetables. Asian Pac J. Trop. Biomed. 4 (3), 189–193. doi:10.1016/S2221-1691(14)60230-6
Brand-Williams, W., Cuvelier, M. E., and Berset, C. (1995). Use of a free radical method to evaluate antioxidant activity. LWT - Food Sci. Technol. 28 (1), 25–30. doi:10.1016/s0023-6438(95)80008-5
Carter, G. T., Duong, V., Ho, S., Ngo, K. C., Greer, C. L., and Weeks, D. L. (2014). Side effects of commonly prescribed analgesic medications. Phys. Med. Rehabil. Clin. N. Am. 25 (2), 457–470. doi:10.1016/j.pmr.2014.01.007
Che, T., English, J., Krumm, B. E., Kim, K., Pardon, E., Olsen, R. H., et al. (2020). Nanobody-enabled monitoring of kappa opioid receptor states. Nat. Commun. 11, 1145. doi:10.1038/s41467-020-14889-7
Cleveland, B. M., Leonard, S. S., Klandorf, H., and Blemings, K. P. (2009). Urate oxidase knockdown decreases oxidative stress in a murine hepatic cell line. Oxid. Med. Cell Longev. 2, 93–98. doi:10.4161/oxim.2.2.8372
Darby, E. M., Trampari, E., Siasat, P., Gaya, M. S., Alav, I., Webber, M. A., et al. (2023). Molecular mechanisms of antibiotic resistance revisited. Nat. Rev. Microbiol. 21 (5), 280–295. doi:10.1038/s41579-022-00820-y
Dev, S., Acharyya, R. N., Shill, M. C., Bari, M. A., Asma, K., Mahmud, I., et al. (2021). Bioactivities of Colocasia affinis Schott and profiling of its bioactive polyphenols by HPLC-DAD. Bangladesh Pharm. J. 24 (1), 1–10. doi:10.3329/bpj.v24i1.51629
De Vincenzi, M., Silano, M., Maialetti, F., and Scazzocchio, B. (2000). Constituents of aromatic plants: II. Estragole. Estragole Fitoter. 71 (6), 725–729. doi:10.1016/s0367-326x(00)00153-2
Donati, M., Mondin, A., Chen, Z., Miranda, F. M., Do Nascimento, B. B., Schirato, G., et al. (2015). Radical scavenging and antimicrobial activities of Croton zehntneri, Pterodon emarginatus and Schinopsis brasiliensis essential oils and their major constituents: estragole, trans-anethole, β-caryophyllene and myrcene. Nat. Prod. Res. 29 (10), 939–946. doi:10.1080/14786419.2014.964709
Edeoga, H. O., Okwu, D. E., and Mbaebie, B. O. (2005). Phytochemical constituents of some Nigerian medicinal plants. Afr. J. Biotechnol. 4 (7), 685–688. doi:10.5897/ajb2005.000-3127
Epand, R. M., and Vogel, H. J. (1999). Diversity of antimicrobial peptides and their mechanisms of action. Biochim. Biophys. Acta - Biomembr. 1462 (1–2), 11–28. doi:10.1016/s0005-2736(99)00198-4
Fenalti, G., Zatsepin, N. A., Betti, C., Giguere, P., Han, G. W., Ishchenko, A., et al. (2015). Structural basis for bifunctional peptide recognition at human ? opioid receptor. Nat. Struct. Mol. Biol. 22 (3), 265–268. doi:10.1038/nsmb.2965
Ghannoum, M. A., and Rice, L. B. (1999). Antifungal agents: mode of action, mechanisms of resistance, and correlation of these mechanisms with bacterial resistance. Clin. Microbiol. Rev. 12 (4), 501–517. doi:10.1128/CMR.12.4.501
Gupta, K., Kumar, A., Tomer, V., Kumar, V., and Saini, M. (2019). Potential of Colocasia leaves in human nutrition: review on nutritional and phytochemical properties. J. Food Biochem. 43 (7), e12878. doi:10.1111/jfbc.12878
Guzow-Krzemińska, B., Guzow, K., and Herman-Antosiewicz, A. (2019). Usnic acid derivatives as cytotoxic agents against cancer cells and the mechanisms of their activity. Curr. Pharmacol. Rep. 5, 429–439. doi:10.1007/s40495-019-00202-8
Hadi, I., and Hussein, H. M. (2016). Antimicrobial Activity and spectral chemical analysis of methanolic leaves extract of Adiantum Capillus-Veneris using GC-MS and FT-IR spectroscopy. Int. J. Pharmacogn. Phytochem. Res. 8 (3), 369–385.
Hamzah, R., Jigam, A., Makun, H., and Egwim, E. (2014). Phytochemical screening and invitro antioxidant activity of methanolic extract of selected nigerian vegetables. Asian J. Basis Appl. Sci. 1 (1), 1–14.
Harborne, A. J. (1998). Phytochemical methods a guide to modern techniques of plant analysis. springer science & business media. Ethnoveterinary Botanical Medicine, 59–84.
Hasnat, H., Shompa, S. A., Islam, M. M., Alam, S., Richi, F. T., Emon, N. U., et al. (2024). Flavonoids: a treasure house of prospective pharmacological potentials. Heliyon 10, e27533. doi:10.1016/j.heliyon.2024.e27533
Hasnat, H., Shompa, S. A., Richi, F. T., Islam, M. M., Suman, M. H., Ahmed, N. U., et al. (2023). Bioactive secondary metabolites to combat diabetic complications: evidenced from in silico study. Bangladesh Pharm. J. 26 (2), 167–184. doi:10.3329/bpj.v26i2.67807
Hayes, J. D., Dinkova-Kostova, A. T., and Tew, K. D. (2020). Oxidative stress in cancer. Cancer Cell 38 (2), 167–197. doi:10.1016/j.ccell.2020.06.001
He, J., Qiao, W., An, Q., Yang, T., and Luo, Y. (2020). Dihydrofolate reductase inhibitors for use as antimicrobial agents. Eur. J. Med. Chem. 195, 112268. doi:10.1016/j.ejmech.2020.112268
Huang, L., Zhu, X., Zhou, S., Cheng, Z., Shi, K., Zhang, C., et al. (2021). Phthalic acid esters: natural sources and biological activities. Toxins (Basel). 13, 495. doi:10.3390/toxins13070495
Huys, G., D’Haene, K., and Swings, J. (2002). Influence of the culture medium on antibiotic susceptibility testing of food-associated lactic acid bacteria with the agar overlay disc diffusion method. Lett. Appl. Microbiol. 34 (6), 402–406. doi:10.1046/j.1472-765x.2002.01109.x
Jendele, L., Krivak, R., Skoda, P., Novotny, M., and Hoksza, D. (2019). PrankWeb: a web server for ligand binding site prediction and visualization. Nucleic Acids Res. 47, W345–W349. doi:10.1093/nar/gkz424
Khatun, M. C. S., Muhit, M. A., Hossain, M. J., Al-Mansur, M. A., and Rahman, S. A. (2021). Isolation of phytochemical constituents from Stevia rebaudiana (Bert.) and evaluation of their anticancer, antimicrobial and antioxidant properties via in vitro and in silico approaches. Heliyon 7, e08475. doi:10.1016/j.heliyon.2021.e08475
Kim, S., Thiessen, P. A., Bolton, E. E., Chen, J., Fu, G., Gindulyte, A., et al. (2016). PubChem substance and compound databases. Nucleic Acids Res. 44, D1202–D1213. doi:10.1093/nar/gkv951
Klaunig, J. E. (2019). Oxidative stress and cancer. Curr. Pharm. Des. 24 (40), 4771–4778. doi:10.2174/1381612825666190215121712
Kodidela, S., Pradhan, S. C., Muthukumaran, J., Dubashi, B., Santos-Silva, T., and Basu, D. (2016). Genotype distribution of dihydrofolatereductase variants and their role in disease susceptibility to acute lymphoblastic leukemia in {I}ndian population: an experimental and computational analysis. J. Leuk. 4, 209.
Kumar, P. S., Krishnaswamy, G., Desai, N. R., Sreenivasa, S., and Kumar, D. A. (2021). Design, synthesis, PASS prediction, in-silico ADME and molecular docking studies of substituted-(Z)-3-benzylidine-5-aza-2-oxindole derivatives (Part-1). Chem. Data Collect 31, 100617. doi:10.1016/j.cdc.2020.100617
Lalremruati, C., Malsawmtluangi, C., and Lalhlenmawia, H. (2018). Phytochemical screening and evaluation of antidepressant activity of methanolic extract of the spadix of Colocasia affinis Schott. Sci. Vis. 18 (4), 104–110. doi:10.33493/scivis.18.04.01
Levine, A. C., Gainey, M., Qu, K., Nasrin, S., Sharif, M. B. E., Noor, S. S., et al. (2023). A comparison of the NIRUDAK models and WHO algorithm for dehydration assessment in older children and adults with acute diarrhoea: a prospective, observational study. Lancet Glob. Heal 11 (11), e1725–e1733. doi:10.1016/S2214-109X(23)00403-5
Liu, R. H. (2007). Whole grain phytochemicals and health. J. Cereal Sci. 46 (3), 207–219. doi:10.1016/j.jcs.2007.06.010
Mannervik, B. (1999). Measurement of glutathione reductase activity. Curr. Protoc. Toxicol. 1, 2–7. doi:10.1002/0471140856.tx0702s00
Maridass, M., and De Britto, A. J. (2008). Origins of plant derived medicines. Ethnobot. Leafl. 12, 373–387.
Medaowe, H., Ali, A., and Dissertation, A. (2020). May god bless her and curse her physico-chemical properties, gas chromatography-mass spectrometric analysis and antioxidant activity of um wedeka (ximenia americana.L) seed oil. (December).
Mekonnen, S. A., Merenstein, D., Fraser, C. M., and Marco, M. L. (2020). Molecular mechanisms of probiotic prevention of antibiotic-associated diarrhea. Curr. Opin. Biotechnol. 61, 226–234. doi:10.1016/j.copbio.2020.01.005
Meyer, B. N., Ferrigni, N. R., Putnam, J. E., Jacobsen, L. B., Nichols, D. E., and McLaughlin, J. L. (1982). Brine shrimp: a convenient general bioassay for active plant constituents. Planta Med. 45 (1), 31–34. doi:10.1055/s-2007-971236
Mondal, M., Hossain, M. S., Das, N., Khalipha, A. B. R., Sarkar, A. P., Islam, M. T., et al. (2019). Phytochemical screening and evaluation of pharmacological activity of leaf Methanolic extract of Colocasia affinis Schott. Clin. Phytoscience 5 (1), 8. doi:10.1186/s40816-019-0100-8
Morvaridzadeh, M., Nachvak, S. M., Agah, S., Sepidarkish, M., Dehghani, F., Rahimlou, M., et al. (2020). Effect of soy products and isoflavones on oxidative stress parameters: a systematic review and meta-analysis of randomized controlled trials. Food Res. Int. 137, 109578. doi:10.1016/j.foodres.2020.109578
Mousa, T. H., Rasool, M. I., and Ali, A. (2022). Isolated chemical compounds from natural origin with anti-inflammatory effect.
Muhammad, N., Shrestha, L., Adhikari, A., Wadood, A., Khan, H., Khan, A. Z., et al. (2015). First evidence of the analgesic activity of govaniadine, an alkaloid isolated from Corydalis govaniana Wall. Nat. Prod. Res. 29 (5), 430–437. doi:10.1080/14786419.2014.951933
Naczk, M., and Shahidi, F. (2006). Phenolics in cereals, fruits and vegetables: occurrence, extraction and analysis. J. Pharm. Biomed. Anal. 41 (5), 1523–1542. doi:10.1016/j.jpba.2006.04.002
Nakaziba, R., Anyolitho, M. K., Amanya, S. B., Sesaazi, C. D., Byarugaba, F., Ogwal-Okeng, J., et al. (2021). Traditional medicinal vegetables in northern Uganda: an ethnobotanical survey. Int. J. Food Sci. 2021, 5588196. doi:10.1155/2021/5588196
Nascimento, G. G. F., Locatelli, J., Freitas, P. C., and Silva, G. L. (2000). Antibacterial activity of plant extracts and phytochemicals on antibiotic-resistant bacteria. Braz. J. Microbiol. 31 (4), 247–256. doi:10.1590/s1517-83822000000400003
Nicholson, R. I., Gee, J. M. W., and Harper, M. E. (2001). EGFR and cancer prognosis. Eur. J. Cancer 37, 9–15. doi:10.1016/s0959-8049(01)00231-3
Nurdiansyah, R., Putra, A. B. N., Nadhifah, A., Chriscensia, E., Kusumo, U. K. G., Yosiano, S. A., et al. (2023). Cytotoxic activity of Indonesian Pogonatum neesii dozy from cibodas botanical garden: in silico molecular docking and in vitro evaluation. Pharm. Sci. 29 (4), 448–458. doi:10.34172/ps.2023.11
Obaidullah, A. J., Alanazi, M. M., Alsaif, N. A., Mahdi, W. A., Fantoukh, O. I., Tareq, A. M., et al. (2021). Deeper insights on cnesmone javanica blume leaves extract: chemical profiles, biological attributes, network pharmacology and molecular docking. Plants 10, 728. doi:10.3390/plants10040728
Octarya, Z., Novianty, R., Suraya, N., and Saryono, S. (2021). Antimicrobial activity and GC-MS analysis of bioactive constituents of Aspergillus fumigatus 269 isolated from sungai pinang hot spring, riau, Indonesia. Biodiversitas 22 (4), 1839–1845. doi:10.13057/biodiv/d220429
Okechukwu, P. N. (2020). Evaluation of anti-inflammatory, analgesic, antipyretic effect of eicosane, pentadecane, octacosane, and heneicosane. Asian J. Pharm. Clin. Res. 13 (4), 29–35. doi:10.22159/ajpcr.2020.v13i4.36196
Omoigui, S. (2007). The biochemical origin of pain: the origin of all pain is inflammation and the inflammatory response. Part 2 of 3 - inflammatory profile of pain syndromes. Med. Hypotheses 69 (6), 1169–1178. doi:10.1016/j.mehy.2007.06.033
Ongko, J., Setiawan, J. V., Feronytha, A. G., Juliana, A., Effraim, A., Wahjudi, M., et al. (2022). June. In-silico Screen Inhib protein epidermal growth factor Recept (EGFR). IOP Conf. Ser. Earth Environ. Sci. 1041, 1.
Pannemans, J., and Corsetti, M. (2018). Opioid receptors in the GI tract: targets for treatment of both diarrhea and constipation in functional bowel disorders? Curr. Opin. Pharmacol. 43, 53–58. doi:10.1016/j.coph.2018.08.008
Park, E. S., Moon, W. S., Song, M. J., Kim, M. N., Chung, K. H., and Yoon, J. S. (2001). Antimicrobial activity of phenol and benzoic acid derivatives. Int. Biodeterior. Biodegr. 47 (4), 209–214. doi:10.1016/s0964-8305(01)00058-0
Phull, A. R., Ahmed, M., and Park, H. J. (2022). Cordyceps militaris as a bio functional food source: pharmacological potential, anti-inflammatory actions and related molecular mechanisms. Microorganisms 10 (2), 405. doi:10.3390/microorganisms10020405
Pornprasertpol, A., Sereemaspun, A., Sooklert, K., Satirapipatkul, C., and Sukrong, S. (2015). Anticancer activity of selected Colocasia gigantia fractions. J. Med. Assoc. Thail. 98, S98–S106.
Prlić, A., Bliven, S., Rose, P. W., Bluhm, W. F., Bizon, C., Godzik, A., et al. (2010). Pre-calculated protein structure alignments at the RCSB PDB website. Bioinformatics 26 (23), 2983–2985. doi:10.1093/bioinformatics/btq572
Qiu, X., Janson, C. A., Smith, W. W., Head, M., Lonsdale, J., and Konstantinidis, A. K. (2001). Refined structures of ? ketoacyl-acyl carrier protein synthase III. J. Mol. Biol. 307 (1), 341–356. doi:10.1006/jmbi.2000.4457
Rahman, M. A., Sultana, R., Emran, B., T, I., R, M. S., A. C, M., et al. (2013). U hasan, CMM eff org extr six Bangladeshi plants vitr thrombolysis cytotoxicity. BMC Complement. Altern. Med. 13, 1–7. doi:10.1186/1472-6882-13-25
Rajlic, S., Treede, H., Münzel, T., Daiber, A., and Duerr, G. D. (2023). Early detection is the best prevention—characterization of oxidative stress in diabetes mellitus and its consequences on the cardiovascular system. Cells 12 (4), 583. doi:10.3390/cells12040583
Retailleau, P., Colloc’h, N., Vivarès, D., Bonneté, F., Castro, B., El, H. M., et al. (2004). Complexed and ligand-free high-resolution structures of urate oxidase (Uox) from Aspergillus flavus: a reassignment of the active-site binding mode. Acta Crystallogr. Sect. D. Biol. Crystallogr. 60 (3), 453–462. doi:10.1107/S0907444903029718
Rudra, S., Sawon, M. S. U., Emon, N. U., Alam, S., Tareq, S. M., Islam, M. N., et al. (2020). Biological investigations of the methanol extract of tetrastigma leucostaphylum (Dennst.) alston ex mabb. (vitaceae): in vivo and in vitro approach. J. Adv. Biotechnol. Exp. Ther. 3 (3), 216–224. doi:10.5455/jabet.2020.d127
Sethi, G., Sung, B., and Aggarwal, B. B. (2008). TNF: a master switch for inflammation to cancer. Front. Biosci. 13 (2), 5094–5107. doi:10.2741/3066
Shahriar, S., Shermin, S. A., Hasnat, H., Hossain, F., Han, A., Geng, P., et al. (2024). Chemico-pharmacological evaluation of the methanolic leaf extract of Catharanthus ovalis: GC--MS/MS, in vivo, in vitro, and in silico approaches. Front. Pharmacol. 15, 1347069. doi:10.3389/fphar.2024.1347069
Sher, A. (2009). Antimicrobial activity of natural products from medicinal plants. Gjms 7 (1), 72–78.
Shoba, F. G., and Thomas, M. (2001). Study of antidiarrhoeal activity of four medicinal plants in castor-oil induced diarrhoea. J. Ethnopharmacol. 76 (1), 73–76. doi:10.1016/s0378-8741(00)00379-2
Shompa, S. A., Hasnat, H., Riti, S. J., Islam, M. M., Alam, S., Shao, C., et al. (2024). Phyto-pharmacological evaluation and characterization of the methanolic extract of the Baccaurea motleyana Müll. Arg. seed: promising insights into its therapeutic uses. Arg. seed Promis. insights into its Ther. uses Front. Pharmacol. 15, 1359815. doi:10.3389/fphar.2024.1359815
Singh, V., Mani, I., Chaudhary, D. K., and Somvanshi, P. (2011). The β-ketoacyl-ACP synthase from Mycobacterium tuberculosis as potential drug targets. Mycobacterium Tuberc. as Potential Drug Targets 18, 1318–1324. doi:10.2174/092986711795029636
Škerget, M., Kotnik, P., Hadolin, M., Hraš, A. R., Simonič, M., and Knez, Ž. (2005). henols, proanthocyanidins, flavones and flavonols in some plant materials and their antioxidant activities. Food chem. 89 (2), 191–198. doi:10.1016/j.foodchem.2004.02.025
Sudhakar, P., Thenmozhi, V., Srivignesh, S., and Dhanalakshmi, M. (2020). Colocasia esculenta (L.) Schott: pharmacognostic and pharmacological review. J. Pharmacogn. Phytochem. 9 (4), 1382–1386. doi:10.22271/phyto.2020.v9.i4s.11937
Taher, M. A., Laboni, A. A., Islam, M. A., Hasnat, H., Hasan, M. M., Ferdous, J., et al. (2024). Isolation, characterization and pharmacological potentials of methanol extract of Cassia fistula leaves: evidenced from mice model along with molecular docking analysis. Heliyon 10, e28460. doi:10.1016/j.heliyon.2024.e28460
Taher, M. A., Laboni, A. A., Shompa, S. A., Rahman, M. M., Hasan, M. M., Hasnat, H., et al. (2023). Bioactive compounds extracted from leaves of G. Cyanocarpa using various solvents in chromatographic separation showed anti-cancer and anti-microbial potentiality in in silico approach. Chin. J. Anal. Chem. 1003. doi:10.1016/j.cjac.2023.100336
Thakur, A. (2018). Health promoting phytochemicals in vegetables: a mini review. Int. J. Food Ferment Technol. 8 (2). doi:10.30954/2277-9396.02.2018.1
Tiwari, G., Chaturvedi, T., Kumar Gupta, A., Kishori Lal, R., Swaroop Verma, R., Kumar Srivastava, R., et al. (2023). Assessment of genetic diversity, micromorphology and antimicrobial activity in nepeta cataria L. Chem. Biodivers. 20 (2), e202200241. doi:10.1002/cbdv.202200241
Uddin, S. J., Grice, D., and Tiralongo, E. (2012). Evaluation of cytotoxic activity of patriscabratine, tetracosane and various flavonoids isolated from the Bangladeshi medicinal plant Acrostichum aureum. Pharm. Biol. 50 (10), 1276–1280. doi:10.3109/13880209.2012.673628
Vanitha, V., Vijayakumar, S., Nilavukkarasi, M., Punitha, V. N., Vidhya, E., and Praseetha, P. K. (2020). Heneicosane—a novel microbicidal bioactive alkane identified from Plumbago zeylanica L. Ind. Crops Prod. 154 (June), 112748. doi:10.1016/j.indcrop.2020.112748
VanWagenen, B. C., Larsen, R., Cardellina, J. H., Randazzo, D., Lidert, Z. C., and Swithenbank, C. (1993). Ulosantoin, a potent insecticide from the sponge ulosa ruetzleri. J. Org. Chem. 58 (2), 335–337. doi:10.1021/jo00054a013
Vijayakumar, K., and MuhilVannan, S. (2021). 3, 5-Di-tert-butylphenol combat against Streptococcus mutans by impeding acidogenicity, acidurance and biofilm formation. World J. Microbiol. Biotechnol. 37, 202. doi:10.1007/s11274-021-03165-5
Webb, A. L., and Villamor, E. (2007). Update: effects of antioxidant and non-antioxidant vitamin supplementation on immune function. Nutr. Rev. 65 (5), 181–217. doi:10.1111/j.1753-4887.2007.tb00298.x
Williams, C. S., Mann, M., and Dubois, R. N. (1999). The role of cyclooxygenases in inflammation, cancer, and development. cancer , Dev. 18, 7908–7916. doi:10.1038/sj.onc.1203286
Wojdyło, A., Oszmiański, J., and Czemerys, R. (2007). Antioxidant activity and phenolic compounds in 32 selected herbs. Food Chem. 105 (3), 940–949. doi:10.1016/j.foodchem.2007.04.038
Wolfender, J. L., Eugster, P. J., Bohni, N., and Cuendet, M. (2011). Advanced methods for natural product drug discovery in the field of nutraceuticals. Chim. (Aarau). 65 (6), 400–406. doi:10.2533/chimia.2011.400
Yip, K. W., and Reed, J. C. (2008). Bcl-2 family proteins and cancer. Oncogene 27 (50), 6398–6406. doi:10.1038/onc.2008.307
Zhao, F., Wang, P., Lucardi, R. D., Su, Z., and Li, S. (2020). Natural sources and bioactivities of 2, 4-di-tert-butylphenol and its analogs. Toxins (Basel). 12, 35. doi:10.3390/toxins12010035
Keywords: Colocasia affinis, in vitro, antioxidant, cytotoxicity, antimicrobial, in vivo, antidiarrheal, analgesic
Citation: Alam S, Richi FT, Hasnat H, Ahmed F, Emon NU, Uddin MJ, Rana GMM, Wang S, Yeasmin MS, Ahmed NU, Khan MS and Al Mamun A (2024) Chemico-pharmacological evaluations of the dwarf elephant ear (Colocasia affinis Schott) plant metabolites and extracts: health benefits from vegetable source. Front. Pharmacol. 15:1428341. doi: 10.3389/fphar.2024.1428341
Received: 06 May 2024; Accepted: 26 June 2024;
Published: 13 August 2024.
Edited by:
Paucean Adriana, University of Agricultural Sciences and Veterinary Medicine of Cluj-Napoca, RomaniaReviewed by:
Snežana Filip, University of Novi Sad, SerbiaCopyright © 2024 Alam, Richi, Hasnat, Ahmed, Emon, Uddin, Rana, Wang, Yeasmin, Ahmed, Khan and Al Mamun. This is an open-access article distributed under the terms of the Creative Commons Attribution License (CC BY). The use, distribution or reproduction in other forums is permitted, provided the original author(s) and the copyright owner(s) are credited and that the original publication in this journal is cited, in accordance with accepted academic practice. No use, distribution or reproduction is permitted which does not comply with these terms.
*Correspondence: Abdullah Al Mamun, cGhhcm1hYWxtYW11bkB5YWhvby5jb20=
†ORCID: Safaet Alam, orcid.org/0000-0002-1831-2278; Fahmida Tasnim Richi, orcid.org/0000-0002-7271-4219; Hasin Hasnat, https://orcid.org/0000-0001-5319-1398; Firoj Ahmed, orcid.org/0000-0003-0690-1451; Nazim Uddin Emon, orcid.org/0000-0001-7567-4796; Md. Jasim Uddin, orcid.org/0000-0001-7637-9457; GM Masud Rana, orcid.org/0009-0007-1977-3096; Shuanghu Wang, orcid.org/0000-0002-0057-267X; Mst. Sarmina Yeasmin, orcid.org/0000-0002-2927-385X; Abdullah Al Mamun, orcid.org/0000-0001-9936-3925
Disclaimer: All claims expressed in this article are solely those of the authors and do not necessarily represent those of their affiliated organizations, or those of the publisher, the editors and the reviewers. Any product that may be evaluated in this article or claim that may be made by its manufacturer is not guaranteed or endorsed by the publisher.
Research integrity at Frontiers
Learn more about the work of our research integrity team to safeguard the quality of each article we publish.